Commentary: Extracellular peptidase hunting for improvement of protein production in plant cells and roots
- 1Laboratory of Plant Physiology, Department of Life Sciences, University of Liège, Liège, Belgium
- 2PhytoSYSTEMS, University of Liège, Liège, Belgium
Plant-based recombinant protein production systems have gained an extensive interest over the past few years, because of their reduced cost and relative safety. Although the first products are now reaching the market, progress are still needed to improve plant hosts and strategies for biopharming. Targeting recombinant proteins toward the extracellular space offers several advantages in terms of protein folding and purification, but degradation events are observed, due to endogenous peptidases. This paper focuses on the analysis of extracellular proteolytic activities in two production systems: cell cultures and root-secretion (rhizosecretion), in Arabidopsis thaliana and Nicotiana tabacum. Proteolytic activities of extracellular proteomes (secretomes) were evaluated in vitro against two substrate proteins: bovine serum albumin (BSA) and human serum immunoglobulins G (hIgGs). Both targets were found to be degraded by the secretomes, BSA being more prone to proteolysis than hIgGs. The analysis of the proteolysis pH-dependence showed that target degradation was mainly dependent upon the production system: rhizosecretomes contained more peptidase activity than extracellular medium of cell suspensions, whereas variations due to plant species were smaller. Using class-specific peptidase inhibitors, serine, and metallopeptidases were found to be responsible for degradation of both substrates. An in-depth in silico analysis of genomic and transcriptomic data from Arabidopsis was then performed and led to the identification of a limited number of serine and metallo-peptidases that are consistently expressed in both production systems. These peptidases should be prime candidates for further improvement of plant hosts by targeted silencing.
Introduction
Since 25 years and the demonstration by Hiatt et al. (1989) that the plant secretory pathway was able to carry out the folding and the assembling of complex eukaryotic proteins such as antibodies, plants have emerged as potential alternative hosts for the production of biopharmaceuticals. The amazing versatility of plant-based systems that have been developed (about a 100 platforms Schillberg et al., 2013), together with the economic and safety advantages they offer, aroused great expectations for this technology known as “molecular pharming.” However, it is only recently (2012) that the first plant-produced biopharmaceutical, a glucocerebrosidase produced in carrot cells as a treatment for the Gaucher’s disease (Shaaltiel et al., 2007), has been approved by the US Food and Drug Administration. Several reasons explain this slow industrial and market uptake: the relatively low and variable yields compared to the gold standard Chinese hamster ovary (CHO) cells for the production of complex human proteins (Twyman et al., 2013), the negative perception and restrictions on genetically modified organisms (GMOs; Schillberg et al., 2013), and the absence of a comprehensive regulatory framework (Fischer et al., 2013). High yields and regulatory compliance are key prerequisites to transform molecular pharming into an industrial success. Thus, while technologies were initially designed for transgenic plants grown in open fields, recent researches are rather focused on systems with a higher containment, which not only reduces the risk of GMOs release in the environment but also leads to a better control of the growing and production conditions (Paul and Ma, 2011; Schillberg et al., 2013).
In this context, systems based on plant cell- or tissue-cultures have emerged. They are either cell suspension cultures, mainly but not limited to tobacco Bright Yellow-2 cells (BY-2), or hairy root cultures induced by Agrobacterium rhizogenes (Schillberg et al., 2013). Both strategies share the advantage of producing biomass faster than whole plant cultures. Moreover, the product is often secreted into the culture medium, making its recovery easier and cheaper than extraction from the biomass (Twyman et al., 2013). Somehow intermediate between suspension and whole plant cultures, ‘floating’ systems based on the use of whole organisms that are fully or partly in contact with a culture medium (micro algae, moss, or aquatic plants) also have the advantages of being fully contained and allowing the secretion-based recovery of the product (Cox et al., 2006; Decker et al., 2014; Mathieu-Rivet et al., 2014). It is also the case of the rhizosecretion strategy where roots of hydroponically growing plants produce and secrete the recombinant protein into the nutrient solution. Such a system was initially proposed by Borisjuk et al. (1999) and later developed by Ma and colleagues (Drake et al., 2002, 2003, 2009).
One major limitation of secretion-based systems comes from proteolytic events frequently observed on the products (Pillay et al., 2014), a problem that is well documented for antibody production (e.g., Sharp and Doran, 2001; Drake et al., 2003; Niemer et al., 2014). Extracellular peptidases were demonstrated to be responsible for these degradations in various production systems: cell suspensions (Sharp and Doran, 2001), leaves (Hehle et al., 2011), or (hairy-)roots (Sharp and Doran, 2001; Drake et al., 2009). The extent of degradation depends on organs or production systems (Drake et al., 2009), developmental stages (De Muynck et al., 2009), culture medium (Häkkinen et al., 2014), or plant species (Magy et al., 2014). Cell wall proteome analyses, mainly performed in Arabidopsis, revealed that peptidases represent more than 10% of the extracellular proteins (Albenne et al., 2013). Moreover, Goulet et al. (2012) showed by a genomic analysis of Arabidopsis, rice, and Nicotiana spp. that, consistently across these species, a large proportion of peptidases are predicted to be targeted to the extracellular space. Considering that little is known about the function or the substrate of apoplastic peptidases (van der Hoorn, 2008; Tsiatsiani et al., 2012), their amount and diversity represent a major obstacle to the use of secretion in molecular pharming. However, counteracting strategies such as co-secretion of a single peptidase inhibitor (Komarnytsky et al., 2006; Robert et al., 2013) or the silencing of a single peptidase gene (Kim et al., 2008a; Mandal et al., 2014) have already proven efficient. To be successful, these strategies rely on a prior knowledge of the proteolytic activities likely to lead, in the operated production system, to the degradation of the target recombinant protein.
In the present paper, we aimed at cross-comparing the extracellular proteolytic activities of two production systems, cell culture and rhizosecretion, set-up from two species, Arabidopsis thaliana and Nicotiana tabacum. We specifically addressed this question in the case of human immunoglobulin (hIgGs) production. We hypothesized that in silico analyses of genomic and transcriptomic data obtained from a model species such as Arabidopsis could be merged with experimental results obtained from biochemical assays with existing production systems to provide robust insights about the major peptidases that limit hIgGs yields.
Materials and Methods
Plant Cultures
Plants of A. thaliana cv. Columbia-0 (Col-0) and N. tabacum cv. Petit Havana SR1 were grown in hydroponics (Araponics, Belgium), at 20°C, with a relative humidity of 70%, a photoperiod of 10 h and a light intensity of 100 μmol m-2 s-1. Plant culture was adapted from Tocquin et al. (2003): seeds were sown on the top of seed-holders filled with a gel [0.5% PhytagelTM (Sigma-Aldrich, St. Louis, MO, USA), 5 mM CaCl2, 0.15% polyvinylpyrrolidone (MW 10,000)] and the nutrient solution was prepared with Flora Series fertilizers (FloraBloom, FloraMicro and FloraGro; GHE, France), 0.5 mL per liter each. The solution was renewed after 5 weeks of cultivation.
Rhizosecretome Harvesting
The direct analysis of the extracellular medium (EM) of hydroponically growing plants is hindered by the high dilution level of endogenous secreted proteins. Moreover, many extracellular proteins, including peptidases, are known to be weakly bound to the cell wall. In order to get a comprehensive overview of the peptidases that could be involved in the degradation of secreted recombinant proteins, we used a harvesting protocol adapted from cell wall proteomics studies (Boudart et al., 2005). 7 weeks after sowing, roots were briefly drained, harvested and weighed. The total fresh weight (FW) measured is comprised of the root biomass, the intercellular fluid and the adsorbed nutrient solution. In preliminary experiments, we estimated the drained FW after centrifugation (10 min, 2700 g) to be ~30 and ~80% of total FW for A. thaliana and N. tabacum, respectively. The water content was taken into account to calculate the volume of a NaCl stock solution that must be added to the fresh root to obtain a final concentration of 1 M. The root samples were then incubated for 1 h at 4°C in 1 M NaCl under strong agitation in order to recover extracellular compounds. Roots were thereafter centrifuged in a PierceTM Centrifuge Column (Thermo Scientific, Rockford, IL, USA) during 10 min at 2700 g and at 4°C. The collected samples, hereafter called rhizosecretomes, were either used freshly prepared or stored at –80°C until use.
Cell Cultures
Cells of A. thaliana cv. plant system biology-dark (PSB-D) were cultivated in a 250-mL Erlenmeyer flask containing 50 mL of a liquid medium [0.44% Linsmaier and Skoog Medium (Duchefa Biochemie, Haarlem, the Netherlands), 3% sucrose, 5.10-5% 1-naphthaleneacetic acid, 5.10-6% kinetin, pH 5.7 (KOH)] in the dark, at 25°C and under agitation at 90 rpm on a rotary shaker. Each week, fresh liquid medium was inoculated with a 10% inoculum of the former cell culture. Cells of N. tabacum cv. BY-2 were grown in the same conditions as A. thaliana cells, in an adapted culture medium [0.44% Murashige and Skoog salts (MP BIOMEDICALS, Solon, OH, USA), 3% sucrose, 0.02% KH2PO4, 2.5.10-4% thiamine, 5.10-3% myo-inositol, 2.10-5% 2,4-D, pH 5.8 (KOH)]. A 5% inoculum was used to inoculate fresh liquid medium each week. Both types of cells were kindly provided by Prof. Marc Boutry and his colleagues (Physiological Biochemistry Unit, Catholic University of Louvain, Louvain-la-Neuve, Belgium).
Extracellular Medium Harvesting
The EM of the 7-days-old Arabidopsis cell cultures was vacuum-filtered through a superposition of a glass fiber prefilter and a cellulose acetate filter, with a pore size of 0.2 μm (Sartorius Stedim Biotech GmbH, Goettingen, Germany); tobacco cell cultures were vacuum-filtered through three layers of Miracloth (Calbiochem; Merck KGaA, Darmstadt, Germany). The EM was either directly used or stored at –80°C.
Gelatin Zymography
Twenty micro liter of rhizosecretome or EM were incubated during 20 min at room temperature in a non-reducing loading buffer (1% SDS, 10% glycerol, 0.02% bromophenol blue, 60 mM Tris-HCl, pH 6.8, final concentrations) and loaded on a 10% polyacrylamide gel containing 0.05% gelatin. After an electrophoresis of 45 min at 180 V, the gel was washed 3 × 20 min in 2.5% Triton X-100 and incubated for 16 h in 10 mM MES, 0.1 mM ZnCl2, 5 mM CaCl2, 1% Triton X-100, pH 5.75 (KOH). The gel was then stained by colloidal Coomassie blue as described further.
Target Proteins in Vitro Degradation
Five micro liter of rhizosecretome or EM were incubated during 6 h at 25°C in a buffer at a fixed value of pH [100 mM glycine (for pH values between 2 and 4.5) or 100 mM Tris (for pH values between 5 and 9), 100 mM MES, 0.1 mM ZnCl2, 5 mM CaCl2, 0.1 M dithiothreitol], with 1 μg bovine serum albumin (BSA; A7906, ≥98% purity, Sigma-Aldrich, St. Louis, MO, USA) or 1 μg immunoglobulin G from human serum (hIgGs; I4506, ≥95% purity, Sigma-Aldrich, St. Louis, MO, USA), in a final volume of 20 μL. Inactivation of peptidase classes was obtained by pre-incubating the rhizosecretome or EM in a buffer during 30 min at room temperature, with class-specific inhibitors: 5 mM PMSF (specific inhibitor of serine peptidases), 40 μM E-64 (specific inhibitor of cysteine peptidases), 16 μM Pepstatin A (specific inhibitor of aspartic peptidases) and 20 mM EDTA (specific inhibitor of metalloproteases). Mixes of all but one inhibitors were used, leaving only one peptidase class active per sample. 1 μg of BSA or human IgG was then added and incubated during 3 h (rhizosecretome) or 6 h (EM) at 25°C. After incubation, the target protein degradation profile was analyzed by SDS-PAGE and colloidal Coomassie blue staining.
SDS-Page and Colloidal Coomassie Blue Staining
Protein samples were incubated during 10 min at 95°C in a reducing loading buffer (0.1 M dithiothreitol, 1% SDS, 10% glycerol, 0.02% bromophenol blue, 60 mM Tris-HCl, pH 6.8, final concentrations) before being separated by electrophoresis in a 10% polyacrylamide gel during 45 min at 180 V. The gel was then washed briefly in water, fixed during 1 h at room temperature in a fixation solution (30% ethanol, 10% acetic acid), washed 2 × 10 min in water, incubated overnight in a colloidal Coomassie blue staining solution [20% methanol, 8% ammonium sulfate, 1.6% phosphoric acid, 0.08% Coomassie Brilliant blue G-250 (Merck KGaA, Darmstadt, Germany)] and finally destained in a destaining solution (5% methanol, 7% acetic acid).
In silico Analyses
Fasta sequences of Arabidopsis peptidases were first retrieved from the FTP server of the MEROPS database1. We then searched for corresponding sequences in the genome of Arabidopsis (TAIR102) by BlastP. The best hit of each sequence was then further analyzed to remove non-perfectly matching sequences (>10% length difference, >2% mismatches).
In silico transcriptomic analyses were performed on Arabidopsis Affymetrix ATH1 raw data retrieved from the ArrayExpress database3 using “roots” and “suspension cells” as queries. The resulting list of microarrays was manually sorted to remove experiments lacking comprehensive methodological informations. The list of experiments included in the survey is available as a supplemental data file. Within each experiment, we manually removed the microarrays performed on leaves and whole seedlings. The subsequent data analysis was performed using the R programming language (R Core Team, 2014). The “simpleaffy” Bioconductor package (Gentleman et al., 2004; Wilson and Miller, 2005) was used to read the raw data and perform the present/absent call on individual arrays using the detection.p.val() function. Genes were considered as being expressed when p-value <0.01. Within each experiment, we computed the proportion of arrays in which the gene of interest could be detected. Data were sorted using the list of peptidases defined above.
Results
Active Peptidases are Species and Production System Specific
Secreted peptidases of Arabidopsis (PSB-D cells and Col-0 strain) and tobacco (BY-2 cells and SR1 strain) were collected by filtration of 7-days-old cell suspensions (EM) or by centrifugation of hydroponic roots, harvested on 7-weeks old plants and incubated in a saline solution to solubilize apoplastic proteins [rhizosecretome (RZ)]. These harvesting timepoints were selected for maximum peptidase activity and diversity, based on preliminary time-lapse experiments (data not shown).
To get a first insight into the diversity of active peptidases, EM and RZ were analyzed by zymography with gelatin as substrate. As shown in Figure 1, the degradation patterns were highly different as regards with species and production systems.
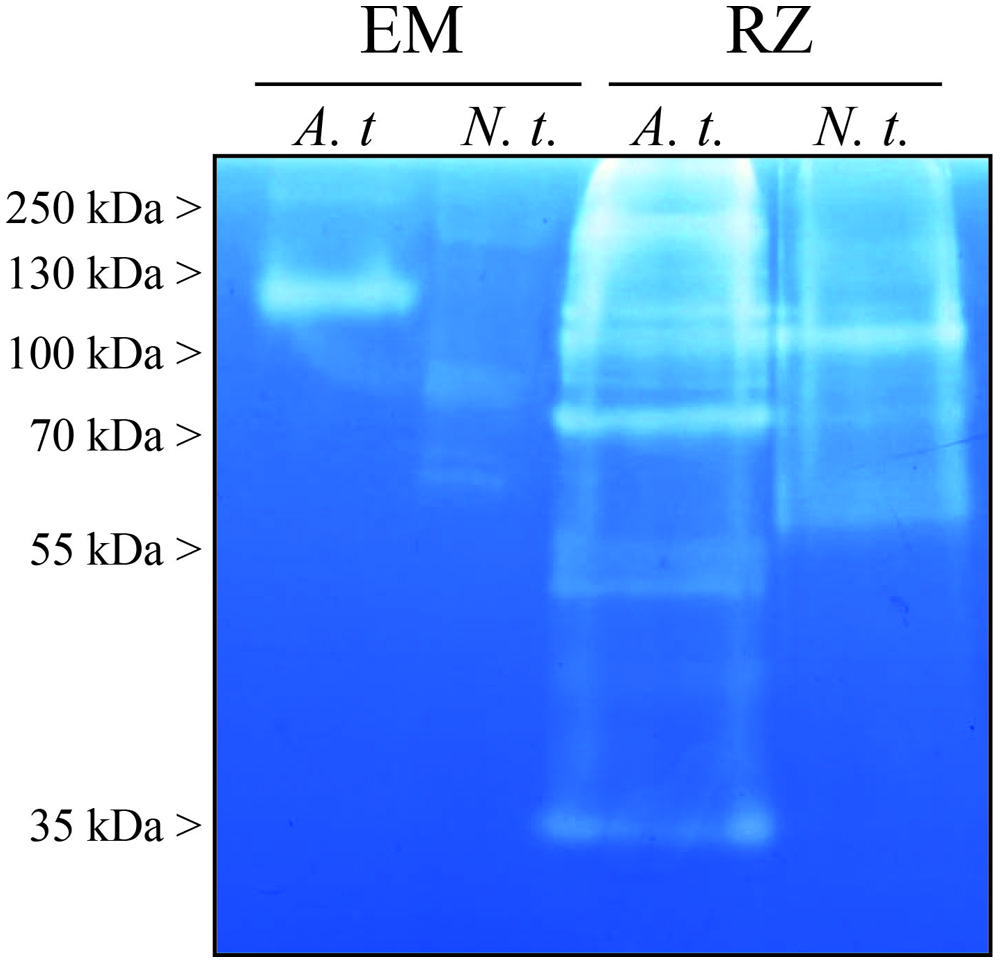
FIGURE 1. Proteolytic activities analyzed by gelatin zymography of the extracellular media (EM) and the rhizosecretomes (RZs) of Arabidopsis thaliana (A.t.), and Nicotiana tabacum (N.t.). Samples were collected from 7-days-old suspension cells (EM) or 7-weeks-old hydroponic cultures (RZ). 20 μl of EM or RZ were loaded on a SDS polyacrylamide gel containing 0.05% of gelatin. After electrophoresis, proteins were renaturated in the gel and the proteolytic activity was revealed after a 16-h incubation required for gelatin degradation. The presented result is representative of two independent experiments.
The diversity of peptidase activities detected on zymogram was larger in RZ: more degradation bands were observed, over a wider range of molecular weights, than in EM (Figure 1). Moreover, in both RZ and EM setups, Arabidopsis samples displayed more degradation bands and bands with stronger activities than those from tobacco.
pH-Dependence Degradation of Selected Targets Mainly Differs According to Production Systems
We then assayed in vitro the proteolytic activities of Arabidopsis and tobacco EM and RZ, against two target proteins: BSA and hIgGs, in pH conditions ranging from 2 to 9. We observed that the proteolytic activity was dependent upon the four experimental variables: the production system, the plant species, the target protein and the pH (Figure 2). However, the overall analysis of Figure 2 clearly points out that the largest variation was due to the production systems, while species and target proteins only added limited variations to the overall pH-dependent activity. Obviously, the RZ production systems contained more proteolytic activity than cell suspensions EM (Figure 1).
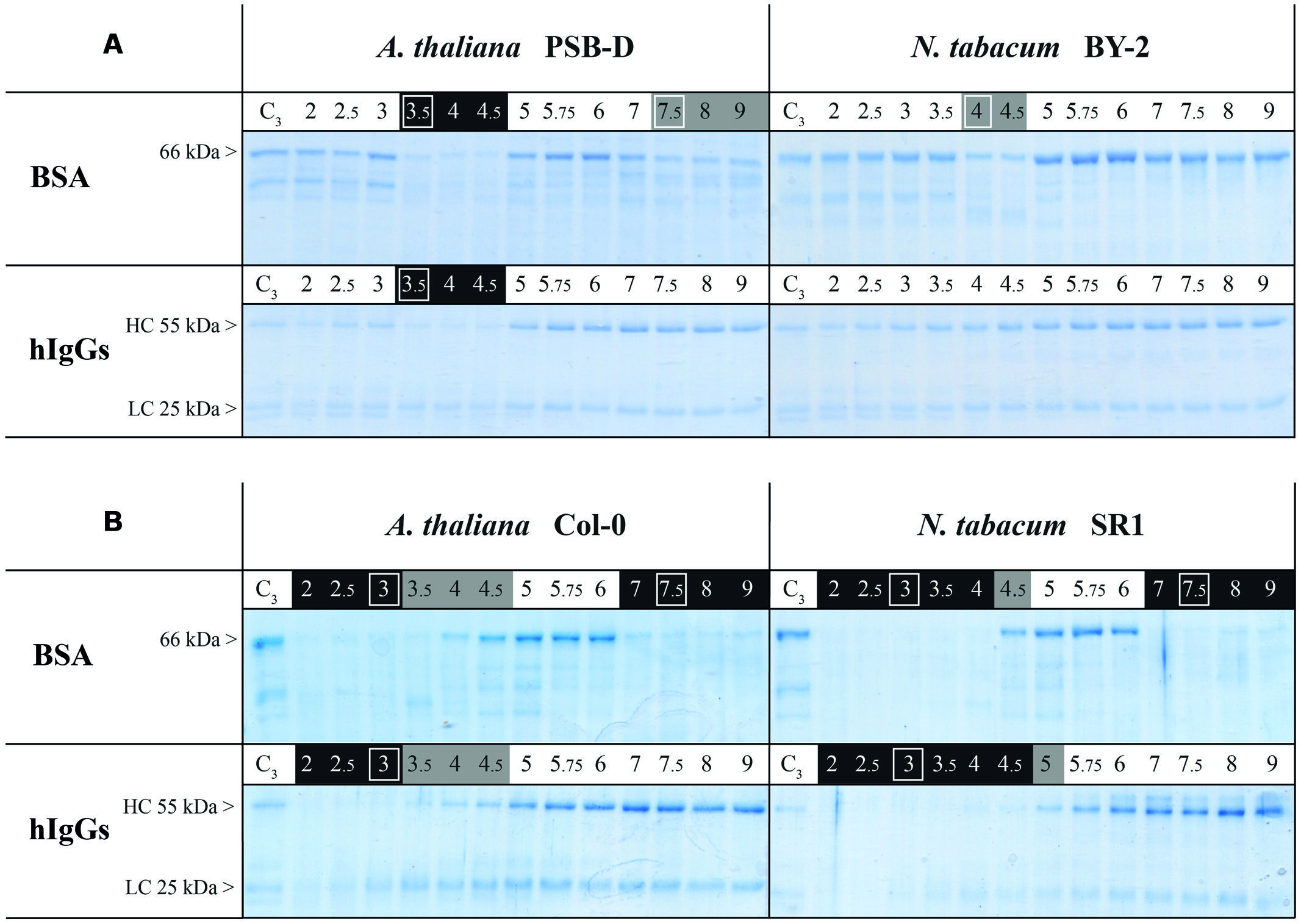
FIGURE 2. Proteolytic activities in (A) extracellular medium of cell suspensions and (B) rhizosecretome in A. thaliana and N. tabacum. Samples were collected from 7-days-old suspension cells (EM) or 7-weeks-old hydroponic cultures (RZ). 1 μg of BSA or hIgGs was incubated at 25°C during 6 h, in a buffer with a pH-value ranging from 2 to 9 and with 5 μl of secretome. It was then analyzed on reducing SDS-PAGE. C3 indicates control samples incubated at pH 3 after heat-inactivation of secretome proteins and thus shows maximum intensities of target-protein band(s). pH values highlighted in black or gray indicate pH ranges where, respectively, strong and moderate degradations were observed. The white frames indicate pH conditions selected for further analyses. The observed gradual decrease in hIgGs band intensities at acidic pH was not related to proteolytic events since this fade-out of the heavy chain band was also observed in control conditions. Each gel is representative of the results obtained from at least two independent experiments.
The species- and target- effects were best observed in the cell suspensions systems (Figure 2A). In Arabidopsis PSB-D EM, degradation of BSA was mainly observed in acidic conditions (from 3.5 to ~5), but partial degradation was also observed at pH greater than 7. The other target, hIgGs, was also sensitive to Arabidopsis EM proteases at acidic pH of 3.5–4.5 but was less prone to degradation at higher pH, and remained intact in Nicotiana EM samples.
In RZ samples (Figure 2B), the same degradation profiles were obtained for both plant species but a strong target dependency was found: while BSA was degraded in acidic (2 to ~4) and neutral to basic (above 6) conditions, hIgGs were only degraded when incubated with RZ at pH below 5.
Secreted Serine- and Metallo-Peptidases are Active Against BSA and hIgGs
In order to figure out which of the four main peptidase functional classes [aspartic (Asp), cysteine (Cys), metallo (Met), and serine (Ser) peptidases] were involved in the degradation of BSA and hIgGs in RZ and EM samples, we added specific peptidase inhibitors to the in vitro assays. Different mixtures of three specific peptidase inhibitors were used, in such a way that only one functional class was active in a single sample.
As two major pH ranges were selected for degradation tests (acidic pH and neutral-basic pH, Figure 2), we performed these assays at pH 3 to 4 (depending on the system and species) and pH 7.5. In all assays, degradation of BSA and hIgGs was prevented by heat inactivation (Figure 3, ‘C’ lanes) or addition of the 4-inhibitor mix (Figure 3, ‘No’ lanes) indicating that any observed degradation was due to the peptidase activity of the samples. These treatments thus provided control samples showing maximum intensities of target bands whereas maximum degradation was observed in the absence of inhibitors (Figure 3, ‘All’ lanes).
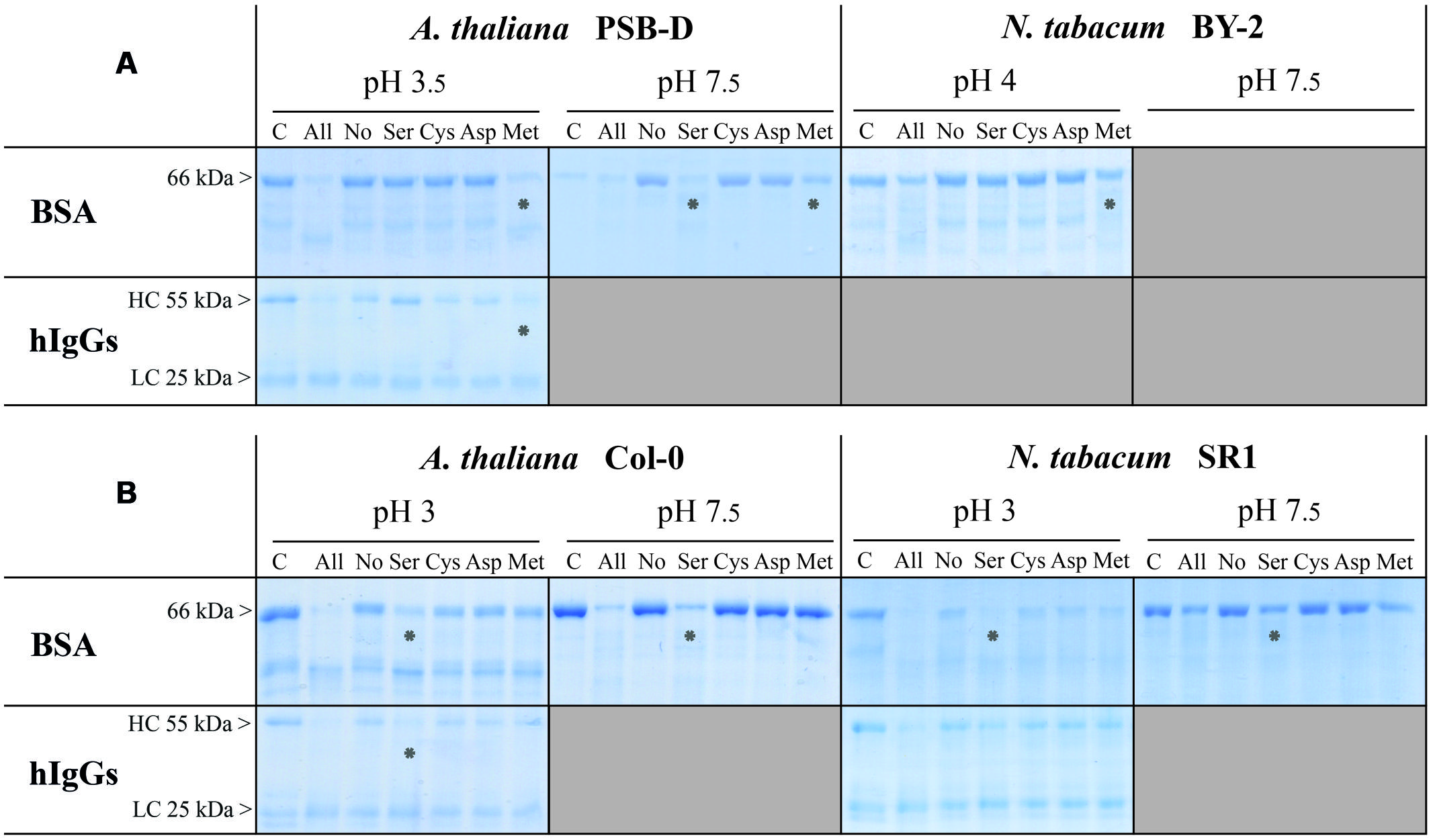
FIGURE 3. Identification of peptidase classes responsible for target degradation in A. thaliana and N. tabacum EM or RZ. Samples were collected from (A) 7-days-old suspension cells (EM) or (B) 7-weeks-old hydroponic cultures (RZ). Before a six- (EM) or a 3-h incubation (RZ) at 25°C with the target proteins (BSA or hIgGs), samples were pre-incubated for 30 min at 25°C without peptidase inhibitors (all proteases are active; ‘All’), or with a mix of four peptidase inhibitors (PMSF, E64, pepstatin A, and EDTA; none is active; ‘No’), or with a mix of three of these inhibitors. Omitting one of the inhibitors left only one peptidase class active: omitting PMSF, E64, pepstatin A, or EDTA left serine-(Ser), cysteine- (Cys), aspartic- (Asp), or metallo-(Met) peptidases active, respectively. The degradation profiles of the target proteins were observed after reducing SDS-PAGE. Lane labels refer to active peptidase classes. Lane C: the extracellular peptidases were heat-inactivated, without inhibitors, before the addition of the target protein. Stars indicate which protease classes degrade target proteins. Shaded areas show combinations that were not tested, because of the lack of target degradation (refer to Figure 2). Each gel is representative of the results obtained from at least two independent experiments.
In cell suspensions EM of both species, we found peptidase activities that were sensitive to PMSF and EDTA, respectively attributable to Ser and Met peptidases (Figure 3A). Met peptidases were the only observed active class at acidic pH, against both BSA and hIgGs, while Ser peptidases were mainly responsible for the proteolytic degradation occurring in neutral to basic pH in EM of Arabidopsis cells (Figure 2).
In RZ of Arabidopsis and N. tabacum, peptidases of the Ser family were responsible for BSA degradation observed at both acidic and neutral-to-basic pH conditions (Figure 3B). Because of the pH-dependent fade-out of the heavy chain band (see Figure 2), the degradation of hIgGs at acidic pH observed in Figure 3 was more difficult to assign to a specific peptidase class but seemed to be, at least in Arabidopsis, also due to Ser peptidases.
Serine Peptidases are Predicted to Be the Most Abundant Catalytic Class in Arabidopsis Secretome
To go further toward the identification of putative Ser and Met peptidases that are responsible for degrading BSA and hIgGs in vitro, we performed an in silico analysis using genomic and transcriptomic data available for Arabidopsis.
We first retrieved the 883 peptidase sequences available from the MEROPS 9.11 database (Rawlings et al., 2014) and filtered them for those corresponding to an existing locus in Arabidopsis genome [TAIR10, (Lamesch et al., 2012); Figure 4, see Materials and Methods].
This analysis revealed 570 unique loci corresponding to peptidases in Arabidopsis genome. Almost half of these peptidases belong to the Ser peptidase class (255). The Cys-, Met-, and Asp- classes follow with 144, 86, and 65 representatives, respectively. The subcellular localization of these peptidases was then evaluated to identify those that could be retrieved in the secretomes, i.e., in the extracellular space. A common strategy is to search for a N-terminal secretion signal in the sequence, using signalP or equivalent predictive tools (Petersen et al., 2011). With signalP 4.1 tool, 216 out of the 570 peptidases were predicted to be secreted. We next compared these results with those available from the Suba3 database, which is a curated subcellular location database for Arabidopsis proteins that combines information collected from literature, tagged protein experiments, protein–protein interaction datasets and results coming from 22 prediction programs (Tanz et al., 2013). As shown in Figure 4, a significant smaller amount of peptidases (147) were predicted to be extracellular by the Suba3 analysis compared with signalP 4.1, except for two families (S8 and S33) for which the opposite result was obtained. Among the 147 predicted secreted peptidases, 85 belong to the Ser-class and 6 to the Met-class and are thus putative candidates to explain proteolytic degradation detected in RZ and EM of Arabidopsis.
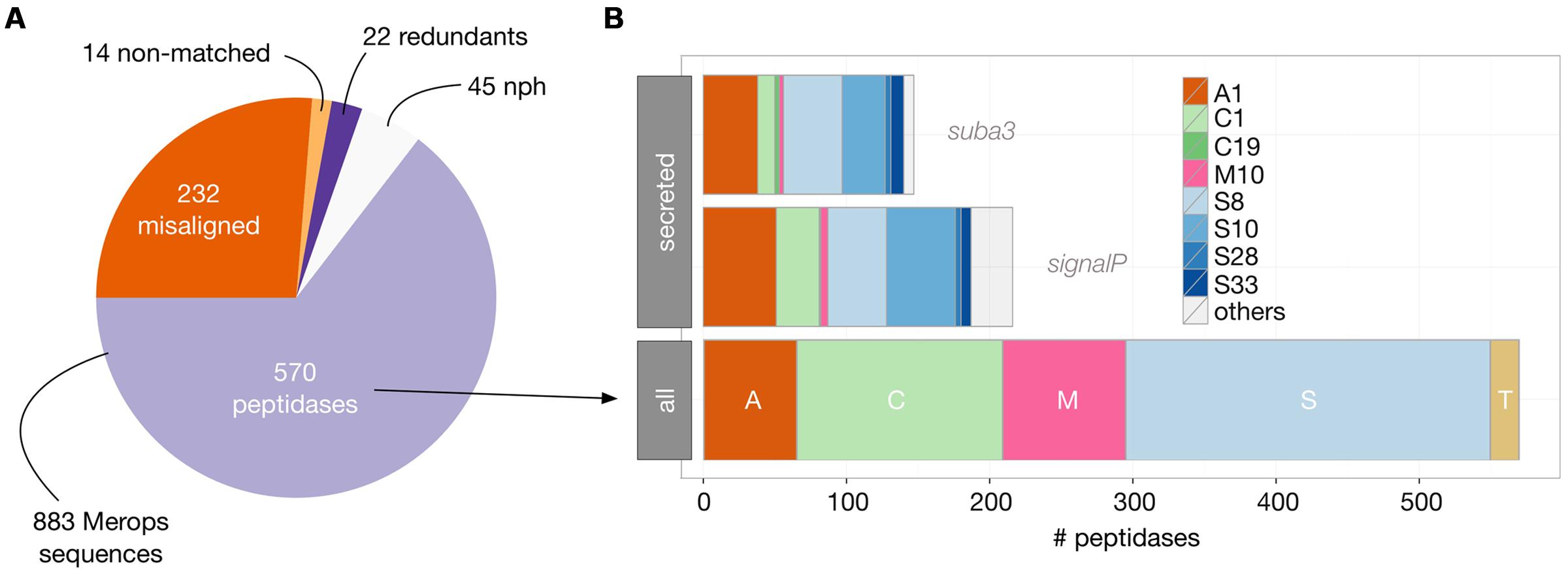
FIGURE 4. Identification and distribution of Arabidopsis peptidases as inferred from MEROPS 9.11 database and TAIR10 genomic data. (A) BlastP analysis (see Materials and Methods) of the 883 MEROPS sequences revealed 570 actual peptidases in Arabidopsis genome; the other MEROPS sequences did not have a perfect match in the genome (misaligned and non-matched), were duplicated (redundant), or were non-peptidase homologs (nph). (B) The 570 peptidases were classified according to their catalytic class (A, Asp peptidases; C, Cys peptidases; M, Met Peptidases; S, Ser peptidases; and T, Threonine peptidases) and family (from A1 to S33). Secreted peptidases were predicted either by the presence of a signal peptide (SignalP) or by their computed subcellular localization available in the SUBA3 database. Only families having at least two representatives are displayed as colored bars.
More Secreted Peptidases Are Expressed in Hydroponics vs. Suspension Cells
To evaluate which of those putative 147 secreted peptidases are most likely expressed in rhizosecretion and cell suspension production systems, we analyzed transcriptomic data available from the EBI Array express repository (Rustici et al., 2013). Experiments retrieved from a search with the ‘root’ and ‘suspension cells’ keywords were manually curated to select the most similar to ours, in terms of growing conditions and tissue sampling. Data gathered for hydroponics encompassed 16 experiments containing 310 arrays while we found 11 cell suspensions experiments with a total of 126 arrays. We then applied a ‘present/absent call’ function for each of the 147 peptidases, using the simpleaffy Bioconductor package (Wilson and Miller, 2005). A peptidase was considered as being expressed in an experiment if it was seen as ‘present’ in at least 90% of the arrays of this experiment (Figure 5). We chose this high cut-off to foster the selection of peptidases that were constitutively expressed, i.e., whose transcripts were present in almost all arrays of an experiment and thus independent of the experimental set-up. We then calculated, for both hydroponics and suspension cells, the proportion of experiments in which a peptidase was expressed. As shown in Figure 5, only about 50% of the putative secreted peptidases were found to be expressed in at least 10% of the experiments. We identified 50 peptidases expressed in a least half of the experiments in hydroponics and 26 in at least half of the experiments in suspension cells (Figure 5, threshold 50%). It is noteworthy that whatever the threshold, the number of expressed peptidases was always greater in hydroponics experiments than in suspensions cells.
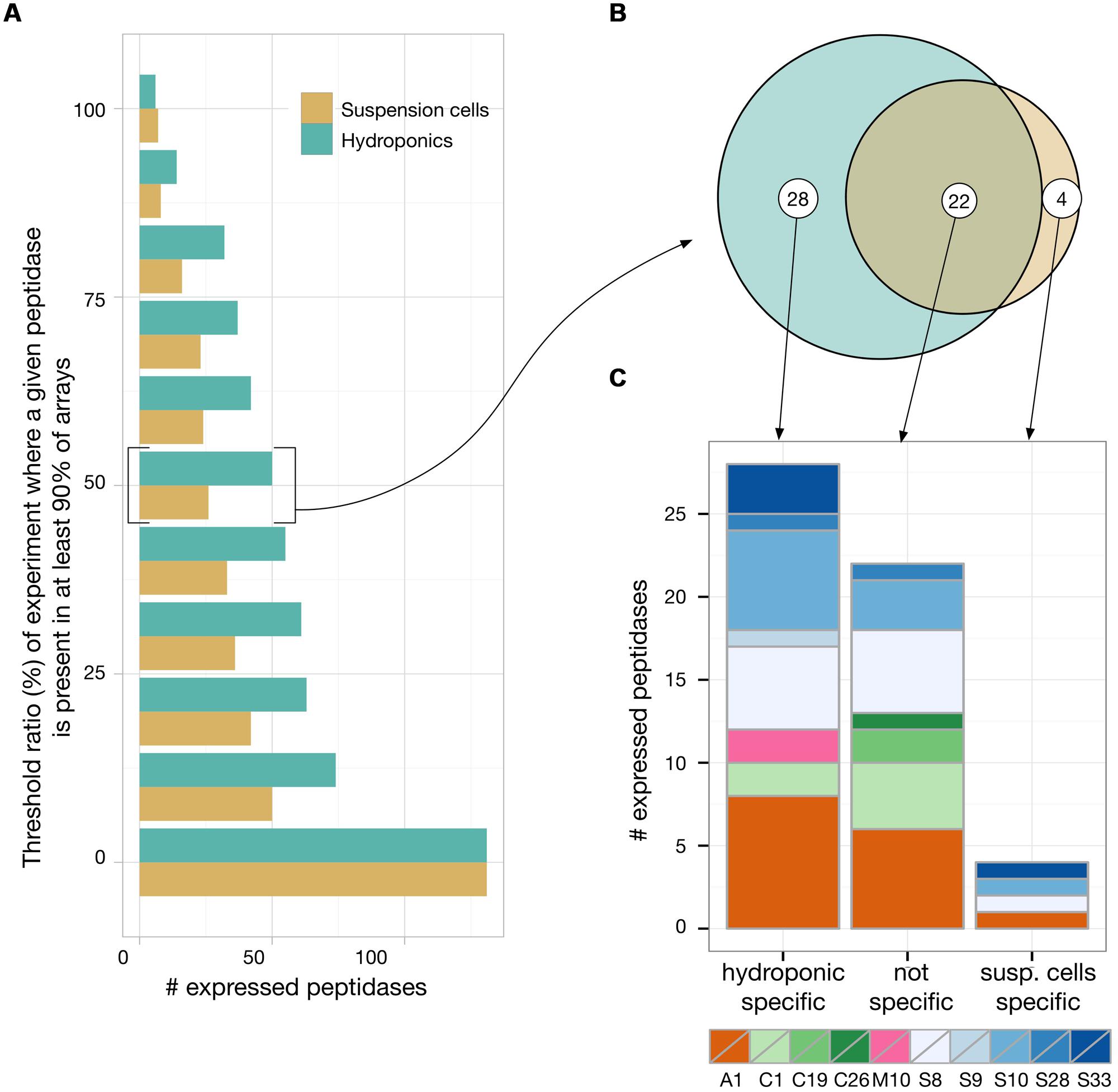
FIGURE 5. Transcriptomic analysis of Arabidopsis secreted peptidases by a meta-analysis of publicly available microarrays data of hydroponic and suspension cell experiments. A present/absent call (p < 0.01) was applied to each peptidase in each arrays of each experiment available. For each experiment, a peptidase was considered as ‘present’ if detected in at least 90% of its arrays. We then plotted in (A) the number of peptidases that were considered as present in at least a given proportion of the experiments. For peptidases that were found to be expressed in at least half of the experiments, we evaluated their specificity toward the expression context (B) and their distribution among the main represented peptidase families (C).
With the threshold ratio set to 50% (i.e., expression detected in a least 50% of the experiments), only four peptidases were revealed as specifically expressed in suspension cells: one Asp and three Ser peptidases (Figures 5B,C). Two matrix metallopeptidases were found to be expressed in hydroponics but none in suspension cell cultures (AT1G59970, AT1G70170). With no threshold ratio set (i.e., expression detected in a least one experiment), two additional Met peptidases were detected in hydroponics (AT1G24140, AT1G71696). Two of these four Met peptidases were also expressed in suspension cells experiments (AT1G59970, AT1G71696). Ser peptidases were always the most represented catalytic class in transcriptomes (data not shown). At the 50% threshold, a total of 28 Ser peptidases were found to be expressed in hydroponics and/or suspension cells. Five of them were expressed in all experiments (threshold 100%) and are listed in Table 1; the complete list of secreted peptidases is available as Supplemental Data file.
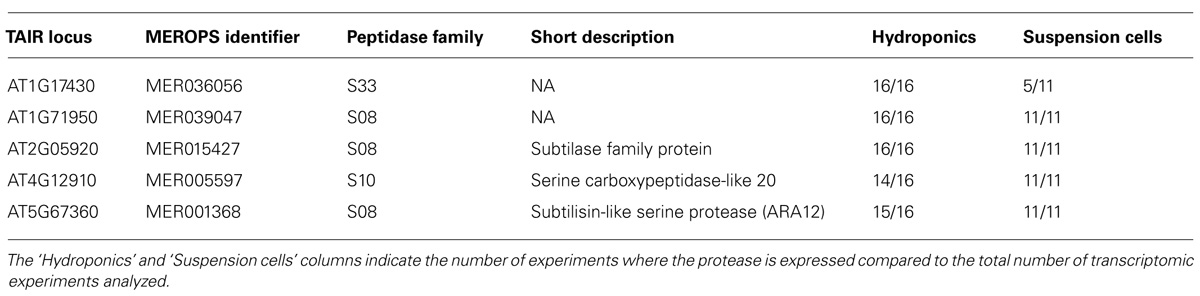
TABLE 1. TAIR10 functional annotation of the five Ser peptidases that were found to be expressed in all hydroponics and/or suspension cells transcriptomic experiments analyzed in this study.
Discussion
Unwanted degradation of recombinant proteins by endogenous peptidases is one of the major problems of plant-based heterologous production systems (Pillay et al., 2014). Peptidases are known to be involved in a multitude of processes from the cellular to whole organism level, but the exact functions and targets of most of them are still unknown (van der Hoorn, 2008). The strategies to prevent proteolysis thus generally rely on broad range inhibition of one or several catalytic classes, either by coexpression of peptidase inhibitors or direct gene silencing (Komarnytsky et al., 2006; Kim et al., 2008b; Goulet et al., 2010, 2012; Redkiewicz et al., 2012).
The increasing amount of ‘omics’ data provides an attractive starting point toward the identification of peptidases potentially at work in a given production system. However, this approach is rapidly limited by (1) the number of peptidases identified in genomes, (2) the limited knowledge about their functions, regulation and targets, and (3) the potential redundancy between peptidases of the same class, clan or family. Because peptidase activity is often controlled at the post-translational level, genomic and transcriptomic information are not sufficient. Moreover, functional redundancy hinders the identification of peptidases by simple activity-based assays. In this paper, we showed that meta-analyses of available genomic and transcriptomic data allow to reduce the huge list of peptidase candidates that results from activity-based assays, which essentially gives information about the catalytic class or the family.
We first focused on identifying active peptidase classes and addressing the question whether substantial differences exist between plant materials, production systems or target proteins, when assayed simultaneously. By using class-specific inhibitors, we showed that only Met and Ser peptidases were active against BSA and/or hIgGs, depending on the production system (RZ or EM) and the target protein.
Extracellular Met peptidases count only a few members, most of them belonging to the matrix metalloproteinases (MMPs) family (Marino and Funk, 2012). In our in silico analysis, only two MMPs were detected in a majority of hydroponic experiments, one of them being observed only in a few suspension cells experiments, together with a Zn2+ carboxypeptidase. Despite the scarcity of Met peptidases in secretomes, our results clearly showed that Met activity was present in EM of both Arabidopsis and BY-2 suspension cells, in agreement with previous reports for BY-2 (Delannoy et al., 2008; Schiermeyer et al., 2009; Mandal et al., 2010). The production system thus appears to be a strong determinant of proteolytic activities. The similarity between PSB-D and BY-2 cells overrides their species and tissue origins, which are Arabidopsis stem and tobacco root explants, respectively. By contrast, the two tobacco-based systems are very different in terms of pH dependence and active peptidases, despite their common species and tissue origins.
In contrast with Met peptidases, Ser peptidases are the most abundant class in plant cells and were therefore primarily targeted for plant-based production system improvement. Co-expression of Ser inhibitor, either as a second transgene or a fusion protein, improved, yet not totally, stability of recombinant proteins (Komarnytsky et al., 2006; Kim et al., 2008b; Goulet et al., 2010, 2012; Redkiewicz et al., 2012). Prior assessment of active peptidases is rarely performed and, if any, is usually done with non-specific targets (Rivard et al., 2006; Goulet et al., 2012). However, even closely related proteins, for example recombinant immunoglobulins, exhibit variable sensitivity to peptidases (Magy et al., 2014; Niemer et al., 2014), and hence end-product evaluation of peptidase activities is needed. The actual risk of proteolytic degradation is even more difficult to predict if several production systems or hosts are available, since each of them has its own peptidase assortment. This diversity was clearly illustrated here by comparing suspension cells and rhizosecretion in Arabidopsis and tobacco and was reported before (Magy et al., 2014; Pillay et al., 2014).
Merging activity assays with geno-transcriptomic data allowed us to narrow down the list of Ser peptidases potentially responsible for target degradations in Arabidopsis RZ and EM: out of 255 Ser peptidases identified in the genome, 85 were predicted to be extracellular and 25 were expressed in conditions similar to the production systems. Five of them were consistently expressed in suspension cells or hydroponics experiments included in the meta-analysis (Table 1), among which the serine carboxypeptidase SCP20 and the subtilisin-like ARA12 (Table 1). SCP20 was detected in the extracellular space of seedlings and leaves, and was reported to be strongly induced following fungal infection (Charmont et al., 2005; Floerl et al., 2012). ARA12 was frequently identified in cell wall proteomics studies either in EM of suspension cell cultures (Hamilton et al., 2003; Shen et al., 2013) or in seedlings and leaves (Boudart et al., 2005; Charmont et al., 2005; Fraser et al., 2005; Floerl et al., 2012). If the exact function of ARA12 remains unknown, its purification and biochemical characterization showed that it is a heat-stable peptidase functioning in a wide range of pH, from 3 to 7 with an optimum around 5 (Hamilton et al., 2003). These properties fit very well with the conditions in which we performed the activity assays presented here. Interestingly, a close N. tabacum homolog of ARA12 was identified in BY-2 EM (Navarre et al., 2012), indicating that production systems are similar, whatever the plant species, as also inferred from our cross-comparison.
Our cross-comparison of production systems and plant hosts, together with our in silico analysis of peptidases, consistently show that suspension cell cultures provide a less proteolytic environment for the production of recombinant proteins, especially antibodies. Even if less degradation was observed in BY-2 tobacco cells compared with Arabidopsis PSB-D cells, the relatively weak influence of the plant species permits to use model species such as Arabidopsis as a starting point for optimization of other host species. The top listed peptidases identified by our combined biochemical/bioinformatic analysis are thus prime candidates for new technology development, e.g., amiRNA- or MIGS multigene silencing, or nuclease assisted genome engineering (Schwab et al., 2006; Felippes et al., 2012; Voytas, 2013). These methods should soon be applicable to various plant species as the acquisition of genomic information increases quickly. Nevertheless, Arabidopsis is a competitive production system, even when compared with the well established N. tabacum BY-2 cells (Magy et al., 2014), and may even take a stronger position if one takes advantage of its technological advance for genetic engineering.
Conflict of Interest Statement
The authors declare that the research was conducted in the absence of any commercial or financial relationships that could be construed as a potential conflict of interest.
Acknowledgments
The research was funded by the Service Public de Wallonie, DG06 (Waléo3 program, 08/1/6861). The authors warmly thank their collaborators Marc Boutry, Jacques Dommes, Christine Dupont, Catherine Navarre, and Marie-France Versali for their critical comments on the experiments and results. Jérôme Lallemand and Frédéric Bouché are grateful to the F.R.S.-FNRS for their PhD fellowships (FC95800; FC87200), as well as Carole Desiron for her F.R.I.A. grant. While working on this paper, we were truly shocked by the terrible terrorist attack against the French weekly “Charlie Hebdo.” The freedom of making and publishing science is so closely related to the freedom of expression that the authors want symbolically to express their complete solidarity with the victims of this attack by these simple words: “We are Charlie.”
Supplementary Material
The Supplementary Material for this article can be found online at: http://www.frontiersin.org/journal/10.3389/fpls.2015.00037/abstract
Footnotes
- ^ftp://ftp.sanger.ac.uk/pub/MEROPS/current_release/protease.lib
- ^ftp://ftp.arabidopsis.org/Sequences/blast_datasets/TAIR10_blastsets/TAIR10_pep_20110103_representative_gene_model_updated
- ^http://www.ebi.ac.uk/arrayexpress/
References
Albenne, C., Canut, H., and Jamet, E. (2013). Plant cell wall proteomics: the leadership of Arabidopsis thaliana. Front. Plant Sci. 4:111. doi: 10.3389/fpls.2013.00111
Pubmed Abstract | Pubmed Full Text | CrossRef Full Text | Google Scholar
Borisjuk, N. V., Borisjuk, L. G., Logendra, S., Petersen, F., Gleba, Y., and Raskin, I. (1999). Production of recombinant proteins in plant root exudates. Nat. Biotechnol. 17, 466–469. doi: 10.1038/8643
Pubmed Abstract | Pubmed Full Text | CrossRef Full Text | Google Scholar
Boudart, G., Jamet, E., Rossignol, M., Lafitte, C., Borderies, G., Jauneau, A.,et al. (2005). Cell wall proteins in apoplastic fluids of Arabidopsis thaliana rosettes: identification by mass spectrometry and bioinformatics. Proteomics 5, 212–221. doi: 10.1002/pmic.200400882
Pubmed Abstract | Pubmed Full Text | CrossRef Full Text | Google Scholar
Charmont, S., Jamet, E., Pont-Lezica, R., and Canut, H. (2005). Proteomic analysis of secreted proteins from Arabidopsis thaliana seedlings: improved recovery following removal of phenolic compounds. Phytochemistry 66, 453–461. doi: 10.1016/j.phytochem.2004.12.013
Pubmed Abstract | Pubmed Full Text | CrossRef Full Text | Google Scholar
Cox, K. M., Sterling, J. D., Regan, J. T., Gasdaska, J. R., Frantz, K. K., Peele, C. G.,et al. (2006). Glycan optimization of a human monoclonal antibody in the aquatic plant Lemna minor. Nat. Biotechnol. 24, 1591–1597. doi: 10.1038/nbt1260
Pubmed Abstract | Pubmed Full Text | CrossRef Full Text | Google Scholar
De Muynck, B., Navarre, C., Nizet, Y., Stadlmann, J., and Boutry, M. (2009). Different subcellular localization and glycosylation for a functional antibody expressed in Nicotiana tabacum plants and suspension cells. Transgenic Res. 18, 467–482. doi: 10.1007/s11248-008-9240-1
Pubmed Abstract | Pubmed Full Text | CrossRef Full Text | Google Scholar
Decker, E. L., Parsons, J., and Reski, R. (2014). Glyco-engineering for biopharmaceutical production in moss bioreactors. Front. Plant Sci. 5:346. doi: 10.3389/fpls.2014.00346
Pubmed Abstract | Pubmed Full Text | CrossRef Full Text | Google Scholar
Delannoy, M., Alves, G., Vertommen, D., Ma, J., Boutry, M., and Navarre, C. (2008). Identification of peptidases in Nicotiana tabacum leaf intercellular fluid. Proteomics 8, 2285–2298. doi: 10.1002/pmic.200700507
Pubmed Abstract | Pubmed Full Text | CrossRef Full Text | Google Scholar
Drake, P. M. W., Barbi, T., Sexton, A., McGowan, E., Stadlmann, J., Navarre, C.,et al. (2009). Development of rhizosecretion as a production system for recombinant proteins from hydroponic cultivated tobacco. FASEB J. 23, 3581–3589. doi: 10.1096/fj.09131771
Pubmed Abstract | Pubmed Full Text | CrossRef Full Text | Google Scholar
Drake, P. M. W., Chargelegue, D., Vine, N. D., Dolleweerd, C. J. V., Obregon, P., and Ma, J. K.-C. (2002). Transgenic plants expressing antibodies: a model for phytoremediation. FASEB J. 16, 1855–1860. doi: 10.1096/fj.02-0148com
Pubmed Abstract | Pubmed Full Text | CrossRef Full Text | Google Scholar
Drake, P. M. W., Chargelegue, D. M., Vine, N. D., van Dolleweerd, C. J., Obregon, P., and Ma, J. K.-C. (2003). Rhizosecretion of a monoclonal antibody protein complex from transgenic tobacco roots. Plant Mol. Biol. 52, 233–241. doi: 10.1023/A:1023909331482
Felippes, F. F., Wang, J.-W., and Weigel, D. (2012). MIGS: miRNA-induced gene silencing. Plant J. 70, 541–547. doi: 10.1111/j.1365-313X.2011.04896.x
Pubmed Abstract | Pubmed Full Text | CrossRef Full Text | Google Scholar
Fischer, R., Schillberg, S., Buyel, J., and Twyman, R. (2013). Commercial aspects of pharmaceutical protein production in plants. Curr. Pharm. Des. 19, 5471–5477. doi: 10.2174/1381612811319310002
Floerl, S., Majcherczyk, A., Possienke, M., Feussner, K., Tappe, H., Gatz, C.,et al. (2012). Verticillium longisporum infection affects the leaf apoplastic proteome, metabolome, and cell wall properties in Arabidopsis thaliana. PLoS ONE 7:e31435. doi: 10.1371/journal.pone.0031435
Pubmed Abstract | Pubmed Full Text | CrossRef Full Text | Google Scholar
Fraser, C. M., Rider, L. W., and Chapple, C. (2005). An expression and bioinformatics analysis of the Arabidopsis serine carboxypeptidase-like gene family. Plant Physiol. 138, 1136–1148. doi: 10.1104/pp.104.057950
Pubmed Abstract | Pubmed Full Text | CrossRef Full Text | Google Scholar
Gentleman, R. C., Carey, V. J., Bates, D. M., Bolstad, B., Dettling, M., Dudoit, S.,et al. (2004). Bioconductor: open software development for computational biology and bioinformatics. Genome Biol. 5:R80. doi: 10.1186/gb-2004-5-10-r80
Pubmed Abstract | Pubmed Full Text | CrossRef Full Text | Google Scholar
Goulet, C., Benchabane, M., Anguenot, R., Brunelle, F., Khalf, M., and Michaud, D. (2010). A companion protease inhibitor for the protection of cytosol-targeted recombinant proteins in plants. Plant Biotechnol. J. 8, 142–154. doi: 10.1111/j.1467-7652.2009.00470.x
Pubmed Abstract | Pubmed Full Text | CrossRef Full Text | Google Scholar
Goulet, C., Khalf, M., Sainsbury, F., D’Aoust, M.-A., and Michaud, D. (2012). A protease activity-depleted environment for heterologous proteins migrating towards the leaf cell apoplast. Plant Biotechnol. J. 10, 83–94. doi: 10.1111/j.1467-7652.2011.00643.x
Pubmed Abstract | Pubmed Full Text | CrossRef Full Text | Google Scholar
Häkkinen, S. T., Raven, N., Henquet, M., Laukkanen, M.-L., Anderlei, T., Pitkänen, J.-P.,et al. (2014). Molecular farming in tobacco hairy roots by triggering the secretion of a pharmaceutical antibody. Biotechnol. Bioeng. 111, 336–346. doi: 10.1002/bit.25113
Pubmed Abstract | Pubmed Full Text | CrossRef Full Text | Google Scholar
Hamilton, J. M. U., Simpson, D. J., Hyman, S. C., Ndimba, B. K., and Slabas, A. R. (2003). Ara12 subtilisin-like protease from Arabidopsis thaliana: purification, substrate specificity and tissue localization. Biochem. J. 370, 57–67. doi: 10.1042/BJ20021125
Pubmed Abstract | Pubmed Full Text | CrossRef Full Text | Google Scholar
Hehle, V. K., Paul, M. J., Drake, P. M., Ma, J. K., and van Dolleweerd, C. J. (2011). Antibody degradation in tobacco plants: a predominantly apoplastic process. BMC Biotechnol. 11:128. doi: 10.1186/1472-6750-11-128
Pubmed Abstract | Pubmed Full Text | CrossRef Full Text | Google Scholar
Hiatt, A., Caffferkey, R., and Bowdish, K. (1989). Production of antibodies in transgenic plants. Nature 342, 76–78. doi: 10.1038/342076a0
Pubmed Abstract | Pubmed Full Text | CrossRef Full Text | Google Scholar
Kim, N.-S., Kim, T.-G., Kim, O.-H., Ko, E.-M., Jang, Y.-S., Jung, E.-S.,et al. (2008a). Improvement of recombinant hGM-CSF production by suppression of cysteine proteinase gene expression using RNA interference in a transgenic rice culture. Plant Mol. Biol. 68, 263–275. doi: 10.1007/s11103-008-9367-8
Pubmed Abstract | Pubmed Full Text | CrossRef Full Text | Google Scholar
Kim, T.-G., Lee, H.-J., Jang, Y.-S., Shin, Y.-J., Kwon, T.-H., and Yang, M.-S. (2008b). Co-expression of proteinase inhibitor enhances recombinant human granulocyte–macrophage colony stimulating factor production in transgenic rice cell suspension culture. Protein Expr. Purif. 61, 117–121. doi: 10.1016/j.pep.2008.06.005
Pubmed Abstract | Pubmed Full Text | CrossRef Full Text | Google Scholar
Komarnytsky, S., Borisjuk, N., Yakoby, N., Garvey, A., and Raskin, I. (2006). Cosecretion of protease inhibitor stabilizes antibodies produced by plant roots. Plant Physiol. 141, 1185–1193. doi: 10.1104/pp.105.074419
Pubmed Abstract | Pubmed Full Text | CrossRef Full Text | Google Scholar
Lamesch, P., Berardini, T. Z., Li, D., Swarbreck, D., Wilks, C., Sasidharan, R.,et al. (2012). The Arabidopsis information resource (TAIR): improved gene annotation and new tools. Nucleic Acids Res. 40, D1202–D1210. doi: 10.1093/nar/gkr1090
Pubmed Abstract | Pubmed Full Text | CrossRef Full Text | Google Scholar
Magy, B., Tollet, J., Laterre, R., Boutry, M., and Navarre, C. (2014). Accumulation of secreted antibodies in plant cell cultures varies according to the isotype, host species and culture conditions. Plant Biotechnol. J. 12, 457–467. doi: 10.1111/pbi.12152
Pubmed Abstract | Pubmed Full Text | CrossRef Full Text | Google Scholar
Mandal, M. K., Fischer, R., Schillberg, S., and Schiermeyer, A. (2010). Biochemical properties of the matrix metalloproteinase NtMMP1 from Nicotiana tabacum cv. BY -2 suspension cells. Planta 232, 899–910. doi: 10.1007/s00425-010-1221-y
Pubmed Abstract | Pubmed Full Text | CrossRef Full Text | Google Scholar
Mandal, M. K., Fischer, R., Schillberg, S., and Schiermeyer, A. (2014). Inhibition of protease activity by antisense RNA improves recombinant protein production in Nicotiana tabacum cv. Bright yellow 2 (BY-2) suspension cells. Biotechnol. J. 9, 1065–1073. doi: 10.1002/biot.201300424
Pubmed Abstract | Pubmed Full Text | CrossRef Full Text | Google Scholar
Marino, G., and Funk, C. (2012). Matrix metalloproteinases in plants: a brief overview. Physiol. Plant. 145, 196–202. doi: 10.1111/j.1399-3054.2011.01544.x
Pubmed Abstract | Pubmed Full Text | CrossRef Full Text | Google Scholar
Mathieu-Rivet, E., Kiefer-Meyer, M.-C., Vanier, G., Ovide, C., Burel, C., Lerouge, P.,et al. (2014). Protein N-glycosylation in eukaryotic microalgae and its impact on the production of nuclear expressed biopharmaceuticals. Front. Plant Sci. 5:359. doi: 10.3389/fpls.2014.00359
Pubmed Abstract | Pubmed Full Text | CrossRef Full Text | Google Scholar
Navarre, C., De Muynck, B., Alves, G., Vertommen, D., Magy, B., and Boutry, M. (2012). Identification, gene cloning and expression of serine proteases in the extracellular medium of Nicotiana tabacum cells. Plant Cell Rep. 31, 1959–1968. doi: 10.1007/s00299-012-1308-y
Pubmed Abstract | Pubmed Full Text | CrossRef Full Text | Google Scholar
Niemer, M., Mehofer, U., Torres Acosta, J. A., Verdianz, M., Henkel, T., Loos, A.,et al. (2014). The human anti-HIV antibodies 2F5, 2G12, and PG9 differ in their susceptibility to proteolytic degradation: down-regulation of endogenous serine and cysteine proteinase activities could improve antibody production in plant-based expression platforms. Biotechnol. J. 9, 493–500. doi: 10.1002/biot.201300207
Pubmed Abstract | Pubmed Full Text | CrossRef Full Text | Google Scholar
Paul, M., and Ma, J. K.-C. (2011). Plant-made pharmaceuticals: leading products and production platforms. Biotechnol. Appl. Biochem. 58, 58–67. doi: 10.1002/bab.6
Pubmed Abstract | Pubmed Full Text | CrossRef Full Text | Google Scholar
Petersen, T. N., Brunak, S., von Heijne, G., and Nielsen, H. (2011). SignalP 4.0: discriminating signal peptides from transmembrane regions. Nat. Methods 8, 785–786. doi: 10.1038/nmeth.1701
Pubmed Abstract | Pubmed Full Text | CrossRef Full Text | Google Scholar
Pillay, P., Schlüter, U., van Wyk, S., Kunert, K. J., and Vorster, B. J. (2014). Proteolysis of recombinant proteins in bioengineered plant cells. Bioengineered 5, 15–20. doi: 10.4161/bioe.25158
Pubmed Abstract | Pubmed Full Text | CrossRef Full Text | Google Scholar
Rawlings, N. D., Waller, M., Barrett, A. J., and Bateman, A. (2014). MEROPS: the database of proteolytic enzymes, their substrates and inhibitors. Nucleic Acids Res. 42, D503–D509. doi: 10.1093/nar/gkt953
Pubmed Abstract | Pubmed Full Text | CrossRef Full Text | Google Scholar
R Core Team. (2014). R: A Language and Environment for Statistical Computing. Vienna: R Foundation for Statistical Computing. Available at: http://www.R-project.org/.
Redkiewicz, P., Więsyk, A., Góra-Sochacka, A., and Sirko, A. (2012). Transgenic tobacco plants as production platform for biologically active human interleukin 2 and its fusion with proteinase inhibitors. Plant Biotechnol. J. 10, 806–814. doi: 10.1111/j.1467-7652.2012.00698.x
Pubmed Abstract | Pubmed Full Text | CrossRef Full Text | Google Scholar
Rivard, D., Anguenot, R., Brunelle, F., Le, V. Q., Vézina, L.-P., Trépanier, S.,et al. (2006). An in-built proteinase inhibitor system for the protection of recombinant proteins recovered from transgenic plants. Plant Biotechnol. J. 4, 359–368. doi: 10.1111/j.1467-7652.2006.00187.x
Pubmed Abstract | Pubmed Full Text | CrossRef Full Text | Google Scholar
Robert, S., Khalf, M., Goulet, M.-C., D’Aoust, M.-A., Sainsbury, F., and Michaud, D. (2013). Protection of recombinant mammalian antibodies from development-dependent proteolysis in leaves of Nicotiana benthamiana. PLoS ONE 8:e70203. doi: 10.1371/journal.pone.0070203
Pubmed Abstract | Pubmed Full Text | CrossRef Full Text | Google Scholar
Rustici, G., Kolesnikov, N., Brandizi, M., Burdett, T., Dylag, M., Emam, I.,et al. (2013). ArrayExpress update–trends in database growth and links to data analysis tools. Nucleic Acids Res. 41, D987–D990. doi: 10.1093/nar/gks1174
Pubmed Abstract | Pubmed Full Text | CrossRef Full Text | Google Scholar
Schiermeyer, A., Hartenstein, H., Mandal, M. K., Otte, B., Wahner, V., and Schillberg, S. (2009). A membrane-bound matrix-metalloproteinase from Nicotiana tabacum cv. BY-2 is induced by bacterial pathogens. BMC Plant Biol. 9:83. doi: 10.1186/1471-2229-9-83
Pubmed Abstract | Pubmed Full Text | CrossRef Full Text | Google Scholar
Schillberg, S., Raven, N., Fischer, R., Twyman, R., and Schiermeyer, A. (2013). Molecular farming of pharmaceutical proteins using plant suspension cell and tissue cultures. Curr. Pharm. Des. 19, 5531–5542. doi: 10.2174/1381612811319310008
Schwab, R., Ossowski, S., Riester, M., Warthmann, N., and Weigel, D. (2006). Highly specific gene silencing by artificial microRNAs in Arabidopsis. Plant Cell 18, 1121–1133. doi: 10.1105/tpc.105.039834
Pubmed Abstract | Pubmed Full Text | CrossRef Full Text | Google Scholar
Shaaltiel, Y., Bartfeld, D., Hashmueli, S., Baum, G., Brill-Almon, E., Galili, G.,et al. (2007). Production of glucocerebrosidase with terminal mannose glycans for enzyme replacement therapy of Gaucher’s disease using a plant cell system. Plant Biotechnol. J. 5, 579–590. doi: 10.1111/j.1467-7652.2007.00263.x
Pubmed Abstract | Pubmed Full Text | CrossRef Full Text | Google Scholar
Sharp, J. M., and Doran, P. M. (2001). Characterization of monoclonal antibody fragments produced by plant cells. Biotechnol. Bioeng. 73, 338–346. doi: 10.1002/bit.1067
Pubmed Abstract | Pubmed Full Text | CrossRef Full Text | Google Scholar
Shen, J., Suen, P. K., Wang, X., Lin, Y., Lo, S. W., Rojo, E.,et al. (2013). An in vivo expression system for the identification of cargo proteins of vacuolar sorting receptors in Arabidopsis culture cells. Plant J. 75, 1003–1017. doi: 10.1111/tpj.12257
Pubmed Abstract | Pubmed Full Text | CrossRef Full Text | Google Scholar
Tanz, S. K., Castleden, I., Hooper, C. M., Vacher, M., Small, I., and Millar, H. A. (2013). SUBA3: a database for integrating experimentation and prediction to define the SUBcellular location of proteins in Arabidopsis. Nucleic Acids Res. 41, D1185–D1191. doi: 10.1093/nar/gks1151
Pubmed Abstract | Pubmed Full Text | CrossRef Full Text | Google Scholar
Tocquin, P., Corbesier, L., Havelange, A., Pieltain, A., Kurtem, E., Bernier, G.,et al. (2003). A novel high efficiency, low maintenance, hydroponic system for synchronous growth and flowering of Arabidopsis thaliana. BMC Plant Biol. 3:2. doi: 10.1186/1471-2229-3-2
Pubmed Abstract | Pubmed Full Text | CrossRef Full Text | Google Scholar
Tsiatsiani, L., Gevaert, K., and Van Breusegem, F. (2012). Natural substrates of plant proteases: how can protease degradomics extend our knowledge? Physiol. Plant. 145, 28–40. doi: 10.1111/j.1399-3054.2011.01534.x
Pubmed Abstract | Pubmed Full Text | CrossRef Full Text | Google Scholar
Twyman, R., Schillberg, S., and Fischer, R. (2013). Optimizing the yield of recombinant pharmaceutical proteins in plants. Curr. Pharm. Des. 19, 5486–5494. doi: 10.2174/1381612811319310004
van der Hoorn, R. A. (2008). Plant proteases: from phenotypes to molecular mechanisms. Annu. Rev. Plant Biol. 59, 191–223. doi: 10.1146/annurev.arplant.59.032607.092835
Pubmed Abstract | Pubmed Full Text | CrossRef Full Text | Google Scholar
Voytas, D. F. (2013). Plant genome engineering with sequence-specific nucleases. Annu. Rev. Plant Biol. 64, 327–350. doi: 10.1146/annurev-arplant-042811–105552
Wilson, C. L., and Miller, C. J. (2005). Simpleaffy: a bioConductor package for affymetrix quality control and data analysis. Bioinformatics 21, 3683–3685. doi: 10.1093/bioinformatics/bti605
Pubmed Abstract | Pubmed Full Text | CrossRef Full Text | Google Scholar
Keywords: molecular pharming, peptidases, Arabidopsis thaliana, Nicotiana tabacum, root-secretion, suspension cells, in silico analysis
Citation: Lallemand J, Bouché F, Desiron C, Stautemas J, de Lemos Esteves F, Périlleux C and Tocquin P (2015) Extracellular peptidase hunting for improvement of protein production in plant cells and roots. Front. Plant Sci. 6:37. doi: 10.3389/fpls.2015.00037
Received: 28 November 2014; Accepted: 14 January 2015;
Published online: 06 February 2015.
Edited by:
Giuseppe Dionisio, Aarhus University, DenmarkReviewed by:
Stefan Schillberg, Fraunhofer Institute for Molecular Biology and Applied Ecology, GermanyKarl Kunert, University of Pretoria, South Africa
Copyright © 2015 Lallemand, Bouché, Desiron, Stautemas, de Lemos Esteves, Périlleux and Tocquin. This is an open-access article distributed under the terms of the Creative Commons Attribution License (CC BY). The use, distribution or reproduction in other forums is permitted, provided the original author(s) or licensor are credited and that the original publication in this journal is cited, in accordance with accepted academic practice. No use, distribution or reproduction is permitted which does not comply with these terms.
*Correspondence: Pierre Tocquin, PhytoSYSTEMS, University of Liège, Boulevard du Rectorat 27, Liège, Belgium e-mail: ptocquin@ulg.ac.be
†Present address: Carole Desiron, Business & Decision Life Sciences, 141 Rue Saint-Lambert, 1200 Woluwe-Saint-Lambert, Belgium; Frédéric de Lemos Esteves, Life Sciences Library, University of Liège, Liège, Belgium