- 1Department of Earth and the Environment, Boston University, Boston, MA, USA
- 2Department of Biology, Boston University, Boston, MA, USA
- 3The Ecosystems Center, Marine Biological Laboratory, Woods Hole, MA, USA
Human activities have greatly altered global carbon (C) and Nitrogen (N) cycling. In fact, atmospheric concentrations of carbon dioxide (CO2) have increased 40% over the last century and the amount of N cycling in the biosphere has more than doubled. In an effort to understand how plants will respond to continued global CO2 fertilization, long-term free-air CO2 enrichment experiments have been conducted at sites around the globe. Here we examine how atmospheric CO2 enrichment and N fertilization affects the uptake of silicon (Si) in the Duke Forest, North Carolina, a stand dominated by Pinus taeda (loblolly pine), and five hardwood species. Specifically, we measured foliar biogenic silica concentrations in five deciduous and one coniferous species across three treatments: CO2 enrichment, N enrichment, and N and CO2 enrichment. We found no consistent trends in foliar Si concentration under elevated CO2, N fertilization, or combined elevated CO2 and N fertilization. However, two-thirds of the tree species studied here have Si foliar concentrations greater than well-known Si accumulators, such as grasses. Based on net primary production values and aboveground Si concentrations in these trees, we calculated forest Si uptake rates under control and elevated CO2 concentrations. Due largely to increased primary production, elevated CO2 enhanced the magnitude of Si uptake between 20 and 26%, likely intensifying the terrestrial silica pump. This uptake of Si by forests has important implications for Si export from terrestrial systems, with the potential to impact C sequestration and higher trophic levels in downstream ecosystems.
Introduction
We are currently conducting a global experiment by exposing Earth’s biosphere to atmospheric carbon dioxide (CO2) concentrations unseen since the early Miocene, some 23 million years ago (Pearson and Palmer, 2000). From the start of the Industrial Revolution we have increased CO2 concentrations by approximately 40% (Pearson and Palmer, 2000) and in the spring of 2014 CO2 levels officially exceeded 400 ppm at the Mauna Loa Observatory (NOAA, 2014). This grand experiment will continue for the foreseeable future, as over the next century CO2 concentrations are expected to increase further (IPCC, 2013).
The impact of increased CO2 concentrations on the terrestrial biosphere has received much research attention over the last several decades. In particular, recent research has focused on how plants have and will respond to rapid CO2 concentration increases in combination with other regional and global climate changes, including warming air temperature and increased N availability (Norby and Luo, 2004; Ainsworth and Long, 2005; Bernhardt et al., 2006; Finzi et al., 2006). Among numerous other impacts, CO2 enrichment can also alter plant stoichiometry (Loladze, 2002). Exposure to elevated CO2 concentrations can cause declines in leaf nutrients, such as N and phosphorus, as well as trace element concentrations (Taub et al., 2008). The mechanism driving this decline is unclear but it has been attributed to nutrient limitation, increased non-structural carbohydrates, lower transpiration rates, or changes in nutrient allocation patterns (Roberntz and Linder, 1999). A compilation of studies on herbaceous and woody plants found that elevated CO2 concentrations decreased foliar element concentrations by up to 15% (Loladze, 2002). Research on rice, a key global crop, found similar declines in essential elements such as N, magnesium, and iron. Such declines in plant elemental concentrations could have serious repercussions for higher trophic levels, including exacerbation of human malnutrition (Loladze, 2002). A meta-analysis by Cotrufo et al. (1998a) found that aboveground N content declined on average by 14% under high CO2 concentrations. Changes in the N content of litter have also been shown to alter rates of decomposition and thus nutrient cycling within terrestrial ecosystems (Cotrufo et al., 1998b). However, changes in foliar elemental composition could also have large-scale impacts on the cycling and transport of nutrients from land to the sea.
Silicon (Si) is the seventh-most-abundant element in the universe and the second-most abundant element in soils, the mineral substrate for most of terrestrial plant life (Epstein, 1994; Tréguer and De La Rocha, 2013). In the ocean, Si is a key nutrient required for diatoms and is used by many species of sponges, radiolarians, silicoflagellates, choanoflagellates, and even picocyanobacteria (Baines et al., 2012). Of particular importance are diatoms, as they form the base of many productive marine food webs and they sequester significant amounts of C to the deep ocean. In fact, a recent modeling effort contributes 50% of global ocean productivity to diatoms (Rousseaux and Gregg, 2013). The primary source of Si to the ocean is the transport of dissolved and biogenic silica (BSi; SiO2) via rivers, which together account for 78% of the net annual Si oceanic inputs (Tréguer and De La Rocha, 2013). The Si transported by rivers ultimately comes from the weathering of the lithosphere, which is dependent on complex interactions between climate, geology, and biology (Bluth and Kump, 1994; Conley, 2002; Derry et al., 2005). Recently there has been emphasis on the role of biology in altering the timing and magnitude of Si export, specifically in terms of biological uptake by terrestrial vegetation (Conley, 2002; Fulweiler and Nixon, 2005; Gérard et al., 2008; Carey and Fulweiler, 2013b) and the role of human activities in directly altering watershed Si export (Clymans et al., 2011; Carey and Fulweiler, 2012a, 2013b; Vandevenne et al., 2012).
Plants readily absorb dissolved silica (DSi), also known as silicic acid (H4SiO4), the dominant form of Si in soil solutions (Epstein, 1994). DSi is taken up with water and carried in the transpiration stream where, with the evaporation of water, it becomes supersaturated and precipitated as BSi or phytoliths (Raven, 2003). Si provides numerous benefits to vegetation including increased resistance to bacteria, fungi, and grazers, as well protection from desiccation and metal toxicity (Hodson and Evans, 1995; Epstein, 1999; Wieczorek et al., 2014). Si is found throughout plants, from their roots to their shoots, but peak concentrations are generally observed at the transpiration termini (Canny, 1990). In fact, Si can compose 10% or more of the dry weight, exceeding those concentrations of well-known macronutrients (i.e., N and potassium; Epstein, 1994). In turn, the accumulation of Si by terrestrial vegetation over the seasonal cycle has the capacity to regulate the watershed export of Si to coastal ecosystems as plants grow and senesce (Fulweiler and Nixon, 2005; Carey and Fulweiler, 2013b). Alternatively, because BSi is 7–20 times more soluble than mineral silicates (Cornelis et al., 2010a), plants may also provide an important source of Si on biological times scales.
Within this context we wanted to determine if trees exposed to elevated CO2 concentrations would exhibit a decline in foliar Si content like those observed for other elements. To do this, we analyzed BSi concentrations of leaf samples from coniferous and deciduous trees from the Duke free-air CO2 enrichment (FACE) experiments in North Carolina. Additionally, we examined Si content under nitrogen (N) enrichment, as well as under the combined impact of CO2 and N enrichment. This is the first study to specifically examine the role of CO2 and N enrichment on Si content in trees.
Materials and Methods
The Duke Face experiment is located in a Pinus taeda L. (P. taeda, loblolly pine) plantation at Duke University in North Carolina (35°58′N, 79°06′W). This plantation was established in 1983 and is characterized as having moderately low-fertility and acidic clay loam (McCarthy et al., 2010). In addition to the dominant pine, deciduous species present include Acer rubrum (A. rubrum, red maple), Cercis canadensis (C. Canadensis, red bud), Cornus florida (C. florida, dogwood), Liquidambar styraciflua (L. styraciflua, sweet gum), and Ulmus alata (U. alata, winged Elm). Mean annual precipitation is 1145 mm.
This study was conducted on leaves collected from CO2 and N enrichment experiments that took place between 1996 and 2006. The technical details of these studies have been published previously (e.g., Andrews et al., 2000; Luo et al., 2003; Finzi et al., 2007; McCarthy et al., 2010). Briefly, triplicate 30 m diameter treatment plots were exposed to current +200 ppm of CO2 above ambient during daylight hours in the growing season. Control plots (n = 3) were treated in a similar manner but with the addition of ambient air instead of CO2 (McCarthy et al., 2010). In 1998, two of these plots were divided in half and N fertilization began (11.2 g N m-2 y-1 as urea). For more detailed information on the experimental design see http://face.env.duke.edu.
For this analysis we used dried green leaf samples from P. taeda and the five broadleaf species listed above, collected in 2002–2003 and 2006, respectively. P. taeda samples (n = 31) were separated and ground into a powder using a mortar and pestle. The pre-milled deciduous samples (n = 98) each contained composites of several individuals.
Biogenic Si concentrations were determined using a wet alkaline chemical extraction in a 1% Na2CO3 solution (Demaster, 1981; Conley and Schelske, 2001). Duplicate samples were weighed to approximately 30 mg (between 28 and 34 mg) and digested in flat bottomed polyethylene bottles in a shaker bath at 85°C and 100 rpm for four hours. We used a Seal AA3 flow injection autoanalyzer to colorimetrically determine DSi from the BSi aliquots using the molybdenum blue colorimetric method (Strickland and Parsons, 1972). Standards made of sodium hexafluorosilicate (Na2SiF6) as well as external standards were used throughout the analysis to check accuracy and were always within 4% of the expected value. We report all BSi values as %Si by dry weight.
All statistical analyses were completed using JMP Pro 10.0 and significance was judged with an alpha of 0.05. BSi concentrations across species and treatments exhibited equal variances according to several commonly used unequal variance tests (O’Brien = 0.6385, Brown-Forsythe = 0.3196, Levene = 0.1978). To explore potential drivers of BSi concentrations we used a linear mixed effects model to address if the BSi concentrations were different across species and treatment alone and combined. In this model we treated station as a random effect to assess the potential random station effects of the block design used at the Duke FACE experiment. We used an ANOVA to further explore differences in BSi concentrations across species and followed it by a post hoc means comparison with Tukey’s test for honestly significant differences (HSD).
Results
Across all sample types and treatments BSi values ranged from 0.05 to 3.01 %Si dry wt., with a median of 0.82 %Si dry wt. and mean of 0.98 %Si dry wt. C. florida exhibited the lowest and least variable BSi concentrations, while U. alata had the highest (Table 1; Figure 1). While some species did exhibit a decline in foliar BSi concentrations under elevated CO2, we found no statistically significant effect of treatment on foliar BSi concentrations (Table 1). In fact, our least squared model showed no effect of treatments, station, or treatment by species.
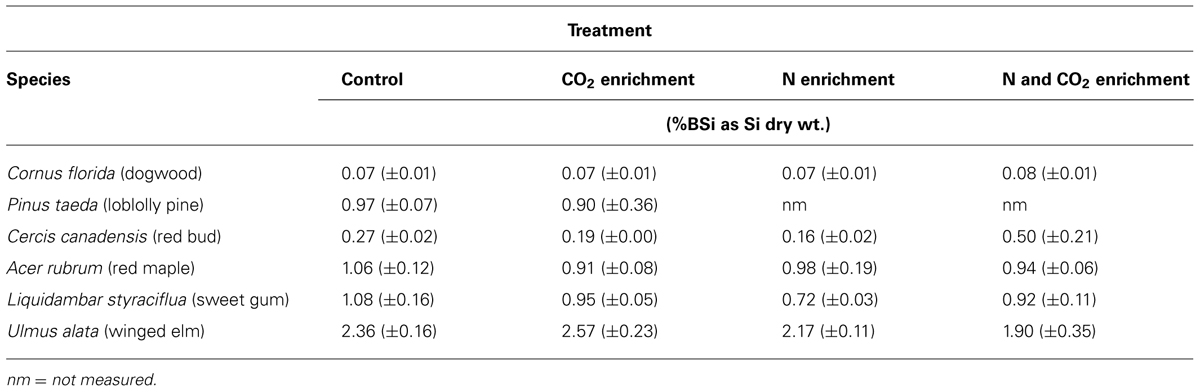
TABLE 1. The mean foliar biogenic silica (BSi) concentrations (%Si by dry wt. ± SE) under the three treatment types and the control for five deciduous and one coniferous species at the Duke FACE experimental forest.
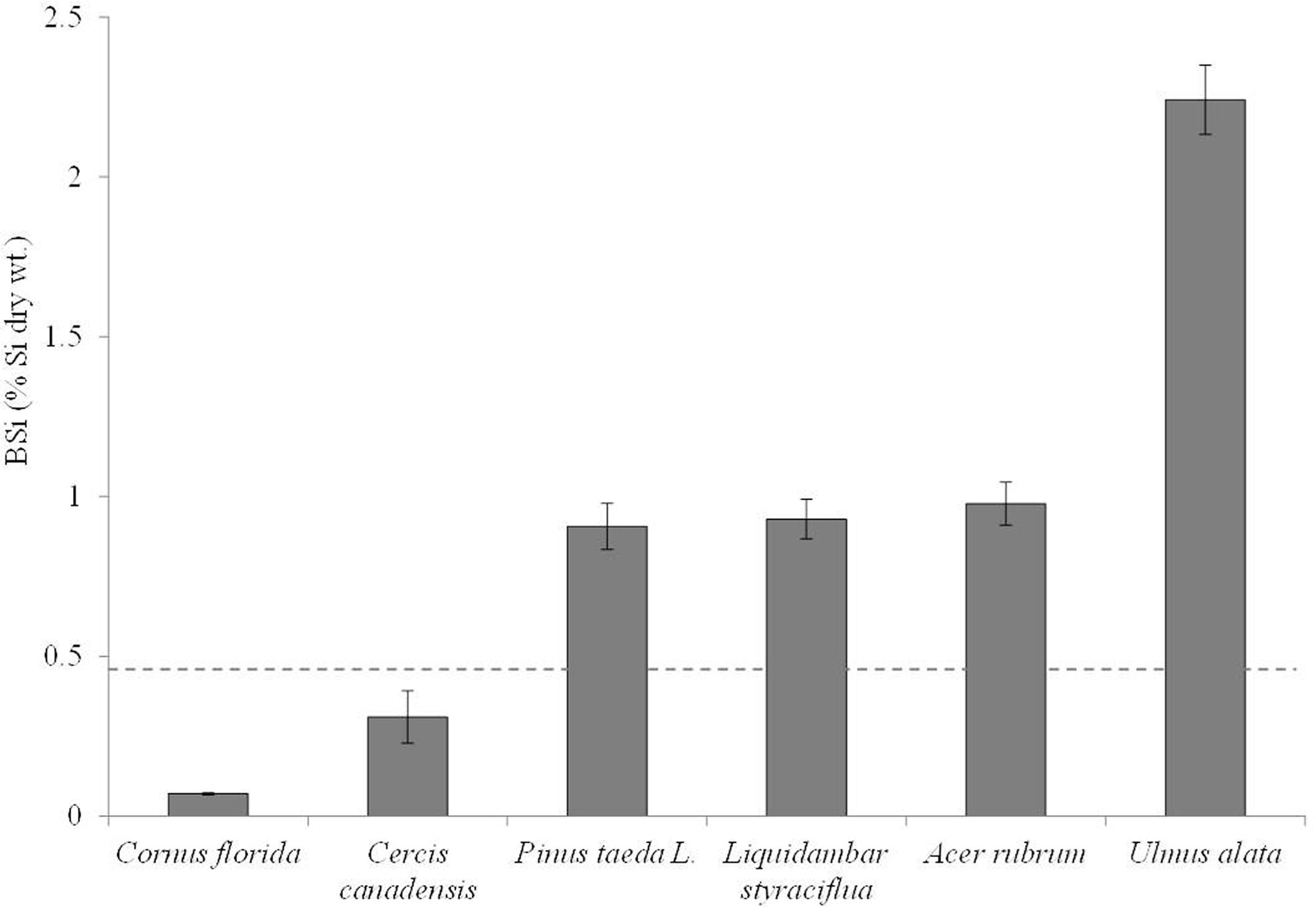
FIGURE 1. Mean BSi concentrations (as %Si by dry wt.) in ascending order for the species analyzed in this study. The most simplistic definition of active Si accumulation in plants is defined as above ground %Si > 0.46 which is shown here with the dashed line.
Because of the lack of statistical difference in plant BSi concentrations across all experimental treatments, we averaged BSi concentrations by species. Clear species differences exist (Figure 1), as the majority of species are statistically different from one another (p < 0.0001). The exceptions are that P. taeda, A. rubrum, and L. styraciflua are not statistically different from each other, but they are statistically different from C. florida, C. canadensis, and U. alata. U. alata exhibited BSi concentrations statistically higher than all other species (p < 0.0001). In all cases, except for C. and C. canadensis, the mean Si foliar content suggests active accumulation (i.e., %Si above 0.46%; Figure 1).
Discussion
In this study we examined foliar BSi concentrations in five deciduous and one coniferous species across three treatments: CO2 enrichment, N enrichment, and N and CO2 enrichment. We expected to see a decrease in Si content as CO2 increased, compared to the control group, as this phenomenon has been observed for a range of other elements such as N, phosphorus, iron, and zinc (Loladze, 2002). In four species (P. taeda, C. canadensis, A. rubrum, L. styraciflua) we did observe a decline in BSi concentrations under elevated CO2 but none of them were statistically significant (Table 1). The largest decrease of 35% was observed in C. canadensis while the others ranged from 7 to 15%. These declines are on par with those reported for essential elements in other plant species and thus, the lack of significance may simply be due to a limited sample number. In one species, U. alata, we observed a small, non-significant increase in BSi concentrations under CO2 enrichment (Table 1). We know of only one other study that examined the impact of elevated CO2 on plant BSi concentrations. In that study of cotton (Gossypium hirsutum L.cv. Deltapine 77), the Si concentrations increased by 26%, although the results were also not significant (Huluka et al., 1994).
We observed a decline in BSi concentrations in N enriched trees compared to the control ranging from 8 to 50%, but none were statistically significant (Table 1). Similarly, no significant differences between BSi concentration in CO2 enriched vs. N enriched were found for any of the species. The combined impact of N and CO2 enrichment again exhibited mixed results. For three species (A. rubrum, L. styraciflua, U. alata) we observed BSi declines from 12 to 21% and in one species (C. canadensis) we observed an increase of 60% (Table 1). Previous work at this same site found no significant differences in leaf N, phosphorus, C, lignin, or total non-structural carbohydrates under elevated CO2 (Finzi et al., 2001). This is in contrast to other studies that found decreases in N and increases in C concentrations in other tree species (quaking aspen, Populus tremuloides and paper birch, Betula papyrifera; Lindroth et al., 2001). Elevated CO2 concentrations also decreased concentrations of N, potassium, phosphorus, and sulfur in Norway spruce (Picea abies L. Karst; Roberntz and Linder, 1999). From these and many other studies, foliar chemistry response to elevated CO2 and N appears to be site-specific. Additionally, although we did not observe statistically significant effects, the trends we document may be ecologically important. For example, Si provides defense against herbivory and the 15% decline we observed in A. rubrum could impact feeding preferences of vertebrate and invertebrate consumers. The lack of significance may be in part driven by our sample numbers and thus, a larger study with more data may produce different results.
Silicon uptake by plants is divided into three broad categories (active, passive, or rejective). In active accumulation, plants acquire more Si than they would through water uptake alone. In rejective accumulation, also known as excluder accumulation, Si is taken up at a slower rate than water. Finally, in passive accumulation, water and Si have similar uptake rates (Raven, 1983; Ma et al., 2001b). Plants are assigned one of these categories according to various definitions, a thorough discussion of which is beyond the scope of this paper, but see Carey and Fulweiler (2014) in this Special Issue for a full description. Briefly, previous definitions have been based on Si concentrations in aboveground tissues, on the ratio of Si to calcium, and on the relationship between porewater Si concentration and aboveground tissue Si concentrations (e.g., Jones and Handreck, 1967; Takahashi et al., 1990; Ma et al., 2001a, respectively). More recent work has focused on the presence/absence of Si transporter genes in roots (Lsi1 and Lsi2) and shoots (Lsi6) of rice (Ma et al., 2008; Yamaji et al., 2008). Research on accumulation modes in trees is surprisingly lacking. Cornelis et al. (2010b) observed both passive and rejective Si accumulation growth in coniferous tree saplings grown hydroponically. And a decrease in Si concentration with depth was observed in a temperate coniferous forest and designated as active accumulation (Gérard et al., 2008). Adult trees likely rely more heavily on groundwater and thus, in order to precisely determine the mode of Si accumulation, measurements of Si concentrations in groundwater, porewater, and within the tree are needed. Unfortunately, this is beyond the scope of this paper. Therefore, we apply the simplest definition that describes accumulation status as a function of Si concentration in the aboveground tissue alone: active accumulators as >0.46% Si by wt., passive accumulators as between 0.25 and 0.46% Si by wt., and excluders as <0.25% Si by wt. (Ma et al., 2001b; Street-Perrott and Barker, 2008). We acknowledge the limitations of this definition, as aboveground BSi tissue concentrations can be impacted by numerous factors, such as porewater DSi availability and external stressors. However, given our dataset, it is the one most appropriate for us to use. Defining Si accumulation status in trees by linking Si concentrations in aboveground vegetation to changes in porewater and groundwater, and locating Si transporter genes within trees are important areas of future research.
We observed a wide range in foliar BSi concentrations (Table 1). The low Si concentrations found in C. florida may indicate that these trees excluded H4SiO4. Overall however, the BSi concentrations observed in the Duke forest are 40 to 150% higher than those previously reported for similar species (Hodson et al., 2005). One reason for higher concentrations found in the Duke Forest could be the different soil and climate in North Carolina compared to the majority of studies reported in Hodson et al. (2005), which were dominated by northern temperate field sites. Our samples could also have higher BSi concentrations because of the land use legacy at the Duke Face site. In 1983, just 13 years before these experiments started, the forest was cut, trunks were removed, and the remaining material was burned (Finzi et al., 2001). The impact of land use change and disturbance on Si cycling is an emerging topic. From what we currently know, greater DSi losses have been observed following deforestation (Likens et al., 1970; Conley et al., 2008).
The median and mean BSi concentration (0.81 and 0.98 %Si dry wt., respectively) of the species we studied here is higher than many well-known actively Si accumulating groups, including those found in grasses (Poaceae) and sedges (Cyperaceae; Jones and Handreck, 1967; Raven, 1983; Ma and Takahashi, 2002). Uptake by forest trees has been hypothesized as a mechanism responsible for both the clear seasonal cycle of Si concentrations in stream water (Fulweiler and Nixon, 2005) and the observation of forested watersheds exporting significantly less Si than watersheds dominated by urban-land uses (Carey and Fulweiler, 2012a, 2013b). Given these data, and the known limitations of Si accumulation definitions, we can only hypothesize that this forest is actively accumulating Si. Regardless, the high Si concentrations we observed support the idea that forests are a critical component in regulating the flux of Si from land to the sea.
Duke Face Si Uptake
The impact of elevated CO2, N, and combined elevated CO2 and N led to an approximately 28% increase in net primary production (NPP) at the Duke Face site between 1996 and 2004 (McCarthy et al., 2010). We used the mean NPP over this period to estimate the amount of Si taken up by the Duke forest for the control and elevated CO2 treatments. We focused on the CO2 treatment because we do not have BSi concentrations for the P. taeda under N fertilization or the combined elevated CO2 and N fertilization treatments. Additionally, biomass at this site is dominated by P. taeda, which comprises ∼98% of the tree basal area (Finzi et al., 2001). We calculated foliar Si:C ratios by dividing the treatment specific median %Si value of either P. taeda alone or all the species together by 0.47, as C concentrations in biomass are well constrained between 45 and 50%. We then multiplied this Si:C ratio by the known amount of C in each treatment to get a treatment specific Si foliar uptake value (Conley, 2002; Carey and Fulweiler, 2012b). We estimated woody biomass Si uptake using the mean temperate woody biomass %Si of 0.08 (Fulweiler and Nixon, 2005) and again divided by 0.47. We then prorated the amount of Si by the proportion of leaves (30%) versus woody biomass (70%) in a typical forest to determine a total Si uptake rate (Figure 2; Litton et al., 2007). Although we observed a decline in Si concentrations under elevated CO2, the total amount of Si taken up by P. taeda was 26% higher at elevated CO2 where NPP was significantly higher compared to ambient CO2 (control: 194 kmol Si km-2, elevated CO2: 251 kmol Si km-2). Together with the hardwood species, Si uptake rate was 20% higher in the elevated compared to ambient CO2 treatment, although absolute rates of uptake were higher than P. taeda alone (elevated CO2 = 313 kmol km-2 y-1; ambient CO2 = 266 kmol Si km-2, Figure 2). These values are on the high end of those reported for forested systems but well within the reported range. For example, Gérard et al. (2008) reported 157 kmol Si km-2 for a Douglas fir forest in France, while Meunier et al. (1999) reported an uptake of over 3400 kmol Si km-2 in a bamboo forest.
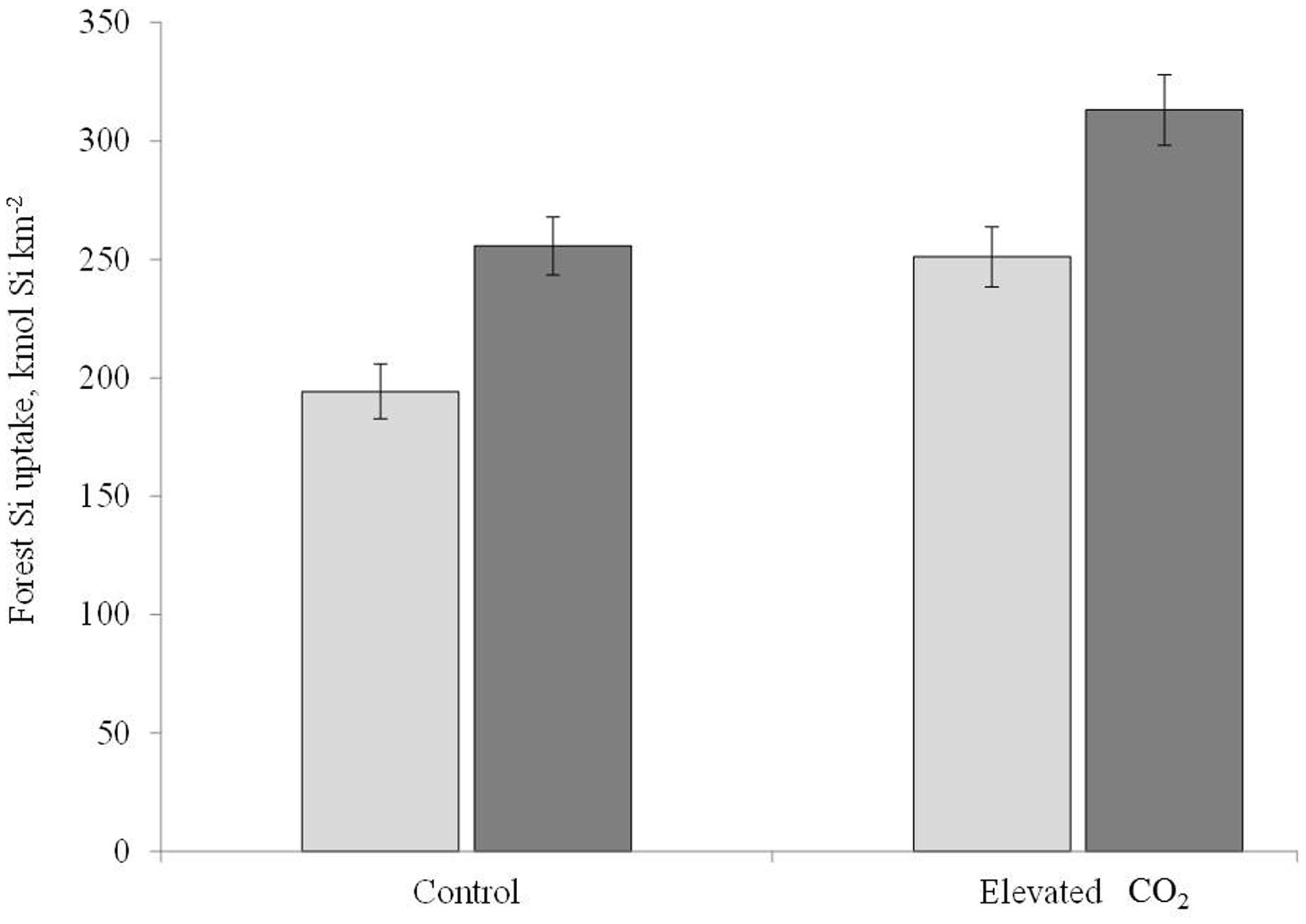
FIGURE 2. A comparison between the control and elevated CO2 treatment of forest Si accumulation for the loblolly pine alone (light gray) and for all the species together (dark gray).
A review of FACE experiments found that irrespective of ecosystem type, aboveground production increased in the presence of higher CO2 and trees were more responsive than herbaceous vegetation (Ward et al., 2013). Other studies have found similar increases in global terrestrial production (Melillo et al., 1993; Nemani et al., 2003). In addition, Pan et al. (2013) found that global forest biomass has increased in established forests. Such changes in primary productivity will also alter Si cycling. In fact, Si accumulating vegetation accounts for 55% of terrestrial NPP (33 Gton C y-1) an amount similar to the C sequestered by marine diatoms (Carey and Fulweiler, 2012b). This terrestrial Si pump has important implications for global climate, as Si cycling helps to control atmospheric CO2 concentrations through a variety of mechanisms, including chemical weathering of mineral silicates and C occlusion in soil phytoliths (Berner and Berner, 1997; Parr and Sullivan, 2005). In addition, this vegetation also plays a critical role in modulating the amount and timing of Si export from watersheds to downstream receiving waters (Carey and Fulweiler, 2012a, 2013b), which has direct implications for marine C dynamics. Here, we show that anthropogenically driven enhanced NPP may result in an increase in the terrestrial Si pump as forests take up more Si. In turn, the increased terrestrial Si sink may alter Si availability in aquatic systems. Diatoms require N and Si on a one to one molar basis (Redfield, 1963). Thus, the ratio of N to phosphorus to Si (N:P:Si) helps to control the composition and abundance of phytoplankton species assemblages. Human activities, such as fertilizer use and land use change have increased N and P loading to coastal systems worldwide. Phytoplankton respond to these elevated nutrients by increased productivity. At first, diatoms will bloom until all the Si is consumed at which point other non-Si requiring species will flourish (Anderson et al., 2002). The enhanced Si uptake by forests under elevated CO2 may be another way in which humans are altering nutrient stoichiometry in coastal receiving waters.
Missing from this discussion is the mechanism driving our findings that CO2 and/or N additions have no significant impact on Si accumulation in aboveground biomass. Of course, with more data and different study sites we might observe a significant impact of these factors on Si accumulation rates in forest or other terrestrial vegetation types. In fact, a recent study found higher Si concentrations in the porewater, sediment, roots, and occasionally the aboveground biomass of a heavily N enriched salt marsh (Carey and Fulweiler, 2013a). Certainly other factors such as changes in water availability and growing season length, as well as warming temperatures, are all factors that might influence foliar BSi accumulation. Here we propose an alternative idea for exploration: we hypothesize that in ecosystems with altered transpiration rates, corresponding changes to leaf Si concentrations will also be observed because Si is delivered to vegetation via water. Higher transpiration rates should, if passive or active accumulation is occurring, result in higher Si concentration in leaves, as Si is typically concentrated at transpiration termini. Conversely, if transpiration rates decline, then less Si will be deposited. Experimental work on Douglas fir saplings found higher Si concentrations that were attributed to greater transpiration in those seedlings (Cornelis et al., 2010b). However, at the Duke FACE experimental site no changes in transpiration were observed and maybe this is why we observed no significant changes in Si concentration (Ward et al., 2013). Complicating these simple ideas is the original hypothesis that motivated this work – enhanced CO2 lowers elemental composition of leaves such as N, phosphorus, and potassium. Thus, quantifying the interplay between structural changes in vegetation and transpiration rates, as well as water availability under future climate change scenarios, will be a critical next step in our understanding of climate change impacts on terrestrial Si cycling.
Conclusion
Our data of BSi concentrations in 6 species from the Duke FACE Experiment does not support our initial hypothesis that elevated CO2 concentrations would decrease foliar Si content. In fact, we observed no consistent or significant impact of any of the treatments on foliar Si content. However, according to the simplest definition based on aboveground tissue Si concentrations, we did find evidence that four out of the six tree species we studied may be active Si accumulators. These tree species had Si concentrations higher than some of the most well-known Si accumulators (e.g., grasses and sedges). Further, the higher NPP values observed under elevated CO2 resulted in higher Si uptake rates under elevated CO2 conditions in the Duke forest. Based on this analysis we hypothesize that anthropogenic change, specifically elevated atmospheric CO2 concentrations, may increase biological Si pumping in forests, increasing the magnitude of the terrestrial Si pump.
Conflict of Interest Statement
The authors declare that the research was conducted in the absence of any commercial or financial relationships that could be construed as a potential conflict of interest.
Acknowledgments
This research was supported in part by the Sloan Foundation in a fellowship to Robinson W. Fulweiler. The Duke Forest FACE was supported by his study was supported by the US Department of Energy (Grant No. DE-FG02-95ER62083) through the Office of Biological and Environmental Research (BER) and its National Institute for Global Environmental Change (NIGEC), Southeast Regional Center (SERC) at the University of Alabama, and by the US Forest Service through both the Southern Global Climate Change Program and the Southern Research Station. Adrien C. Finzi acknowledges ancillary support from the US NSF (DEB0236356). Thanks to Jeffrey Pippen for his assistance with the field sampling at Duke. We also thank Ken Czapla for training Timothy J. Maguire on the Seal Autoanalyzer and running many of the Si samples. We thank Hollie Emery for her statistical guidance. We thank our two reviewers who provided helpful suggestions and edits that improved our manuscript.
References
Ainsworth, E. A., and Long, S. P. (2005). What have we learned from 15 years of free-air CO2 enrichment (FACE)? A meta-analytic review of the responses of photosynthesis, canopy properties and plant production to rising CO2. New Phytol. 165, 351–371. doi: 10.1111/j.1469-8137.2004.01224.x
Pubmed Abstract | Pubmed Full Text | CrossRef Full Text | Google Scholar
Anderson, D. M., Glibert, P. M., and Burkholder, J. M. (2002). Harmful algal blooms and eutrophication: nutrient sources, composition, and consequences. Estuaries 25, 704–726. doi: 10.1007/Bf02804901
Andrews, J. A., Matamala, R., Westover, K. M., and Schlesinger, W. H. (2000). Temperature effects on the diversity of soil heterotrophs and the delta C-13 of soil-respired CO2. Soil Biol. Biochem. 32, 699–706. doi: 10.1016/S0038-0717(99)00206-0
Baines, S. B., Twining, B. S., Brzezinski, M. A., Krause, J. W., Vogt, S., Assael, D.,et al. (2012). Significant silicon accumulation by marine picocyanobacteria. Nat. Geosci. 5, 886–891. doi: 10.1038/ngeo1641
Berner, R. A., and Berner, E. K. (1997). “Silicate weathering and climate,” in Tectonic Uplift and Climate Change, ed. W. F. Ruddiman (New York, NY: Springer), 353–365. doi: 10.1007/978-1-4615-5935-1_15
Bernhardt, E. S., Barber, J. J., Pippen, J. S., Taneva, L., Andrews, J. A., and Schlesinger, W. H. (2006). Long-term effects of free air CO2 enrichment (FACE) on soil respiration. Biogeochemistry 77, 91–116. doi: 10.1007/s10533-005-1062-0
Bluth, G. J. S., and Kump, L. R. (1994). Lithologic and climatologic controls of river chemistry. Geochim. Cosmochim. Acta 58, 2341–2359. doi: 10.1016/0016-7037(94)90015-9
Canny, M. (1990). Tansley Review No. 22. What becomes of the transpiration stream? New Phytol. 114, 341–368. doi: 10.1111/j.1469-8137.1990.tb00404.x
Carey, J. C., and Fulweiler, R. W. (2012a). Human activities directly alter watershed dissolved silica fluxes. Biogeochemistry 111, 125–138. doi: 10.1007/s10533-011-9671-2
Carey, J. C., and Fulweiler, R. W. (2012b). The terrestrial silica pump. PLoS ONE 7:e52932. doi: 10.1371/journal.pone.0052932
Pubmed Abstract | Pubmed Full Text | CrossRef Full Text | Google Scholar
Carey, J. C., and Fulweiler, R. W. (2013a). N enrichment increases net silica accumulation in a temperate salt marsh. Limnol. Oceanogr. 58, 99–111. doi: 10.4319/lo.2013.58.1.0099
Carey, J. C., and Fulweiler, R. W. (2013b). Watershed land use alters riverine silica cycling. Biogeochemistry 113, 525–544. doi: 10.1007/s10533-012-9784-2
Carey, J. C., and Fulweiler, R. W. (2014). Silica uptake by Spartina-evidence of multiple modes of accumulation from salt marshes around the world. Front. Plant Sci. 5:186. doi: 10.3389/Fpls.2014.00186
Pubmed Abstract | Pubmed Full Text | CrossRef Full Text | Google Scholar
Clymans, W., Struyf, E., Govers, G., Vandevenne, F., and Conley, D. J. (2011). Anthropogenic impact on amorphous silica pools in temperate soils. Biogeosciences 8, 2281–2293. doi: 10.5194/bg-8-2281-2011
Conley, D. J. (2002). Terrestrial ecosystems and the global biogeochemical silica cycle. Global Biogeochem. Cycles 16:1121. doi: 10.1029/2002gb001894
Pubmed Abstract | Pubmed Full Text | CrossRef Full Text | Google Scholar
Conley, D. J., Likens, G. E., Buso, D. C., Saccone, L., Bailey, S. W., and Johnson, C. E. (2008). Deforestation causes increased dissolved silicate losses in the Hubbard Brook Experimental Forest. Global Change Biol. 14, 2548–2554. doi: 10.1111/j.1365-2486.2008.01667.x
Conley, D. J., and Schelske, C. L. (2001). “Biogenic silica” in Tracking Environmental Change Using Lake Sediments. Terresterial, Algal, and Siliceous Indicators, eds J. P. Smol, H. J. B. Birks, and W. M. Last (Dordrecht: Kluwar).
Cornelis, J. T., Ranger, J., Iserentant, A., and Delvaux, B. (2010a). Tree species impact the terrestrial cycle of silicon through various uptakes. Biogeochemistry 97, 231–245. doi: 10.1007/s10533-009-9369-x
Cornelis, J. T., Delvaux, B., and Titeux, H. (2010b). Contrasting silicon uptakes by coniferous trees: a hydroponic experiment on young seedlings. Plant soil 336, 99–106. doi: 10.1007/s11104-010-0451-x
Cotrufo, M. F., Ineson, P., and Scott, A. (1998a). Elevated CO2 reduces the N concentration of plant tissues. Glob. Chang. Biol. 4, 43–54. doi: 10.1046/j.1365-2486.1998.00101.x
Cotrufo, M. F., Briones, M. J. I., and Ineson, P. (1998b). Elevated CO2 affects field decomposition rate and palatability of tree leaf litter: importance of changes in substrate quality. Soil Biol. Biochem. 30, 1565–1571. doi: 10.1016/S0038-0717(98)00032-7
Demaster, D. J. (1981). The supply and accumulation of silica in the marine environment. Geochim. Cosmochim. Acta 45, 1715–1732. doi: 10.1016/0016-7037(81)90006-5
Derry, L. A., Kurtz, A. C., Ziegler, K., and Chadwick, O. A. (2005). Biological control of terrestrial silica cycling and export fluxes to watersheds. Nature 433, 728–731. doi: 10.1038/nature03299
Pubmed Abstract | Pubmed Full Text | CrossRef Full Text | Google Scholar
Epstein, E. (1994). The anomaly of silicon in plant biology. Proc. Natl. Acad. Sci. U.S.A. 91, 11–17. doi: 10.1073/Pnas.91.1.11
Pubmed Abstract | Pubmed Full Text | CrossRef Full Text | Google Scholar
Epstein, E. (1999). Silicon. Annu. Rev. Plant Phys. 50, 641–664. doi: 10.1146/annurev.arplant.50.1.641
Pubmed Abstract | Pubmed Full Text | CrossRef Full Text | Google Scholar
Finzi, A. C., Allen, A. S., Delucia, E. H., Ellsworth, D. S., and Schlesinger, W. H. (2001). Forest litter production, chemistry, and decomposition following two years of free-air CO2 enrichment. Ecology 82, 470–484. doi: 10.1890/0012-9658(2001)082[0470:Flpcad]2.0.Co;2
Finzi, A. C., Moore, D. J. P., Delucia, E. H., Lichter, J., Hofmockel, K. S., Jackson, R. B.,et al. (2006). Progressive N limitation of ecosystem processes under elevated CO2 in a warm-temperate forest. Ecology 87, 15–25. doi: 10.1890/04-1748
Finzi, A. C., Norby, R. J., Calfapietra, C., Gallet-Budynek, A., Gielen, B., Holmes, W. E.,et al. (2007). Increases in N uptake rather than N-use efficiency support higher rates of temperate forest productivity under elevated CO2. Proc. Natl. Acad. Sci. U.S.A. 104, 14014–14019. doi: 10.1073/pnas.0706518104
Pubmed Abstract | Pubmed Full Text | CrossRef Full Text | Google Scholar
Fulweiler, R. W., and Nixon, S. W. (2005). Terrestrial vegetation and the seasonal cycle of dissolved silica in a southern New England coastal river. Biogeochemistry 74, 115–130. doi: 10.1007/s10533-004-2947-z
Gérard, F., Mayer, K. U., Hodson, M. J., and Ranger, J. (2008). Modelling the biogeochemical cycle of silicon in soils: application to a temperate forest ecosystem. Geochim. Cosmochim. Acta 72, 741–758. doi: 10.1016/j.gca.2007.11.010
Hodson, M. J., and Evans, D. E. (1995). Aluminum-silicon interactions in higher plants. J. Exp. Bot. 46, 161–171. doi: 10.1093/Jxb/46.2.161
Hodson, M. J., White, P. J., Mead, A., and Broadley, M. R. (2005). Phylogenetic variation in the silicon composition of plants. Ann. Bot. 96, 1027–1046. doi: 10.1093/Aob/Mci255
Pubmed Abstract | Pubmed Full Text | CrossRef Full Text | Google Scholar
Huluka, G., Hileman, D. R., Biswas, P. K., Lewin, K. F., Nagy, J., and Hendrey, G. R. (1994). Effects of elevated CO2 and water-stress on mineral concentration of cotton. Agric. For. Meteorol. 70, 141–152. doi: 10.1016/0168-1923(94)90053-1
IPCC. (2013). “Summary for policymakers,” in Climate Change 2013: The Physical Science Basis. Contribution of Working Group I to the Fifth Assessment Report of the Intergovernmental Panel on Climate Change, eds T. F. Stocker, D. Qin, G.-K. Plattner, M. Tignor, S. K. Allen, J. Boschung,et al. (Cambridge: Cambridge University Press).
Jones, L., and Handreck, K. (1967). Silica in soils, plants, and animals. Adv. Agron. 19, 107–149. doi: 10.1016/S0065-2113(08)60734-8
Likens, G. E., Bormann, F. H., Johnson, N. M., Fisher, D. W., and Pierce, R. S. (1970). Effects of forest cutting and herbicide treatment on nutrient budgets in Hubbard Brook watershed-ecosystem. Ecol. Monogr. 40, 23–47. doi: 10.2307/1942440
Lindroth, R. L., Kopper, B. J., Parsons, W. F. J., Bockheim, J. G., Karnosky, D. F., Hendrey, G. R.,et al. (2001). Consequences of elevated carbons dioxide and ozone for foliar chemical composition and dynamics in trembling aspen (Populus tremuloides) and paper birch (Betula papyrifera). Environ. Pollut. 115, 395–404. doi: 10.1016/S0269-7491(01)00229-9
Pubmed Abstract | Pubmed Full Text | CrossRef Full Text | Google Scholar
Litton, C. M., Raich, J. W., and Ryan, M. G. (2007). Carbon allocation in forest ecosystems. Glob. Chang. Biol. 13, 2089–2109. doi: 10.1111/j.1365-2486.2007.01420.x
Loladze, I. (2002). Rising atmospheric CO2 and human nutrition: toward globally imbalanced plant stoichiometry? Trends Ecol. Evol. 17, 457–461. doi: 10.1016/S0169-5347(02)02587-9
Luo, Y. Q., White, L. W., Canadell, J. G., Delucia, E. H., Ellsworth, D. S., Finzi, A. C.,et al. (2003). Sustainability of terrestrial carbon sequestration: a case study in Duke forest with inversion approach. Global Biogeochem. Cycles 17:1021 doi: 10.1029/2002gb001923
Ma, J., Miyake, Y., and Takahashi, E. (2001a). Silicon as a beneficial element for crop plants. Stud. Plan. S. 8, 17–39. doi: 10.1016/S0928-3420(01)80006-9
Ma, J. F., Goto, S., Tamai, K., and Ichii, M. (2001b). Role of root hairs and lateral roots in silicon uptake by rice. Plant Physiol. 127, 1773–1780. doi: 10.1104/pp.127.4.1773
Pubmed Abstract | Pubmed Full Text | CrossRef Full Text | Google Scholar
Ma, J. F., and Takahashi, E. (2002). Soil, Fertilizer, and Plant Silicon Research in Japan. Amsterdam: Elsevier.
Ma, J. F., Yamaji, N., Mitani, N., Xu, X.-Y., Su, Y.-H., Mcgrath, S. P.,et al. (2008). Transporters of arsenite in rice and their role in arsenic accumulation in rice grain. Proc. Natl. Acad. Sci. U.S.A. 105, 9931–9935. doi: 10.1073/pnas.0802361105
Pubmed Abstract | Pubmed Full Text | CrossRef Full Text | Google Scholar
McCarthy, H. R., Oren, R., Johnsen, K. H., Gallet-Budynek, A., Pritchard, S. G., Cook, C. W.,et al. (2010). Re-assessment of plant carbon dynamics at the Duke free-air CO2 enrichment site: interactions of atmospheric CO2 with N and water availability over stand development. New Phytol. 185, 514–528. doi: 10.1111/j.1469-8137.2009.03078.x
Pubmed Abstract | Pubmed Full Text | CrossRef Full Text | Google Scholar
Melillo, J. M., Mcguire, A. D., Kicklighter, D. W., wMoore, B., Vorosmarty, C. J., and Schloss, A. L. (1993). Global climate-change and terrestrial net primary production. Nature 363, 234–240. doi: 10.1038/363234a0
Meunier, J. D., Colin, F., and Alarcon, C. (1999). Biogenic silica storage in soils. Geology 27, 835–838. doi: 10.1130/0091-7613(1999)027<0835:BSSIS>2.3.CO;2
National Oceanic and Atmospheric Administration (NOAA). (2014). Available at: http://www.esrl.noaa.gov/gmd/ccgg/trends/weekly.html
Nemani, R. R., Keeling, C. D., Hashimoto, H., Jolly, W. M., Piper, S. C., Tucker, C. J.,et al. (2003). Climate-driven increases in global terrestrial net primary production from 1982 to 1999. Science 300, 1560–1563. doi: 10.1126/science.1082750
Pubmed Abstract | Pubmed Full Text | CrossRef Full Text | Google Scholar
Norby, R. J., and Luo, Y. Q. (2004). Evaluating ecosystem responses to rising atmospheric CO2 and global warming in a multi-factor world. New Phytol. 162, 281–293. doi: 10.1111/j.1469-8137.2004.01047.x
Pan, Y. D., Birdsey, R. A., Phillips, O. L., and Jackson, R. B. (2013). The structure, distribution, and biomass of the world’s forests. Annu. Rev. Ecol. Evol. Syst. 44, 593–622. doi: 10.1146/annurev-ecolsys-110512-135914
Parr, J. F., and Sullivan, L. A. (2005). Soil carbon sequestration in phytoliths. Soil Biol. Biochem. 37, 117–124. doi: 10.1016/j.soilbio.2004.06.013
Pearson, P. N., and Palmer, M. R. (2000). Atmospheric carbon dioxide concentrations over the past 60 million years. Nature 406, 695–699. doi: 10.1038/35021000
Pubmed Abstract | Pubmed Full Text | CrossRef Full Text | Google Scholar
Raven, J. A. (1983). The transport and function of silicon in plants. Biol. Rev. 58, 179–207. doi: 10.1111/j.1469-185X.1983.tb00385.x
Raven, J. A. (2003). Cycling silicon – the role of accumulation in plants – commentary. New Phytol. 158, 419–421. doi: 10.1046/j.1469-8137.2003.00778.x
Redfield, A. C., Ketchum, B. H., and Richards, F. A. (1963). “The influence of organisms on the composition of sea-water,” in The Sea, Vol. 2, The Composition of Sea-Water Comparative and Descriptive Oceanography, ed. M. N. Hill (New York: Interscience Publishers), 26–77.
Roberntz, P., and Linder, S. (1999). Effects of long-term CO2 enrichment and nutrient availability in Norway spruce. II. Foliar chemistry. Trees 14, 17–27. doi: 10.1007/s004680050003
Rousseaux, C. S., and Gregg, W. W. (2013). Interannual variation in phytoplankton primary production at a global scale. Remote Sens. 6, 1–19. doi: 10.3390/rs6010001
Street-Perrott, F. A., and Barker, P. A. (2008). Biogenic silica: a neglected component of the coupled global continental biogeochemical cycles of carbon and silicon. Earth Surf. Proc. Land. 33, 1436–1457. doi: 10.1002/esp.1712
Strickland, J. D. H., and Parsons, T. R. (1972). A Practical Handbook of Seawater Analysis, 2nd Edn, Vol. 67 (Ottawa: Fisheries Research Board of Canada), 310.
Takahashi, E., Ma, J., and Miyake, Y. (1990). The possibility of silicon as an essential element for higher plants. Comm. Agric. Food Chem. 2, 99–102.
Taub, D. R., Miller, B., and Allen, H. (2008). Effects of elevated CO2 on the protein concentration of food crops: a meta-analysis. Glob. Chang. Biol. 14, 565–575. doi: 10.1111/j.1365-2486.2007.01511.x
Tréguer, P. J., and De La Rocha, C. L. (2013). The world ocean silica cycle. Annu. Rev. Mar. Sci. 5, 477–501. doi: 10.1146/annurev-marine-121211-172346
Pubmed Abstract | Pubmed Full Text | CrossRef Full Text | Google Scholar
Vandevenne, F., Struyf, E., Clymans, W., and Meire, P. (2012). Agricultural silica harvest: have humans created a new loop in the global silica cycle? Front. Ecol. Environ. 10, 243–248. doi: 10.1890/110046
Ward, E. J., Bell, D. M., Clark, J. S., and Oren, R. (2013). Hydraulic time constants for transpiration of loblolly pine at a free-air carbon dioxide enrichment site. Tree Physiol. 33, 123–134. doi: 10.1093/treephys/tps114
Pubmed Abstract | Pubmed Full Text | CrossRef Full Text | Google Scholar
Wieczorek, M., Zub, K., Szafrańska, P. A., Książek, A., and Konarzewski, M. (2014). Plant–herbivore interactions: silicon concentration in tussock sedges and population dynamics of root voles. Funct. Ecol. doi: 10.1111/1365-2435.12327
Yamaji, N., Mitatni, N., and Ma, J. F. (2008). A transporter regulating silicon distribution in rice shoots. Plant Cell 20, 1381–1389. doi: 10.1105/tpc.108.059311
Pubmed Abstract | Pubmed Full Text | CrossRef Full Text | Google Scholar
Keywords: elevated CO2, silicon, forest Si uptake, terrestrial Si pump, active Si accumulation, Si cycling
Citation: Fulweiler RW, Maguire TJ, Carey JC and Finzi AC (2015) Does elevated CO2 alter silica uptake in trees? Front. Plant Sci. 5:793. doi: 10.3389/fpls.2014.00793
Received: 06 September 2014; Accepted: 19 December 2014;
Published online: 13 January 2015.
Edited by:
Jane DeGabriel, University of Western Sydney, AustraliaReviewed by:
Martin Karl-Friedrich Bader, New Zealand Forest Research Institute Ltd (SCION), New ZealandWim Clymans, Lund University, Sweden
Copyright © 2015 Fulweiler, Maguire, Carey and Finzi. This is an open-access article distributed under the terms of the Creative Commons Attribution License (CC BY). The use, distribution or reproduction in other forums is permitted, provided the original author(s) or licensor are credited and that the original publication in this journal is cited, in accordance with accepted academic practice. No use, distribution or reproduction is permitted which does not comply with these terms.
*Correspondence: Robinson W. Fulweiler, Department of Earth and the Environment, Boston University, 685 Commonwealth Avenue, Boston, MA 02215, USA e-mail: rwf@bu.edu