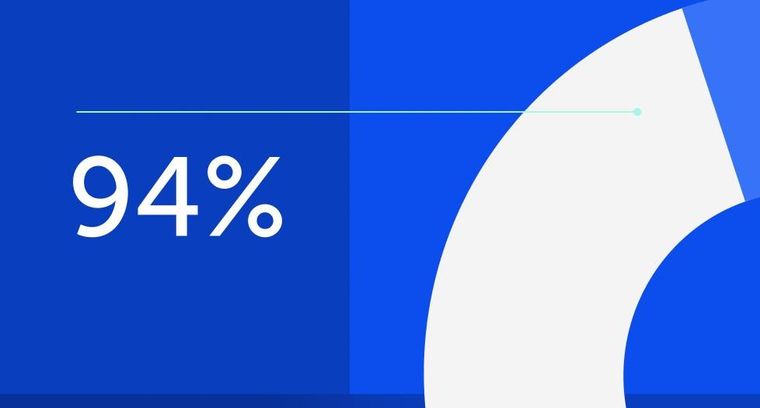
94% of researchers rate our articles as excellent or good
Learn more about the work of our research integrity team to safeguard the quality of each article we publish.
Find out more
MINI REVIEW article
Front. Plant Sci., 22 July 2014
Sec. Plant Physiology
Volume 5 - 2014 | https://doi.org/10.3389/fpls.2014.00363
This article is part of the Research TopicPlant Glycobiology – a sweet world of lectins, glycoproteins, glycolipids and glycansView all 14 articles
Asparagine (N)-linked protein glycosylation is a ubiquitous co- and post-translational modification which can alter the biological function of proteins and consequently affects the development, growth, and physiology of organisms. Despite an increasing knowledge of N-glycan biosynthesis and processing, we still understand very little about the biological function of individual N-glycan structures in plants. In particular, the N-glycan-processing steps mediated by Golgi-resident enzymes create a structurally diverse set of protein-linked carbohydrate structures. Some of these complex N-glycan modifications like the presence of β1,2-xylose, core α1,3-fucose or the Lewis a-epitope are characteristic for plants and are evolutionary highly conserved. In mammals, complex N-glycans are involved in different cellular processes including molecular recognition and signaling events. In contrast, the complex N-glycan function is still largely unknown in plants. Here, in this short review, I focus on important recent developments and discuss their implications for future research in plant glycobiology and plant biotechnology.
N-Glycosylation is a major co- and post-translational modification of proteins in all eukaryotes. It has been estimated that the majority of all secretory proteins are N-glycosylated (Apweiler et al., 1999). N-Glycosylation is initiated in the ER by transfer of a preassembled oligosaccharide (Glc3Man9GlcNAc2) precursor to asparagine residues within the sequence motif Asn–X–Ser/Thr (X represents any amino acid except proline) on nascent polypeptide chains. In addition, N-glycosylation at the unusual Asn–X–Cys site has also been described for some proteins (Matsui et al., 2011; Zielinska et al., 2012). Upon transfer of the oligosaccharide, the N-glycan is rapidly processed by highly specific α-glucosidases and α-mannosidases that remove terminal glucose and mannose residues, respectively. Incompletely trimmed N-glycans (Glc0-3Man5-9GlcNAc2) that contain different amounts of mannose residues (also called oligomannosidic N-glycans) are mainly found on ER-resident proteins (Figure 1A). The mannose trimming reactions are carried out by α-mannosidases (MNS1–MNS3) that act in the ER and Golgi (Liebminger et al., 2009). The Man5GlcNAc2 oligosaccharide, which is the final product of these early N-glycan-processing steps is used by GNTI as a acceptor substrate for the transfer of a single N-acetylglucosamine (GlcNAc) residue to the exposed α1,3-mannose of the N-glycan (Strasser et al., 1999). This enzymatic reaction is absolutely required for all further N-glycan modifications and results in the formation of complex N-glycans in the Golgi apparatus. In particular, GNTI generates the GlcNAc1Man5GlcNAc2 N-glycan that is further processed by Golgi-α-mannosidase II (GMII), GNTII, XYLT, and FUT11/12 (Figure 1B). All these enzymes are absolutely dependent on GNTI activity and reside in the cis/medial-Golgi apparatus of plants where they might form a multi-protein complex that could play a role for the organization of the glycosylation enzymes within the Golgi and subsequently also for the controlled processing of N-glycans (Schoberer and Strasser, 2011; Schoberer et al., 2013). GNTI is evolutionary highly conserved and present in land plants including mosses as well as in some microalgae (Strasser et al., 1999; Koprivova et al., 2003; Baïet et al., 2011). Due to its central function in initiation of complex N-glycan formation, GNTI controls the final N-glycosylation pattern on individual glycoproteins which can influence their biological function. XYLT and FUT11/12 attach β1,2-xylose and core α1,3-fucose residues, respectively, to different acceptor substrates and create common complex plant N-glycans like GlcNAc2XylFucMan3GlcNAc2 (GnGnXF, Figure 1A). Such complex N-glycans are not present in mammals and thus can elicit an unwanted anti-carbohydrate immune response when for example present on plant-produced recombinant glycoproteins (Bardor et al., 2003; Jin et al., 2008).
FIGURE 1. (A) Examples for two characteristic types of N-glycans linked to the Asn–X–Ser/Thr sequence of proteins: oligomannosidic (e.g., Man8) and complex-type (e.g., GnGnXF) N-glycans. (B) Possible route in the formation of complex N-glycans in plants. Upon transfer of the preassembled oligosaccharide, the first N-glycan trimming reactions are catalyzed by α-glucosidases I (GCSI)/ II (GCSII) and α-mannosidase 3 (MNS3). Complex N-glycan formation is initiated in the Golgi apparatus by β1,2-N-acetylglucosaminyltransferase I (GNTI, highlighted in red). MNS1/2, Golgi α-mannosidase I (two forms with largely redundant function are present in A. thaliana); GMII, Golgi α-mannosidase II; GNTII, β1,2-N-acetylglucosaminyltransferase II; XYLT, β1,2-xylosyltransferase; FUT11/12, core α1,3-fucosyltransferases (two forms with largely redundant function are present in A. thaliana); GALT1, Lewis-type β1,3-galactosyltransferase; FUT13, α1,4-fucosyltransferase. Structural analysis of N-glycans from different A. thaliana mutants and in vitro enzyme activity assays revealed that downstream of GNTI the substrate specificity of the processing enzymes is less stringent (Strasser et al., 2006, 2007b). Not shown: the possible removal of terminal GlcNAc residues by β-hexosaminidases (HEXO proteins) which generates paucimannosidic N-glycans in post-Golgi compartments or in the extracellular space (Liebminger et al., 2011). (C) The phenotypes of characteristic N-glycan-processing mutants are shown. While an N-glycan-processing defect (mns1 mns2 mns3) upstream of GNTI results in a severe root and shoot phenotype in A. thaliana (Liebminger et al., 2009), cgl1 (or gntI, the allelic A. thaliana T-DNA knockout mutant) does not display any growth or developmental phenotype under normal growth conditions (von Schaewen et al., 1993; Kang et al., 2008). In contrast, rice gnt1 displays a severe growth phenotype resulting in early lethality (Fanata et al., 2013). The major N-glycan structures of the mutants are indicated.
The final N-glycan modification steps take place in the trans-Golgi and are carried out by the Lewis-type β1,3-galactosyltransferase (GALT1) and the α1,4-fucosyltransferase (FUT13) which generate the Lewis a-trisaccharide [Fucα1-4(Galβ1-3)GlcNAc-R] on complex N-glycans (Strasser et al., 2007b). The Lewis a-type structures seem ubiquitous in the plant kingdom (Fitchette et al., 1999; Wilson et al., 2001), but they are only present on a small number of still widely unknown glycoproteins and the biological function of these large complex N-glycans remains to be shown.
Truncated N-glycans are generated by removal of terminal GlcNAc residues in post-Golgi compartments. These paucimannosidic N-glycans have been found on vacuolar and extracellular glycoproteins (Strasser et al., 2007a; Liebminger et al., 2011).
Early N-glycan-processing reactions mediated by α-glucosidase I and II are essential for Arabidopsis and presumably also for other plant species (Taylor et al., 2000; Boisson et al., 2001; Gillmor et al., 2002; Soussilane et al., 2009; Farid et al., 2011; Wang et al., 2014). The generated oligomannosidic N-glycans are implicated in folding of nascent polypeptides and play an important role during ER-quality control processes and ERAD of misfolded or incompletely assembled glycoproteins (Aebi, 2013). The overall principles of these processes are conserved in eukaryotes. Recent findings suggest that monoglucosylated N-glycans in the ER are important for association with the lectins calreticulin or calnexin also in plants. For example, the pattern recognition receptor EFR involved in innate immunity and a misfolded variant of the brassinosteroid receptor BRI1 displayed a selective interaction with the plant-specific calreticulin 3 (Jin et al., 2009; Li et al., 2009). Additional data suggest that N-glycans present on these heavily glycosylated leucine-rich repeat receptor kinases are subjected to re-glucosylation by the folding sensor UDP-glucose:glycoprotein glucosyltransferase (UGGT) and glucosidase-mediated de-glucosylation followed by a release from the calreticulin/calnexin quality control cycle (reviewed in Liu and Li, 2014; Tintor and Saijo, 2014). Moreover, specific mannose residues present on terminally misfolded glycoproteins play also a crucial role for the selective disposal via ERAD (Hong et al., 2009, 2012; Hüttner et al., 2012, 2014) and a complete block of mannose removal in the Arabidopsis mns1 mns2 mns3 triple mutant causes also a severe root growth phenotype (Figure 1C; Liebminger et al., 2009).
In all higher eukaryotes, GNTI is the central enzyme that initiates complex N-glycan formation on secreted and membrane-bound proteins that are trafficking through the Golgi to their final destination. Early studies in mice revealed that GNTI is essential for the development of embryos (Ioffe and Stanley, 1994; Metzler et al., 1994), but cultured mammalian cells can survive in the absence of complex N-glycans (Stanley et al., 1975). More recent genetic approaches revealed that the structurally diverse complex N-glycans on mammalian proteins participate in many different biological processes and distinct alterations are often associated with diseases (Lowe and Marth, 2003; Dennis et al., 2009). Drosophila melanogaster deficient in GNTI activity are viable, but display distinct phenotypes like abnormal brain development and a reduced life span (Sarkar et al., 2006). Caenorhabditis elegans GNTI-null mutants develop normally but are more susceptible to bacterial pathogens (Schachter, 2010). Together these findings highlight the importance of complex N-glycan modifications in various organisms.
In spite of the fact that complex N-glycans are ubiquitously present in plants (Wilson et al., 2001), their biological function is virtually unknown. The first mutant lacking complex N-glycans was isolated more than two decades ago by EMS mutagenesis of Arabidopsis and subsequent screening for lines that lack β1,2-linked xylose and core α1,3-fucose residues (von Schaewen et al., 1993). The isolated complex glycan 1 (cgl1) mutants displayed a defect in the formation of complex N-glycans due to a point mutation in the gene coding for GNTI (Strasser et al., 2005). Consequently, in cgl1 all endogenous glycoproteins carry exclusively oligomannosidic N-glycans with Man5GlcNAc2 as predominant oligosaccharide. Remarkably, the Arabidopsis cgl1 plants are viable, fertile and do not display any obvious phenotype under different growth conditions including heat (30°C) and cold (8°C) stress or increased light conditions (von Schaewen et al., 1993; Figure 1C). Related studies identified various other Arabidopsis mutants with distinct defects in N-glycan-processing steps downstream of GNTI. In line with data for cgl1, no clear growth or developmental phenotypes were observed for Arabidopsis mutants that produce hybrid structures (Strasser et al., 2006) or complex N-glycans devoid of β1,2-xylose and core α1,3-fucose residues (Strasser et al., 2004). In agreement with these findings, neither the complete elimination nor the overexpression of the Lewis a-type structures on complex N-glycans caused a substantial change in Arabidopsis growth or development when grown under long day conditions (16 h-light/8 h-dark) at 22°C (Strasser et al., 2007b). Up to now, the only evidence for a biological function of complex N-glycans in Arabidopsis was found when cgl1 and other mutants were subjected to osmotic and salt stress (Kang et al., 2008). Reduced root growth on media containing high NaCl concentrations indicated that complex N-glycans are implicated in tolerance to salt stress. However, a deeper understanding of complex N-glycan function in Arabidopsis and studies that associate distinct complex N-glycan structures on individual glycoproteins with the enhanced salt sensitivity are completely missing.
Based on the aforementioned studies, it has been suggested that N-glycan processing in the Golgi is dispensable for the normal development of plants and plays only a role under certain stress conditions. A recent study by Fanata et al. (2013) challenges our current view and provides strong evidence that complex N-glycans play indeed an essential role in some plant species. A homozygous Oryza sativa line (gnt1) with a T-DNA insertion in the single rice GNTI gene was identified that completely abolished GNTI mRNA expression. As a consequence of missing GNTI transcripts and in accordance with the central function of GNTI in the formation of complex N-glycans, the rice gnt1 mutant displayed only oligomannosidic N-glycans with approximately 75% Man5GlcNAc2 structures. Almost the same amounts of Man5GlcNAc2 N-glycans were found for Arabidopsis cgl1 (von Schaewen et al., 1993; Strasser et al., 2005). However, in marked contrast to Arabidopsis cgl1, a severe phenotype with arrested seedling development and lethality before reaching the reproductive stage was reported for rice gnt1 (Fanata et al., 2013; Figure 1C). In addition, rice gnt1 plants displayed defects in cell wall composition and cytokinin insensitivity. Although the final confirmation that the observed severe phenotypes are indeed linked to defects in gnt1 is missing as the cytokinin defect caused problems with complementation of the gnt1 plants, all other data are convincing and indicate that complex N-glycans are essential in some plant species. How can we explain this discrepancy between Arabidopsis and rice? Based on data from total N-glycan analysis and annotation of the rice genome, it is quite likely that the N-glycan-processing steps in the Golgi are very similar between the two species (Figure 1B). However, there might be subtle differences in cell-/tissue-specific expression of certain N-glycan-processing enzymes that might have been missed by total N-glycan analysis from whole plant organs. Interestingly, the rice genome contains more than one glycosyltransferase with homology to Arabidopsis GALT1 (Strasser et al., 2007b) and it seems that the formation of Lewis a-type structures occurs more frequently in rice than in Arabidopsis (Léonard et al., 2004; Strasser et al., 2004, 2007b). The rice GALT1 homologs belong to Carbohydrate-Active enzyme glycosyltransferase-family 31, which contains a large number of enzymes with quite diverse functions (Strasser et al., 2007b; Basu et al., 2013). These GALT1 candidates have not been characterized and in the absence of data from plants devoid of Lewis a-type structures, their contribution to the development of rice remains an open question. Moreover, N-glycosylation defects are generally pleiotropic and affect numerous secretory as well as membrane-anchored proteins. Consequently, the observed phenotype in rice gnt1 could arise from several different glycoproteins that are dysfunctional in the absence of Golgi-mediated N-glycan processing. As rice gnt1 displays reduced cellulose contents, glycoproteins involved in cellulose biosynthesis could be affected (Fanata et al., 2013). While impaired N-glycosylation or N-glycan processing has also been linked to changes in cellulose contents in Arabidopsis (Burn et al., 2002; Gillmor et al., 2002; Zhang et al., 2009) gntI/cgl1 does not contain significantly altered cellulose contents compared to wild-type Arabidopsis (Kang et al., 2008). Recently, it was also shown that the heavily glycosylated endoglucanase KORRIGAN1, whose enzymatic activity is important for efficient cellulose formation, does not need complex N-glycans for its function (Liebminger et al., 2013).
Based on the detected cytokinin insensitivity it was speculated that members of the cytokinin-receptor family are N-glycosylated and their function might be impaired in the rice gnt1 line (Fanata et al., 2013). These histidine sensor kinases contain an extracellular domain of approximately 280 amino acids with putative N-glycosylation sites (Caesar et al., 2011; Steklov et al., 2013). The degree of N-glycosylation and the N-glycan structures of cytokinin receptors are not very well known, but for Arabidopsis AHK3 N-glycosylation could be shown by transient expression in tobacco (Caesar et al., 2011). Notably, in Arabidopsis as well as in maize these receptors were primarily found in the ER implying that cytokinin binding takes place in this compartment (Caesar et al., 2011; Lomin et al., 2011; Wulfetange et al., 2011). If so, then Golgi-processed complex N-glycans are very likely not present on cytokinin receptors and consequently these receptors are not directly affected in GNTI-deficient rice.
To understand the mechanisms underlying the observed defects in rice gnt1 and compare them with data from other plants species a number of key experimental approaches have to be explored: (i) It is very important to isolate other rice N-glycan-processing mutants to pin down the complex N-glycan structure or individual sugar residue that is crucial for the growth and development of rice. (ii) There is an urgent need for high-throughput glycoproteome approaches that enable the isolation of a large number of glycoproteins and mapping of the corresponding N-glycan structures from different plant species. Advances in this field will be crucial for structure–function analysis and identification of target glycoproteins. Plant N-glycoproteome studies have been reported recently (Zhang et al., 2011; Zielinska et al., 2012; Song et al., 2013), but compared to other posttranslational modifications these approaches are still too limited (Albenne et al., 2013). (iii) Information on regulation of glycosylation enzymes as well as information on cell-type or organ-specific occurrence of certain glycan structures is almost completely missing. Tools that have been used for the cell-type-specific analysis of protein expression (Petricka et al., 2012) should also be applied to unravel the N-glycoproteome in different plant species. (iv) Up to now, null mutants devoid of N-glycan processing have been characterized from Arabidopsis and rice, but information on the significance of N-glycosylation and complex N-glycan formation in other vascular plants is missing. Together, the highlighted experimental approaches will enable us to decode the biological function of the so far largely unknown complex N-glycan modifications like the attachment of β1,2-xylose, core α1,3-fucose, and the formation of the Lewis a-type structures.
Plants are emerging hosts for the manufacturing of valuable recombinant proteins. Recently, the first plant-produced recombinant biopharmaceutical, a recombinant human glucocerebrosidase, has been approved for enzyme replacement therapy in humans and is commercially available in the United States (Grabowski et al., 2014). Many biopharmaceutical proteins like human immunoglobulins or hormones are glycosylated and the composition of the glycans very often affect protein–protein interactions leading to altered efficacies of the recombinant drugs or unwanted side-effects like fast clearance from the blood or increased immunogenicity. Consequently, for the pharmaceutical industry as well as for structure–function studies, there is a growing demand to modify and control protein glycosylation of expression hosts. The ultimate aim of these approaches is the production of recombinant glycoproteins with defined and homogenous glycan structures (Rich and Withers, 2009; Dalziel et al., 2014). Developments during the last 10 years have shown that plants are amenable to glyco-engineering and capable of producing valuable recombinant glycoproteins with defined human-like structures (Castilho and Steinkellner, 2012; Nagels et al., 2012; Bosch et al., 2013). The absence of any growth phenotype in Arabidopsis cgl1 laid the foundation for N-glycan engineering of other species like Nicotiana benthamiana and Lemna minor as well as of rice suspension cells (Cox et al., 2006; Strasser et al., 2008; Shin et al., 2011). In these studies, gene silencing of XYLT and FUT11/12 was used to eliminate the non-human and potentially immunogenic β1,2-xylose and core α1,3-fucose residues from complex N-glycans of recombinant proteins. Overall, these glyco-engineering efforts were quite successful, but the plants still produced low amounts of complex N-glycans like GnGnXF. A detailed characterization of null mutants for XYLT and FUT11/12 will reveal whether these and other plant species tolerate the absence of β1,2-xylose and core α1,3-fucose residues on endogenous glycoproteins during their whole life cycle. In addition, further studies are necessary to investigate in detail the consequences on growth, development, reproduction and stress response of stable engineered plants that carry human-type complex N-glycan modifications. So far, these knock-in approaches were limited to a small number of plant species and mainly to stable expression of single mammalian glycosyltransferases (Bakker et al., 2001; Rouwendal et al., 2007; Castilho et al., 2008; Sourrouille et al., 2008; Frey et al., 2009; Nagels et al., 2011). In contrast, most of the more advanced glyco-engineering approaches that require the concerted action of several mammalian enzymes were done by simultaneous transient expression of whole glycosylation pathways (Castilho et al., 2010, 2012, 2013). The stable expression of the proteins and enzymes involved in multi-step N-glycan processing like the formation of highly sialylated complex N-glycans without any negative effects on plant growth and development remains to be shown.
In the light of the recent findings from rice, glyco-engineering in some plant species might require new strategies and implementation of more elaborate tools to overcome adverse phenotypes linked with extensive N-glycan remodeling. In terms of plant glycobiology, the new findings from rice open the door for an exciting new era.
The author declares that the research was conducted in the absence of any commercial or financial relationships that could be construed as a potential conflict of interest.
The author apologizes to colleagues whose work is not cited due to space limitations. This work was supported by a grant from Austrian Science Fund (FWF): P23906-B20.
EMS, ethyl methanesulfonate; ERAD, ER-associated degradation; GNTI, β1,2-N-acetylglucosaminyltransferase I; FUT11/12, core α1,3-fucosyltransferase; XYLT, β1,2-xylosyltransferase
Aebi, M. (2013). N-linked protein glycosylation in the ER. Biochim. Biophys. Acta 1833, 2430–2437. doi: 10.1016/j.bbamcr.2013.04.001
Albenne, C., Canut, H., and Jamet, E. (2013). Plant cell wall proteomics: the leadership of Arabidopsis thaliana. Front. Plant Sci. 4:111. doi: 10.3389/fpls.2013.00111
Apweiler, R., Hermjakob, H., and Sharon, N. (1999). On the frequency of protein glycosylation, as deduced from analysis of the SWISS-PROT database. Biochim. Biophys. Acta 1473, 4–8. doi: 10.1016/S0304-4165(99)00165-8
Baïet, B., Burel, C., Saint-Jean, B., Louvet, R., Menu-Bouaouiche, L., Kiefer-Meyer, M. C.,et al. (2011). N-glycans of Phaeodactylum tricornutum diatom and functional characterization of its N-acetylglucosaminyltransferase I enzyme. J. Biol. Chem. 286, 6152–6164. doi: 10.1074/jbc.M110.175711
Bakker, H., Bardor, M., Molthoff, J., Gomord, V., Elbers, I., Stevens, L.,et al. (2001). Galactose-extended glycans of antibodies produced by transgenic plants. Proc. Natl. Acad. Sci. U.S.A. 98, 2899–2904. doi: 10.1073/pnas.031419998
Bardor, M., Faveeuw, C., Fitchette, A., Gilbert, D., Galas, L., Trottein, F.,et al. (2003). Immunoreactivity in mammals of two typical plant glyco-epitopes, core alpha(1,3)-fucose and core xylose. Glycobiology 13, 427–434. doi: 10.1093/glycob/cwg024
Basu, D., Liang, Y., Liu, X., Himmeldirk, K., Faik, A., Kieliszewski, M.,et al. (2013). Functional identification of a hydroxyproline-o-galactosyltransferase specific for arabinogalactan protein biosynthesis in Arabidopsis. J. Biol. Chem. 288, 10132–10143. doi: 10.1074/jbc.M112.432609
Boisson, M., Gomord, V., Audran, C., Berger, N., Dubreucq, B., Granier, F.,et al. (2001). Arabidopsis glucosidase I mutants reveal a critical role of N-glycan trimming in seed development. EMBO J. 20, 1010–1019. doi: 10.1093/emboj/20.5.1010
Bosch, D., Castilho, A., Loos, A., Schots, A., and Steinkellner, H. (2013). N-glycosylation of plant-produced recombinant proteins. Curr. Pharm. Des. 19, 5503–5512. doi: 10.2174/1381612811319310006
Burn, J., Hurley, U., Birch, R., Arioli, T., Cork, A., and Williamson, R. (2002). The cellulose-deficient Arabidopsis mutant rsw3 is defective in a gene encoding a putative glucosidase II, an enzyme processing N-glycans during ER quality control. Plant J. 32, 949–960. doi: 10.1046/j.1365-313X.2002.01483.x
Caesar, K., Thamm, A. M., Witthöft, J., Elgass, K., Huppenberger, P., Grefen, C.,et al. (2011). Evidence for the localization of the Arabidopsis cytokinin receptors AHK3 and AHK4 in the endoplasmic reticulum. J. Exp. Bot. 62, 5571–5580. doi: 10.1093/jxb/err238
Castilho, A., Neumann, L., Daskalova, S., Mason, H. S., Steinkellner, H., Altmann, F.,et al. (2012). Engineering of sialylated mucin-type O-glycosylation in plants. J. Biol. Chem. 287, 36518–36526. doi: 10.1074/jbc.M112.402685
Castilho, A., Neumann, L., Gattinger, P., Strasser, R., Vorauer-Uhl, K., Sterovsky, T.,et al. (2013). Generation of biologically active multi-sialylated recombinant human EPO-Fc in plants. PLoS ONE 8:e54836. doi: 10.1371/journal.pone.0054836
Castilho, A., Pabst, M., Leonard, R., Veit, C., Altmann, F., Mach, L.,et al. (2008). Construction of a functional CMP-sialic acid biosynthesis pathway in Arabidopsis. Plant Physiol. 147, 331–339. doi: 10.1104/pp.108.117572
Castilho, A., and Steinkellner, H. (2012). Glyco-engineering in plants to produce human-like N-glycan structures. Biotechnol. J. 7, 1088–1098. doi: 10.1002/biot.201200032
Castilho, A., Strasser, R., Stadlmann, J., Grass, J., Jez, J., Gattinger, P.,et al. (2010). In planta protein sialylation through overexpression of the respective mammalian pathway. J. Biol. Chem. 285, 15923–15930. doi: 10.1074/jbc.M109.088401
Cox, K., Sterling, J., Regan, J., Gasdaska, J., Frantz, K., Peele, C.,et al. (2006). Glycan optimization of a human monoclonal antibody in the aquatic plant Lemna minor. Nat. Biotechnol. 24, 1591–1597. doi: 10.1038/nbt1260
Dalziel, M., Crispin, M., Scanlan, C. N., Zitzmann, N., and Dwek, R. A. (2014). Emerging principles for the therapeutic exploitation of glycosylation. Science 343, 1235681. doi: 10.1126/science.1235681
Dennis, J. W., Nabi, I. R., and Demetriou, M. (2009). Metabolism, cell surface organization, and disease. Cell 139, 1229–1241. doi: 10.1016/j.cell.2009.12.008
Fanata, W. I., Lee, K. H., Son, B. H., Yoo, J. Y., Harmoko, R., Ko, K. S.,et al. (2013). N-glycan maturation is crucial for cytokinin-mediated development and cellulose synthesis in Oryza sativa. Plant J. 73, 966–979. doi: 10.1111/tpj.12087
Farid, A., Pabst, M., Schoberer, J., Altmann, F., Glössl, J., and Strasser, R. (2011). Arabidopsis thaliana alpha1,2-glucosyltransferase (ALG10) is required for efficient N-glycosylation and leaf growth. Plant J. 68, 314–325. doi: 10.1111/j.1365-313X.2011.04688.x
Fitchette, A., Cabanes-Macheteau, M., Marvin, L., Martin, B., Satiat-Jeunemaitre, B., Gomord, V.,et al. (1999). Biosynthesis and immunolocalization of Lewis a-containing N-glycans in the plant cell. Plant Physiol. 121, 333–344. doi: 10.1104/pp.121.2.333
Frey, A. D., Karg, S. R., and Kallio, P. T. (2009). Expression of rat beta(1,4)-N-acetylglucosaminyltransferase III in Nicotiana tabacum remodels the plant-specific N-glycosylation. Plant Biotechnol. J. 7, 33–48. doi: 10.1111/j.1467-7652.2008.00370.x
Gillmor, C., Poindexter, P., Lorieau, J., Palcic, M., and Somerville, C. (2002). Alpha-glucosidase I is required for cellulose biosynthesis and morphogenesis in Arabidopsis. J. Cell Biol. 156, 1003–1013. doi: 10.1083/jcb.200111093
Grabowski, G. A., Golembo, M., and Shaaltiel, Y. (2014). Taliglucerase alfa: an enzyme replacement therapy using plant cell expression technology. Mol. Genet. Metab. 112, 1–8. doi: 10.1016/j.ymgme.2014.02.011
Hong, Z., Jin, H., Fitchette, A., Xia, Y., Monk, A., Faye, L.,et al. (2009). Mutations of an alpha1,6 mannosyltransferase inhibit endoplasmic reticulum-associated degradation of defective brassinosteroid receptors in Arabidopsis. Plant Cell 21, 3792–3802. doi: 10.1105/tpc.109.070284
Hong, Z., Kajiura, H., Su, W., Jin, H., Kimura, A., Fujiyama, K.,et al. (2012). Evolutionarily conserved glycan signal to degrade aberrant brassinosteroid receptors in Arabidopsis. Proc. Natl. Acad. Sci. U.S.A. 109, 11437–11442. doi: 10.1073/pnas.1119173109
Hüttner, S., Veit, C., Schoberer, J., Grass, J., and Strasser, R. (2012). Unraveling the function of Arabidopsis thaliana OS9 in the endoplasmic reticulum-associated degradation of glycoproteins. Plant Mol. Biol. 79, 21–33. doi: 10.1007/s11103-012-9891-4
Hüttner, S., Veit, C., Vavra, U., Schoberer, J., Liebminger, E., Maresch, D.,et al. (2014). Arabidopsis class I mannosidases MNS4 and MNS5 are involved in ER-associated degradation of misfolded glycoproteins. Plant Cell 26, 1712–1728. doi: 10.1105/tpc.114.123216
Ioffe, E., and Stanley, P. (1994). Mice lacking N-acetylglucosaminyltransferase I activity die at mid-gestation, revealing an essential role for complex or hybrid N-linked carbohydrates. Proc. Natl. Acad. Sci. U.S.A. 91, 728–732. doi: 10.1073/pnas.91.2.728
Jin, C., Altmann, F., Strasser, R., Mach, L., Schähs, M., Kunert, R.,et al. (2008). A plant-derived human monoclonal antibody induces an anti-carbohydrate immune response in rabbits. Glycobiology 18, 235–241. doi: 10.1093/glycob/cwm137
Jin, H., Hong, Z., Su, W., and Li, J. (2009). A plant-specific calreticulin is a key retention factor for a defective brassinosteroid receptor in the endoplasmic reticulum. Proc. Natl. Acad. Sci. U.S.A. 106, 13612–13617. doi: 10.1073/pnas.0906144106
Kang, J., Frank, J., Kang, C., Kajiura, H., Vikram, M., Ueda, A.,et al. (2008). Salt tolerance of Arabidopsis thaliana requires maturation of N-glycosylated proteins in the Golgi apparatus. Proc. Natl. Acad. Sci. U.S.A. 105, 5933–5938. doi: 10.1073/pnas.0800237105
Koprivova, A., Altmann, F., Gorr, G., Kopriva, S., Reski, R., and Decker, E. (2003). N-Glycosylation in the moss Physcomitrella patens is organized similarly to higher plants. Plant Biol. 5, 582–591. doi: 10.1055/s-2003-44721
Léonard, R., Kolarich, D., Paschinger, K., Altmann, F., and Wilson, I. (2004). A genetic and structural analysis of the N-glycosylation capabilities of rice and other monocotyledons. Plant Mol. Biol. 55, 631–644. doi: 10.1007/s11103-004-1558-3
Li, J., Zhao-Hui, C., Batoux, M., Nekrasov, V., Roux, M., Chinchilla, D.,et al. (2009). Specific ER quality control components required for biogenesis of the plant innate immune receptor EFR. Proc. Natl. Acad. Sci. U.S.A. 106, 15973–15978. doi: 10.1073/pnas.0905532106
Liebminger, E., Grass, J., Altmann, F., Mach, L., and Strasser, R. (2013). Characterizing the link between glycosylation state and enzymatic activity of the endo-β1,4-glucanase KORRIGAN1 from Arabidopsis thaliana. J. Biol. Chem. 288, 22270–22280. doi: 10.1074/jbc.M113.475558
Liebminger, E., Hüttner, S., Vavra, U., Fischl, R., Schoberer, J., Grass, J.,et al. (2009). Class I alpha-mannosidases are required for N-glycan processing and root development in Arabidopsis thaliana. Plant Cell 21, 3850–3867. doi: 10.1105/tpc.109.072363
Liebminger, E., Veit, C., Pabst, M., Batoux, M., Zipfel, C., Altmann, F.,et al. (2011). N-Acetylhexosaminidases HEXO1 and HEXO3 are responsible for the formation of paucimannosidic N-glycans in Arabidopsis thaliana. J. Biol. Chem. 286, 10793–10802. doi: 10.1074/jbc.M110.178020
Liu, Y., and Li, J. (2014). Endoplasmic reticulum-mediated protein quality control in Arabidopsis. Front. Plant Sci. 5:162. doi: 10.3389/fpls.2014.00162
Lomin, S. N., Yonekura-Sakakibara, K., Romanov, G. A., and Sakakibara, H. (2011). Ligand-binding properties and subcellular localization of maize cytokinin receptors. J. Exp. Bot. 62, 5149–5159. doi: 10.1093/jxb/err220
Lowe, J. B., and Marth, J. D. (2003). A genetic approach to mammalian glycan function. Annu. Rev. Biochem. 72, 643–691. doi: 10.1146/annurev.biochem.72.121801.161809
Matsui, T., Takita, E., Sato, T., Kinjo, S., Aizawa, M., Sugiura, Y.,et al. (2011). N-glycosylation at noncanonical Asn-X-Cys sequences in plant cells. Glycobiology 21, 994–999. doi: 10.1093/glycob/cwq198
Metzler, M., Gertz, A., Sarkar, M., Schachter, H., Schrader, J. W., and Marth, J. D. (1994). Complex asparagine-linked oligosaccharides are required for morphogenic events during post-implantation development. EMBO J. 13, 2056–2065.
Nagels, B., Van Damme, E. J., Pabst, M., Callewaert, N., and Weterings, K. (2011). Production of complex multiantennary N-glycans in Nicotiana benthamiana plants. Plant Physiol. 155, 1103–1112. doi: 10.1104/pp.110.168773
Nagels, B., Weterings, K., Callewaert, N., and Van Damme, E. J. M. (2012). Production of plant made pharmaceuticals: from plant host to functional protein. Crit. Rev. Plant Sci. 31, 148–180. doi: 10.1080/07352689.2011.616075
Petricka, J. J., Schauer, M. A., Megraw, M., Breakfield, N. W., Thompson, J. W., Georgiev, S.,et al. (2012). The protein expression landscape of the Arabidopsis root. Proc. Natl. Acad. Sci. U.S.A. 109, 6811–6818. doi: 10.1073/pnas.1202546109
Rich, J. R., and Withers, S. G. (2009). Emerging methods for the production of homogeneous human glycoproteins. Nat. Chem. Biol. 5, 206–215. doi: 10.1038/nchembio.148
Rouwendal, G. J., Wuhrer, M., Florack, D. E., Koeleman, C. A., Deelder, A. M., Bakker, H.,et al. (2007). Efficient introduction of a bisecting GlcNAc residue in tobacco N-glycans by expression of the gene encoding human N-acetylglucosaminyltransferase III. Glycobiology 17, 334–344. doi: 10.1093/glycob/cwl078
Sarkar, M., Leventis, P. A., Silvescu, C. I., Reinhold, V. N., Schachter, H., and Boulianne, G. L. (2006). Null mutations in Drosophila N-acetylglucosaminyltransferase I produce defects in locomotion and a reduced life span. J. Biol. Chem. 281, 12776–12785. doi: 10.1074/jbc.M512769200
Schachter, H. (2010). Mgat1-dependent N-glycans are essential for the normal development of both vertebrate and invertebrate metazoans. Semin. Cell Dev. Biol. 21, 609–615. doi: 10.1016/j.semcdb.2010.02.010
Schoberer, J., Liebminger, E., Botchway, S. W., Strasser, R., and Hawes, C. (2013). Time-resolved fluorescence imaging reveals differential interactions of N-glycan processing enzymes across the Golgi stack in planta. Plant Physiol. 161, 1737–1754. doi: 10.1104/pp.112.210757
Schoberer, J., and Strasser, R. (2011). Sub-compartmental organization of Golgi-resident N-glycan processing enzymes in plants. Mol. Plant 4, 220–228. doi: 10.1093/mp/ssq082
Shin, Y. J., Chong, Y. J., Yang, M. S., and Kwon, T. H. (2011). Production of recombinant human granulocyte macrophage-colony stimulating factor in rice cell suspension culture with a human-like N-glycan structure. Plant Biotechnol. J. 9, 1109–1119. doi: 10.1111/j.1467-7652.2011.00636.x
Song, W., Mentink, R. A., Henquet, M. G., Cordewener, J. H., van Dijk, A. D., Bosch, D.,et al. (2013). N-glycan occupancy of Arabidopsis N-glycoproteins. J. Proteomics 93, 343–355. doi: 10.1016/j.jprot.2013.07.032
Sourrouille, C., Marquet-Blouin, E., D’Aoust, M. A., Kiefer-Meyer, M. C., Seveno, M., Pagny-Salehabadi, S.,et al. (2008). Down-regulated expression of plant-specific glycoepitopes in alfalfa. Plant Biotechnol. J. 6, 702–721. doi: 10.1111/j.1467-7652.2008.00353.x
Soussilane, P., Soussillane, P., D’Alessio, C., Paccalet, T., Fitchette, A., Parodi, A.,et al. (2009). N-glycan trimming by glucosidase II is essential for Arabidopsis development. Glycoconj. J. 26, 597–607. doi: 10.1007/s10719-008-9201-1
Stanley, P., Narasimhan, S., Siminovitch, L., and Schachter, H. (1975). Chinese hamster ovary cells selected for resistance to the cytotoxicity of phytohemagglutinin are deficient in a UDP-N-acetylglucosamine-glycoprotein N-acetylglucosaminyltransferase activity. Proc. Natl. Acad. Sci. U.S.A. 72, 3323–3327. doi: 10.1073/pnas.72.9.3323
Steklov, M. Y., Lomin, S. N., Osolodkin, D. I., and Romanov, G. A. (2013). Structural basis for cytokinin receptor signaling: an evolutionary approach. Plant Cell Rep. 32, 781–793. doi: 10.1007/s00299-013-1408-3
Strasser, R., Altmann, F., Mach, L., Glössl, J., and Steinkellner, H. (2004). Generation of Arabidopsis thaliana plants with complex N-glycans lacking beta1,2-linked xylose and core alpha1,3-linked fucose. FEBS Lett. 561, 132–136. doi: 10.1016/S0014-5793(04)00150-4
Strasser, R., Bondili, J., Schoberer, J., Svoboda, B., Liebminger, E., Glössl, J.,et al. (2007a). Enzymatic properties and subcellular localization of Arabidopsis N-acetylhexosaminidases. Plant Physiol. 145, 5–16. doi: 10.1104/pp.107.101162
Strasser, R., Bondili, J., Vavra, U., Schoberer, J., Svoboda, B., Glössl, J.,et al. (2007b). A unique beta1,3-galactosyltransferase is indispensable for the biosynthesis of N-glycans containing Lewis a structures in Arabidopsis thaliana. Plant Cell 19, 2278–2292. doi: 10.1105/tpc.107.052985
Strasser, R., Mucha, J., Schwihla, H., Altmann, F., Glössl, J., and Steinkellner, H. (1999). Molecular cloning and characterization of cDNA coding for beta1,2N-acetylglucosaminyltransferase I (GlcNAc-TI) from Nicotiana tabacum. Glycobiology 9, 779–785. doi: 10.1093/glycob/9.8.779
Strasser, R., Schoberer, J., Jin, C., Glössl, J., Mach, L., and Steinkellner, H. (2006). Molecular cloning and characterization of Arabidopsis thaliana Golgi alpha-mannosidase II, a key enzyme in the formation of complex N-glycans in plants. Plant J. 45, 789–803. doi: 10.1111/j.1365-313X.2005.02648.x
Strasser, R., Stadlmann, J., Schähs, M., Stiegler, G., Quendler, H., Mach, L.,et al. (2008). Generation of glyco-engineered Nicotiana benthamiana for the production of monoclonal antibodies with a homogeneous human-like N-glycan structure. Plant Biotechnol. J. 6, 392–402. doi: 10.1111/j.1467-7652.2008.00330.x
Strasser, R., Stadlmann, J., Svoboda, B., Altmann, F., Glössl, J., and Mach, L. (2005). Molecular basis of N-acetylglucosaminyltransferase I deficiency in Arabidopsis thaliana plants lacking complex N-glycans. Biochem. J. 387, 385–391. doi: 10.1042/BJ20041686
Taylor, M., Ross, H., Mcrae, D., Stewart, D., Roberts, I., Duncan, G.,et al. (2000). A potato alpha-glucosidase gene encodes a glycoprotein-processing alpha-glucosidase II-like activity. Demonstration of enzyme activity and effects of down-regulation in transgenic plants. Plant J. 24, 305–316. doi: 10.1046/j.1365-313x.2000.00873.x
Tintor, N., and Saijo, Y. (2014). ER-mediated control for abundance, quality, and signaling of transmembrane immune receptors in plants. Front. Plant Sci. 5:65. doi: 10.3389/fpls.2014.00065
von Schaewen, A., Sturm, A., O’Neill, J., and Chrispeels, M. (1993). Isolation of a mutant Arabidopsis plant that lacks N-acetyl glucosaminyl transferase I and is unable to synthesize Golgi-modified complex N-linked glycans. Plant Physiol. 102, 1109–1118. doi: 10.1104/pp.102.4.1109
Wang, S., Xu, Y., Li, Z., Zhang, S., Lim, J. M., Lee, K. O.,et al. (2014). OsMOGS is required for N-glycan formation and auxin-mediated root development in rice (Oryza sativa L.). Plant J. 78, 632–645. doi: 10.1111/tpj.12497
Wilson, I., Zeleny, R., Kolarich, D., Staudacher, E., Stroop, C., Kamerling, J.,et al. (2001). Analysis of Asn-linked glycans from vegetable foodstuffs: widespread occurrence of Lewis a, core alpha1,3-linked fucose and xylose substitutions. Glycobiology 11, 261–274. doi: 10.1093/glycob/11.4.261
Wulfetange, K., Lomin, S. N., Romanov, G. A., Stolz, A., Heyl, A., and Schmülling, T. (2011). The cytokinin receptors of Arabidopsis are located mainly to the endoplasmic reticulum. Plant Physiol. 156, 1808–1818. doi: 10.1104/pp.111.180539
Zhang, M., Henquet, M., Chen, Z., Zhang, H., Zhang, Y., Ren, X.,et al. (2009). LEW3, encoding a putative alpha-1,2-mannosyltransferase (ALG11) in N-linked glycoprotein, plays vital roles in cell-wall biosynthesis and the abiotic stress response in Arabidopsis thaliana. Plant J. 60, 983–999. doi: 10.1111/j.1365-313X.2009.04013.x
Zhang, Y., Giboulot, A., Zivy, M., Valot, B., Jamet, E., and Albenne, C. (2011). Combining various strategies to increase the coverage of the plant cell wall glycoproteome. Phytochemistry 72, 1109–1123. doi: 10.1016/j.phytochem.2010.10.019
Keywords: endoplasmic reticulum, Golgi apparatus, protein glycosylation, N-glycosylation, glycoprotein, N-acetylglucosaminyltransferase
Citation: Strasser R (2014) Biological significance of complex N-glycans in plants and their impact on plant physiology. Front. Plant Sci. 5:363. doi: 10.3389/fpls.2014.00363
Received: 30 May 2014; Accepted: 08 July 2014;
Published online: 22 July 2014.
Edited by:
Els J. M. Van Damme, Ghent University, BelgiumCopyright © 2014 Strasser. This is an open-access article distributed under the terms of the Creative Commons Attribution License (CC BY). The use, distribution or reproduction in other forums is permitted, provided the original author(s) or licensor are credited and that the original publication in this journal is cited, in accordance with accepted academic practice. No use, distribution or reproduction is permitted which does not comply with these terms.
*Correspondence: Richard Strasser, Department of Applied Genetics and Cell Biology, University of Natural Resources and Life Sciences, Muthgasse 18, 1190 Vienna, Austria e-mail:richard.strasser@boku.ac.at
Disclaimer: All claims expressed in this article are solely those of the authors and do not necessarily represent those of their affiliated organizations, or those of the publisher, the editors and the reviewers. Any product that may be evaluated in this article or claim that may be made by its manufacturer is not guaranteed or endorsed by the publisher.
Research integrity at Frontiers
Learn more about the work of our research integrity team to safeguard the quality of each article we publish.