- 1College of Life Science, Northeast Forestry University, Harbin, China
- 2Forest Genetics and Ecophysiology Research Group, School of Forest Engineering, Technical University of Madrid, Madrid, Spain
- 3School of Forestry, Northeast Forestry University, Harbin, China
While plant roots are specialized organs for the uptake and transport of water and nutrients, the absorption of gaseous or liquid mineral elements by aerial plant parts has been recognized since more than one century. Nitrogen (N) is an essential macronutrient which generally absorbed either as nitrate (NO−3) or ammonium (NH+4) by plant roots. Gaseous nitrogen pollutants like N dioxide (NO2) can also be absorbed by plant surfaces and assimilated via the NO−3 assimilation pathway. The subsequent NO−3 flux may induce or repress the expression of various NO−3-responsive genes encoding for instance, the transmembrane transporters, NO−3/NO−2 (nitrite) reductase, or assimilatory enzymes involved in N metabolism. Based on the existing information, the aim of this review was to theoretically analyze the potential link between foliar NO2 absorption and N transport and metabolism. For such purpose, an overview of the state of knowledge on the NO−3 transporter genes identified in leaves or shoots of various species and their roles for NO−3 transport across the tonoplast and plasma membrane, in addition to the process of phloem loading is briefly provided. It is assumed that a NO2-induced accumulation of NO−3/NO−2 may alter the expression of such genes, hence linking transmembrane NO−3 transporters and foliar uptake of NO2. It is likely that NRT1/NRT2 gene expression and species-dependent apoplastic buffer capacity may be also related to the species-specific foliar NO2 uptake process. It is concluded that further work focusing on the expression of NRT1 (NRT1.1, NRT1.7, NRT1.11, and NRT1.12), NRT2 (NRT2.1, NRT2.4, and NRT2.5) and chloride channel family genes (CLCa and CLCd) may help us elucidate the physiological and metabolic response of plants fumigated with NO2.
Introduction
Nitrate (NO−3) is the most common form of nitrogen used by plants for growth and development (Bertoni, 2012). Despite the major role of plant roots is absorbing and transporting water and mineral elements, there is abundant evidence showing that nutrients can also be taken up by aerial plant parts (e.g., leaves, fruits and stems) (Eichert and Fernández, 2012; Fernández and Brown, 2013). Foliar applied NO−3 may be absorbed and assimilated efficiently as shown in several studies carried out with different plant species (e.g., Stiegler et al., 2011; Uscola et al., 2014). Gaseous air pollutants like nitrogen dioxide (NO2) can also be deposited into plant leaves and be taken up mainly through stomata (Eichert and Fernández, 2012). NO2 molecules may dissolve in the aqueous phase of the apoplastic space being consequently transformed into nitrate (NO−3) and/or nitrite (NO−2) by chemical reactions. Thereafter, NO2-derived NO−3 may be transported across the plasma membrane by NO−3 transporters and reach the cytoplasm for further incorporation into cellular N-compounds and/or storage in vacuoles (Hawkesford et al., 2012). The NO−3 stored in the vacuoles may be exported to compensate for the consumption of NO−3 in the metabolic pool (De Angeli et al., 2006), which suggests that vacuolar NO−3 may largely serve as N buffer for transport processes (Hawkesford et al., 2012). NO−3/proton antiporters, which may be encoded by chloride channel family (CLC) genes, are responsible for NO−3 influx into plant vacuoles (Geelen et al., 2000). NO−3 transmembrane transporters may be expected to play a role after the uptake of exogenous NO2 or NO−3 by the foliage. Several NO−3 transporters identified in leaves have been demonstrated to be closely correlated with e.g., stomatal opening (Guo et al., 2003), NO−3 reductase activity (Loqué et al., 2003), accumulation and remobilization of NO−3 (De Angeli et al., 2006; Fan et al., 2009; Lv et al., 2009). Thereby, such physiological processes may significantly influence and also be affected by the foliar uptake of NO2 or NO−3.
Recently some NO−3 transporter genes were detected in leaves including several members of plant NRT1 family genes (e.g., AtNRT1.1, AtNRT1.4, AtNRT1.7, AtNRT1.11, and AtNRT1.12,.), NRT2 family genes (e.g., AtNRT2.1, AtNRT2.3, AtNRT2.4, AtNRT2.5, AtNRT2.6, AtNRT2.7, NpNRT2.1 and ZmNrt2.1.), and CLC family genes such as AtClCa and AtClCd (Orsel et al., 2002; Guo et al., 2003; Chopin et al., 2007; Fan et al., 2009; Hsu and Tsay, 2013) (Figure 1). These genes show various expression levels in leaves and play diverse roles in regulating NO−3 metabolism (Hawkesford et al., 2012). For example, AtNRT1.1, a dual-affinity NO−3-inducible transporter, showed a strong expression in guard cells and supports the stomatal function in the presence of NO−3 (Guo et al., 2003). McNRT1, LeNRT1.1, and NpNRT1.1 were detected in the leaves of M. crystallinum, tomato and Nicotiana plumbaginifolia, respectively. McNRT1, sharing 60% homology with the AtNRT1.1, was expressed in the mesophyll cells and cells adjacent to metaxylem vessels in the leaves (Popova et al., 2003). The gene plays potential roles in NO−3 uptake in the mesophyll cells, distribution and partitioning of NO−3 within the leaves. Moreover, AtNRT1.4 and AtNRT1.7 are of pure low-affinity transporters. AtNRT1.4 was expressed primarily in the leaf petiole (Chiu et al., 2004). NRT1.7 mRNA was detected in the distal lamina of older leaves, but not in the roots (Fan et al., 2009). The two transporter genes participate in the process of leaf NO−3 storage and remobilization. For the members of the NRT2 family, the amounts of AtNRT2.4 transcripts were predominant in leaves of the adult plants, followed by AtNRT2.5; the expression of AtNRT2.1, AtNRT2.6, and AtNRT2.7 were at low levels (Orsel et al., 2002). AtNRT2.1, AtNRT2.3, AtNRT2.4, and AtNRT2.5 are NO−3-responsive genes, whereas AtNRT2.6 and AtNRT2.7 appear to be constitutive genes (Loqué et al., 2003). Particularly, AtNRT2.7 showed a strong leaf- and seed-specific expression pattern (Orsel et al., 2002; Chopin et al., 2007), while AtNRT2.3 was specifically expressed in leaves at a reproductive stage.
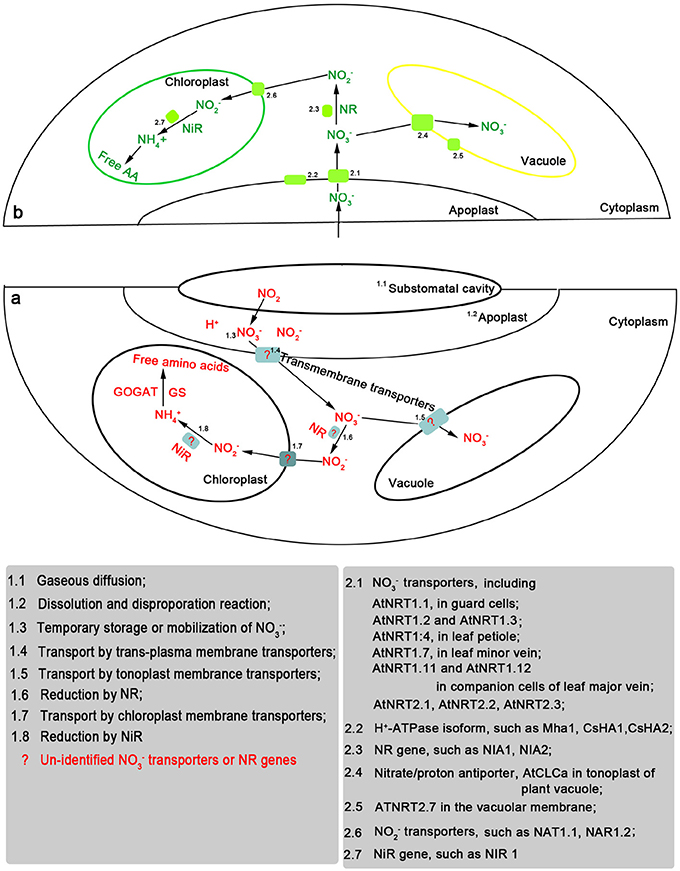
Figure 1. Nitrate (NO−3) transporters and NR/NiR genes potentially involved after foliar uptake and assimilation of exogenous nitrogen dioxide (NO2) (a)/ NO−3 (b).
Previous studies on foliar uptake of NO2 mainly focused on the deposition pathways, metabolic processes associated with NO2-derived NO−3 (Hu, 2011; Hu et al., 2014), and downstream products of NO2-N assimilation (Nussbaum et al., 1993; Weber et al., 1995). The current state of knowledge on the potential plant responses to NO2 exposure is summarized in Table 1. However, the relationship between NO−3 transmembrane transporters and foliar NO2 uptake has received only limited scientific attention so far. Foliar uptake of NO2 seems to be species-specific and concentration-dependent (Hu and Sun, 2010). Expression of the genes encoding leaf NO−3 transporters also appears to be species-specific (Ono et al., 2000; Orsel et al., 2002). The contribution of various expression patterns of transporter genes to species-specific NO2 uptake is currently unknown. Given the N transport mechanisms described above, a potential relationship between foliar NO2 uptake (substomatal build-up of NO2 and the subsequent reduction, storage, and remobilization of NO−3) and NO−3-responsive genes encoding the transmembrane transporters and NO−3/NO−2 reductases may be hypothesized. For validating such hypothesis, future work focusing on the relationship between organ-specific expression of NRT1/NRT2 genes and species-specific NO2 uptake should be carried out.
Substomatal Build-Up of NO2 may Disturb Apoplastic pH and NO−3 Transporters
The apoplast is defined as the area within the plant tissues which is beyond the cell plasma membrane, and includes the cell wall, middle lamella, xylem and gas and water filled intercellular spaces (Sattelmacher, 2001). The leaf apoplastic space plays a role in ion exchange and as a diffusion barrier (Sattelmacher, 2001). Estimates of the volume of leaf water in the apoplast vary from 10 to 35% of total leaf water (Speer and Kaiser, 1991; Wardlaw, 2005). Dissolution of NO2 in the apoplast may produce H+ and NO−3/NO−2. Foliar NO2 uptake is calculated to yield at most 0.22 mol excess H+ per mol N (Raven, 1988). Therefore, a build-up of NO2 in the leaf substomatal cavities may lead to apoplastic pH disturbances. Among other factors, the resulting apoplastic pH changes will depend on NO2 concentration, root N supply and plant N status. The supply of NO−3 via the root system significantly increased the leaf apoplastic pH of Phaseolus vulgaris and Helianthus annuus, whereas the depletion of NO−3 in nutrient solution led to lower leaf apoplastic pH values in Zea mays (Mühling and Lauchli, 2001). NH4NO3 nutrition did not change the leaf apoplastic pH in sunflower (Kosegarten et al., 1999). Moreover, foliar NH+4 fertilization may either lead to apoplastic alkalinization (Felle and Hanstein, 2002) or acidification (Mühling and Lauchli, 2001). When supplying NH4C1 (1 mM) via the root to soybean plants, low concentrations of NO2 (0.2–0.25 μL· L−1) significantly increased the leaf apoplastic pH (Qiao and Murray, 1997), whereas under a higher root NO−3 dose (5 mM), high concentrations of NO2 (1.1 μ l· l−1) increased the acidity of the leaves (Qiao and Murray, 1998). Apoplastic pH is an important factor affecting plasmalemma proton pumps (Hoffmann et al., 1992; Sattelmacher, 2001). Apoplastic alkalinization or acidification may induce plasma membrane depolarization or hyperpolarization (Hedrich et al., 2001). This may further modulate the deactivation or activation of membrane-bound proton-transporting enzymes, and the corresponding ion channel regulation for co-transport of anions (Savchenko et al., 2000). Wippel et al. (2010) found that the fluctuation of apoplastic pH had a regulatory effect on plant sucrose transporters. Based on the above information, it can be reckoned that the apoplastic pH changes caused by NO2 may repress or induce NO−3-responsive genes encoding the transmembrane transporters.
Some NO−3 transporter genes (such as AtNRT1.1 and ZmNrt2.1) in leaves are NO−3-inducible, while others such as AtNRT2.5 are NO−3-repressible (Okamoto et al., 2003). Low-affinity transporter systems (NRT1 family) may significantly contribute to NO−3 uptake at external NO−3 concentrations above 250 μ M. However, high-affinity transporters (NRT2 family) including the constitutive (cHATS Km = 6–20 mM) and inducible HATS (Km = 20–100 mM), are active at low external concentrations of 0–0.5 mM (Crawford and Glass, 1998; Quaggiotti et al., 2003; Hawkesford et al., 2012). When analyzing the AtNRT1.7 NO−3 transporter gene in Arabidopsis, Fan et al. (2009) applied 50 mM K15NO3 to carry out measurements on distal parts of the rosette leaf. The 15N-NO−3 tracing assay showed that the percentage of total 15N in the leaves ranged from 0 to 10% for wild-type plants, and between 5 and 15% for the nrt1.7 mutants. The percentage of NO−3–15N was in the range of the NO2-derived reduced N of wild herbaceous plants (from 0.98 to 10.1%) and woody plants (0.15–12.7%) for the 217 taxa fumigated with 4.0 ± 0.1 μmol· mol−1 NO2 (Morikawa et al., 1998). Accordingly, the content of NO2-derived reduced N ranged from 0.25 to 5.72 mg N· g−1 dry weight for wild herbaceous plants, and 0.04–6.57 mg N· g−1 dry weight for woody plants. This comparison suggests that the amounts of NO2-derived NO−3 in leaves are in the range of the NO−3 concentrations which may induce the two types of transporter systems (i.e., high and low affinity).
From the reasoning provided above, it can be reckoned that substomatal build-up of NO2 may lead to concentration-dependent changes of apoplastic pH and NO−3 concentration. Such pH fluctuations may influence NO−3 transmembrane transport by the induction or repression of the transporters and transporter gene expression, and may provide some sort of feedback regulation on the uptake of NO2 by the foliage. For example, apoplastic mesophyll signals have been recently found to induce rapid stomatal responses in Commelina communis (Fujita et al., 2013). In response to NO2 fumigation, multiple physiological and metabolic responses may occur which could either ultimately favor or inhibit the process of symplastic N uptake (Table 1). The multi-responses of NO−3 transporters to the substomatal build-up of NO2 may partially contribute to species-specific NO2 uptake, but future studies with different plant species shall be carried out for clarifying this complex issue.
Nitrate Transporters are Possibly Involved in the Reduction and Accumulation of NO2-Derived NO−3
In leaf cytoplasm, NO2-derived NO−3 has at least two fates: (i) assimilation into amino acids, and (ii) accumulation in vacuole (Hawkesford et al., 2012). The metabolic pathway will depend on the external NO−3 concentration and leaf N demand (Stulen et al., 1998). NO2-derived NO−3 will be assimilated mainly through the NO−3 assimilation pathway (Morikawa et al., 1998). NO−3 reductase (NR) is considered as a key rate-limiting enzyme of NO2-N assimilation (Hawkesford et al., 2012). A linear correlation was found between NR activity, NO2 concentration and amounts of N incorporated into amino acids (Sparks et al., 2001). However, high levels of NO2 fumigation resulted into a loss of NR activity or a rapid inactivation of the leaf NR (Takeuchi et al., 1985; Hisamatsu et al., 1988), and NO−3 accumulation (Ma et al., 2007). This down-regulation of the NR may be ascribed to at least one of the following phenomena: (i) a high NO2 concentration will inhibit the activities of glutamine synthetase and glutamate synthase, which leads to NH+4 accumulation and subsequently brings about a loss of NR activity (Orebamjo and Stewart, 1975; Padidam et al., 1991), and (ii) a high NO2-induced stomatal closure may lead to a rapid NR inactivation due to a low CO2 availability. High NO2 rapidly induced stomatal closure (Qiao and Murray, 1998). Stomatal closure may trigger a chain reaction wherein the lower CO2 availability will lead to the subsequent leaf NR activity decrease (Kaiser and Forster, 1989). NIA1 and NIA2 genes encode the two isoforms of the NR apoprotein (Wilkinson and Crawford, 1993). Recent reports show a close relationship between the expression of NIA1/NIA2 genes and NRT1/NRT2 genes. Addition of external NO−3 strongly induced the expression of genes encoding the NR (AtNIA1 and AtNIA2) and the transmembrane transporters (AtNRT1.1, AtNRT2.1, NpNRT2.1; Fraisier et al., 2001; Jonassen et al., 2009). In contrast, high levels of external NO−3 caused a down-regulation of AtNRT1.1 and AtNIA1 through a pathway of NO−2-induced repression (Loqué et al., 2003). This down-regulation of the AtNRT1.1 gene is associated with a decrease in the NO−3 influx. Earlier studies showed that high NO2 concentration fumigation under light or dark conditions resulted in leaf NO−2 accumulation (Yoneyama and Sasakawa, 1979; Yu et al., 1988). Thus, we may assume that a high NO2-caused NO−2 accumulation may lead to a negative feedback regulation on leaf NO2 uptake through the down-regulation of the NRT1.1 gene and the subsequent repression of the NO−3 influx. Moreover, studies on NR mutants showed that AtNRT1.1, AtNRT1.7, NpNRT2.1, and AtNIA1 are up-regulated in NR-deficient mutants (NIA2- and/or MoCo biosynthesis-deficient mutants) (Lejay et al., 1999; Vidmar et al., 2000; Fan et al., 2009). Under NR-repressible or -deficient conditions, this up-regulation of the transporter genes may be beneficial to an exportation of excess NO−3 in the leaf (Fan et al., 2009). Moreover, Jonassen et al. (2009) demonstrated that the bZIP transcription factors HY5 and HYH regulate positively NIA2 gene and negatively NRT1.1 gene. However, HY5 and HYH appear to be mediated by light but not by external NO−3.
Excess NO−3 may be accumulated in leaf vacuole (Hawkesford et al., 2012). The H+/NO−3 antiport across tonoplast is responsible for NO−3 influx and H+/NO−3 symport for NO−3 efflux (Figure 1). The flux direction will depend on the requirements and conditions of the cell (Schumaker and Sze, 1987). Three members of the chloride channel family (CLC) genes AtClCa (De Angeli et al., 2006), AtClCc (Harada et al., 2004) and AtClCd (Lv et al., 2009) have been identified in the leaf tonoplast. De Angeli et al. (2009) demonstrated that adenosine triphosphate (ATP) induces a negative regulation on AtCLCa activity. NO2 fumigation significantly increased ATP amounts of Lolium perenne and Phleum pratense, the amounts increasing with raising NO2 concentrations (Wellburn et al., 1981). This may be due to the formation of free radicals in response to NO2 fumigation, which may damage photosynthetic membranes and hence alter the proton gradients to which ATP formation is linked (Wellburn et al., 1981). Yoneyama and Sasakawa (1979) found that 8 ppm NO2 fumigation under dark conditions resulted in NO−2 accumulation in spinach leaves. High doses of NO−2 resulted in the peroxidation of lipid constituents of chloroplastic membrane (Ezzine and Ghorbel, 2006). Chen et al. (2010) found that leaf uptake of NO2 reduced the rate of photosynthesis and increased the malondialdehyde (MDA) concentration, may be due to a competition for nicotinamide adenine dinucleotide phosphate (NADPH) between the processes of NO−2 reduction vs. carbon assimilation, and the generation of reactive oxygen species (ROS) (Sabaratnam and Gupat, 1988; Shimazaki et al., 1992).
Transmembrane Transporters in Leaves Mediating NO−3 Signaling
NO2 fumigation may significantly disrupt plant morphology and physiology by, for instance, changing the shoot to root ratio, stomatal, and gas exchange dynamics, or modifying root N uptake (Qiao and Murray, 1997, 1998; Table 1). The exposure of plants to NO2 increased the total content of soluble free amino acids in leaves and shoots (Nussbaum et al., 1993). Most of the amino acids may be used locally for the synthesis of e.g., Chlorophyll and Rubisco during rapid vegetative growth, or be ultimately designated for e.g., filling pods (Imsande and Touraine, 1994). NO−3 assimilation products (protein/nucleic acids and amino acids/amides) can also be transferred into roots under soil N deficit (Wellburn, 1990). Under a low NO−3 supply, gaseous NO2 may change the amino acid ratio of the xylem. For example, the amount of serine, asparagine and glutamine were high in the xylem of plants exposed to atmospheric NO2, whereas arginine, cysteine, valine and lysine were high in the control plants (Wellburn, 1990; Table 1). Moreover, NO2 treatment increased phloem transport of organic N and inhibited the rate of xylem N translocation.
NO2-N metabolism and the mobilization of metabolic products will trigger various signaling pathways that regulate the physiological and metabolic processes. The dissolution of NO2 and subsequent reduction can result in root NO−3 uptake changes. The xylem is part of leaf apoplast (Felle and Hanstein, 2002). Thus, NO2-caused apoplastic pH changes may serve as a signal to modify the uptake of NO−3 via the root system. This NO−3 signaling pathway has been explained by Qiao and Murray (1998). Moreover, NO−3 reduction can produce malate (Touraine et al., 1988); the organic acid needs to be membrane transported to be loaded into the phloem. A transport of malate from leaves to roots can serve as another signal to control root uptake of NO−3 (Touraine et al., 1992). This signaling pathway has been reported by Imsande and Touraine (1994). Tonoplast transport of malate plays an important role in physiological regulation in NO−3 nutrition (Hawkesford et al., 2012). At the cellular level, NO−3 accumulation led to increased expression of genes encoding organic acid synthesis (PPC, cytosolic PK, CS, ICDH-1) and accumulation of malate and a-oxoglutarat. In contrast, leaf malate supply can inhibit NIA expression, affecting both the NIA transcript level and the activity (Müller et al., 2001).
NO−3 itself may serve as a signaling molecule (Scheible et al., 1997). NO−3 addition to the growing media can induce or repress the expression of various genes encoding e.g., NO−3 transporters, NO−3/NO−2 reductase, ferredoxin reductase, and the enzymes in the pentose phosphate pathway, or iron or sulfate transport and metabolism (Wang et al., 2003; Marschner, 2012). The expression of NO−3-responsive genes [such as NADH-specific and NAD(P)H-bispecific NR genes] is dependent upon NO−3 flux but not on the NO−3 amount stored in the tissue (Gojon et al., 1991). Excess external NO−3 may be stored in several vacuoles and recirculated after storage (Hawkesford et al., 2012). NO−3 remobilization may occur among different organs, for instance, from older leaves to younger leaves during the vegetative stage or from leaves to seeds during the reproductive stage (Schiltz et al., 2005; Hawkesford et al., 2012). N remobilization is rate-limited by the transport of NO−3 across tonoplast of vacuole, plasma membrane of mesophyll cell, plasma membrane of companion cell and sieve element, and phloem loading (Fan et al., 2009). CLC genes (AtClCa, AtClCc, and AtClCd) are required for the transport of NO−3 across tonoplast in vacuole. Disruption of one of these genes will influence the flux of NO−3 in vacuoles (De Angeli et al., 2006). AtNRT2.4 showed a strong induction in a low NO−3 provision. Orsel et al. (2004) suggested that AtNRT2.4 and AtNRT2.5 participate in the transport of NO−3 from stored pools (vacuoles) to cytoplasm. Moreover, AtNRT2.7 was also involved in this type of NO−3 flux; this gene could play roles in leaf balance between the amount of NO−3 used for assimilation and that re-absorbed for further transport (Orsel et al., 2002). Four NRT1 family genes (AtNRT1.4, AtNRT1.7, AtNRT1.11, and AtNRT1.12) participate in the phloem- and/or xylem-loading of NO−3 (Figure 1). AtNRT1.4 was expressed predominantly in the leaf petiole and involved in petiole NO−3 accumulation (Chiu et al., 2004). The mutation of the AtNRT1:4 resulted in significant changes of NO−3 content in leaf petiole and the lamina. Furthermore, the deficiency of AtNRT1.4 can alter leaf development. NRT1.7 was expressed in the phloem of the leaf minor vein and mediated the remobilization of excess NO−3 from older leaves to younger leaves (Fan et al., 2009). Compared with the wild-type plants, the nrt1.7 null mutants accumulated a higher amount of NO−3 in the older leaves and decreased the NO−3 content of phloem exudates from older leaves. The newly identified NO−3 transporters (NRT1.11 and NRT1.12) were expressed in the companion cells of the major vein (Hsu and Tsay, 2013). They play roles in xylem-to-phloem transfer for redistributing NO−3 into developing leaves. Moreover, several NRT2 genes may also be involved in the N remobilization. For example, ZmNrt2.1 plays a potential role in NO−3 loading from the xylem or in its compartmentation (Quaggiotti et al., 2003).
Conclusion
The substomatal build-up of NO2 and subsequent NO−3 metabolism may lead to apoplastic alkalinization or acidification and to NO−3/NO−2 concentration fluctuations in the leaf apoplast and symplast (e.g., cytoplasm and vacuole), which depend on NO2 concentration and root N supply. These changes will cause complex responses of NO−3-responsive genes encoding NO−3 transporters and NO−3/NO−2 reductase. For example, addition of external NO−3 produced a strong induction on NR genes (such as AtNIA1 and AtNIA2) and the transporter genes (such as AtNRT1.1, AtNRT2.1, and NpNRT2.1). However, excess NO−2 significant inhibited the expression of AtNRT1.1 and AtNIA1, and disturbed CLC family genes by regulating the generation of ATP. This down-regulation of the NRT1.1 gene is associated with a decrease in NO−3 influx. Moreover, AtNRT2.4, AtNRT2.5, and AtNRT2.7 may participate in the transfer of NO−3 from stored pools (vacuoles) to cytoplasm. AtNRT1.4, AtNRT1.7, AtNRT1.11, and AtNRT1.12 are involved in the phloem- and/or xylem-loading of NO−3. Thus, these genes are suggested to play rate-limiting roles in foliar uptake of NO2. Further work is proposed to investigate the relationship between organ specificity of NRT1/NRT2 gene expression and species-specific NO2 uptake.
In practical terms, a high rate of low concentration NO2 absorption by the foliage may be positive for preserving an adequate plant N status. However, a high NO2 concentration may alter leaf apoplast chemistry, leading to the accumulation of NO−3 and NO−2, and providing signals which may negatively affect plant N nutrition. These factors are however closely linked with leaf NO−3 transporters and may also interact with the foliar uptake processes (e.g., by promoting stomatal closure). Thereby, a low NO2 concentration may act as a positive regulation signal (Takahashi et al., 2014) by stimulating the leaf NO−3 transporters, and enhancing NO−3 transport and distribution. In contrast, a high NO2 concentration in relation to a high rate of foliar NO2 absorption, may repress the expression of NO−3 transporters and enzymes, which may protect the cells or organelles from NO2 damage.
Author Contributions
Yanbo Hu organized and wrote the original manuscript; Yanbo Hu and Victoria Fernandez discussed and revised the revising manuscript and approved the final version; Ling Ma collected the information for Table 1, and went through the manuscript.
Conflict of Interest Statement
The authors declare that the research was conducted in the absence of any commercial or financial relationships that could be construed as a potential conflict of interest.
Acknowledgments
This work was supported by grants from “Fundamental Research Funds for the Central Universities” (Grant No. 2572014CA24, DL10BB24) and “National Natural Science Foundation” (Grant No. 31300506).
References
Bertoni, G. (2012). A nitrate transporter for both roots and shoots. Plant Cell 24, 1. doi: 10.1105/tpc.112.240110
Carlson, R. W. (1983). Interaction between SO2 and NO2 and their effects on photosynthetic properties of soybean Glycine max. Environ. Pollut. Ecol. Biol. 32, 11–38. doi: 10.1016/0143-1471(83)90071-5
Chen, Z. M., Chen, Y. X., Du, G. J., Wu, X. L., and Li, F. (2010). Effects of 60-day NO2 fumigation on growth, oxidative stress and antioxidative response in Cinnamomum camphora seedlings. J. Zhejiang Univ. Sci. B 11, 190–199. doi: 10.1631/jzus.B0910350
Chiu, C. C., Lin, C. S., Hsia, A. P., Su, R. C., Lin, H. L., and Tsay, Y. F. (2004). Mutation of a nitrate transporter, AtNRT1:4, results in a reduced petiole nitrate content and altered leaf development. Plant Cell Physiol. 45, 1139–1148. doi: 10.1093/pcp/pch143
Chopin, F., Orsel, M., Dorbe, M. F., Chardon, F., Truong, H. N., Miller, A. J., et al. (2007). The Arabidopsis AtNRT2.7 nitrate transporter controls nitrate content in seeds. Plant Cell 19, 1590–1602. doi: 10.1105/tpc.107.050542
Crawford, N. M., and Glass, A. D. M. (1998). Molecular and physiological aspects of nitrate uptake in plants. Trends Plant Sci. 10, 389–395. doi: 10.1016/S1360-1385(98)01311-9
De Angeli, A., Monachello, D., Ephritikhine, G., Frachisse, J. M., Thomine, S., Gambale, F., et al. (2006). The nitrate/proton antiporter AtCLCa mediates nitrate accumulation in plant vacuoles. Nature 442, 939–942. doi: 10.1038/nature05013
De Angeli, A., Moran, O., Wege, S., Filleur, S., Ephritikhine, G., Thomine, S., et al. (2009). ATP binding to the C terminus of the Arabidopsis thaliana nitrate/proton antiporter, AtCLCa, regulates nitrate transport into plant vacuoles. J. Biol. Chem. 284, 26526–26532. doi: 10.1074/jbc.M109.005132
Dolzmann, P., and Ullrich, H. (1966). Einige Beobachtungen iiber Beziehungen zwischen Chloroplasten und Mitchondrien im Palisadenparenchym von Phaseolus vulgaris. Zeitschrift fiir Pflanzenphysiologie 55, 165–180.
Eastham, A. M., and Ormrod, D. P. (1986). Visible injury and growth responses of young cuttings of Populus Canadensis and P. nigra to nitrogen dioxide and sulphur dioxide. Can J. For. Res. 16, 1289–1292. doi: 10.1139/x86-228
Eichert, T., and Fernández, V. (2012). “Uptake and release of elements by leaves and other aerial plant parts,” in Marschner's Mineral Nutrition of Higher Plants, ed P. Marschner (Oxford: Academic Press), 71–84.
Ezzine, M., and Ghorbel, M. H. (2006). Physiological and biochemical responses resulting from nitrite accumulation in tomato. (Lycopersicon esculentum Mill. cv. Ibiza F1). J. Plant Physiol. 163, 1032–1039. doi: 10.1016/j.jplph.2005.07.013
Fan, S. C., Lin, C. S., Hsu, P. K., Lin, S. H., and Tsay, Y. F. (2009). The Arabidopsis nitrate transporter NRT1.7, expressed in phloem, is responsible for source-to-sink remobilization of nitrate. Plant Cell 21, 2750–2761. doi: 10.1105/tpc.109.067603
Felle, H. H., and Hanstein, S. (2002). The apoplastic pH of the substomatal cavity of Vicia faba leaves and its regulation responding to different stress factors. J. Exp. Bot. 53, 73–82. doi: 10.1093/jexbot/53.366.73
Fernández, V., and Brown, P. H. (2013). From plant surface to plant metabolism: the uncertain fate of foliar-applied nutrients. Front. Plant Sci. 4:289. doi: 10.3389/fpls.2013.00289
Fraisier, V., Dorbe, M. F., and Daniel-Vedele, F. (2001). Identification and expression analyses of two genes encoding putative low-affinity nitrate transporters from Nicotiana plumbaginifolia. Plant Mol. Biol. 45, 181–190. doi: 10.1023/A:1006426616760
Fujita, T., Noguchi, K., and Terashima, I. (2013). Apoplastic mesophyll signals induce rapid stomatal responses to CO2 in Commelinacommunis. New Phytol. 199, 395–406. doi: 10.1111/nph.12261
Geelen, D., Lurin, C., Bouchez, D., Frachisse, J. M., Lelièvre, F., Courtial, B., et al. (2000). Disruption of putative anion channel gene AtCLC-a in Arabidopsis suggests a role in the regulation of nitrate content. Plant J. 21, 259–267. doi: 10.1046/j.1365-313x.2000.00680.x
Gojon, A., Wakrim, R., Passama, L., and Robin, P. (1991). Regulation of NO–3 assimilation by anion availability in excised soybean leaves. Plant Physiol. 96, 398–405. doi: 10.1104/pp.96.2.398
Guo, F. Q., Young, J., and Crawford, N. M. (2003). The nitrate transporter AtNRT1.1 (CHL1) functions in stomatal opening and contributes to drought susceptibility in Arabidopsis. Plant Cell 15, 107–117. doi: 10.1105/tpc.006312
Harada, H., Kuromori, T., Hirayama, T., Shinozaki, K., and Leigh, R. A. (2004). Quantitative trait loci analysis of nitrate storage in Arabidopsis leading to an investigation of the contribution of the anion channel gene, AtCLC-c, to variation in nitrate levels. J. Exp. Bot. 55, 2005–2014. doi: 10.1093/jxb/erh224
Hawkesford, M., Horst, W., Kichey, T., Lambers, H., Schjoerring, J., Skrumsager, I., et al. (2012). “Functions of Macronutrients,” in Marschner's Mineral Nutrition of Higher Plants, 3rd Edn., eds P. Marschner (London: Elsevier), 135–178.
Hedrich, R., Neimainis, S., Savchenko, G., Felle, H. H., Kaiser, W. M., and Heber, U. (2001). Changes in apoplastic pH and membrane potential in leaves in relation to stomatal responses to CO2, malate, abscisic acid or interruption of water supply. Planta 213, 594–601. doi: 10.1007/s004250100524
Hisamatsu, S., Nihira, J., Takeuchi, Y. C., Satoh, S., and Kondo, N. (1988). NO2 suppression of light-induced nitrate reductase in squash cotyledons. Plant Cell Physiol. 29, 395-401.
Hoffmann, B., Plänker, R., and Mengel, K. (1992). Measurements of pH in the apoplast of sunflower leaves by means of fluorescence. Physiol. Plant. 84, 146-153. doi: 10.1111/j.1399-3054.1992.tb08777.x
Hsu, P. K., and Tsay, Y. F. (2013). Two phloem nitrate transporters, NRT1.11 and NRT1.12, are important for redistributing xylem-borne nitrate to enhance plant growth. Plant Physiol. 163, 844–856. doi: 10.1104/pp.113.226563
Hu, Y. B. (2011). NO2-drived NO−3 metabolism in leaves. Insci. J. 1, 90–101. doi: 10.5640/insc.010290
Hu, Y. B., Bellaloui, N., Sun, G. Y., Tigabu, M., and Wang, J. H. (2014). Exogenous sodium sulfide improves morphological and physiological responses of a hybrid Populus species to nitrogen dioxide. J. Plant Physiol. 171, 868–875. doi: 10.1016/j.jplph.2013.10.018
Hu, Y. B., and Sun, G. Y. (2010). Leaf nitrogen dioxide uptake coupling apoplastic chemistry, carbon/sulfur assimilation, and plant nitrogen status. Plant Cell Rep. 29, 1069-1077. doi: 10.1007/s00299-010-0898-5
Imsande, J., and Touraine, B. (1994). N demand and the regulation of nitrate uptake. Plant Physiol. 105, 3-7.
Ito, O., Okano, K., Kuroiwa, M., and Totsuka, T. (1985). Effects of NO2 and O3 alone or in combination on kidney bean plants (Phaseolus vulgaris L.): partitioning of assimilates and root activities. J. Exp. Bot. 36, 652–662. doi: 10.1093/jxb/36.4.652
Jonassen, E. M., Sevin, D. C., and Lillo, C. (2009). The bZIP transcription factors HY5 and HYH are positive regulators of the main nitrate reductase gene in Arabidopsis leaves, NIA2, but negative regulators of the nitrate uptake gene NRT1.1. J. Plant Physiol. 166, 2071–2076. doi: 10.1016/j.jplph.2009.05.010
Kaiser, W. M., and Forster, J. (1989). Low CO2 prevents nitrate reduction in leaves. Plant Physiol. 91, 970-974. doi: 10.1104/pp.91.3.970
Kondo, K., Yamada, K., Nakagawa, A., Takahashi, M., Morikawa, H., and Sakamoto, A. (2008). Molecular characterization of atmospheric NO2-responsive germin-like proteins in azalea leaves. Biochem. Biophys. Res. Commun. 377, 857–861. doi: 10.1016/j.bbrc.2008.10.060
Kosegarten, H. U., Hoffmann, B., and Mengel, K. (1999). Apoplastic pH and Fe3+ reduction in intact sunflower leaves. Plant Physiol. 121, 1069-1079. doi: 10.1104/pp.121.4.1069
Lejay, L., Tillard, P., Lepetit, M., Olive, F. D., Filleur, S., Daniel-Vedele, F., et al. (1999). Molecular and functional regulation of two NO−3 uptake systems by N- and C-status of Arabidopsis plants. Plant J. 18, 509–519. doi: 10.1046/j.1365-313X.1999.00480.x
Loqué, D., Tillard, P., Gojon, A., and Lepetit, M. (2003). Gene expression of the NO−3 transporter NRT1.1 and the nitrate reductase NIA1 is repressed in Arabidopsis roots by NO−2, the product of NO−3 reduction. Plant Physiol. 132, 958–967. doi: 10.1104/pp.102.018523
Lv, Q. D., Tang, R. J., Liu, H., Gao, X. S., Li, Y. Z., Zheng, H. Q., et al. (2009). Cloning and molecular analyses of the Arabidopsis thaliana chloride channel gene family. Plant Sci. 176, 650–661. doi: 10.1016/j.plantsci.2009.02.006
Ma, C. Y., Xu, X., Hao, L., and Cao, J. (2007). Nitrogen dioxide-induced responses in Brassica campestris seedlings: the role of hydrogen peroxide in the modulation of antioxidative level and induced resistance. Agric. Sci. China 6, 1193–1200. doi: 10.1016/S1671-2927(07)60163-1
Morikawa, H., Higaki, A., Nohno, M., Takahashi, M., Kamada, M., Nakata, M., et al. (1998). More than a 600-fold variation in nitrogen dioxide assimilation among 217 plant taxa. Plant Cell Environ. 21, 180–190. doi: 10.1046/j.1365-3040.1998.00255.x
Mühling, K. H., and Lauchli, A. (2001). Influence of chemical form and concentration of nitrogen on apoplastic pH of leaves. J. Plant Nutr. 25, 399–411. doi: 10.1081/PLN-100104968
Müller, C., Scheible, W. R., Stitt, M., and Krapp, A. (2001). Influence of malate and 2-oxoglutarate on the NIA transcript level and nitrate reductase activity in tobacco leaves. Plant Cell Environ. 24, 191–203. doi: 10.1111/j.1365-3040.2001.00664.x
Murray, F., Wilson, S., and Samaraweera, S. (1994). NO2 increases wheat grain yield even in the presence of SO2. Agric. Ecosyst. Environ. 48, 115–123. doi: 10.1016/0167-8809(94)90082-5
Nussbaum, S., Ballmoos, P. V., Gfeller, H., Schlunegger, U. P., Fuhrer, J., Rhodes, D., et al. (1993). Incorporation of atmospheric 15NO2-nitrogen into free amino acids by Norway spruce Picea abies (L.) Karst. Oecologia 94, 408–414. doi: 10.1007/BF00317117
Okamoto, M., Vidmar, J. J., and Glass, A. D. (2003). Regulation of NRT1 and NRT2 gene families of Arabidopsis thaliana: responses to nitrate provision. Plant Cell Physiol. 44, 304–317. doi: 10.1093/pcp/pcg036
Okano, K., Totsuka, T., Fukuzawa, T., and Tazakl, T. (1985). Growth responses of plants to various concentrations of nitrogen dioxide. Environ. Pollut. 38, 361–373. doi: 10.1016/0143-1471(85)90107-2
Ono, F., Frommer, W. B., and von Wire'n, N. (2000). Coordinated diurnal regulation of low- and high-affinity nitrate transporters in tomato. Plant Biol. 2, 17–23. doi: 10.1055/s-2000-297
Orebamjo, T. O., and Stewart, G. R. (1975). Ammonium inactivation of nitrate reductase in Lemna minor L. Planta 122, 37–44. doi: 10.1007/BF00385402
Orsel, M., Eulenburg, K., Krapp, A., and Daniel-Vedele, F. (2004). Disruption of the nitrate transporter genes AtNRT2.1 and AtNRT2.2 restricts growth at low external nitrate concentration. Planta 219, 714–721. doi: 10.1007/s00425-004-1266-x
Orsel, M., Krapp, A., and Daniel-Vedele, F. (2002). Analysis of the NRT2 nitrate transporter family in Arabidopsis: structure and gene expression. Plant Physiol. 129, 886–896. doi: 10.1104/pp.005280
Padidam, M., Venkateswarlu, K., and Johri, M. M. (1991). Ammonium represses NADPH nitrate reductase in the moss Funaria hygrometrica. Plant Sci. 75, 185–194. doi: 10.1016/0168-9452(91)90233-X
Popova, O. V., Dietz, K. J., and Golldack, D. (2003). Salt-dependent expression of a nitrate transporter and two amino acid transporter genes in Mesembryanthemum crystallinum. Plant Mol. Biol. 52, 569–578. doi: 10.1023/A:1024802101057
Qiao, Z., and Murray, F. (1997). Effects of atmospheric nitrogen dioxide on uptake and assimilation of ammonium in soybean plants. J. Plant Nutr. 20, 1183–1190. doi: 10.1080/01904169709365326
Qiao, Z., and Murray, F. (1998). The effects of NO2 on the uptake and assimilation of nitrate by soybean plants. Environ. Exp. Bot. 10, 33–40. doi: 10.1016/S0098-8472(97)00023-3
Quaggiotti, S., Ruperti, B., Borsa, P., Destro, T., and Malagoli, M. (2003). Expression of a putative high-affinity NO−3 transporter and of an H+-ATPase in relation to whole plant nitrate transport physiology in two maize genotypes differently responsive to low nitrogen availability. J. Exp. Bot. 54, 1023–1031. doi: 10.1093/jxb/erg106
Raven, J. A. (1988). Acquisition of nitrogen by the shoots of land plants: its occurrence and implication for acid-base regulation. New Phytol. 109, 1–20. doi: 10.1111/j.1469-8137.1988.tb00212.x
Sabaratnam, S., and Gupat, G. (1988). Effects of nitrogen dioxide on biochemical and physiological characteristics of soybean. Environ. Pollut. 55, 149–158. doi: 10.1016/0269-7491(88)90125-X
Sattelmacher, B. (2001). The apoplast and its significance for plant mineral nutrition. New Phytol. 149, 167–192. doi: 10.1046/j.1469-8137.2001.00034.x
Savchenko, G., Wiese, C., Neimanis, S., Hedrich, R., and Heber, U. (2000). pH regulation in apoplastic and cytoplasmic cell compartments of leaves. Planta 211, 246–255. doi: 10.1007/s004250000280
Scheible, W. R., Gonzalez-Fontes, A., Lauerer, M., Muller-Rober, B., Caboche, M., and Stitt, M. (1997). Nitrate acts as a signal to induce organic acid metabolism and repress starch metabolism in tobacco. Plant Cell 9, 783–798. doi: 10.1105/tpc.9.5.783
Schiffgens-Gruber, A., and Lutz, C. (1992). Ultrastructure of mesophyll cell chloroplasts of spruce needles exposed to O3, SO2 and NO2 alone and in combination. Environ. Exp. Bot. 32, 243–254. doi: 10.1016/0098-8472(92)90007-O
Schiltz, S., Munier-Jolain, N., Jeudy, C., Burstin, J., and Salon, C. (2005). Dynamics of exogenous nitrogen partitioning and nitrogen remobilization from vegetative organs in pea revealed by 15N in vivo labeling throughout seed filling. Plant Physiol. 137, 1463–1473. doi: 10.1104/pp.104.056713
Schmutz, P., Tarjan, D., Günthardt-Goerg, M. S., Matyssek, R., and Bucher, J. B. (1995). Nitrogen dioxide- a gaseous fertilizer of Poplar trees. Phyton 35, 219–232.
Schumaker, K. S., and Sze, H. (1987). Decrease of pH gradients in tonoplast vesicles by NO−3 and Cl−: evidence for H+-coupled anion transport. Plant Physiol. 83, 490–496. doi: 10.1104/pp.83.3.490
Shimazaki, K., Yu, S. W., Sakaki, T., and Tanaka, K. (1992). Differences between spinach and kidney bean plants in terms of sensitivity to fumigation with NO2. Plant Cell Physiol. 33, 267–273.
Siegwolf, R. T. W., Matyssek, R., Saurer, M., Maurer, S., Günthardt-Goerg, M. S., Schmutz, P., et al. (2001). Stable isotope analysis reveals differential effects of soil nitrogen and nitrogen dioxide on the water use efficiency in hybrid poplar leaves. New Phytol. 149, 233–246. doi: 10.1046/j.1469-8137.2001.00032.x
Sparks, J. P., Monson, R. K., Sparks, K. L., and Lerdau, M. (2001). Leaf uptake of nitrogen dioxide (NO2) in a tropical wet forest: implications for tropospheric chemistry. Oecologia 127, 214–221. doi: 10.1007/s004420000594
Speer, M., and Kaiser, W. M. (1991). Ion relations of symplastic and apoplastic space in leaves from Spinacia oleracea L. and Pisum sativum L. under salinity. Plant Physiol. 97, 990–997. doi: 10.1104/pp.97.3.990
Srivastava, H. S., Jolliffe, P. A., and Runeckles, V. C. (1974). Inhibition of gas exchange in bean leaves by NO2. Can. J. Bot. 53, 466–474. doi: 10.1139/b75-057
Stiegler, J. C., Richardson, M. D., and Karcher, D. E. (2011). Foliar nitrogen uptake following urea application to putting green turfgrass species. Crop Sci. 51, 1253–1260. doi: 10.2135/cropsci2010.06.0377
Stulen, I., Perez-Soba, M., De Kok, L. J., and Van Der Eerden, L. (1998). Impact of gaseous nitrogen deposition on plant functioning. New Phytol. 139, 61–70 doi: 10.1046/j.1469-8137.1998.00179.x
Takagi, M., and Gyokusen, K. (2004). Light and atmospheric pollution affect photosynthesis of street trees in urban environments. Urban For. Urban Green. 2, 167–171. doi: 10.1078/1618-8667-00033
Takahashi, M., Furuhashi, T., Ishikawa, N., Horiguchi, G., Sakamoto, A., Tsukaya, H., et al. (2014). Nitrogen dioxide regulates organ growth by controlling cell proliferation and enlargement in Arabidopsis. New Phytol. 201, 1304–1315. doi: 10.1111/nph.12609
Takahashi, M., Sakamoto, A., Ezura, H., and Morikawa, H. (2011). Prolonged exposure to atmospheric nitrogen dioxide increases fruit yield of tomato plants. Plant Biotechnol. 8, 485–487. doi: 10.5511/plantbiotechnology.11.0819a
Takeuchi, Y. C., Nihira, J., Kondo, N., and Tezuka, T. (1985). Change in Nitrate-reducing activity in squash seedlings with NO2 fumigation. Plant Cell Physiol. 26, 1027-1035.
Touraine, B., Grignon, N., and Grignon, C. (1988). Charge balance in NO–3 fed soybean: estimation of K+ and carboxylate recirculation. Plant Physiol. 88, 605–612. doi: 10.1104/pp.88.3.605
Touraine, B., Muller, B., and Grignon, C. (1992). Effect of phloem-translocated malate on NO−3 uptake by roots of intact soybean plants. Plant Physiol. 99, 1118–1123. doi: 10.1104/pp.99.3.1118
Uscola, M., Villar-Salvador, P., Oliet, J., and Warren, C. (2014). Foliar absorption and root translocation of nitrogen from different chemical forms in seedlings of two Mediterranean trees. Environ. Exp. Bot. 104, 34–43. doi: 10.1016/j.envexpbot.2014.03.004
Vidmar, J. J., Zhuo, D., Siddiqi, M. Y., Schjoerring, J. K., Touraine, B., and Glass, A. D. M. (2000). Regulation of high-affinity nitrate transporter genes and high-affinity nitrate influx by nitrogen pools in roots of barley. Plant Physiol. 123, 307–318. doi: 10.1104/pp.123.1.307
Wang, R. C., Okamoto, M., Xing, X., and Crawford, N. M. (2003). Microarray analysis of the nitrate response in Arabidopsis roots and shoots reveals over 1000 rapidly responding genes and new linkages to glucose, trehalose-6-phosphate, iron, and sulfate metabolism. Plant Physiol. 132, 556–567. doi: 10.1104/pp.103.021253
Wardlaw, I. F. (2005). Viewpoint: consideration of apoplastic water in plant organs: a reminder. Funct. Plant Biol. 32, 561–569. doi: 10.1071/FP04127
Weber, P., Nussbaum, S., Fuhrer, J., Gfeller, H., Schlunegger, U. P., Brunold, C., et al. (1995). Uptake of atmospheric 15NO2 and its incorporation into free amino acids in wheat (Triticum aestivum L.). Physiol. Plant 94, 71–77. doi: 10.1111/j.1399-3054.1995.tb00786.x
Wellburn, A. R. (1990). Why are atmospheric oxides of nitrogen usually phytotoxic and not alternative fertilizers? New Phytol. 115, 395–429. doi: 10.1111/j.1469-8137.1990.tb00467.x
Wellburn, A. R., Higginson, C., Robinson, D., and Walmsley, C. (1981). Biochemical explanations of more than additive inhibitory effects of low atmospheric levels of sulphur dioxide plus nitrogen dioxide upon plants. New Phytol. 88, 223–237. doi: 10.1111/j.1469-8137.1981.tb01719.x
Wilkinson, J. Q., and Crawford, N. M. (1993). Identification and characterization of a chlorate-resistant mutant of Arabidopsis thaliana with mutations in both nitrate reductase structural genes NIA1 and NIA2. Mol. Gen. Genet. 239, 289–297.
Wippel, K., Wittek, A., Hedrich, R., and Sauer, N. (2010). Inverse pH regulation of plant and fungal sucrose transporters: a mechanism to regulate competition for sucrose at the host/pathogen interface? PLoS ONE 5:e12429. doi: 10.1371/journal.pone.0012429
Yoneyama, T., and Sasakawa, H. (1979). Transformation of atmospheric NO2 absorbed in spinach leaves. Plant Cell Physiol. 20, 263-266.
Yoneyama, T., Sasakawa, H., Ishizuka, S., and Totsuka, T. (1979). Absorption of atmospheric NO2 by plants and soils. Soil Sci. Plant Nutr. 25, 267–275. doi: 10.1080/00380768.1979.10433167
Keywords: chloride channel gene, nitrate reductase, nitrate transporter, nitrogen dioxide, signal transmission
Citation: Hu Y, Fernández V and Ma L (2014) Nitrate transporters in leaves and their potential roles in foliar uptake of nitrogen dioxide. Front. Plant Sci. 5:360. doi: 10.3389/fpls.2014.00360
Received: 28 March 2014; Accepted: 04 July 2014;
Published online: 30 July 2014.
Edited by:
Ebrahim Hadavi, Islamic Azad University, IranReviewed by:
Abdullahil Baque, Sher-e-Bangla Agricultural University, BangladeshBrian Grout, University of Copenhagen, Denmark
Copyright © 2014 Hu, Fernández and Ma. This is an open-access article distributed under the terms of the Creative Commons Attribution License (CC BY). The use, distribution or reproduction in other forums is permitted, provided the original author(s) or licensor are credited and that the original publication in this journal is cited, in accordance with accepted academic practice. No use, distribution or reproduction is permitted which does not comply with these terms.
*Correspondence: Yanbo Hu and Ling Ma, Northeast Forestry University, 26 Hexing Road, Xiangfang District, Harbin 150040, P. R. China e-mail: huybnefu@yahoo.com
†This paper was dedicated to Professor Hartmut K. Lichtenthaler on the occasion of his 80th birthday.