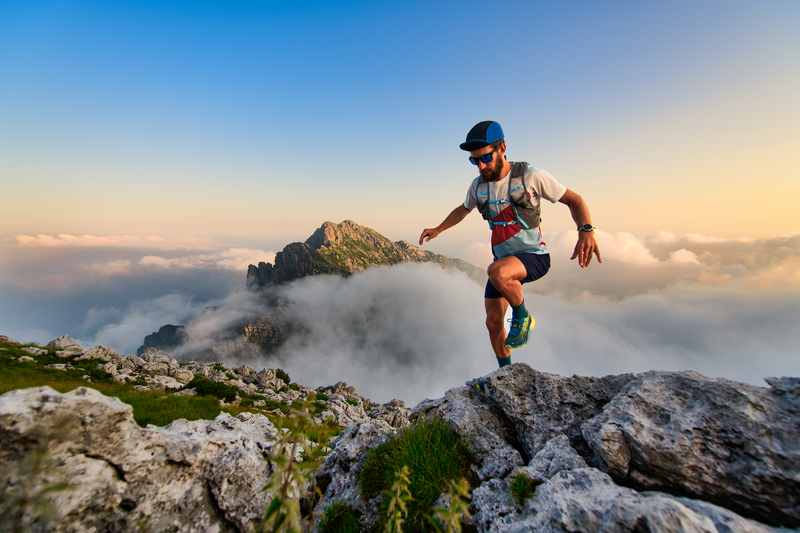
95% of researchers rate our articles as excellent or good
Learn more about the work of our research integrity team to safeguard the quality of each article we publish.
Find out more
ORIGINAL RESEARCH article
Front. Plant Sci. , 29 July 2014
Sec. Plant Physiology
Volume 5 - 2014 | https://doi.org/10.3389/fpls.2014.00371
This article is part of the Research Topic Lipid signalling in plant development and responses to environmental stresses. View all 12 articles
Diacylglycerol pyrophosphate (DGPP) is a minor lipid that attenuates the phosphatidic acid (PA) signal, and also DGPP itself would be a signaling lipid. Diacylglycerol pyrophosphate is an anionic phospholipid with a pyrophosphate group attached to diacylglycerol that was shown to respond to changes of pH, thus affecting the surface organization of DGPP and their interaction with PA. In this work, we have investigated how the presence of Zn2+ modulates the surface organization of DGPP and its interaction with PA at acidic and basic pHs. Both lipids formed expanded monolayers at pHs 5 and 8. At pH 5, monolayers formed by DGPP became stiffer when Zn2+was added to the subphase, while the surface potential decreased. At this pH, Zn2+ induced a phase transition from an expanded to a condensed-phase state in monolayers formed by PA. Conversely, at pH 8 the effects induced by the presence of Zn2+ on the surface behaviors of the pure lipids were smaller. Thus, the interaction of the bivalent cation with both lipids was modulated by pH and by the ionization state of the polar head groups. Mixed monolayers of PA and DGPP showed a non-ideal behavior and were not affected by the presence of Zn2+ at pH 8. This could be explained considering that when mixed, the lipids formed a closely packed monolayer that could not be further modified by the cation. Our results indicate that DGPP and PA exhibit expanded- and condensed-phase states depending on pH, on the proportion of each lipid in the film and on the presence of Zn2+. This may have implications for a possible role of DGPP as a signaling lipid molecule.
Phospholipids are mostly conceived as playing a structural role in lipid bilayers but important aspects on the implications of several phospholipids in lipid-mediated signal transduction have emerged over the past decade (Wang et al., 2006).
Diacylglycerol pyrophosphate (DGPP) is a minor phospholipid found in biological membranes, with a relatively simple chemical structure within the glycerophospholipid family (Wissing and Behrbohm, 1993a). Diacylglycerol pyrophosphate is synthesized from phosphatidic acid (PA) and ATP via the reaction catalyzed by phosphatidate kinase (PAK) and dephosphorylated to PA by the enzyme DGPP phosphatase (Wissing and Behrbohm, 1993b). The average concentration of DGPP in cell membranes is usually very low but evidence suggests that DGPP may act as a novel second messenger with important roles in diverse cellular processes in plants that are related to drought and osmotic stress or salinity (van Schooten et al., 2006). Diacylglycerol pyrophosphate formation is transient and it is always associated with variations of the amount of PA, therefore its synthesis may also be involved in attenuating PA levels (Munnik et al., 1996; van Schooten et al., 2006; Racagni et al., 2008; Paradis et al., 2011). The concentration of PA is maintained at low levels in the cell as a result of its continuous conversion into other lipid species (Arisz et al., 2009; Villasuso et al., 2013).
Phosphatidic acid, the lipid precursor of DGPP, is the glycerophospholipid with the simplest chemical structure in biological membranes; its behavior is crucial for cell survival since it is a phospholipid involved in the synthesis of phospholipids and triacylglycerols thus playing a focal role in cell signaling (Athenstaedt and Daum, 1999). Phosphatidic acid signaling acts by binding effector proteins and recruiting them to a membrane, which regulates the proteins’ activity in cellular pathways (Testerink and Munnik, 2011). Binding is mainly dependent on the concentration of the lipid in the bilayer and it depends on non-specific electrostatic interactions between clusters of positively charged amino acids in the protein and the negatively charged phosphomonoester head group of PA (Shin and Loewen, 2011).
Diacylglycerol pyrophosphate is an anionic phospholipid with a pyrophosphate group attached to diacylglycerol. It was shown that, depending on the pH, the pyrophosphate moiety of DGPP could display 2 or 3 negative charges making it a highly polar molecule (Villasuso et al., 2010; Strawn et al., 2012). Consequently, the ionization of the pyrophosphate group may be important for allowing electrostatic interactions between DGPP and proteins as well as with bivalent cations such as Zn2+ and Ca2+ (Han et al., 2001; Zalejski et al., 2006; Strawn et al., 2012). This can participate in regulating Zn2+-mediated enzyme activities (Han et al., 2001; Kim et al., 2013). Therefore, it is possible that PA and DGPP could be involved in Zn2+ binding thus affecting the lipid signal although the interaction between zinc and DGPP has not been directly demonstrated.
Zinc (Zn) is an essential element in all organisms that plays a fundamental role in numerous cellular functions (Broadley et al., 2007; Cherif et al., 2011). Zn is involved in the catalytic function of many enzymes and structural stability of various cell proteins (Vallee and Falchuk, 1993). Moreover, it has an important role in stabilization and protection of the biological membranes against oxidative stress and the loss of plasma membrane integrity (Aravind and Prasad, 2003). Therefore, Zn deficiency can cause an increase in membrane permeability and a decrease in detoxification mechanisms (Cakmak, 2000). Respect to, it has been shown that the plant roots treated with Cd, an heavy metal that frequently accompanies Zn in the environment, generated oxidative stress, while in combined treatments it was less prominent, indicating that Zn could alleviate oxidative damage (Tkalec et al., 2014). On the other hand, high levels of Zn inhibit many metabolic processes in plants, which can result in limited growth and root development and induce plant senescence (Uruc Parlak and Demirezen Yilmaz, 2012). The extent of Zn phytotoxicity varies in a wide range but mostly depends on plant species, age, environmental conditions, and combinations with other heavy metals (Tsonev and Lidon, 2012). Consequently, the study of the interaction of simple systems with Zn2+ may be relevant and a starting point for future studies with other bivalent ions in relation to abiotic stress and the DGPP and PA signaling.
Although, several aspects of DGPP are unknown, it has been shown that the effective lipid molecular shape and the ionization properties of the phosphomonoester head group of DGPP are similar to PA (Kooijman et al., 2007; Kooijman and Burger, 2009). Thus, the ionization properties of the phosphomonoester of DGPP mimic those of PA following the electrostatic-hydrogen bond switch model (Strawn et al., 2012). However, DGPP is not a cone-shaped lipid, i.e., it is not capable of imparting negative curvature stress to the membrane that could facilitate the insertion of hydrophobic protein domains in the membrane (Strawn et al., 2012).
Little is known on the interaction of PA with DGPP and how these are affected by pH and divalent cations, whether these lipids can molecularly mix, or undergo interactions that may modify their individual properties. Within the context briefly reviewed above, it becomes important to understand details of the effects of Zn2+ and pH on the surface packing and electrostatic behavior of DGPP, and on its interaction with PA. In a previous study with Langmuir monolayers, we demonstrated that the packing and electrostatic properties of films of pure DGPP and PA were affected by the subphase pH as a consequence of changes in the ionization state of the molecules and that the lipids molecularly mix to form closely packed monolayers at basic pHs (Villasuso et al., 2010). In the present work, we provide further evidences on the molecular packing, in-plane elasticity, and surface electrostatic of films of pure DGPP and 1-palmitoyl-2-oleoyl-sn-glycerol 3 phosphate (POPA) and their mixtures on subphases with ZnCl2 at different pHs. In addition, we show how the cation affects the monolayer organization by using fluorescence and Brewster angle microscopy (BAM).
Langmuir monolayers of the individual lipids and their binary mixtures were spread from premixed solutions in chloroform/methanol (2:1, v/v) onto different subphases at a molecular area larger than the lift off area. Before isometric compression of the film, the solvent was allowed to evaporate for 5 min. All experiments were performed at 24 ± 1°C. Temperature was maintained within ±1°C with a refrigerated Haake F3C thermocirculator and air-conditioning the room temperature. DGPP, POPA, and the lipophilic, fluorescent probe l-α-phosphatidylethanolamine-N-(lissaminerhodamine B sulfonyl)-ammonium salt were purchased from Avanti polar lipids Inc. (Alabaster, AL, USA). The lipids were dissolved in chloroform/methanol (2:1, v/v) to a final concentration of 1 nmol μL-1. In all the experiments, the subphase was 150 mM NaCl, 5 mM EDTA, pH 8 or 5, with or without 8 mM ZnCl2. The pH was stable during the time of the experiment. The subphase was prepared with ultra-pure water obtained from a Millipore purification system (18.2 MΩ). Solvents were of the highest available purity from Merck (Darmstadt Germany).
Surface pressure and surface potential versus molecular area isotherms were obtained at 24 ± 1°C in a Teflon trough of a Langmuir film balance (Monofilmmeter, Mayer Feintechnik, Germany).
Surface pressure was measured with a platinized-PtWilhelmy plate. The surface potential measurements were carried out with a high-impedance millivoltmeter connected to a surface ionizing 241Am electrode positioned 5 mm above the monolayer surface and to a reference Ag/AgCl/Cl-1 (3 M) electrode connected to the aqueous subphase.
Absence of surface-active impurities in the subphase and in the spreading solvents was routinely controlled as described elsewhere (Maggio, 2004). At least triplicate monolayer isotherms were obtained and averaged at a compression rate of 0.45–0.60 nm2 molecule-1 min-1; for each mixture, it was ascertained that reducing the compression speed produced no change in the isotherms and that recompression after 5 min equilibration of the expanded isotherm at surface pressures below 2 mN m-1 led to no significant changes of the limiting mean molecular areas which rules out film loss or kinetically limited artifacts.
Reproducibility was within a maximum SEM of ±1 mN m-1 for surface pressure, ±30 mV for surface potential, and ±0.04 nm2 for molecular areas. The monolayers of the pure components and of all mixed films were stable and reproducible by recompression.
The monolayer compressibility modulus (κ), also known as in-plane elasticity, was calculated for the pure monolayers and for each mixture in the different conditions as κ = -Am(∂π/∂Am)T. The effect of ZnCl2 in the subphase as well as the interactions and molecular miscibility were ascertained from the behavior of the mean molecular area, of the compressibility modulus, and of the average dipole potential per unit of molecular surface density (ΔV⋅A, being ΔV the surface (dipole) potential and A the mean molecular area at a defined surface pressure); this magnitude is directly proportional to the overall resultant dipole moment in the direction perpendicular to the interface (Gaines, 1966).
The surfaces of the films were observed by fluorescence microscopy (FM), while simultaneously registering the surface pressure vs. molecular area isotherms. The setup consisted of an automated Langmuir balance (KIBRON microtrough) with a Wilhelmy plate for surface pressure determination, mounted on the stage of a Zeiss Axiovert 200 (Carl Zeiss, Oberkochem, Germany) fluorescence microscope with a CCD video camera Zeiss commanded through the Axiovision software of the Zeiss microscope. Long-distance 20× and 40× objectives were employed. Monolayers with different mole fractions of lipids were spread from chloroform/methanol (2:1, v/v) solutions onto different subphases at a molecular area larger than the lift off area. The fluorescent probe was incorporated in the lipid solution before spreading (1 mol %). Before isometric compression of the film, the solvent was allowed to evaporate for 5 min. All experiments were performed at 24 ± 1°C. This method allows analyzing the presence of micron-sized domains of lipids in different phase state and thus, studying bidimensional phase transitions and phase diagrams of mixtures in different conditions (Rosetti et al., 2010; Vega Mercado et al., 2011; Mangiarotti et al., 2014).
For the BAM experiments, an EP3 Imaging ellipsometer (Accurion, Goettingen, Germany) with a 20× objective was used. These observations were performed in order to ensure that the textures on the micron scale observed in FM experiments were not a consequence of the presence of the fluorescent probe; the latter was reported for some systems (Rosetti et al., 2010), while in others the same texture was observed with both techniques (Mangiarotti et al., 2014). All the experiments were performed at 24 ± 1°C.
In a previous article, the surface behavior of monolayers prepared with DGPP and POPA was described at different pHs. The mixture of both lipids was also analyzed on subphases at basic pHs. As mentioned above, the polar head group of DGPP is a pyrophosphate moiety, and the net charge on the molecule change with the subphase pH (Villasuso et al., 2010). Thus, the polar head group of DGPP may bear from 1 to 3 negative charges, depending on pH (Strawn et al., 2012). Taking the pyrophosphoric acid pKa’s in consideration, we performed compression isotherms on subphases with or without ZnCl2 at pH 5 (pyrophosphate moiety with 1 or 2 negative charges), and at pH 8 (pyrophosphate moiety with 2 or 3 negative charges) with the aim of analyzing the effect of Zn2+on the molecular packing behavior of DGPP and POPA monolayers in different ionization states.
Figure 1 shows the compression isotherms of DGPP (A) and POPA (C) on subphases at pH 5 with and without 8 mM ZnCl2. The compression isotherms in the absence of ZnCl2 have been previously reported (Villasuso et al., 2010). Briefly, both lipids formed expanded monolayers with compressibility moduli increasing continuously up to about 100 mN m-1 at collapse (Figures 1B,D, black lines), which occurred at about 40 mN m-1 for both lipids on acid subphases.
FIGURE 1. Compression isotherms for monolayers of diacylglycerol pyrophosphate (DGPP) and palmitoyl-2-oleoyl-sn-glycerol 3 phosphate (POPA) on subphases at pH 5: (A) Lateral pressure (solid lines, left scale) and ΔV⋅A (dashed line, right scale) as a function of the mean molecular area for DGPP monolayers. (B) Compressibility modulus for the isotherms shown in A. (C) Lateral pressure (solid lines, left scale) and ΔV⋅A (dashed line, right scale) as a function of the mean molecular area for POPA monolayers. Inset: zoom for ΔV⋅A of POPA on solutions with Zn2+ (same as in the main plot). (D) Compressibility modulus for the isotherms shown in C. Insets: representative images for POPA films on solutions with Zn2+ obtained with FM at the indicated lateral pressures. Real size: 100 μm × 100 μm. Subphase composition: 0.15 M NaCl + 5 mM EDTA, pH 5 (black lines) and 0.15 M NaCl, 5 mM EDTA + 8 mM ZnCl2, pH 5 (gray line). All curves show a single representative experiment from a set of triplicates.
The presence of Zn2+ in the subphase caused a shift of the whole isotherm of DGPP to smaller average molecular area (Figure 1A, solid gray line) with a concomitant increase of the compressibility modulus at high molecular densities (Figure 1B, gray line), without an appreciable change of the collapse pressure (collapse point: 42 mN m-1 at 0.58 nm2). The lift off area with zinc in the subphase was 1.05 nm2. The compressibility modulus ranged from 25–30 mN m-1 to 130 mN m-1, indicating that in the presence of Zn2+, DGPP also formed monolayers with a liquid-expanded behavior (Davies and Rideal, 1963) but with a more condensed character under compression compared to the behavior on subphases without Zn2+.
In order to explore the electrostatics of these monolayers, the surface potential was determined in the absence and in the presence of Zn2+. The cation induced a three to fivefold diminution in ΔV⋅A depending on the film packing (Figure 1A, dotted lines).
For POPA, the presence of zinc in the subphase induced a phase transition from a liquid-expanded to a liquid-condensed state at about 11.5 mN m-1 (Figure 1C, solid gray line). The phase transition was clearly observed as a minimum in the compressibility modulus (Figure 1D, gray line). The condensed state showed compressibility moduli up to 150 mN m-1 at collapse (Figure 1D, gray line), corresponding to a liquid-condensed character (Davies and Rideal, 1963). The phase transition induced by Zn2+ probably reflects a decrease of lateral repulsion in the charged polar head groups caused by the divalent cation as previously observed for POPA monolayers in the presence of Mg2+(Brockman et al., 2003).
The Zn2+ induced phase transition corresponded to a first-order, phase transition since micron-sized domains formed by lipids in the condensed state were observed in the two-phase coexistence region using BAM and FM. In Figure 1D, representative images for POPA on solutions with Zn2+ at the indicated surface pressure obtained with FM are shown; similar images were obtained using BAM while in the absence of Zn2+ domains were not observed at any surface pressure (data not shown).
The effect of ZnCl2 on ΔV⋅A at pH 5 was similar to that observed for DGPP, i.e., Zn2+ inducing a 2–4 times decrease depending on the molecular packing density. The phase transition was detected as a change of slope of the ΔV⋅A vs. A isotherm at about 0.65 nm2 (see arrow in Figure 1C inset). In Figure 2, the effect of Zn2+ ions is shown at basic pH. At pH 8, the films of DGPP were loosely packed (Villasuso et al., 2010) and the effect of Zn2+was less marked than at acid pH (Figure 2A). However, a small condensation of the film could still be observed when the cation was added to the subphase and the compressibility modulus increased from 60 to 80 mN m-1 at collapse (Figure 2B). A similar effect was induced by Zn2+ in POPA monolayers (see Figures 2C,D). Regarding the interfacial electrostatics, the presence of Zn2+ at this pH induced an increase of ΔV⋅A, contrary to the effect observed on subphases at acid pH. The increase of ΔV⋅A was of about 20%.
FIGURE 2. Compression isotherms for monolayers of DGPP and POPA on subphases at pH 8: (A) Lateral pressure (solid lines, left scale) and ΔV⋅A (dashed line, right scale) as a function of the mean molecular area for DGPP monolayers. (B) Compressibility modulus for the isotherms shown in A. (C) Lateral pressure (solid lines, left scale) and ΔV⋅A (dashed line, right scale) as a function of the mean molecular area for POPA monolayers. (D) Compressibility modulus for the isotherms shown in C. Subphase composition: 0.15 M NaCl + 5 mM EDTA, pH 8 (black lines) and 0.15 M NaCl, 5 mM EDTA + 8 mM ZnCl2, pH 8 (gray line). All curves show a single representative experiment from a set of triplicates.
It was shown that the effective molecular shape of PA is highly influenced by pH, temperature, and the presence of divalent cations (Minones et al., 2002; Kooijman et al., 2003, 2005; Villasuso et al., 2010). At pH 7 in the presence of magnesium ion, PA acquires a cylindrical shape and thus stabilizes the bilayer. However, once subjected to an acid environment, the head group of PA decreases its effective size, and the lipid undergoes a shape change to a cone-like shape, thus affecting the membrane by promoting an increase of negative membrane curvature (Kooijman et al., 2003). Diacylglycerol pyrophosphate formation after stimulus takes place after a transient increase of the PA levels (Munnik et al., 1995). As a consequence, a temporary and local accumulation of DGPP and its precursor at the membrane interface should be expected. Since the monolayer packing properties are affected by the interactions of POPA and DGPP at pH 8 (Villasuso et al., 2010), the phospholipid packing may be affected during the signaling processes. Therefore, we studied the packing properties of mixed films of DGPP and POPA in the presence of Zn2+ on subphases at acidic and basic pHs.
Figure 3 shows the behavior of mixed monolayers of DGPP and POPA when ZnCl2 is added to the subphase at pHs 5 and 8. Figure 3A shows the compression isotherm for a 1:1 mixture at pH 5 with Zn2+ compared with the isotherm in the absence of Zn2+. Similar to the pure lipids, the cation decreased the potential from 8–6 to 3–2 fV cm2 and a phase transition from a liquid-expanded to a liquid-condensed film was induced. However, the transition occurred at 30 mN m-1 and was less marked. As shown in Figure 2B, the phase transition of POPA induced by Zn2+ was observed at increasing pressures and in a less marked fashion as the proportion of DGPP in the mixtures increased. The fact that the lateral pressure for the phase transition was higher as the proportion of DGPP increased in the film indicates that DGPP mixed preferentially with POPA in the liquid-expanded state, thus stabilizing the latter with respect to the condensed phase. Figure 3C shows representative FM images of mixed monolayers at the surface pressures in which the phase coexistence is observed in each case, similar images were obtained using BAM (data not shown). At 75% of DGPP, no domains were observed up to 36 mN m-1. Nevertheless, as the monolayer approached the collapse point, the fluorescent probe was segregated in bright spots indicating that the monolayer acquired a more condensed character, expelling the bulky probe (see image in Figure 3C).
FIGURE 3. Compression isotherms for mixed monolayers of DGPP and POPA on subphases at different pHs: (A) Lateral pressure (solid lines, left scale) and ΔV⋅A (dashed line, right scale) as a function of the mean molecular area for monolayers composed of DGPP/POPA (1:1) on subphases at pH 5. (B) Lateral pressure as a function of the mean molecular area for monolayers composed pure DGPP and POPA (dashed lines) or mixtures of DGPP/POPA in a proportion (1:3) (black line) and (3:1) (gray line). (C) Representative images of mixed films at the indicated proportions and pressures obtained with FM on subphases at pH 5 with Zn2+. Real size: 100 μm × 100 μm. (D) Lateral pressure (solid lines, left scale) and ΔV⋅A (dashed line, right scale) as a function of the mean molecular area for monolayers composed of DGPP/POPA (1:1) on subphases at pH 8. Subphase composition: 0.15 M NaCl + 5 mM EDTA, pH 8 (black lines) and 0.15 M NaCl, 5 mM EDTA + 8 mM ZnCl2, pH 8 (gray line).
At pH 8, the surface pressure- and ΔV⋅A-mean molecular area compression isotherms for mixed monolayers of DGPP with POPA were only slightly affected by the presence of the divalent cation (see Figure 3D).
The behavior upon compression of films composed of DGPP, POPA, and their mixtures in the presence of ZnCl2 was investigated on acid and basic subphases. Figure 4 summarizes the effect of the presence of ZnCl2 at 10 and 40 mN m-1 at pH 5 (lefts panels) compared to pH 8 (right panels). The mean molecular area, the compressibility of the film, and ΔV.A are shown as a function of the mole fraction of DGPP in mixed films with POPA. The same scale was used for both pHs in panels A–D in order to facilitate comparison. For panels E and F, different scales were used for better understanding of the data.
FIGURE 4. Mean molecular area (A,B), compressibility modulus (C,D), and surface potential density (E,F) as a function of the mole fraction of DGPP in the mixture at pH 5 (left panel) and pH 8 (right panel). The lateral pressures are: 10 mN m-1 (circles) and 40 mN m-1 (squares). Subphase composition: 0.15 M NaCl + 5 mM EDTA, (black circle) and 0.15 M NaCl, 5 mM EDTA + 8 mM ZnCl2, (gray circle).
At pH 5, the molecular density of pure DGPP and of films enriched in this lipid was markedly increased by the divalent cation while the effect was reduced in films with a high proportion of POPA (Figure 4A). This effect was more marked at low than at high surface pressure, where the mean molecular area decreased 0.1–0.2 nm2. This is probably a consequence that the mean molecular area at 30 mN m-1 or higher pressures was close to the minimal area occupied by two hydrocarbon chains (0.38–0.40 nm2), and thus the cation was not able to further reduce the area occupied by the lipid. However, slope of the isotherms in the whole range of surface pressures was higher in the presence of Zn2+ with a concomitant increase in the surface compressibility modulus. At pH 5, monolayers in the presence of Zn2+ were less compressible than in its absence (see Figure 4C). Exceptions were observed for the proportions in which the induced phase transition coincides with the lateral pressure analyzed (25% at 10 mN m-1 and 75% at 40 mN m-1). The compression isotherms of monolayers for a particular lipid species depends on the length and unsaturation of the hydrocarbon chain and on the bulkiness and charge of the polar head group. Long and saturated hydrocarbon chains generally promote more condensed monolayer. In contrast, ionization of the lipid head groups should result in repulsive interaction, leading to loosely packed monolayers (Brown and Brockman, 2007). Thus, the observed compression isotherms of DGPP and POPA at pH 5 in the presence of the cation are in agreement with a decreased electrostatic repulsion where a lower net charge is expected. In similar experiments, it was observed that DPPA monolayers are also influenced by pH as a consequence of the change in the ionization of the lipid (Minones et al., 2002). At pH 3, DPPA molecules are uncharged showing a more rigid monolayer structure compared to monolayer spread on subphases at higher pHs. It is possible that hydrogen bonding between protonated PA groups of adjacent molecules could lead to a more stable and coherent monolayer. In contrast, DPPA monolayer at pH 6 exhibits a larger molecular area due to the ionization of the first phosphate of PA, decreasing the possibility for intermolecular hydrogen bonding. The effect of divalent cations on the interfacial behavior of anionic lipid was also evaluated with other phospholipids in model system. Apparently some divalent cations at high concentration may induce the formation of clusters of phospholipids. In the case of the precursor of second messenger PIP2, only Ca2+, but not Mg2+, Zn2+, or polyamines induces a surface pressure drop that coincides with the formation of PIP2 clusters (Wang et al., 2012). Our results with Zn2+ at high concentration (ca. 3 mM free ion) indicated that the ion promotes the generation of domains of POPA in a condensed phase state. Which is the lower amount of this ion capable of induce a phase transition in monolayers of this lipid is however, not investigated, since it is beyond the scope of this research.
At pH 8, only the monolayers composed of pure DGPP or POPA were condensed by Zn2+, while the film density of the mixed monolayers was unaffected by this cation (Figure 4B). This is probably a consequence of the fact that the mixture at basic pHs forms closely packed films and the process of mixing is thermodynamically favored (Villasuso et al., 2010), therefore, the Zn2+ ions are probably not able to penetrate and further modify the compact film formed by the mixture. The compressibility modulus as a function of the proportion of DGPP in the mixture at pH 8 is shown in Figure 4D, and the comparison with Figure 4C indicates that at this pH only a slight increase of the compressibility was observed at 10 mN m-1 while the effect was negligible at 40 mN m-1.
Regarding the surface electrostatics, Figures 4E,F show that the ΔV⋅A of the pure lipids and their mixtures changed from 4–6 to 2 fV cm2 when the pH increased. The negative charge on the lipids signifies an anionic surface with a corresponding distribution of mobile ions in the immediate aqueous milieu (the double-layer potential) which is also included in the surface potential measured. It was previously observed in stearic acid monolayers that an increase of the negative charge in the monolayer may decrease the double-layer potential by values as high as 200 mV (Vega Mercado et al., 2011). The surface potential of charged films depends in a rather complex manner on the double-layer potential because of the simultaneous contribution of hydrocarbon chain dipoles, of water dipoles from the polar head group hydration shell, and intrinsic polar head group dipoles, as well as from the electrostatic field brought about from the relative position of mobile ions close to the monolayer. Therefore, a decrease of the double-layer potential when the lipid becomes ionized may result in a lower surface potential even if the overall lipid dipole is being increased in the negatively charged molecule (Vega Mercado et al., 2011). Thus, the decrease of ΔV⋅A observed at pH 8 compared to pH 5 could most probably be a consequence of the decrease of the double-layer potential as the monolayer charge increased. Studies of the interaction between PA and Ba2+ suggested that PA is extremely effective in binding divalent ions through its oxygen atoms, with a broad distribution of binding constants and exhibiting the phenomenon of charge inversion (a total number of bound counterion charges that exceeds the negative PA charge; Faraudo and Travesset, 2007). The authors predict that a PA-rich domain undergoes a drastic reorganization when divalent cations as Ca2+ and Ba2+ reach micromolar concentrations (i.e., typical physiological conditions), as PA lipids become doubly charged by releasing their protons. Although restricted to PA, those results could be qualitatively similar for other phospholipids, as DGPP, which play somewhat similar roles as PA.
When Zn2+ was present in the subphase, the measured ΔV⋅A was about 2 fV cm2 at both pHs. This leads to the conclusion that this parameter is very sensitive to the Zn2+ cation at pH 5 and at all pressures, while at pH 8 only the pure POPA monolayers were affected by the cation and, in a less marked manner than at pH 5. Besides, at pH 8 the interface was slightly depolarized while at pH 5 it became highly hyperpolarized.
The relatively low response of the surface electrostatics at pH 8 may be explained by considering that in this condition, the charge density of the interface was quite negative, and a compact, double layer was already formed in the absence of Zn2+. The addition of this cation may not induce further changes in the system, in spite of any specific binding with the film molecules. Also, it is possible that at basic pHs, Zn2+ did not interact specifically with the film and thus, only changed slightly the ionic strength of the subphase with correspondingly small changes in the film properties (Figures 4B,D,F).
At pH 5, on the other hand, the divalent cation appeared to interact with the film and to induce changes in the film properties studied (Figures 4A,C,E). The important changes induced by Zn2+ clearly indicate an interaction with the phosphate groups, decreasing the in-plane repulsion and hyperpolarizing the interface. How this interaction may translate to changes in ΔV⋅A is not easy to explain because this parameter depends on the location of Zn2+ ions relative to the polar head groups and to the interface.
Our results indicate that the film properties of DGPP and its precursor PA were sensitive to Zn2+ in a pH-dependent manner, and thus depend on the ionization state of the molecules forming the film. This effect was further modulated by the relative proportions of DGPP and POPA in the film. The changes promoted by Zn2+ were more marked on subphases at pH 5, while at pH 8 the influence of the cation in the film surface properties was attenuated.
Regarding the ionization behavior of PA, it was recently proposed that upon initial ionization, the remaining hydrogen may form hydrogen bonds with this phosphomonoester head group, resulting in an easier deprotonation compared to the situation lacking the hydrogen bond. This peculiar ionization behavior was summarized in the electrostatic-hydrogen bond switch model. Similarly, the phosphomonoester head group of DGPP was proposed to follow this behavior. In addition, it was observed that at constant pH, DGPP carries more negative charge than the phosphomonoester head group (Strawn et al., 2012). Strawn et al. (2012) suggested that the higher charges would favor the interaction of positive domains of proteins and might result in a displacement of a PA-bound protein to DGPP. If in the complex mixture, POPA and DGPP also form a stable film when locally mixed, then in the presence of DGPP, POPA would not be as free as in its absence. Furthermore, the interaction of both molecules with Zn2+ ions would be precluded in the mixed film.
If the surface behavior described could happen in biomembranes, it may be speculated that such effects could occur locally when DGPP is generated by PAK or hydrolyzed by DGPP phosphatase. In this work, we demonstrate that a local increase in the proportion of this lipid can affect the local film properties, in a pH-dependent manner which is also sensitive to the ionic composition of the aqueous milieu. Taking together, all these effects may constitute structural-electrostatic signal transduction mechanism involving DGPP in the turning off/on of PA signaling.
Ana L. Villasuso and Natalia Wilke conceived and designed the experiments. Ana L. Villasuso performed the experiments. Ana L. Villasuso and Natalia Wilke analyzed the data. Estela Machado and Bruno Maggio contributed reagents/materials/analysis tools. Ana L. Villasuso, Natalia Wilke, Estela Machado, and Bruno Maggio involved in the Result discussion. Ana L. Villasuso, Natalia Wilke, Estela Machado, and Bruno Maggio wrote the paper. Ana L. Villasuso, Natalia Wilke, Estela Machado, and Bruno Maggio edited the manuscript, wrote figure legends, and helped with the outline of the paper:.
The authors declare that the research was conducted in the absence of any commercial or financial relationships that could be construed as a potential conflict of interest.
This work was supported in part by SECyT-UNRC, SECyT-UNC, FONCYT (PICT, 2006-1513; 2007-02212; 2010-0415), CONICET and Agencia Cordoba-Ciencia. Bruno Maggio, Natalia Wilke, and Ana L. Villasuso are Career Investigators of CONICET.
Aravind, P., and Prasad, M. N. V. (2003). Zinc alleviates cadmium induced toxicity in Ceratophyllum demersum, a fresh water macrophyte. Plant Physiol. Biochem. 41, 391–397. doi: 10.1016/S0981-9428(03)00035-4
Arisz, S. A., Testerink, C., and Munnik, T. (2009). Plant PA signaling via diacylglycerol kinase. Biochim. Biophys. Acta 1791, 869–875. doi: 10.1016/j.bbalip.2009.04.006
Athenstaedt, K., and Daum, G. (1999). Phosphatidic acid, a key intermediate in lipid metabolism. Eur. J. Biochem. 266, 1–16. doi: 10.1046/j.1432-1327.1999.00822.x
Broadley, M. R., White, P. J., Hammond, J. P., Zelko, I., and Lux, A. (2007). Zinc in plants. New Phytol. 173, 677–702. doi: 10.1111/j.1469-8137.2007.01996.x
Brockman, H. L., Applegate, K. R., Momsen, M. M., King, W. C., and Glomset, J. A. (2003). Packing and electrostatic behavior of sn-2-docosahexaenoyl and -arachidonoyl phosphoglycerides. Biophys. J. 85, 2384–2396. doi: 10.1016/S0006-3495(03)74662-1
Brown, R. E., and Brockman, H. L. (2007). Using monomolecular films to characterize lipid lateral interactions. Methods Mol. Biol. 398, 41–58. doi: 10.1007/978-1-59745-513-8_5
Cakmak, I. (2000). Role of zinc in protecting plant cells from reactive oxygen species. New Phytol. 146, 185–205. doi: 10.1046/j.1469-8137.2000.00630.x
Cherif, J., Mediouni, C., Ben Ammar, W., and Jemal, F. (2011). Interactions of zinc and cadmium toxicity in their effects on growth and in antioxidative systems in tomato plants (Solanum lycopersicum). J. Environ. Sci. (China) 23, 837–844. doi: 10.1016/S1001-0742(10)60415-9
Faraudo, J., and Travesset, A. (2007). Phosphatidic acid domains in membranes: effect of divalent counterions. Biophys. J. 92, 2806–2818. doi: 10.1529/biophysj.106.092015
Gaines, G. L. (1966). “Insoluble monolayers at liquid-gas interfaces,” in Interscience Monographs on Physical Chemistry, ed. I. Prigogine (New York: John Wiley and Sons), 136–207.
Han, G. S., Johnston, C. N., Chen, X., Athenstaedt, K., Daum, G., and Carman, G. M. (2001). Regulation of the Saccharomyces cerevisiae DPP1-encoded diacylglycerol pyrophosphate phosphatase by zinc. J. Biol. Chem. 276, 10126–10133. doi: 10.1074/jbc.M011421200
Kim, S.-C., Guo, L., and Wang, X. (2013). Phosphatidic acid binds to cytosolic glyceraldehyde-3-phosphate dehydrogenase and promotes its cleavage in Arabidopsis. J. Biol. Chem. 288, 11834–11844. doi: 10.1074/jbc.M112.427229
Kooijman, E. E., and Burger, K. N. (2009). Biophysics and function of phosphatidic acid: a molecular perspective. Biochim. Biophys. Acta 1791, 881–888. doi: 10.1016/j.bbalip.2009.04.001
Kooijman, E. E., Carter, K. M., Van Laar, E. G., Chupin, V., Burger, K. N., and De Kruijff, B. (2005). What makes the bioactive lipids phosphatidic acid and lysophosphatidic acid so special? Biochemistry 44, 17007–17015. doi: 10.1021/bi0518794
Kooijman, E. E., Chupin, V., De Kruijff, B., and Burger, K. N. (2003). Modulation of membrane curvature by phosphatidic acid and lysophosphatidic acid. Traffic 4, 162–174. doi: 10.1034/j.1600-0854.2003.00086.x
Kooijman, E. E., Tieleman, D. P., Testerink, C., Munnik, T., Rijkers, D. T., Burger, K. N.,et al. (2007). An electrostatic/hydrogen bond switch as the basis for the specific interaction of phosphatidic acid with proteins. J. Biol. Chem. 282, 11356–11364. doi: 10.1074/jbc.M609737200
Maggio, B. (2004). Favorable and unfavorable lateral interactions of ceramide, neutral glycosphingolipids and gangliosides in mixed monolayers. Chem. Phys. Lipids 132, 209–224. doi: 10.1016/j.chemphyslip.2004.07.002
Mangiarotti, A., Caruso, B., and Wilke, N. (2014). Phase coexistence in films composed of DLPC and DPPC: a comparison between different model membrane systems. Biochim. Biophys. Acta 1838, 1823–1831. doi: 10.1016/j.bbamem.2014.02.012
Minones, J. Jr., Rodriguez Patino, J. M., Minones, J., Dynarowicz-Latka, P., and Carrera, C. (2002). Structural and topographical characteristics of dipalmitoyl phosphatidic acid in Langmuir monolayers. J. Colloid Interf. Sci. 249, 388–397. doi: 10.1006/jcis.2002.8285
Munnik, T., Arisz, S. A., De Vrije, T., and Musgrave, A. (1995). G protein activation stimulates phospholipase D signaling in plants. Plant Cell 7, 2197–2210. doi: 10.1105/tpc.7.12.2197
Munnik, T., De Vrije, T., Irvine, R. F., and Musgrave, A. (1996). Identification of diacylglycerol pyrophosphate as a novel metabolic product of phosphatidic acid during G-protein activation in plants. J. Biol. Chem. 271, 15708–15715. doi: 10.1074/jbc.271.26.15708
Paradis, S., Villasuso, A. L., Aguayo, S. S., Maldiney, R., Habricot, Y., Zalejski, C.,et al. (2011). Arabidopsis thaliana lipid phosphate phosphatase 2 is involved in abscisic acid signalling in leaves. Plant Physiol. Biochem. 49, 357–362. doi: 10.1016/j.plaphy.2011.01.010
Racagni, G., Villasuso, A. L., Pasquare, S. J., Giusto, N. M., and Machado, E. (2008). Diacylglycerol pyrophosphate inhibits the alpha-amylase secretion stimulated by gibberellic acid in barley aleurone. Physiol. Plant. 134, 381–393. doi: 10.1111/j.1399-3054.2008.01148.x
Rosetti, C. M., Maggio, B., and Wilke, N. (2010). Micron-scale phase segregation in lipid monolayers induced by myelin basic protein in the presence of a cholesterol analog. Biochim. Biophys. Acta 1798, 498–505. doi: 10.1016/j.bbamem.2009.11.006
Shin, J. J., and Loewen, C. J. (2011). Putting the pH into phosphatidic acid signaling. BMC Biol. 9:85. doi: 10.1186/1741-7007-9-85.
Strawn, L., Babb, A., Testerink, C., and Kooijman, E. E. (2012). The physical chemistry of the enigmatic phospholipid diacylglycerol pyrophosphate. Front. Plant Sci. 3:40. doi: 10.3389/fpls.2012.00040
Testerink, C., and Munnik, T. (2011). Molecular, cellular, and physiological responses to phosphatidic acid formation in plants. J. Exp. Bot. 62, 2349–2361. doi: 10.1093/jxb/err079
Tkalec, M., Štefanić, P. P., Cvjetko, P., Šikić, S., Pavlica, M., and Balen, B. (2014). The effects of cadmium–zinc interactions on biochemical responses in tobacco seedlings and adult plants. PLoS ONE 9:e87582. doi: 10.1371/journal.pone.0087582
Tsonev, T., and Lidon, F. J. C. (2012). Zinc in plants – an overview. Emir. J. Food Agric. 24, 322–333.
Uruc Parlak, K., and Demirezen Yilmaz, D. (2012). Response of antioxidant defences to Zn stress in three duckweed species. Ecotoxicol. Environ. Saf. 85, 52–58. doi: 10.1016/j.ecoenv.2012.08.023
Vallee, B. L., and Falchuk, K. H. (1993). The biochemical basis of zinc physiology. Physiol. Rev. 73, 79–118.
van Schooten, B., Testerink, C., and Munnik, T. (2006). Signalling diacylglycerol pyrophosphate, a new phosphatidic acid metabolite. Biochim. Biophys. Acta 1761, 151–159. doi: 10.1016/j.bbalip.2005.12.010
Vega Mercado, F., Maggio, B., and Wilke, N. (2011). Phase diagram of mixed monolayers of stearic acid and dimyristoylphosphatidylcholine. Effect of the acid ionization. Chem. Phys. Lipids 164, 386–392. doi: 10.1016/j.chemphyslip.2011.05.004
Villasuso, A. L., Di Palma, M. A., Aveldano, M., Pasquare, S. J., Racagni, G., Giusto, N. M.,et al. (2013). Differences in phosphatidic acid signalling and metabolism between ABA and GA treatments of barley aleurone cells. Plant Physiol. Biochem. 65, 1–8. doi: 10.1016/j.plaphy.2013.01.005
Villasuso, A. L., Wilke, N., Maggio, B., and Machado, E. (2010). The surface organization of diacylglycerol pyrophosphate and its interaction with phosphatidic acid at the air-water interface. Chem. Phys. Lipids 163, 771–777. doi: 10.1016/j.chemphyslip.2010.09.002
Wang, X., Devaiah, S. P., Zhang, W., and Welti, R. (2006). Signaling functions of phosphatidic acid. Prog. Lipid Res. 45, 250–278. doi: 10.1016/j.plipres.2006.01.005
Wang, Y. H., Collins, A., Guo, L., Smith-Dupont, K. B., Gai, F., Svitkina, T.,et al. (2012). Divalent cation-induced cluster formation by polyphosphoinositides in model membranes. J. Am. Chem. Soc. 134, 3387–3395. doi: 10.1021/ja208640t
Wissing, J. B., and Behrbohm, H. (1993a). Diacylglycerol pyrophosphate, a novel phospholipid compound. FEBS Lett. 315, 95–99. doi: 10.1016/0014-5793(93)81141-L
Wissing, J. B., and Behrbohm, H. (1993b). Phosphatidate kinase, a novel enzyme in phospholipid metabolism (Purification, Subcellular Localization, and Occurrence in the Plant Kingdom). Plant Physiol. 102, 1243–1249. doi: 10.1104/pp.102.4.1243
Zalejski, C., Paradis, S., Maldiney, R., Habricot, Y., Miginiac, E., Rona, J. P.,et al. (2006). Induction of abscisic acid-regulated gene expression by diacylglycerol pyrophosphate involves Ca2+ and anion currents in Arabidopsis suspension cells. Plant Physiol. 141, 1555–1562. doi: 10.1104/pp.106.080218
Keywords: diacylglycerol pyrophosphate, phosphatidic acid, plant lipid signaling, membrane packing, glycerophospholipid monolayers, Zn2+
Citation: Villasuso AL, Wilke N, Maggio B and Machado E (2014) Zn2+ - dependent surface behavior of diacylglycerol pyrophosphate and its mixtures with phosphatidic acid at different pHs. Front. Plant Sci. 5:371. doi: 10.3389/fpls.2014.00371
Received: 09 June 2014; Accepted: 11 July 2014;
Published online: 29 July 2014.
Edited by:
Eric Ruelland, Centre National de la Recherche Scientifique, FranceReviewed by:
Alain Zachowski, Université Pierre et Marie Curie, FranceCopyright © 2014 Villasuso, Wilke, Maggio and Machado. This is an open-access article distributed under the terms of the Creative Commons Attribution License (CC BY). The use, distribution or reproduction in other forums is permitted, provided the original author(s) or licensor are credited and that the original publication in this journal is cited, in accordance with accepted academic practice. No use, distribution or reproduction is permitted which does not comply with these terms.
*Correspondence: Ana L. Villasuso, Departamento de Biología Molecular, FCEFQN, Universidad Nacional de Río Cuarto, Ruta Nacional 36, Km 601, X5804BYA Río Cuarto, Córdoba, Argentina e-mail:bHZpbGxhc3Vzb0BleGEudW5yYy5lZHUuYXI=
†Ana L. Villasuso and Natalia Wilke have contributed equally to this work.
Disclaimer: All claims expressed in this article are solely those of the authors and do not necessarily represent those of their affiliated organizations, or those of the publisher, the editors and the reviewers. Any product that may be evaluated in this article or claim that may be made by its manufacturer is not guaranteed or endorsed by the publisher.
Research integrity at Frontiers
Learn more about the work of our research integrity team to safeguard the quality of each article we publish.