- 1Key Laboratory of Plant Resources Conservation and Sustainable Utilization, South China Botanical Garden, Chinese Academy of Sciences, Guangzhou, China
- 2College of Life Sciences, University of Chinese Academy of Sciences, Beijing, China
- 3Institute of Plant Biology, National Taiwan University, Taipei, Taiwan
Histone acetylation and deacetylation at the N-terminus of histone tails play crucial roles in the regulation of eukaryotic gene activity. Histone acetylation and deacetylation are catalyzed by histone acetyltransferases and histone deacetylases (HDACs), respectively. A growing number of studies have demonstrated the importance of histone deacetylation/acetylation on genome stability, transcriptional regulation, development and response to stress in Arabidopsis. However, the biological functions of HDACs in tomato have not been investigated previously. Fifteen HDACs identified from tomato (Solanum lycopersicum) can be grouped into RPD3/HDA1, SIR2 and HD2 families based on phylogenetic analysis. Meanwhile, 10 members of the RPD3/HDA1 family can be further subdivided into four groups, namely Class I, Class II, Class III, and Class IV. High similarities of protein sequences and conserved domains were identified among SlHDACs and their homologs in Arabidopsis. Most SlHDACs were expressed in all tissues examined with different transcript abundance. Transient expression in Arabidopsis protoplasts showed that SlHDA8, SlHDA1, SlHDA5, SlSRT1 and members of the HD2 family were localized to the nucleus, whereas SlHDA3 and SlHDA4 were localized in both the cytoplasm and nucleus. The difference in the expression patterns and subcellular localization of SlHDACs suggest that they may play distinct functions in tomato. Furthermore, we found that three members of the RPD3/HDA1 family, SlHDA1, SIHDA3 and SlHDA4, interacted with TAG1 (TOMATO AGAMOUS1) and TM29 (TOMATO MADS BOX29), two MADS-box proteins associated with tomato reproductive development, indicating that these HDACs may be involved in gene regulation in reproductive development.
Introduction
In eukaryote cells, genetic information encoded by DNA is packed extensively forming the chromatin structure. The fundamental unit of chromatin is a nucleosome containing a histone octamer (two copies of histone H2A, H2B, H3, and H4) wrapped on approximately 147 base pairs of DNA (Luger et al., 1997). Each histone has a structured globular domain and an unstructured amino-terminal tail that extends from the core nucleosome (Campos and Reinberg, 2009). The N-terminal tails of histone proteins provide sites for a variety of post-translational modifications, such as acetylation, phosphorylation, methylation, glycosylation, ubiquitation and ADP-ribosylation [3]. Among these modifications, histone acetylation is one of the well characterized post-translational modifications (Allfrey et al., 1964; Lusser et al., 2001).
The acetylation state of the ε-amino group of conserved lysine residues within all four core histones was regulated by the opposing activities of histone acetyltransferases (Brownell et al., 1996) and histone deacetylases (HDACs) (Nagy et al., 1997). HDACs can remove acetyl groups from histone and non-histone substrates including transcription factors and other proteins involved in DNA repair and replication, metabolism, cytoskeleton dynamics, apoptosis and cell signaling (Yang and Seto, 2007). Based on sequence similarity and cofactor dependency, HDACs in all eukaryotes are divided into three families: RPD3/HDA1 (Reduced Potassium Dependence 3/Histone Deacetylase 1), SIR2 (Silent Information Regulator 2), and plant-specific HD2 (Histone Deacetylase 2) (Pandey et al., 2002; Yang and Seto, 2007). Members of the SIR2 family (sirtuins) have a catalytic domain that is characterized by the requirement for nicotine adenine dinucleotide (NAD) as a cofactor (Haigis and Guarente, 2006), while members of the RPD3/HDA1 family share sequence homology in the HDAC domain and require the Zn2+ cofactor for deacetylase activity (Yang and Seto, 2007).
In the past decade, plant HDACs have drawn considerable research attention and an increasing number of HDACs were purified, identified and characterized from plants such as maize, Arabidopsis, rice, barley (Demetriou et al., 2009), potato (Lagace et al., 2003), grape (Busconi et al., 2009), tobacco (Bourque et al., 2011), and tomato (Cigliano et al., 2013b). The Arabidopsis genome encodes 18 HDACs. Twelve of them belong to the RPD3/HDA1 superfamily, two are the SIR2 family and four are members of the plant specific HD2 family (Pandey et al., 2002; Alinsug et al., 2009). The Arabidopsis HDACs play a vital role in regulating gene expression in various biological processes. For instance, HDA6 was involved in transgene silencing and maintain of DNA methylation (Aufsatz et al., 2002; Probst et al., 2004). While HDA19, the closest homolog of HDA6, was shown to be important for proper vegetative development as hda19 mutants displayed various developmental abnormalities (Tian and Chen, 2001; Long et al., 2006; Zhou et al., 2013). HDA18 is required for the cellular patterning in the root epidermis (Xu et al., 2005; Liu et al., 2013a), whereas HDA7 is crucial for female gametophyte development and embryogenesis in Arabidopsis (Cigliano et al., 2013a). Furthermore, HDA14 is an α-tubulin decetylase associated with α/β-tubulin and enriched in microtubule fractions by direct association with the PPP-type phosphatases PP2A (Tran et al., 2012). Silencing of HD2A in Arabidopsis resulted in aborted seed development (Wu et al., 2000), while overexpression of HD2A caused morphological defects of leaves and flowers, delayed flowering and aborted seed development (Zhou et al., 2004). In addition, HD2A and HD2B were found to act independently with ASYMMETRIC LEAVES1 (AS1) and AS2 to control miR165/166 distribution and the development of adaxial–abaxial leaf polarity (Ueno et al., 2007). More recently, the SRT2 in Arabidopsis was showed to be predominantly localized at the inner mitochondrial membrane and to interact with a small number of protein complexes mainly involved in energy metabolism and metabolite transport (Konig et al., 2014).
The molecular mechanisms of fruit development are not well-understood. Genetic studies in Arabidopsis have uncovered the genetic network that patterns the Arabidopsis fruit. For instance, the members of MADS-box transcription factor family, FRUITFULL (FUL) and SHATTERPROOF 1/2 (SHP 1/2), are necessary for proper valve development (Gu et al., 1998; Ferrandiz et al., 2000). Furthermore, INDEHISCENT (IND) and ALCATRAZ (ALC), two bHLH transcription factors, are both necessary for the differentiation of the dehiscence zone between the valve and replum regions (Girin et al., 2011; Groszmann et al., 2011). Previous data also showed that HDACs were involved in fruit development. For instance, AtHDA19 mutations induce embryonic defects and seed set reduction (Tian et al., 2003). Silencing and overexpression of AtHD2A both severely affect seed development (Wu et al., 2000; Zhou et al., 2004). In addition, hda6 mutants were reported to exhibit reduced fertility (Aufsatz et al., 2002), while mutations of AtHDA7 led to partial failure of ovule/female gametophyte development and seed abortion (Cigliano et al., 2013a).
Compared to Arabidopsis, relatively few HDACs were characterized in other plant species. In this study, 15 HDACs were identified in the tomato genome. The expression patterns and subcellular localization of tomato HDACs (SlHDACs) were investigated. Furthermore, the interaction between SlHDACs and the MADS-box proteins involved in reproductive development sheds light on potential functions of SlHDACs during reproductive development.
Materials and Methods
Plant Material and Growth Conditions
Solanum lycopersicum cultivar Henz1706 was kindly provided by Wang Ying (South China Botanical Garden). Plants were grown in soil in a controlled-environment greenhouse with a long photoperiod (16 h light/8 h dark) at 23 ± 1°C. The tomato seeds were accelerated germination by putting the seeds in a 28°C incubator for 2 days before sowing.
Identification of SlHDAC Genes
The HDACs sequences of Arabidopsis thaliana obtained in the TAIR database (http://www.arabidopsis.org/) were used to perform a search in the Solamum lycopersicum genome using the BLASTP program in the SGN browser (http://solgenomics.net/tools/blast/index.pl). All the sequences acquired were removed the duplicates and analyzed for the recognizable domains using BLAST-based NCBI conserved domain searches (http://www.ncbi.nlm.nih.gov/Structure/lexington/lexington.cgi). The protein sequences were further verified using the HMMER-based SMAT Website (http://smart.embl-heidelberg.de/) and the Pfam program (http://pfam.janelia.org/). The domain architecture was drawn using DOG2.0 software (Ren et al., 2009).
Phylogenetic Construction
The tomato HDAC proteins identified in this work along with the proteins from Arabidopsis were aligned with ClustalX. The phylogenetic analysis was performed using the MEGA3.0 program (Kumar et al., 2004). Neighbor-Joining method and the Poisson correction were used to infer the evolutionary history and compute the evolutionary distances. In addition, all positions containing alignment gaps and missing data were eliminated only in pairwise sequence comparisons.
Quantitative Real-Time Reverse Transcription-PCR (qRT-PCR) Assays
Total RNA was extracted with Trizol reagent (Invitrogen) according to the manufacturer's protocol. The cDNAs were synthesized from 2 μg total RNA using the TransScript™ One-Step gDNA Removal and cDNA Synthesis Supermix kit (TransGen Biotech). Real-Time PCR was performed with iTaq™ Universal SYBR® Green Supermix (BIO-RAD) using ABI 7500 Fast Real-Time PCR system. The gene-specific primers for real-time PCR were designed by primer 3.0 (Untergasser et al., 2012) and listed in Supplementary Table 1. Tomato Actin (Solyc03g078400) served as an internal control.
Subcellular Localization Assays
The full length cDNAs of SlHDACs were subcloned into the pSAT6-EYFP_N1 vector (Tzfira et al., 2005) to create the SlHDAC-YFP constructs. The protoplast isolation and transient expression were conducted as described previously (Yoo et al., 2007). Mesophyll protoplasts were isolated using the well-expanded leaves from 3-week-old Arabidopsis Col-0 plants. The SlHDAC-YFP fusion plasmid and the nuclear maker VirD2-NLS mCherry were co-transfected into protoplasts (3 × 104 protoplasts) using the PEG-calcium solution (0.4 g/mL PEG 4000, 0.2 M mannitol, 0.1 M CaCl2). After washed and resuspended with W5 solution (154 mM Nacl, 125 mM CaCl2, 5 mM KCl, 5 mM glucose, 2 mM MES), mesophyll protoplasts were incubated under white light for 12–18 h. The YFP fluorescence was examined and imaged with a confocal microscope.
Yeast Two-Hybrid, BiFC and Pull-Down Assays
Yeast two-hybrid assays were performed using the Matchmaker™ Gold Yeast Two-Hybrid Systems (Clontech). Constructs were generated by cloning SlHDACs into pGADT7 vectors and four selected MADS-box genes into pGBKT7 vectors. Different pairs of bait and prey constructs were co-transformed into yeast strain Gold by the lithium acetate method, and yeast cells were grown on minimal medium/-Leu-Trp according to the manufacturer's instructions. Transformed colonies were plated onto minimal medium/-Leu/-Trp/-Ade/-His and minimal medium/-Leu/-Trp/-Ade/-His plates containing 4 mg/mL X-α-Gal.
For BiFC assay, the cDNAs of SIHDA1, SIHDA2, SIHDA4, TAG1, and TM29 were cloned into serial pSAT1 vectors (Tzfira et al., 2005) containing either amino- or carboxyl terminal Enhanced Yellow Fluorescence Protein (EYFP) fragments by In-Fusion cloning (Clontech, In-Fusion® HD Cloning Kit, Cat#639650). Plasmids of the corresponding N- and C-terminal fusions of YFP were cotransformed into Arabidopsis protoplasts as described previously (Walter et al., 2004; Yoo et al., 2007). The protoplasts were incubated for 12–16 h, and the fluorescence was determined using a confocal microscope (ZEISS-510Meta). The YFP fluorescence was excited by a 514-nm laser and captured at 523–600 nm, and the chlorophyll autofluorescence was captured at 650–750 nm.
The procedures used for pull-down assays were described previously with some modifications (Yang et al., 2008; Liu et al., 2013b). GST, GST-SIHDA1, GST-SIHDA3, and GST-SIHDA4 recombinant proteins were incubated with 30 ul of GST resin in a binding buffer (50 mM Tris-HCl, pH 7.5, 100 mM NaCl, 0.25% Triton X-100, and 35 mM β-mercaptoethanol) for 2 h at 4°C, the binding reaction was washed three times with the binding buffer and then the TM29-His or TAG1-His recombinant protein was added and incubated for an additional 2 h at 4°C. After extensive washing, the pulled-down proteins were eluted by boiling, separated by 10% SDS-PAGE, and detected by immunoblotting using an anti-His antibody.
Results
Identification of HDACs in Tomato
The HDACs of Arabidopsis amino acid sequences were used as queries to search against the SGN annotation database with the BLAST program. All sequences with an E-value below 10−2 were selected for further analysis. NCBI Conserved Domain Search, Pfam and SMART database were used to confirm each candidate protein sequence. Previously, 14 genes were identified as deduced HDAC genes in tomato (Cigliano et al., 2013b). We identified a new HDAC gene, SlHDA10, belonging to the RPD3/HDA1 family in the tomato genome (Table 1, Figure 1). Fifteen HDAC genes distribute in different tomato chromosomes with various numbers of exons (Table 1). To further investigate the evolutionary relationships, phylogenetic analyses were performed using the Mega 3.0 software. The phylogenetic tree indicates that 15 HDACs in tomato can be divided into three clades: RPD3/HDA1, SIR2, and HD2 families (Table 1, Figure 1A). There are 10 members in the RPD3/HDA1 family, which can be further divided into four subclasses as Class I, Class II, Class III, and Class IV. In addition, there are two and three members in the SIR2 and HD2 family, respectively.
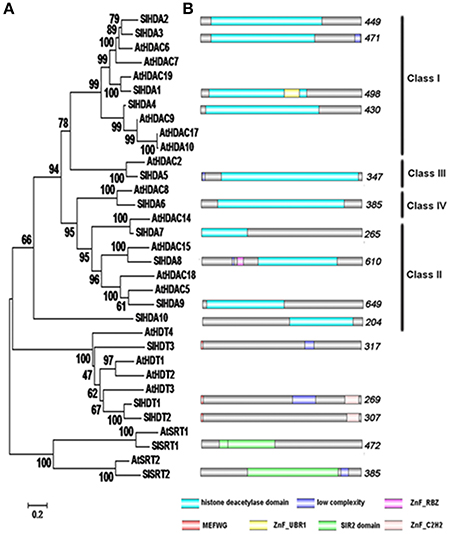
Figure 1. Phylogenetic analysis and domain organization of HDACs in tomato. (A) The neighbor joining phylogenetic tree constructed by MEGA 3 refers to the evolutionary relationship between the HDACs in tomato and Arabidopsis. (B) Domain architecture of the HDACs in tomato was drawn by DOG 2.0. The location and size of domains are shown by different color as indicated. The proteins belonging to each family are grouped together.
The conserved domains were predicted with hammer-based website Smart and Pfam (Figure 1B). The members of RPD3/HDA1 family all have a deacetylase catalytic domain. Both SlHDA1 and SlHDA8 have the zinc-finger domain which might be involved in the binding of DNA. The N-terminus of the HD2 family contains the conserved typical pentapeptide motif (MEFWG) (see Supplementary Figure 1). In addition, SlHDT2 and SlHDT1 both contain a C2H2 zinc-finger that might be involved in protein-protein interaction. Furthermore, the members of SIR2 family have the conserved domain that is dependent on NAD.
The Expression Pattern of SlHDACs
Considering the typical fruit development and maturation processes of tomato, we focused on the several important stages such as fruits on 10, 20, and 30 days post anthesis (dpa) as well as fruits of the maturing green stage (MG), breaker stage, turning stage, pink stage and red ripe stage (Teyssier et al., 2008) (see Supplementary Figure 2). Except for SlHDA2, the transcripts of other 14 SlHDACs were detectable in roots, hypocotyls, cotyledons, euphylla, leaves and fruits from 10 dpa to the red ripe stage (Figure 2). The Class I members of RPD3/HDA1 family, SlHDA3, SlHDA1, and SlHDA4, were all highly expressed in flowers but lowly expressed in later fruit stages. In addition, SlHDA1 was also highly expressed in the red ripe stage (Figure 2). The transcript of the Class III member, SlHDA5, accumulated to a high level in flowers and 10 dpa, but decreased as fruit development and ripening (Figure 2). The SlHDA6, a member of Class IV, was highly expressed in the red ripe stage but lower expressed in cotyledons, flowers and fruits at 10, 20, and 30 dpa stages. Two members of Class II subfamily, SlHDA7 and SlHDA10, shared the similar expression pattern and were highly expressed in cotyledons, euphylla and leaves (Figure 2). Unlike SlHDA7 and SlHDA10, the other members of Class II subfamily, SlHDA8 and SlHDA9, showed different expression patterns. SlHDA8 was highly expressed in 10 dpa stage, while the SlHDA9 transcript was accumulated in cotyledons and euphylla (Figure 2).
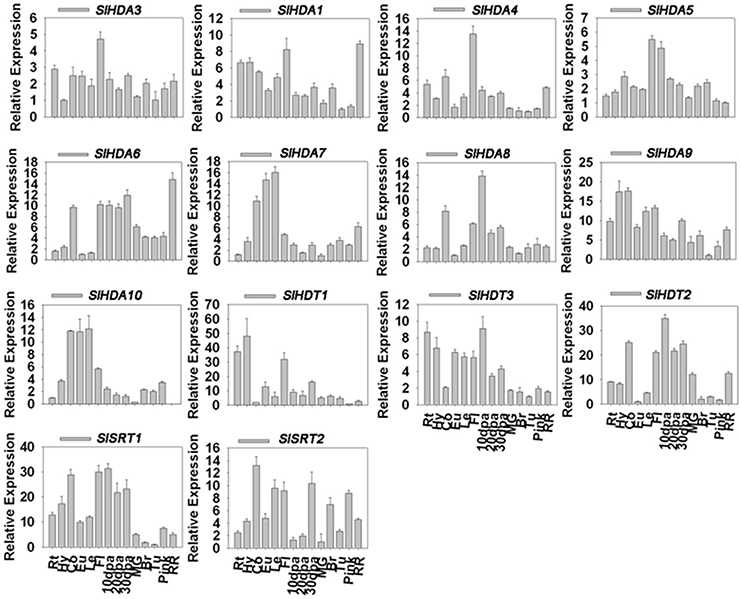
Figure 2. Quantitative RT-PCR analysis of the expression of HDACs in different tissues and developmental stages. Roots (Rt), hypocotyls (Hy), cotyledons (Co), euphylla (Eu), and leaves (Le) of 5-week-old plate cultured plants and flowers (Fl), fruits at 10 days post anthesis (10 dpa), 20 dpa, 30 dpa, mature green stage (MG), break stage (Br), turning stage (Tu), pink stage (pink), and red ripe stage (RR) were collected for total RNA isolation. RT-PCR was amplified using gene-specific primers. The tomato Actin (Solyc03g078400) was used as an internal control.
In comparison, the two members of HD2 family, SlHDT3 and SlHDT1, were highly expressed in roots and hypocotyls. In addition, transcripts of SlHDT2 and SlHDT1 were also accumulated in flowers and 10 dpa stage (Figure 2). Furthermore, the members of SIR2 family, SlSRT1 and SlSRT2, were highly expressed in cotyledons, flowers and 10 dpa stage (Figure 2).
The Subcellular Localization of SlHDACs
WoLF PSORT was used to determine the predicted subcellular localizations of SlHDACs. Interestingly, SlHDACs were predicted to have various subcellular localizations including nuclei, peroxisomes, plasma membranes, cytoplasms, mitochondria and chloroplasts (Table 1).
To further determine the subcellular localization of SlHDACs, the cDNA of each SlHDAC was fused with Yellow Fluorescent Protein (YFP) driven by the Cauliflower mosaic virus 35S promoter and transiently expressed in Arabidopsis protoplasts. As shown in Figure 3, SlHDACs of the three subfamilies showed different subcellular localization. The members of the RPD3/HDA1 subfamily displayed variable subcellular localizations. SlHDA1, SlHDA5, and SlHDA8 were localized in the nucleus, while SlHDA9 was only localized in the cytoplasm (Figure 3A). In contrast, SlHDA3 and SlHDA4 were localized in both the cytosol and nucleus, implying the possibility of shuttling between the nucleus and cytoplasm. Interestingly, SlHDA10 was localized in the chloroplast, which is consistent with the result of the WoLF PSORT programs analysis.
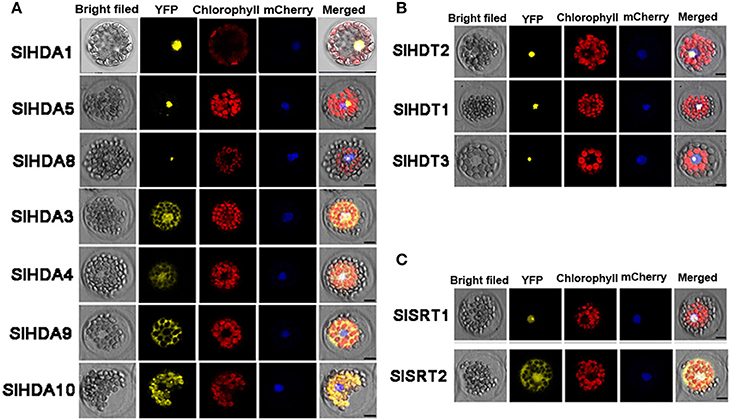
Figure 3. Protoplast transient expression analysis using HDAC-YFP fusion constructs. Subcellular location of RPD3/HDA1 (A), HD2 (B), and SRT2 (C) family HDACs was determined via Arabidopsis protoplast PEG transfection using HDAC-YFP fusion constructs. Red color indicated autofluorescence emitted by chloroplasts. The blue color indicates the nucleus using the mCherry as the nuclear marker. Bar represents 7.5 μm.
Consistent with the predicted localization using WoLF PSORT programs, SlSRT1 and all the three members of HD2 subfamily were localized in the nucleus (Table 1, Figures 3B,C). A recent study showed that the SRT2 in Arabidopsis was localized at the inner mitochondrial membrane (Konig et al., 2014). Nevertheless, SlSRT2, the homolog of Arabidopsis SRT2 in tomato, was localized in both the nucleus and cytosol (Figure 3C).
Members of RPD3/HDA1 Subfamily Interact with MADS-Box Proteins
In Arabidopsis, two MADS-box proteins associated with flowering, AGAMOUS-like 15 (AGL15) and AGAMOUS-like 24 (AGL24), were shown to interact with SAP18, a subunit of the SIN3-HDAC complex involved in transcriptional repression (Hill et al., 2008; Liu et al., 2009). Furthermore, a recent study found that SlMADS1/LeMADS1 interacted with the N-terminal domain of mammalian HDAC5 in vitro (Gaffe et al., 2011). These data suggest that MADS transcription factors may be associated with a HDAC protein complex. The interactions between the members of RPD3/HDA1 family HDACs and the MADS-box proteins involved in fruit development were performed using yeast two-hybrid assays. Our results show that two MADS-box proteins, TM29 and TAG1, interacted with SlHDA1 and SlHDA4 (Figure 4A). In addition, SlHDA3, the homolog of Arabidopsis HDA6 in tomato, also interacted with TAG1 in yeast cells (Figure 4A). Interestingly, we also observed TM29 and TAG1 interacted with SlHDA6 and SlHDA7 in yeast cells (see Supplementary Figure 3).
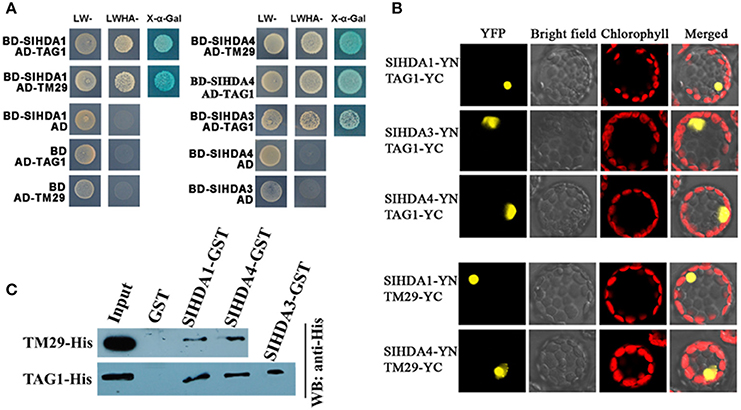
Figure 4. SIHDACs interacted with MDAS-box proteins, TM29 and TAG1. (A) SIHDACs interacted with TM29 and TAG1 in yeast two-hybrid assays. SlHDA1, SlHDA3, and SlHDA4 were cloned into pGADT7 vector, whereas TM29 and TAG1 were cloned into pGBKT7 vector, respectively. The plasmids were cotransformed into the yeast strain AH109. The transformants were grown on the selective minimal medium without Leu and Trp (LW-) or without Leu, Trp, Ade and His (LWHA-). SlHDACs interacted with TM29 and TAG1 in Arabidopsis protoplasts in BiFC assays. (B) SlHDA1/SlHDA2/SlHDA4 fused with the N terminus (YN) and TAG1/TM29 fused with the C terminus (YC) of YFP were cotransformed into protoplasts and then incubated in the 100 μmol.m-2.s-1 for 12 h. The fluorescence was determined using a confocal microscope. The YFP fluorescence was excited by a 514 nm laser and captured at 523–600 nm, and the chlorophyll autofluorescence was captured at 650–750 nm. Bar = 20 μm. SlHDACs interacted with TM29 and TAG1 in pull-down assays. (C) GST- SlHDAC1, GST- SlHDAC3, GST- SlHDAC4 or GST was incubated with either TM29-His or TAG1-His and GST affinity resin, and the bound proteins were then eluted from resin and probed with the anti-His antibody.
The interaction of SlHDACs and these MADS-box proteins was further studied in vivo by the bimolecular fluorescence complementation (BiFC) and in vitro pull-down assays. For BiFC assays, SlHDACs and the MADS-box proteins were fused to either the N-terminal or C-terminal portion of YFP in the pSAT1 vector. The constructs were codelivered into Arabidopsis protoplasts and then incubated under light for 12 h. Strong YFP signal was observed in the nucleus when transiently coexpressing SlHDA1-YN/ SlHDA3-YN/ SlHDA4-YN/ with TAG1-YC (Figure 4B). Similarly, strong YFP signal was also observed in the nucleus when transiently coexpressing SlHDA1-YN/ SlHDA4-YN/ with TM29-YC (Figure 4B). As a control, no YFP signal was observed when these construct were cotransformed with the YN or NC empty vector (see Supplementary Figure 4).
For in vitro pull-down assays, purified TM29-His or TAG1-His recombinant protein was incubated with Glutathione S-Transferase (GST)-SIHDA1, GST-SIHDA3 or GST-SIHDA4 protein, respectively. As shown in Figure 4C, TM29-His was pulled down by GST-SIHDA1 and GST-SIHDA4. Similarly, TAG1-His was also pulled down by GST-SIHDA1, GST-SIHDA4 and GST-SIHDA3. These results indicate that TM29 is directly associated with SIHDA1 and SIHDA4, whereas TAG1 is directly associated with SIHDA1, SIHDA3 and SIHDA4.
Discussion
In Arabidopsis, HDACs were found to be crucial players in all aspects of plant development including embryogenesis, abaxial/adaxial polarity determination, flowering, senescence, responses to day length and environmental stresses (Hollender and Liu, 2008; Ma et al., 2013; Liu et al., 2014). In comparison, relatively few HDACs were characterized in other plant species. In this study, 15 HDACs in tomato were identified using the bioinformatics analysis. The SlHDACs can be separated into three families: RPD3/HDA1, HD2 and SIR2 (Figure 1). Ten SlHDACs belong to the RPD3/HDA1 family and they all contain a typical deacetylase catalytic domain that is essential for the deacetylation activity (Figure 1B). In addition, the two members of the SIR2 family do not share sequence homology with other HDAC family members (Figure 1B). The three members of the HD2 family in tomato all contain a conserved pentapeptide (MEFWG) on the N-terminus of the protein, while the C-terminus has a variant domain (Additional file 1). A C2H2 zinc finger domain was identified on the C-terminus of the SlHDT1 and SlHDT2, which may participate in the protein-protein interaction (Figure 1). Compared with the previous report (Cigliano et al., 2013b), a new HDAC protein, SlHDA10, belonging to the RPD3/HDA1 family, was also found in the tomato (Table 1, Figure 1). The Conserved Domain search result clearly showed that SlHDA10 contains an Arginase HDAC domain (Accession NO. cl17011).
The development progress of tomato can be divided into several stages after the flower anthesis. Mature green (MG) stage, breaker stage (Br), turning stage (Tu) and the final red ripe stage (RR) are all the key development stages of tomato. MG stages can also be divided into different stages according to the gel production in locules, seed maturation and the external color (Teyssier et al., 2008). The fruit ripening is regulated by numerous genes which participate in different pathways influencing texture, color, pigment and aroma. Previous RNA-seq expression data demonstrate that SlHDACs may play roles in tomato fruit ripening, since SlHDA1 and SlHDA3 are highly expressed at B10 (10 days after breaking) and B fruit stages, respectively (Sato et al., 2012; Cigliano et al., 2013b). Consistent with this report, our data show that SlHDA4, SlHDA8, SlHDA6, and SlHDT2 were all highly expressed in inflorescences to fruits development stages, indicating the potential role of SlHDACs in fruits development (Figure 2). SlHDA3, the close homolog of AtHDA6 (Tanaka et al., 2008; Chen et al., 2010; Yu et al., 2011), was constitutively expressed in all stage of tomato development, especially in flowers (Figure 2). The two members of the SIR2 family show markedly different expression profiles, implying that they may be involved in different cellular processes (Figure 2). We also found that the expression profiles of some SlHDACs were not consistent with previous data based on RNA-seq analysis (Cigliano et al., 2013b). The discrepancy may be derived from the fact that plant materials at different developmental stages were used for the gene expression analysis. Interestingly, we found that the transcript of SlHDA2 was not detected in all tissues and fruits of different development stages analyzed. Similarly, previous RNA-seq data also showed that the transcript level of SlHDA2 is very low in tomato (Sato et al., 2012).
We found that the members of tomato RPD3/HDA1 family show a variety of subcellular localizations (Figure 3). SlHDA1, SlHDA5, and SlHDA8 were localized in the nucleus. In contrast, SlHDA3 and SlHDA4 were localized in both the nucleus and cytoplasm, suggesting the possibility of shuttling between the nucleus and cytoplasm. The shuttling of HDACs between the nucleus and the cytoplasm was previously reported in Arabidopsis and mammalian cells (Sengupta and Seto, 2004; Alinsug et al., 2009). In contrast, SlHDA9, the member Class II HDACs, was only localized in cytoplasm, implying that its substrates may be cytoplasmic proteins. Interestingly, SlHDA10 was localized in the chloroplast and the SlHDA10 transcript was highly expressed in leaf tissues. It remains to be determined whether SlHDA10 deacetylates chloroplast proteins. Like their Arabidopsis homologs, the members of tomato HD2 subfamily were all localized in the nucleus (Zhou et al., 2004). Previous studies indicate that the Arabidopsis AtSRT2 is localized mitochondria (Konig et al., 2014). In contrast, we found that SlSRT2 was localized in both the nucleus and cytoplasm. The difference in subcellular localization suggests that different members of the same HDAC family may have distinctive functions in tomato.
To date, a number of MADS-box proteins attributing to fruit ripening of tomato have been identified (Vrebalov et al., 2002, 2009; Victoria et al., 2003; Giovannoni, 2007; Dong et al., 2013). In Arabidopsis, it was reported that the MADS-box proteins, AGL15 and AGL24, can interact with SAP18, a component of the SIN3/HDAC complex involved in transcriptional repression (Hill et al., 2008; Liu et al., 2009), suggesting that these MADS transcription factors may be associated with a HDAC protein complex. A previous study indicates that the tomato MADS protein SlMADS1/LeMADS1 interacts with the N-terminal domain of the mammalian HDAC5 in vitro (Gaffe et al., 2011). Our data show that SlHDA1 and SlHDA4 interacted TAG1 and TM29, the MADS-box proteins associated with tomato reproductive development (Figure 4). TAG1 is a member of the AGAMOUS clade of MADS-box genes in tomato and it is expressed in flowers and ripening fruits. Suppression of the TAG1 gene in tomato leads to a variety of floral defects and production of smaller amounts of pollen (Pan et al., 2010). In addition, TAG1 is necessary for the expression of both ethylene-dependent and independent genes during ripening (Klee and Giovannoni, 2011). On the other hand, TM29, a tomato SEPALLATA homolog, is highly expressed in the primordia of all four whorls of floral organs (Ampomah-Dwamena et al., 2002). Down-regulation of TM29 via cosuppression or antisense techniques causes parthenocarpic fruits and aberrant flowers, as the petals and stamens are partially converted to a sepaloid identity (Ampomah-Dwamena et al., 2002). SIHDAC1, SIHDAC4, TAG1, and TM29 all have peak expression at the flowering stage and all of them are expressed during fruit development (Ampomah-Dwamena et al., 2002; Pan et al., 2010) (See Supplementary Figure 5). The interaction of TAG1 and TM29 with SlHDA1 and SlHDA4 indicates that these MADA-box proteins may recruit HDACs to regulate gene expression in reproductive development in tomato.
Conclusion
Fifteen SlHDACs identified from the genome of Solanum lycopersicum can be divided into RPD3/HDA1, SIR2, and HD2 families. Most SlHDACs were expressed in all tissues examined with different transcript abundance. SlHDA8, SlHDA1, SlHDA5, SlSRT1 and members of the HD2 family were localized to the nucleus, whereas SlHDA3 and SlHDA4 were localized in both the cytoplasm and nucleus. Furthermore, TAG1 and TM29 interacted with SlHDA1 and SlHDA4, indicating that these MADA-box proteins may recruit HDACs to regulate gene expression in reproductive development in tomato.
Author Contributions
Songguang Yang and Keqiang Wu conceived this project and designed all research. Linmao Zhao, Jingxia Lu, Pei-Ying Wu, and Jianxia Zhang performed the research. Songguang Yang and Keqiang Wu and Jingxia Lu and Linmao Zhao analyzed data. Songguang Yang and Keqiang Wu wrote the article.
Conflict of Interest Statement
The authors declare that the research was conducted in the absence of any commercial or financial relationships that could be construed as a potential conflict of interest.
Acknowledgments
We are grateful to Dr. Ying Wang (South China Botanical Garden) for providing the seeds of Solanum lycopersicum cultivar Henz1706. We thank Technology Commons, College of Life Science, National Taiwan University for the convenient use of the Bio-Rad real-time PCR system and the confocal spectral microscope imaging system. This work was supported by grants from the National Basic Research Program of China (973 program No. 2012CB910900), National Natural Science Foundation of China (No. 31201106, No. 31128001 and No. 31371308). This work was also supported by the Ministry of Science and Technology of Taiwan (101-2923-B-002-005-MY3, 101-2311-B-002-012-MY3 and 102-2321-B-002-039-) and National Taiwan University (103R892005).
Supplementary Material
The Supplementary Material for this article can be found online at: http://www.frontiersin.org/journal/10.3389/fpls.2014.00760/abstract
Abbreviations
bp, base pair; BiFC, bimolecular fluorescence complementation; cDNA, complementary DNA; dpa, days post anthesis; GST, glutathione S-transferase; HATs, histone acetyltransferases; HDACs, histone deacetylases; MADS-box, Minichromosome Maintenance 1, Agamous, Deficiens and human serum response factor SRF; MG, mature green; PCR, polymerase chain reaction; RR, red ripe; SEP, SEPELATTA; TAG1, TOMATO AGAMOUS11; TAGL11, TOMATO AGAMOUS-LIKE1; TM4, TOMATO MADS BOX4; TM29, TOMATO MADS BOX29; YFP, yellow fluorescence proteins.
References
Alinsug, M. V., Yu, C. W., and Wu, K. Q. (2009). Phylogenetic analysis, subcellular localization, and expression patterns of RPD3/HDA1 family histone deacetylases in plants. BMC Plant Biol. 9:37. doi: 10.1186/1471-2229-9-37
Pubmed Abstract | Pubmed Full Text | CrossRef Full Text | Google Scholar
Allfrey, V. G., Faulkner, R., and Mirsky, A. E. (1964). Acetylation and methylation of histones and their possible role in regulation of Rna synthesis. Proc. Natl. Acad. Sci. U.S.A. 51, 786–794. doi: 10.1073/pnas.51.5.786
Pubmed Abstract | Pubmed Full Text | CrossRef Full Text | Google Scholar
Ampomah-Dwamena, C., Morris, B. A., Sutherland, P., Veit, B., and Yao, J. L. (2002). Down-regulation of TM29, a tomato SEPALLATA homolog, causes parthenocarpic fruit development and floral reversion. Plant Physiol. 130, 605–617. doi: 10.1104/pp.005223
Pubmed Abstract | Pubmed Full Text | CrossRef Full Text | Google Scholar
Aufsatz, W., Mette, M. F., Van Der Winden, J., Matzke, M., and Matzke, A. J. M. (2002). HDA6, a putative histone deacetylase needed to enhance DNA methylation induced by double-stranded RNA. EMBO J. 21, 6832–6841. doi: 10.1093/emboj/cdf663
Pubmed Abstract | Pubmed Full Text | CrossRef Full Text | Google Scholar
Bourque, S., Dutartre, A., Hammoudi, V., Blanc, S., Dahan, J., Jeandroz, S., et al. (2011). Type-2 histone deacetylases as new regulators of elicitor-induced cell death in plants. New Phytol. 192, 127–139. doi: 10.1111/j.1469-8137.2011.03788.x
Pubmed Abstract | Pubmed Full Text | CrossRef Full Text | Google Scholar
Brownell, J. E., Zhou, J. X., Ranalli, T., Kobayashi, R., Edmondson, D. G., Roth, S. Y., et al. (1996). Tetrahymena histone acetyltransferase A: a homolog to yeast Gcn5p linking histone acetylation to gene activation. Cell 84, 843–851. doi: 10.1016/S0092-8674(00)81063-6
Pubmed Abstract | Pubmed Full Text | CrossRef Full Text | Google Scholar
Busconi, M., Reggi, S., Fogher, C., and Bavaresco, L. (2009). Evidence of a sirtuin gene family in grapevine (Vitis vinifera L.). Plant Physiol. Biochem. 47, 650–652. doi: 10.1016/j.plaphy.2009.03.003
Pubmed Abstract | Pubmed Full Text | CrossRef Full Text | Google Scholar
Campos, E. I., and Reinberg, D. (2009). Histones: annotating chromatin. Annu. Rev. Genet. 43, 559–599. doi: 10.1146/annurev.genet.032608.103928
Pubmed Abstract | Pubmed Full Text | CrossRef Full Text | Google Scholar
Chen, L. T., Luo, M., Wang, Y. Y., and Wu, K. Q. (2010). Involvement of Arabidopsis histone deacetylase HDA6 in ABA and salt stress response. J. Exp. Bot. 61, 3345–3353. doi: 10.1093/jxb/erq154
Pubmed Abstract | Pubmed Full Text | CrossRef Full Text | Google Scholar
Cigliano, R. A., Cremona, G., Paparo, R., Termolino, P., Perrella, G., Gutzat, R., et al. (2013a). Histone deacetylase AtHDA7 is required for female gametophyte and embryo development in Arabidopsis. Plant Physiol. 163, 431–440. doi: 10.1104/pp.113.221713
Pubmed Abstract | Pubmed Full Text | CrossRef Full Text | Google Scholar
Cigliano, R. A., Sanseverino, W., Cremona, G., Ercolano, M. R., Conicella, C., and Consiglio, F. M. (2013b). Genome-wide analysis of histone modifiers in tomato: gaining an insight into their developmental roles. MBC Genomics 14:57. doi: 10.1186/1471-2164-14-57
Pubmed Abstract | Pubmed Full Text | CrossRef Full Text | Google Scholar
Demetriou, K., Kapazoglou, A., Tondelli, A., Francia, E., Stanca, M. A., Bladenopoulos, K., et al. (2009). Epigenetic chromatin modifiers in barley: I. Cloning, mapping and expression analysis of the plant specific HD2 family of histone deacetylases from barley, during seed development and after hormonal treatment. Physiol. Plant. 136, 358–368. doi: 10.1111/j.1399-3054.2009.01236.x
Pubmed Abstract | Pubmed Full Text | CrossRef Full Text | Google Scholar
Dong, T. T., Hu, Z. L., Deng, L., Wang, Y., Zhu, M. K., Zhang, J. L., et al. (2013). A tomato MADS-box transcription factor, SlMADS1, acts as a negative regulator of fruit ripening. Plant Physiol. 163, 1026–1036. doi: 10.1104/pp.113.224436
Pubmed Abstract | Pubmed Full Text | CrossRef Full Text | Google Scholar
Ferrandiz, C., Liljegren, S. J., and Yanofsky, M. F. (2000). Negative regulation of the SHATTERPROOF genes by FRUITFULL during Arabidopsis fruit development. Science 289, 436–438. doi: 10.1126/science.289.5478.436
Pubmed Abstract | Pubmed Full Text | CrossRef Full Text | Google Scholar
Gaffe, J., Lemercier, C., Alcaraz, J. P., and Kuntz, M. (2011). Identification of three tomato flower and fruit MADS-box proteins with a putative histone deacetylase binding domain. Gene 471, 19–26. doi: 10.1016/j.gene.2010.10.002
Pubmed Abstract | Pubmed Full Text | CrossRef Full Text | Google Scholar
Giovannoni, J. J. (2007). Fruit ripening mutants yield insights into ripening control. Curr. Opin. Plant Biol. 10, 283–289. doi: 10.1016/j.pbi.2007.04.008
Pubmed Abstract | Pubmed Full Text | CrossRef Full Text | Google Scholar
Girin, T., Paicu, T., Stephenson, P., Fuentes, S., Korner, E., O'brien, M., et al. (2011). INDEHISCENT and SPATULA interact to specify carpel and valve margin tissue and thus promote seed dispersal in Arabidopsis. Plant Cell 23, 3641–3653. doi: 10.1105/tpc.111.090944
Pubmed Abstract | Pubmed Full Text | CrossRef Full Text | Google Scholar
Groszmann, M., Paicu, T., Alvarez, J. P., Swain, S. M., and Smyth, D. R. (2011). SPATULA and ALCATRAZ, are partially redundant, functionally diverging bHLH genes required for Arabidopsis gynoecium and fruit development. Plant J. 68, 816–829. doi: 10.1111/j.1365-313X.2011.04732.x
Pubmed Abstract | Pubmed Full Text | CrossRef Full Text | Google Scholar
Gu, Q., Ferrandiz, C., Yanofsky, M. F., and Martienssen, R. (1998). The FRUITFULL MADS-box gene mediates cell differentiation during Arabidopsis fruit development. Development 125, 1509–1517.
Haigis, M. C., and Guarente, L. P. (2006). Mammalian sirtuins—emerging roles in physiology, aging, and calorie restriction. Genes Dev. 20, 2913–2921. doi: 10.1101/gad.1467506
Pubmed Abstract | Pubmed Full Text | CrossRef Full Text | Google Scholar
Hill, K., Wang, H., and Perry, S. E. (2008). A transcriptional repression motif in the MADS factor AGL15 is involved in recruitment of histone deacetylase complex components. Plant J. 53, 172–185. doi: 10.1111/j.1365-313X.2007.03336.x
Pubmed Abstract | Pubmed Full Text | CrossRef Full Text | Google Scholar
Hollender, C., and Liu, Z. C. (2008). Histone deacetylase genes in Arabidopsis development. J. Integr. Plant Biol. 50, 875–885. doi: 10.1111/j.1744-7909.2008.00704.x
Pubmed Abstract | Pubmed Full Text | CrossRef Full Text | Google Scholar
Klee, H. J., and Giovannoni, J. J. (2011). Genetics and control of tomato fruit ripening and quality attributes. Ann. Rev. Genet. 45, 41–59. doi: 10.1146/annurev-genet-110410-132507
Pubmed Abstract | Pubmed Full Text | CrossRef Full Text | Google Scholar
Konig, A. C., Hartl, M., Pham, P. A., Laxa, M., Boersema, P. J., Orwat, A., et al. (2014). The Arabidopsis class II sirtuin is a lysine deacetylase and interacts with mitochondrial energy metabolism. Plant Physiol. 164, 1401–1414. doi: 10.1104/pp.113.232496
Pubmed Abstract | Pubmed Full Text | CrossRef Full Text | Google Scholar
Kumar, S., Tamura, K., and Nei, M. (2004). MEGA3: integrated software for molecular evolutionary genetics analysis and sequence alignment. Brief. Bioinform. 5, 150–163. doi: 10.1093/bib/5.2.150
Pubmed Abstract | Pubmed Full Text | CrossRef Full Text | Google Scholar
Lagace, M., Chantha, S. C., Major, G., and Matton, D. P. (2003). Fertilization induces strong accumulation of a histone deacetylase (HD2) and of other chromatin-remodeling proteins in restricted areas of the ovules. Plant Mol. Biol. 53, 759–769. doi: 10.1023/B:PLAN.0000023665.36676.89
Pubmed Abstract | Pubmed Full Text | CrossRef Full Text | Google Scholar
Liu, C., Li, L. C., Chen, W. Q., Chen, X., Xu, Z. H., and Bai, S. N. (2013a). HDA18 Affects cell fate in Arabidopsis root epidermis via histone acetylation at four kinase genes. Plant Cell 25, 257–269. doi: 10.1105/tpc.112.107045
Pubmed Abstract | Pubmed Full Text | CrossRef Full Text | Google Scholar
Liu, C., Xi, W. Y., Shen, L. S., Tan, C. P., and Yu, H. (2009). Regulation of floral patterning by flowering time genes. Dev. Cell 16, 711–722. doi: 10.1016/j.devcel.2009.03.011
Pubmed Abstract | Pubmed Full Text | CrossRef Full Text | Google Scholar
Liu, X. C., Chen, C. Y., Wang, K. C., Luo, M., Tai, R., Yuan, L. Y., et al. (2013b). PHYTOCHROME INTERACTING FACTOR3 associates with the histone deacetylase HDA15 in repression of chlorophyll biosynthesis and photosynthesis in etiolated Arabidopsis seedlings. Plant Cell 25, 1258–1273. doi: 10.1105/tpc.113.109710
Pubmed Abstract | Pubmed Full Text | CrossRef Full Text | Google Scholar
Liu, X. C., Yang, S. G., Zhao, M. L., Luo, M., Yu, C. W., Chen, C. Y., et al. (2014). Transcriptional repression by histone deacetylases in plants. Mol. Plant 7, 764–772. doi: 10.1093/mp/ssu033
Pubmed Abstract | Pubmed Full Text | CrossRef Full Text | Google Scholar
Long, J. A., Ohno, C., Smith, Z. R., and Meyerowitz, E. M. (2006). TOPLESS regulates apical embryonic fate in Arabidopsis. Science 312, 1520–1523. doi: 10.1126/science.1123841
Pubmed Abstract | Pubmed Full Text | CrossRef Full Text | Google Scholar
Luger, K., Mader, A. W., Richmond, R. K., Sargent, D. F., and Richmond, T. J. (1997). Crystal structure of the nucleosome core particle at 2.8 angstrom resolution. Nature 389, 251–260. doi: 10.1038/38444
Pubmed Abstract | Pubmed Full Text | CrossRef Full Text | Google Scholar
Lusser, A., Kolle, D., and Loidl, P. (2001). Histone acetylation: lessons from the plant kingdom. Trends Plant Sci. 6, 59–65. doi: 10.1016/S1360-1385(00)01839-2
Pubmed Abstract | Pubmed Full Text | CrossRef Full Text | Google Scholar
Ma, X. J., Lv, S. B., Zhang, C., and Yang, C. P. (2013). Histone deacetylases and their functions in plants. Plant Cell Rep. 32, 465–478. doi: 10.1007/s00299-013-1393-6
Pubmed Abstract | Pubmed Full Text | CrossRef Full Text | Google Scholar
Nagy, L., Kao, H. Y., Chakravarti, D., Lin, R. J., Hassig, C. A., Ayer, D. E., et al. (1997). Nuclear receptor repression mediated by a complex containing SMRT, mSin3A, and histone deacetylase. Cell 89, 373–380. doi: 10.1016/S0092-8674(00)80218-4
Pubmed Abstract | Pubmed Full Text | CrossRef Full Text | Google Scholar
Pan, I. L., Mcquinn, R., Giovannoni, J. J., and Irish, V. F. (2010). Functional diversification of AGAMOUS lineage genes in regulating tomato flower and fruit development. J. Exp. Bot. 61, 1795–1806. doi: 10.1093/jxb/erq046
Pubmed Abstract | Pubmed Full Text | CrossRef Full Text | Google Scholar
Pandey, R., Muller, A., Napoli, C. A., Selinger, D. A., Pikaard, C. S., Richards, E. J., et al. (2002). Analysis of histone acetyltransferase and histone deacetylase families of Arabidopsis thaliana suggests functional diversification of chromatin modification among multicellular eukaryotes. Nucleic Acids Res. 30, 5036–5055. doi: 10.1093/nar/gkf660
Pubmed Abstract | Pubmed Full Text | CrossRef Full Text | Google Scholar
Probst, A. V., Fagard, M., Proux, F., Mourrain, P., Boutet, S., Earley, K., et al. (2004). Arabidopsis histone deacetylase HDA6 is required for maintenance of transcriptional gene silencing and determines nuclear organization of rDNA repeats. Plant Cell 16, 1021–1034. doi: 10.1105/tpc.018754
Pubmed Abstract | Pubmed Full Text | CrossRef Full Text | Google Scholar
Ren, J., Wen, L. P., Gao, X. J., Jin, C. J., Xue, Y., and Yao, X. B. (2009). DOG 1.0: illustrator of protein domain structures. Cell Res. 19, 271–273. doi: 10.1038/cr.2009.6
Pubmed Abstract | Pubmed Full Text | CrossRef Full Text | Google Scholar
Sato, S., Tabata, S., Hirakawa, H., Asamizu, E., Shirasawa, K., Isobe, S., et al. (2012). The tomato genome sequence provides insights into fleshy fruit evolution. Nature 485, 635–641. doi: 10.1038/nature11119
Sengupta, N., and Seto, E. (2004). Regulation of histone deacetylase activities. J. Cell. Biochem. 93, 57–67. doi: 10.1002/jcb.20179
Pubmed Abstract | Pubmed Full Text | CrossRef Full Text | Google Scholar
Tanaka, M., Kikuchi, A., and Kamada, H. (2008). The Arabidopsis histone deacetylases HDA6 and HDA19 contribute to the repression of embryonic properties after germination. Plant Physiol. 146, 149–161. doi: 10.1104/pp.107.111674
Pubmed Abstract | Pubmed Full Text | CrossRef Full Text | Google Scholar
Teyssier, E., Bernacchia, G., Maury, S., Kit, A. H., Stammitti-Bert, L., Rolin, D., et al. (2008). Tissue dependent variations of DNA methylation and endoreduplication levels during tomato fruit development and ripening. Planta 228, 391–399. doi: 10.1007/s00425-008-0743-z
Pubmed Abstract | Pubmed Full Text | CrossRef Full Text | Google Scholar
Tian, L., and Chen, Z. J. (2001). Blocking histone deacetylation in Arabidopsis induces pleiotropic effects on plant gene regulation and development. Proc. Natl. Acad. Sci. U.S.A. 98, 7647–7647. doi: 10.1073/pnas.98.1.200
Tian, L., Wang, J. L., Fong, M. P., Chen, M., Cao, H. B., Gelvin, S. B., et al. (2003). Genetic control of developmental changes induced by disruption of Arabidopsis histone deacetylase 1 (AtHD1) expression. Genetics 165, 399–409.
Tran, H. T., Nimick, M., Uhrig, R. G., Templeton, G., Morrice, N., Gourlay, R., et al. (2012). Arabidopsis thaliana histone deacetylase 14 (HDA14) is an alpha-tubulin deacetylase that associates with PP2A and enriches in the microtubule fraction with the putative histone acetyltransferase ELP3. Plant J. 71, 263–272. doi: 10.1111/j.1365-313X.2012.04984.x
Pubmed Abstract | Pubmed Full Text | CrossRef Full Text | Google Scholar
Tzfira, T., Tian, G. W., Lacroix, B., Vyas, S., Li, J. X., Leitner-Dagan, Y., et al. (2005). pSAT vectors: a modular series of plasmids for autofluorescent protein tagging and expression of multiple genes in plants. Plant Mol. Biol. 57, 503–516. doi: 10.1007/s11103-005-0340-5
Pubmed Abstract | Pubmed Full Text | CrossRef Full Text | Google Scholar
Ueno, Y., Ishikawa, T., Watanabe, K., Terakura, S., Iwakawa, H., Okada, K., et al. (2007). Histone deacetylases and ASYMMETRIC LEAVES2 are involved in the establishment of polarity in leaves of Arabidopsis. Plant Cell 19, 445–457. doi: 10.1105/tpc.106.042325
Pubmed Abstract | Pubmed Full Text | CrossRef Full Text | Google Scholar
Untergasser, A., Cutcutache, I., Koressaar, T., Ye, J., Faircloth, B. C., Remm, M., et al. (2012). Primer3-new capabilities and interfaces. Nucleic Acids Res. 40:e115. doi: 10.1093/nar/gks596
Pubmed Abstract | Pubmed Full Text | CrossRef Full Text | Google Scholar
Victoria, B. M., Claudia, B., Cecilia, D., Mauricio, H. C., Silvana, B. B., Estela, V. M., et al. (2003). MADS-box genes expressed during tomato seed and fruit development. Plant Mol. Biol. 52, 801–815. doi: 10.1023/A:1025001402838
Pubmed Abstract | Pubmed Full Text | CrossRef Full Text | Google Scholar
Vrebalov, J., Pan, I. L., Arroyo, A. J. M., Mcquinn, R., Chung, M., Poole, M., et al. (2009). Fleshy fruit expansion and ripening are regulated by the tomato SHATTERPROOF gene TAGL1. Plant Cell 21, 3041–3062. doi: 10.1105/tpc.109.066936
Pubmed Abstract | Pubmed Full Text | CrossRef Full Text | Google Scholar
Vrebalov, J., Ruezinsky, D., Padmanabhan, V., White, R., Medrano, D., Drake, R., et al. (2002). A MADS-box gene necessary for fruit ripening at the tomato ripening-inhibitor (Rin) locus. Science 296, 343–346. doi: 10.1126/science.1068181
Pubmed Abstract | Pubmed Full Text | CrossRef Full Text | Google Scholar
Walter, M., Chaban, C., Schutze, K., Batistic, O., Weckermann, K., Nake, C., et al. (2004). Visualization of protein interactions in living plant cells using bimolecular fluorescence complementation. Plant J. 40, 428–438. doi: 10.1111/j.1365-313X.2004.02219.x
Pubmed Abstract | Pubmed Full Text | CrossRef Full Text | Google Scholar
Wu, K. Q., Tian, L. N., Malik, K., Brown, D., and Miki, B. (2000). Functional analysis of HD2 histone deacetylase homologs in Arabidopsis thaliana. Plant J. 22, 19–27. doi: 10.1046/j.1365-313x.2000.00711.x
Pubmed Abstract | Pubmed Full Text | CrossRef Full Text | Google Scholar
Xu, C. R., Liu, C., Wang, Y. L., Li, L. C., Chen, W. Q., Xu, Z. H., et al. (2005). Histone acetylation affects expression of cellular patterning genes in the Arabidopsis root epidermis. Proc. Natl. Acad. Sci. U.S.A. 102, 14469–14474. doi: 10.1073/pnas.0503143102
Pubmed Abstract | Pubmed Full Text | CrossRef Full Text | Google Scholar
Yang, J. Y., Iwasaki, M., Machida, C., Machida, Y., Zhou, X. P., and Chua, N. H. (2008). beta C1, the pathogenicity factor of TYLCCNV, interacts with AS1 to alter leaf development and suppress selective jasmonic acid responses. Genes Dev. 22, 2564–2577. doi: 10.1101/gad.1682208
Pubmed Abstract | Pubmed Full Text | CrossRef Full Text | Google Scholar
Yang, X. J., and Seto, E. (2007). HATs and HDACs: from structure, function and regulation to novel strategies for therapy and prevention. Oncogene 26, 5310–5318. doi: 10.1038/sj.onc.1210599
Pubmed Abstract | Pubmed Full Text | CrossRef Full Text | Google Scholar
Yoo, S. D., Cho, Y. H., and Sheen, J. (2007). Arabidopsis mesophyll protoplasts: a versatile cell system for transient gene expression analysis. Nat. Protoc. 2, 1565–1572. doi: 10.1038/nprot.2007.199
Pubmed Abstract | Pubmed Full Text | CrossRef Full Text | Google Scholar
Yu, C. W., Liu, X. C., Luo, M., Chen, C. Y., Lin, X. D., Tian, G., et al. (2011). HISTONE DEACETYLASE6 interacts with FLOWERING LOCUS D and regulates flowering in Arabidopsis. Plant Physiol. 156, 173–184. doi: 10.1104/pp.111.174417
Pubmed Abstract | Pubmed Full Text | CrossRef Full Text | Google Scholar
Zhou, C. H., Labbe, H., Sridha, S., Wang, L., Tian, L., Latoszek-Green, M., et al. (2004). Expression and function of HD2-type histone deacetylases in Arabidopsis development. Plant J. 38, 715–724. doi: 10.1111/j.1365-313X.2004.02083.x
Pubmed Abstract | Pubmed Full Text | CrossRef Full Text | Google Scholar
Zhou, Y., Tan, B., Luo, M., Li, Y., Liu, C., Chen, C., et al. (2013). HISTONE DEACETYLASE19 interacts with HSL1 and participates in the repression of seed maturation genes in Arabidopsis seedlings. Plant Cell 25, 134–148. doi: 10.1105/tpc.112.096313
Pubmed Abstract | Pubmed Full Text | CrossRef Full Text | Google Scholar
Keywords: histone deacetylases, subcellular localization, gene expression, MADS-box proteins, tomato
Citation: Zhao L, Lu J, Zhang J, Wu P-Y, Yang S and Wu K (2015) Identification and characterization of histone deacetylases in tomato (Solanum lycopersicum). Front. Plant Sci. 5:760. doi: 10.3389/fpls.2014.00760
Received: 23 September 2014; Accepted: 10 December 2014;
Published online: 06 January 2015.
Edited by:
Mario Alberto Arteaga-Vazquez, Universidad Veracruzana, MexicoReviewed by:
Zhong Chen, National Institute of Education/Nanyang Technological University, SingaporeShaomin Bian, Jilin University, China
Copyright © 2015 Zhao, Lu, Zhang, Wu, Yang and Wu. This is an open-access article distributed under the terms of the Creative Commons Attribution License (CC BY). The use, distribution or reproduction in other forums is permitted, provided the original author(s) or licensor are credited and that the original publication in this journal is cited, in accordance with accepted academic practice. No use, distribution or reproduction is permitted which does not comply with these terms.
*Correspondence: Songguang Yang, Key Laboratory of Plant Resources Conservation and Sustainable Utilization, South China Botanical Garden, Chinese Academy of Sciences, Xingke Road 723, Tianhe District, Guangzhou 510650, China e-mail: yangsongguang@scbg.ac.cn;
Keqiang Wu, Institute of Plant Biology, National Taiwan University, Taipei 106, No. 1, Sec. 4, Roosevelt Road, 10617 Taipei, Taiwan e-mail: kewu@ntu.edu.tw
†These authors have contributed equally to this work.