- Department of Molecular Genetics and Microbiology, Faculty of Biological Sciences, FONDAP Center for Genome Regulation, Millennium Nucleus Center for Plant Functional Genomics, Pontifical Catholic University of Chile, Santiago, Chile
Nitrogen (N) is an essential macronutrient for plant growth and development. Plants adapt to changes in N availability partly by changes in global gene expression. We integrated publicly available root microarray data under contrasting nitrate conditions to identify new genes and functions important for adaptive nitrate responses in Arabidopsis thaliana roots. Overall, more than 2000 genes exhibited changes in expression in response to nitrate treatments in Arabidopsis thaliana root organs. Global regulation of gene expression by nitrate depends largely on the experimental context. However, despite significant differences from experiment to experiment in the identity of regulated genes, there is a robust nitrate response of specific biological functions. Integrative gene network analysis uncovered relationships between nitrate-responsive genes and 11 highly co-expressed gene clusters (modules). Four of these gene network modules have robust nitrate responsive functions such as transport, signaling, and metabolism. Network analysis hypothesized G2-like transcription factors are key regulatory factors controlling transport and signaling functions. Our meta-analysis highlights the role of biological processes not studied before in the context of the nitrate response such as root hair development and provides testable hypothesis to advance our understanding of nitrate responses in plants.
Introduction
Nitrogen (N) is a constituent of nucleic acids, amino acids, chlorophyll, and phytohormones among many other biomolecules. N is quantitatively the most abundant element in plant tissues after C, H, and O, representing up to 5% of dry weight (Miller and Cramer, 2005). However, biologically available N is often in short supply in natural as well as agricultural systems, limiting plant growth and development. Understanding the molecular mechanisms underlying plant N responses is an important issue for plant biology as well as agriculture. Transcriptomics approaches have been used extensively to investigate these mechanisms. Transcriptomic studies have evaluated different aspects of the plant nitrogen response, such as the effect of N source (e.g., Wang et al., 2007; Patterson et al., 2010), time after N treatments (e.g., Krouk et al., 2010a), N concentration (e.g., Wang et al., 2007), tissue type (e.g., Wang et al., 2003), and cell type (e.g., Gifford et al., 2008). These studies have identified a myriad of genes and functions modulated by N nutrient/metabolites such as uptake, transport and metabolism (reviewed in Vidal and Gutiérrez, 2008; Alvarez et al., 2012).
The nitrate signaling pathway is still poorly understood with only a handful regulatory factors modulating gene expression at different levels identified. Plants perceive changes in the concentration of nitrate by specific receptors. It has been established that the dual-affinity nitrate transporter NRT1.1 is able to function as nitrate sensor in Arabidopsis thaliana (Ho et al., 2009). Additional regulatory factors include protein kinases from the CIPK family. For example, loss of function of CIPK8 gene reduces by 40–65% the expression of several primary response genes such as NRT1.1, NIA1, or GLN2 (Hu et al., 2009). Another level of control of gene expression by nitrate is carried out by transcription factors. Only a few transcription factors directly implicated in regulating nitrate responses have been characterized to date (Gutiérrez, 2012). Some of these transcription factors are important for the control of nitrate assimilation, while others have been involved in modulation of root system architecture. The first transcription factor identified in the N response was a MADS box transcription factor called ANR1 (Zhang et al., 1999). ANR1 is involved in the control of lateral root growth in response to localized nitrate supply. Recent studies indicate NLPs are also important transcription factors in the nitrate response because they regulate many known nitrate signaling and assimilation genes (Konishi and Yanagisawa, 2013; Marchive et al., 2013). Additional transcription factors known to regulate N responses include members of the LBD family (Rubin et al., 2009); HY5, which is related to phytochrome-mediated effects on enzymes involved in nitrogen assimilation (Lillo, 2008); and a nitrate-induced NIGT1 member of the GARP family, which has been suggested to be involved in the control of nitrate utilization (Sawaki et al., 2013). Recently, NAC4 was found to be a key regulatory element controlling a nitrate-responsive network and lateral root development in Arabidopsis (Vidal et al., 2013). However, nitrate regulation of other important features of root system architecture such as primary root growth and root hair development have not yet been explored in depth (Forde and Walch-Liu, 2009). Several studies reported root hairs are important for nutrient uptake, including nitrogen uptake (Libault et al., 2010). Wang et al. (2002) observed root hair growth in response to nitrate treatment in rice. In the case of other macronutrients, such as phosphate, many genes involved in root morphological response to nutrient availability (Niu et al., 2013), including root hair responses (Lin et al., 2011a,b), have been identified. However, the role of root hairs and root hair development in nitrate responses has not been addressed.
The development of high-throughput technologies enabled identification of more than 2000 genes with changes in gene expression in response to various N treatments (Krouk et al., 2010b). However, functional characterization of these genes is long and laborious and lags far behind global gene expression studies. In order to aid prioritizing, we integrated publicly available root nitrate transcriptome data obtained under various experimental conditions. The analysis presented here (i) identifies the most consistent genes and biological functions regulated in response to nitrate treatments across multiple experiments (ii) highlights important biological process associated with nitrate root responses that have not been previously addressed and (iii) proposes key regulatory factors controlling major gene network modules. Our integrated systems analysis provides concrete testable hypothesis to advance our understanding of plant root nitrate responses.
Methods
Microarray Data Collection and Preprocessing
Microarray data used in this work was obtained from experiments published in: (Wang et al., 2003, 2004, 2007; Gutiérrez et al., 2007a; Gifford et al., 2008; Hu et al., 2009; Krouk et al., 2010a; Patterson et al., 2010; Ruffel et al., 2011; Vidal et al., 2013 and Álvarez et al., submitted.) CEL files of these experiments are available in the public microarrays databases GEO, EBI, or NASC. Accession numbers for each data set are indicated in Table 1. Background correction and normalization of the raw data sets was performed using Robust MultiChip Analysis (RMA) implemented in “affy” R package (Gautier et al., 2004).
Identification of Differentially Expressed Genes
The non-parametric RankProduct method (Breitling et al., 2004) was used to identify differentially expressed genes between treatment and control conditions (potassium nitrate vs. potassium chloride), as this method has been shown to be robust in analyzing microarray data that comprise small numbers of replicates (Hong and Breitling, 2008). A gene was considered statistically significant if its false discovery rate (FDR) adjusted p-value was equal or smaller than 0.05.
Network Construction and Coexpression Module Detection
A weighted gene coexpression network was constructed using the WGCNA R package version 1.27.1 (Langfelder and Horvath, 2008) with differentially expressed genes. First, the Pearson correlation matrix was weighted by raising it to a power (β). To choose the appropriate power, the network topology for various soft-thresholding powers was evaluated using pickSoftThreshold function and β = 7 was chosen because this ensured an approximate scale-free topology of the resulting network as previously described (Zhang and Horvath, 2005). Next, the pairwise measure of gene coexpression of the resulting weighted network was transformed into a topological overlap (TO) similarity measure, which is a robust measure of pairwise interconnectedness (Yip and Horvath, 2007). A TO similarity measure between two genes (ij) is defined as: where ki = ∑uaiu was the node connectivity and a is the network adjacency. Finally, TO similarity measure coupled with average linkage hierarchical clustering was performed for module detection using the Dynamic Tree Cut algorithm (Langfelder et al., 2008). The coexpression network was visualized using Cytoscape v 3.0 (File S1) and analyzed using the NetworkAnalyser plugin (Doncheva et al., 2012). In order to simplify the display of the network and to focus on relevant relationships, only edges in this network of the corresponding TO similarity measure above a threshold of 0.10 are shown in Figure 3. Further, NetworkAnalyser plugin was used to assess which genes in the network form hubs. VirtualPlant platform (Katari et al., 2010) was used to generate a subnetwork of transcription factors and putative targets taking into account the TO similarity measure and over-represented transcription factors binding sites.
Functional Enrichment Analysis
Fisher's exact test was performed for declaring a GO (Gene Ontology) category as significantly over-represented (Benjamini–Yekutieli method for controlling FDR, adjusted p-value < 0.05) using PlantGSEA toolkit (Yi et al., 2013). GO level was defined as the number of edges in the shortest path connecting a node to the root node, this information was calculated using SQL queries on the GO database. To determine protein sequence similarity for genes associated to GO terms, we performed pair-wise BLASTP sequence comparisons. We only analyzed alignments with an E-value smaller or equal than 10−10. To compare the results we used protein sequence similarity scores normalized by query length.
Results and Discussion
Members of G2-Like and LBD Transcription Factor Families are the Most Consistently Regulated Genes in Response to Nitrate Treatments
Meta-analysis of microarray data has been used to define robust sets of regulated genes (e.g., Gutiérrez et al., 2007b; Bhargava et al., 2013). To gain new insights into the plant N response we carried out a meta-analysis of transcriptome data based on the following criteria: First, we focused on experiments performed with nitrate as the only experimental factor. Nitrate is one of most important nitrogen sources in agricultural soils (Glass, 2009) and it can act as a signal to regulate global gene expression as well as different aspects of plant growth and development (Wang et al., 2004). Second, we focused on transcriptome studies carried out in root organs because it is the first organ encountering nitrate and initiates plant responses (Wang et al., 2003) and it is the organ for which more data sets are available. Finally, we focused on studies with wild-type genetic background and Affymetrix microarray platform to avoid cross-platform variation as well as unbalanced data sets. Using these criteria, 27 experimental datasets corresponding to 131 ATH1 Affymetrix genechip hybridizations were selected for further analysis (Table 1). To focus on relevant N-responsive genes, RMA normalization (Irizarry et al., 2003) was performed and Rank Products (Breitling et al., 2004) was used to identify genes that exhibited expression differences between nitrate treatment and control (KCl) samples in each experiment. A total of 2286 genes were identified as differentially expressed in at least one experiment, with a maximum FDR of 5% (Table S1). Next, we ranked differentially expressed genes by the number of experiments in which they were regulated. The top 50 genes according to this criterion are shown in Table 2. No gene was differentially expressed in response to nitrate treatments in all experiments. The expression of all genes in Table 2 was induced by nitrate treatments in at least 40% of the experiments analyzed, with a maximum of 75%. The majority of these core nitrate-response genes were upregulated. Starvation pre-treatment causes a decrease in expression levels of many genes related to nitrate assimilation, signaling, and transport. An increase in the expression of many genes is therefore expected after the nitrate treatment. Interestingly, the top 5 most consistent genes found in our analysis code for transcription factors. HRS1 (AT1G13300), a G2-like transcription factor, was statistically induced in 20 out of 27 experiments (Table 2). HRS1 is expressed in root hairs and is induced under low phosphate concentration (Liu et al., 2009). HRS1 may be involved in modulation of primary root and root hair growth in response to phosphate starvation (Liu et al., 2009). Our results suggest that in addition to phosphate, HRS1 is a regulator of root growth in response to nitrogen availability. Three other members of this transcription factor family respond to nitrate in multiple experiments: AT3G25790, AT1G68670, and AT1G25550. AT1G68670 is a direct target of SHORT ROOT (SHR) (Cui et al., 2011), a key regulator of root growth and development in Arabidopsis. Moreover, SHR regulates cytokinin homeostasis by directly controlling transcription of CYTOKININ OXIDASE 3 gene (Cui et al., 2011). Several studies indicate nitrate induces the expression of cytokinin biosynthesis genes resulting in cytokinin accumulation in response to nitrate (Kiba et al., 2011). These observations suggest G2-like transcription factor (AT1G68670) is part of the SHR regulatory network modulating root development and cytokinin levels. The second G2-like family gene AT1G25550, was found to bind the E2Fa promoter. E2Fa is an essential transcription factor in A. thaliana that regulates asymmetric cell division marking lateral root initiation (Berckmans et al., 2011). These findings suggest that G2-like transcription factors may be important regulators of root morphology in response to nitrate availability. Other transcription factors that respond to nitrate in a large number of experiments (70%) are LBD37 and LBD39. Transcription factors of the LBD family have been shown to have important roles in regulation of anthocyanin synthesis and nitrogen metabolism by repressing transcripts that are critical for nitrate transport and assimilation (Rubin et al., 2009). Additional transcription factors involved in Arabidopsis nitrate root responses include other previously characterized NAC and NLP transcription factors as well as a list of new candidates. Figure 1 shows the most represented transcription factor families in decreasing order. The most represented families are ERF/AP2 (31) and WRKY (15) transcription factors, with an overrepresentation of nitrate responsive genes as expected from their gene family size. These two transcription factor families are involved in plant development and responses to biotic and abiotic stress (Rushton et al., 2010; Mizoi et al., 2012). However, their role in nitrate responses is not clear yet. Another family of transcription factors with many members are MYB-type transcription factors (22). MYB75 is known for its role in response to nitrogen limitation (Lea et al., 2007). In other plant species, MYB proteins are known to control expression of genes coding for important nitrogen assimilation enzymes, such as glutamine synthetase (Gómez-Maldonado et al., 2004) and asparagine synthetase (Canales et al., 2012). These results point toward specific transcription factors as candidate regulators controlling hallmark responses to nitrate treatments such as metabolism and root system architecture.
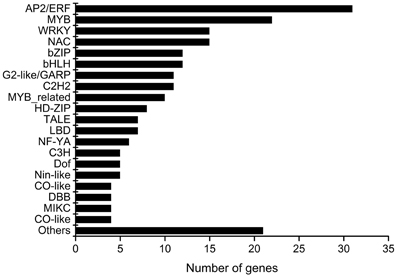
Figure 1. Distribution of the 219 nitrate-responsive transcription factors according to family. Transcription factors were classify following PlantTFDB2.0 database annotation (Zhang et al., 2011).
Nitrate Regulates a Core Set of Biological Functions Regardless of the Experimental Context
As shown in Figure 2A, ~60% of differentially expressed genes were regulated by nitrate in only one experiment, consistent with the idea that nitrate regulation of gene expression depends largely on the experimental context (Gutiérrez et al., 2007b; Krouk et al., 2010b). However, analysis of regulated biological functions showed that responses at the functional level are more robust from experiment to experiment as compared to genes (Figure 2B). The average number of genes shared between any two experiments is 6.7%, while 19.5% of overrepresented GO terms (FDR < 0.05) are shared in the same two experiments (Figure 2B). This difference in the percentage of shared genes vs. GO terms increases with the number of experiments compared. For example, the number of GO terms shared between any five experiments is 10 times higher than the number of shared genes. To evaluate whether this difference between intersection of genes and GO terms was biological or an artifact due to the nature of the gene to GO association data we carried out three control experiments: (1) Conservation of GO terms could be explained by genes annotated to very general GO term categories increasing the chance of intersection at the GO term level. To address this potential issue, we compared the distribution of total and shared over-represented GO terms between any combination of two experiments. As shown in Figure S1A, the distribution of levels is similar for total and shared GO terms indicating differentially expressed genes are not biased toward general GO term categories. (2) Nitrate-responsive genes identified here may have many GO annotations, thus, explaining increased overlap in GO terms. To rule out this possibility, we analyzed the number of GO terms associated with the list of 2286 nitrate responsive genes used in this study and compared with the GO annotations found in 1000 lists of 2286 randomly selected genes represented in the ATH1 Affymetrix Gene Chip. As shown in Figure S1B, the average number of GO terms and their distribution are very similar in both cases. This result indicates nitrate responsive genes have no more annotations than a random set of genes of the same size. (3) If the genes are shared when comparing experiments the GO terms will be shared as well. However, GO terms shared between any two experiments contained on average only 22.4% of the same genes (Figure S2). This result indicates most of the genes contributing to over-represented GO terms are different in each experiment.
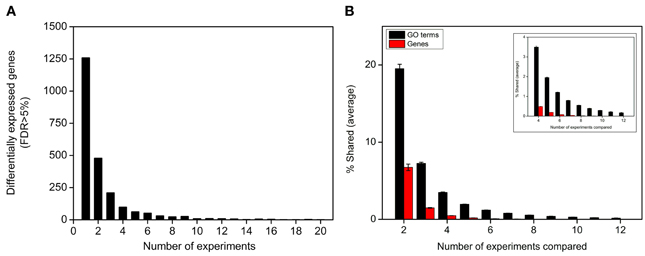
Figure 2. Biological functions are more robust than gene identities in the nitrate response. (A) Histogram of 2286 significantly nitrate responsive genes (rankproduct, FDR < 5%) vs. the number of experiments in which were regulated. (B) Histogram of the average percentage of elements (over-represented GO terms or genes) shared between different number of experiments. Inset shows the same graph but starting from 4 experiments.
A prediction of our hypothesis is that different members of the same gene family should be found contributing to shared GO terms between different experiments. To systematically test this hypothesis, we performed pair-wise comparisons of protein sequences for all genes annotated to shared GO terms between any two experiments. We then compared the distribution of protein sequence similarities for all pair-wise comparisons between genes contributing to a shared GO term in our data set vs. all genes annotated to that GO term. We found shared GO terms (74.5%) have more pairs of similar protein sequences coming from different experiments than expected by chance (α < 0.05). For example, NRT2.2 gene was found differentially expressed in experiment 21 and NRT2.5 was found differentially expressed in experiment 23. Both NRT2.2 and NRT2.5 are annotated to the shared GO term “Transport (GO:0006810).” Similarly, the shared GO term “Response to Carbohydrate (GO:0009743)” contains GLN1;2 and GLN1;1, each regulated in different experiments.
The easiest interpretation of these results is that nitrate responses at the biological function level are more robust to experimental context than genes. This phenomenon could be explained by functional redundancy of different genetic components, a feature that is common to biological networks and has been proposed as a mechanism for robustness toward stochastic fluctuations (Whitacre, 2012). A similar idea is the degeneracy concept proposed by Edelman and Gally (2001), which defines the property whereby structurally different elements may perform the same or similar functions. This feature has been attributed not only to gene networks but also to neural networks and evolution (Edelman and Gally, 2001). This phenomenon may be particularly relevant in plants, where increased gene family sizes may provide higher adaptive capacity to environmental perturbations.
Robust Nitrate Responsive Biological Processes Highlight New Nitrate Controlled Developmental Processes
Which biological functions are most relevant for nitrate responses in roots? To answer this question, we ranked GO terms by the number of experiments in which they were present based on regulated genes with the corresponding annotation. In order to focus on specific functions, we only considered GO terms at level 7 and 8 and removed redundant terms using the REVIGO tool (Supek et al., 2011). Table 3 shows the list of the most consistent biological functions. In contrast to genes, the most consistent GO terms appear regulated in ~90% of the experiments analyzed. The most consistent biological functions are those related to nitrate transport and carbon metabolism. We also found categories associated with root morphogenesis that have not been studied in the context of nitrate responses such as trichoblast differentiation (GO:0010054, 64 genes). Trichoblasts are a subset of specialized epidermal cells from which root hairs emerge. These specialized cells play an important role in the uptake of water and nutrients by increasing root absorption surface (Gilroy and Jones, 2001). Phytohormones are important regulators of root hair growth and development. It has been reported that auxin and ethylene promote root hair elongation and growth (Muday et al., 2012). Interestingly, biological processes that are statistically enriched in response to nitrate (Table S2) include auxin biosynthesis (GO:0009851, 32 genes, p-value = 1.25 × 10−7). Genes associated with this function include several genes from the tryptophan-dependent auxin biosynthetic pathway (Mano and Nemoto, 2012) such as TAR2 (Stepanova et al., 2008), CYP79B2, and CYP79B3 (Zhao et al., 2002). Nitrate can also regulate the expression of several auxin-signaling genes including the AFB3 auxin receptor (Vidal et al., 2010, 2013). It has been recently reported that nitrogen and small GTPase proteins act synergistically to regulate root hair growth in Arabidopsis (Bloch et al., 2011). In addition, it is known that auxin signaling pathway controls root hair growth (Lee and Cho, 2013). Based on these observations, we hypothesize that nitrate modulates root hair development by modulating auxin biosynthesis and signaling.
Weighted Correlation Gene Networks Predict Functional Modules in Response to Nitrate
To analyze functional relationships among the 2286 nitrate responsive genes identified above we inferred a network as described previously (Langfelder and Horvath, 2008). In our analysis, 11 coexpression modules were identified and functional analysis indicated 9 out of the 11 modules had overrepresented biological functions. Interestingly, the gene network modules identified include robust functions of the nitrate response such as ion transport, carbon metabolism, response to chemical stimulus and trichoblast differentiation (Figure 3). The most consistent biological functions found associated to the nitrate response (Table 3) metal ion transport, monocarboxylic acid metabolic process, nitrate transport, glucose catabolic process or regulation of transcription are also overrepresented in these modules. Below we describe in more detail gene network modules 1, 6, 7, 8, and 9 containing these functions.
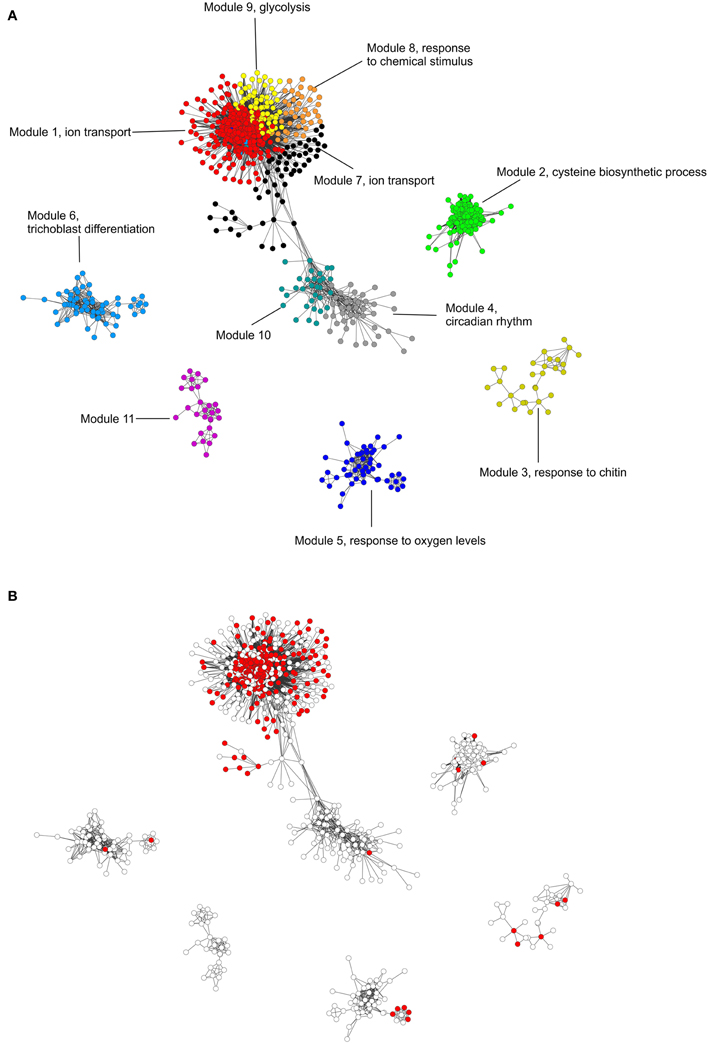
Figure 3. Nitrate-responsive gene coexpression network. (A) Colors are used to distinguish each gene network module. The most over-represented and consistent biological process GO terms (appear in at least 14 different experiments) are indicated in each module (File S2). (B) Red nodes indicate genes that respond similarly to nitrate treatments in NR-null mutants and wild-type plants indicating they respond directly to a nitrate signal (Wang et al., 2004). X and Y axes does not represent any particular scale (Table S3).
Module 1 is the largest module in the nitrate-responsive gene coexpression network, in terms of number of genes and number of connections. The top categories in this module are anion transport (GO:0006820, p-value = 2.60 × 10−18) and response to nitrate (GO:0010167, p-value = 7.75 × 10−17). Other biological functions involved in the nitrate response were also overrepresented, such as signaling (GO:0023052, p-value = 1.42 × 10−7) and regulation of transcription (GO:0006355, p-value = 8.54 × 10−6). Within the latter function, we found several families of transcription factors that were enriched, such as the G2-like or LBD discussed above.
Because hub genes play key functional roles in gene networks (Zotenko et al., 2008; Vidal et al., 2013), we identified those genes with the highest number of connections (degree) within each network module. The gene with highest degree in module 1 is an unknown HPP family protein (AT3G47980, pfam04982). HPP proteins are integral membrane proteins with four transmembrane helices. The identified protein has a predicted size of 27 kDa and a high pI (10.7) and based on his signal peptide sequence it is likely localized in the plastidic membrane. Another HPP family member (AT5G62720) has been identified in chloroplastidic membrane fractions by mass spectrometry (Ferro et al., 2010) and is also induced in response to nitrate. Using the eFP Browser (Winter et al., 2007), we found that the HPP genes are expressed in a tissue-specific manner (Figure S3). AT5G62720 expression is most abundant in photosynthetic tissues, while AT3G47980 is preferentially expressed in the roots suggesting the physiological roles of these two proteins may be different. Further research will be required to elucidate the role of HPP proteins in plants and their putative role in nitrate responses. However, because module 1 is enriched in transport functions, it is likely that highly connected genes such as the HPP genes identified may play a role in intracellular transport in the context of the nitrate response.
Enriched biological functions in module 7 are ion transport (GO:0006820, p-value = 1.73 × 10−3) and response to nitrate (GO:0010167, p-value = 2.46 × 10−3). These biological functions are also among the most enriched in gene network module 8. Genes of the CIPK family are among the most connected genes in both modules. CIPKs are Ser/Thr protein kinases that interact with calcineurin B-like calcium sensors and are involved environmental stress responses and nutrient sensing (Luan et al., 2009). Specifically, CIPK3 is the second most connected gene in module 8 and CIPK8 is the third most connected gene in module 7. CIPK8 plays a role in regulation of gene expression of primary nitrate response genes (Hu et al., 2009). On the other hand, CIPK3 has been widely analyzed in several experimental contexts, demonstrating its importance in plant development and adaptation to stress (Kim et al., 2003). However, its role in nitrate response has not yet been addressed. Interestingly, several protein phosphatases are also present in these modules, including PP2C (AT5G27930, AT5G26010, AT5G26010, AT3G16560, AT1G67820), dual phosphatase ATPFA-DSP1 (AT1G05000), AtMTM2 (AT5G04540), and PP2A (AT5G03470). With respect to the PP2A family, Heidari et al. (2011) showed that PP2A is required for the activation of nitrate reductase (NR). These results suggest the new kinases and protein phosphatases identified here may be important in phosphoproteome homeostasis for signaling and control of nitrogen responses in Arabidopsis roots.
Module 9 contains many overrepresented biological functions related to metabolism such as glycolysis (GO:0006096, p-value = 6.21 × 10−9), carboxylic acid metabolic process (GO:0019752, p-value = 1.12 × 10−4), hexose biosynthetic process (GO:0019319, p-value = 2.62 × 10−3), pyruvate metabolic process (GO:0006090, p-value = 5.85 × 10−3) and cellular nitrogen compound metabolic process (GO:0034641, p-value = 1.23 × 10−3). In addition, among the most connected genes we find several genes related to ammonium assimilation, such as glutamine synthetase (AT5G35630), glutamate synthase (AT5G53460), and isocitrate dehydrogenase (AT4G35260).
Finally, module 6 is a particularly interesting module in the context of nitrate responses because it is enriched in genes associated with trichoblast differentiation. Several genes within this module are essential for root hair development. One example is Arabidopsis EXPANSIN 7 (AtEXPA7) gene, which is expressed specifically in root hairs (Lin et al., 2011a,b; Lan et al., 2013). The reduction of mRNA levels of AtEXPA7 significantly affects root hair length (Lin et al., 2011a,b). Furthermore, it has been shown that this gene is able to complement a mutation in the rice OsEXPA17 gene, suggesting functional conservation of root hair expansins in monocots and dicots (ZhiMing et al., 2011). Module 6 also includes the AtXET14 gene, which encodes a xyloglucan endotransglucosylase enzyme implicated in root hair development (Maris et al., 2009). Adding purified recombinant AtXET14 protein to MS medium for 2 days decreases growth of initiated root hairs and reduced the root elongation zone. In addition, MRH6 and COBL9 genes were also found in this module. These two genes were identified in a screening for root hair morphology mutants (Jones et al., 2006). Finally, peroxidase and extensin genes were over-represented in this module. Hydrogen peroxide is involved in root hair development (Dunand et al., 2007) and peroxidases are proposed candidate genes involved in this developmental process (Kwasniewski et al., 2013).
Recently, an R2R3-MYB transcription factor was found to control development of root hairs in Arabidopsis (Slabaugh et al., 2011). We found five members of R2R3-MYB family (AT1G48000, AT5G14340, AT3G06490, AT5G60890, AT1G73410) within module 6. These genes belong to the only transcription factor family over-represented in the trichoblast differentiation network module, representing attractive candidate regulatory factors for root hair development in response to nitrate treatments in Arabidopsis. Figure 4 integrates these findings and proposes a simplified model for how nitrate may modulate root hair development. Nitrate is able to regulate expression of auxin biosynthesis genes. Auxin promotes initiation and growth of root hairs (Muday et al., 2012). It has been demonstrated that a significant amount of auxin is synthesized in the roots (Ljung et al., 2005), specifically in the apical region and CYP79B2 and CYP79B3 genes are involved in this localized auxin synthesis. Consistent with this model, we found nitrate induces expression of CYP79B3 in 4 independent experiments (Gifford et al., 2008; Ruffel et al., 2011; Vidal et al., 2013 Álvarez et al., submitted) and CYP79B2 in two different microarray experiments (Gifford et al., 2008; Vidal et al., 2013). Based on coexpression network analysis, we propose that these morphogenic changes would be mediated by cell wall proteins as extensins, expansins and peroxidases, which could be regulated by R2R3-MYB transcription factors (Figure 4B).
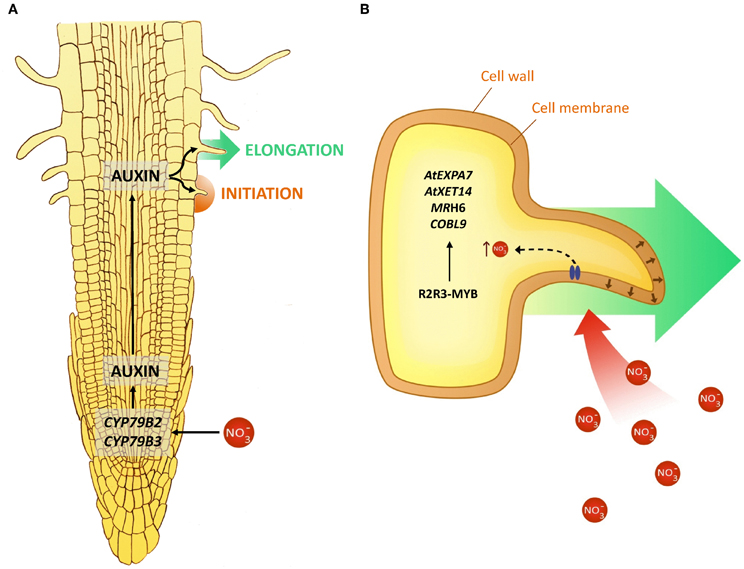
Figure 4. Proposed model of how nitrate modulates root hair development in Arabidopsis. (A) Nitrate treatments induce auxin biosynthesis and this phytohormone promotes root hair initiation and elongation. (B) Schematic detail of a root hair and genes involved in their growth mediated by nitrate.
Nitrate Signals and not Downstream Products of Nitrate Reduction Regulate Genes Found Mainly in Modules 1, 7, 8, and 9
Analysis of a NR-null mutant has shown that nitrate serves as a signal to control the expression of many genes in Arabidopsis (Wang et al., 2004). In order to distinguish nitrate-regulated modules vs. modules controlled by other N forms produced by nitrate reduction and assimilation, we integrated NR-null mutant transcriptome data (Wang et al., 2004) with our network analysis. As shown in Figure 3B, most of the nitrate-regulated genes are concentrated in central modules 1, 7, 8, and 9. Based on these results, transport, metabolism, and signaling biological functions represented in these network modules are robustly controlled by a nitrate signal. These results also suggest that biological functions such as circadian rhythms (module 4), response to oxygen levels (module 5), and trichoblast differentiation (module 6) are regulated by products of nitrate reduction. These results are consistent with previous findings such as the case of the master clock gene CCA1 previously found to be regulated by organic nitrogen signals (Gutiérrez et al., 2008).
Network Analysis Predicts Central Nitrate Response Modules are Controlled by bZIP and Myb Transcription Factors
To identify transcription factors that control essential and robust functions in the root nitrate response such as nitrate transport and assimilation, we focused in transcription factors from modules 1, 7, 8, and 9 and their possible targets. Figure 5 shows the subnetwork with edges between transcription factors and their putative targets taking into account the over-represented binding sites for the transcription factor in the promoter region of corresponding target genes using VirtualPlant software (Katari et al., 2010). In this network, MYB-related (AT5G58900) and bZIP (AT5G65210) genes showed the highest degree. Three different G2-like transcription factors (AT1G68670, AT1G25550, AT1G13300) were also found in top positions of the ranking of transcription factors with higher degree. As shown in Figure 5B, MYB-related gene coexpressed with nitrite reductase, 2-oxoglutarate/malate chloroplast transporter and a 6-phosphogluconate dehydrogenase gene from the oxidative pentose phosphate pathway. These results suggest this MYB-related factor controls basic aspects of nitrate metabolism, such as nitrate reduction, GS/GOGAT cycle and the generation of reducing equivalents.
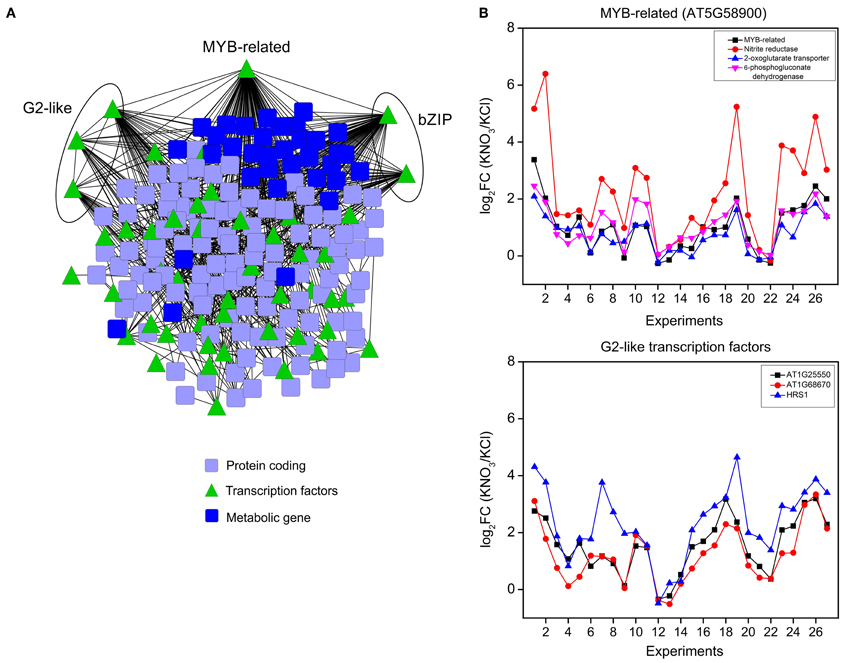
Figure 5. Transcriptional control of the central modules in the nitrate gene network. (A) A subnetwork of transcription factors and their potential targets derived from modules 1, 7, 8, and 9. Edges represent predicted regulatory interactions between transcription factors and target genes based on overrepresented transcription factors binding sites on the promoter of the targets. The most connected transcription factors are highlighted. (B) Expression patters of MYB-related transcription factor together with their three most correlated targets. Expression profiles of the three G2-like transcription factors are shown below.
A bZIP transcription factor identified as potential network driver (TGA1) belong to the subfamily TGA and another member of this family (TGA4) occupied the fifth position in the ranking of transcription factors with higher degree. These transcription factors have been implicated in bacterial defense responses. tga1/tga4 double mutant plants show a greater susceptibility to infection by Pseudomonas syringae (Shearer et al., 2012). However, our analysis suggests that these transcription factors (TGA1, TGA4) could be important in the nitrate response of Arabidopsis roots. TGA1 is the second gene of this subnetwork in terms of number of connections and it is differentially expressed in 9 different microarray experiments. TGA1 is highly coexpressed with metabolic genes such as urophorphyrin methylase 1 and phosphoglucose isomerase 1. In fact, the biological function overrepresented with a lower p-value among the possible targets of TGA1 is primary metabolic process (GO:0044238, p-value = 0.002). We have recently validated this hypothesis, demonstrating TGA1 and TGA4 transcription factors are important regulatory factors of the root response to nitrate treatments in Arabidopsis thaliana (Álvarez et al., submitted). Another transcription factor of the bZIP family implicated in N-responses is bZIP11. bZIP11 has been shown to regulate asparagine synthetase 1 and proline dehydrogenase 2 in Arabidopsis (Hanson et al., 2011).
G2-like transcription factors are members of the GARP superfamily and are characterized by a conserved domain (GARP) that is a single Myb-related DNA-binding domain (Sawaki et al., 2013). It is interesting to note that there are several connections between the three members of the G2-like transcription factors family, suggesting they respond to nitrate treatments in a coordinated fashion (Figure 5A). AT1G68670 is the G2-like transcription factor with the higher degree in this subnetwork and is coexpressed with other transcription factors such as another G2-like (Figure 5B), ZINC FINGER PROTEIN 4 (AT1G66140) and MYB-related (AT5G58900). Moreover, because several protein kinases such as MAPKKK16, WNK7, and CIPK1 are also present in this regulatory network, we hypothesize G2-like transcription factors are key regulatory genes involved in nitrate signaling leading to metabolic and developmental responses in Arabidopsis roots.
Conclusions
Integrated network analysis of transcriptome data provided novel hypothesis about functions and regulatory mechanisms by which Arabidopsis plants respond to nitrate. Our meta-analysis better assessed the nitrate functional space than any single or integrated transcriptome study previously published. We estimated the mean functional coverage of any single experiment at about 31%. This result highlights the need for integrated data analysis to better map the functional space for any given perturbation. Moreover it underscores the need for using experiments carried out under non-redundant environmental conditions.
Our Systems approach identified nitrate regulation of root hairs as an important component of the plant developmental response to changes in N nutrition, a yet unexplored research area at the intersection of N nutrition and root biology. We provided concrete hypothesis for genes and connections among genes related to root hair differentiation in response to nitrate that have not been previously highlighted nor addressed experimentally. Our results also highlight the role of bZIP and G2-like transcription factors for regulation of important functions related to nitrate transport and signaling. G2-like transcription factors have not been characterized in the context of nitrate responses. Functional studies of these new candidate genes should help better understand regulatory mechanisms underlying root nitrate responses in Arabidopsis and other plants.
Conflict of Interest Statement
The authors declare that the research was conducted in the absence of any commercial or financial relationships that could be construed as a potential conflict of interest.
Acknowledgments
This work was funded by Fondo Nacional de Desarrollo Científico y Tecnológico (1100698), the International Early Career Scientist program from the Howard Hughes Medical Institute (Award 55007421), Fondo de Desarrollo de Areas Prioritarias Center for Genome Regulation (Grant 1509007), Millennium Nucleus Center for Plant Functional Genomics (Grant P10-062-F). Javier Canales is supported by Fondo de Desarrollo Científico y Tecnológico postdoctoral fellowship (Grant 3130315). We would like to thank Dr. Elena Vidal for constructive discussions.
Supplementary Material
The Supplementary Material for this article can be found online at: http://www.frontiersin.org/journal/10.3389/fpls.2014.00022/abstract
Figure S1 | Controls performed to evaluate GO term robustness. (A) Distribution of total and shared over-represented GO term levels between any combination of two experiments. To generate this graph we analyzed all possible combinations of two experiments. Level 1 is the most general GO term category and level 11 the most specific. The x-axis corresponds to the depth of a concept while the y-axis shows average number of GO terms for a given level. (B) Number of GO associated with each 2286 nitrate responsive genes or with 2286 randomly selected genes (average of 1000 iterations).
Figure S2 | Histogram of the percentage of genes associated with the shared GO terms between any combination of two experiments.
Figure S3 | Gene expression patterns of HPP genes (AT3G47980 and AT5G62720) according to the Arabidopsis eFP browser.
File S1 | Cytoscape file of the nitrate-responsive gene coexpression network.
File S2 | Cytoscape file of the subnetwork present in Figure 5A.
Table S1 | List of 2286 nitrate-responsive genes.
Table S2 | Biological processes that are statistically enriched in response to nitrate.
Table S3 | List of genes that were commonly expressed in nitrate reductase-null mutant and wild type.
References
Alvarez, J. M., Vidal, E. A., and Gutiérrez, R. A. (2012). Integration of local and systemic signaling pathways for plant N responses. Curr. Opin. Plant Biol. 15, 185–191. doi: 10.1016/j.pbi.2012.03.009
Berckmans, B., Vassileva, V., Schmid, S. P., Maes, S., Parizot, B., Naramoto, S., et al. (2011). Auxin-dependent cell cycle reactivation through transcriptional regulation of Arabidopsis E2Fa by lateral organ boundary proteins. Plant Cell 23, 3671–3683. doi: 10.1105/tpc.111.088377
Bhargava, A., Clabaugh, I., To, J. P., Maxwell, B. B., Chiang, Y. H., Schaller, G. E., et al. (2013). Identification of cytokinin-responsive genes using microarray meta-analysis and RNA-Seq in Arabidopsis. Plant Physiol. 162, 272–294. doi: 10.1104/pp.113.217026
Bloch, D., Monshausen, G., Singer, M., Gilroy, S., and Yalovsky, S. (2011). Nitrogen source interacts with ROP signalling in root hair tip-growth. Plant Cell Environ. 34, 76–88. doi: 10.1111/j.1365-3040.2010.02227.x
Breitling, R., Armengaud, P., Amtmann, A., and Herzyk, P. (2004). Rank products: a simple, yet powerful, new method to detect differentially regulated genes in replicated microarray experiments. FEBS Lett. 573, 83–92. doi: 10.1016/j.febslet.2004.07.055
Canales, J., Rueda-López, M., Craven-Bartle, B., Avila, C., and Cánovas, F. M. (2012). Novel insights into regulation of asparagine synthetase in conifers. Front. Plant Sci. 3:100. doi: 10.3389/fpls.2012.00100
Cui, H., Hao, Y., Kovtun, M., Stolc, V., Deng, X. W., Sakakibara, H., et al. (2011). Genome-wide direct target analysis reveals a role for SHORT-ROOT in root vascular patterning through cytokinin homeostasis. Plant Physiol. 157, 1221–1231. doi: 10.1104/pp.111.183178
Doncheva, N. T., Assenov, Y., Domingues, F. S., and Albrecht, M. (2012). Topological analysis and interactive visualization of biological networks and protein structures. Nat. Protoc. 15, 670–685. doi: 10.1038/nprot.2012.004
Dunand, C., Crèvecoeur, M., and Penel, C. (2007). Distribution of superoxide and hydrogen peroxide in Arabidopsis root and their influence on root development: possible interaction with peroxidases. New Phytol. 174, 332–341. doi: 10.1111/j.1469-8137.2007.01995.x
Edelman, G. M., and Gally, J. A. (2001). Degeneracy and complexity in biological systems. Proc. Natl. Acad. Sci. U.S.A. 98, 13763–13768. doi: 10.1073/pnas.231499798
Ferro, M., Brugière, S., Salvi, D., Seigneurin-Berny, D., Court, M., Moyet, L., et al. (2010). AT_CHLORO, a comprehensive chloroplast proteome database with subplastidial localization and curated information on envelope proteins. Mol. Cell. Proteomics 9, 1063–1084. doi: 10.1074/mcp.M900325-MCP200
Forde, B. G., and Walch-Liu, P. (2009). Nitrate and glutamate as environmental cues for behavioural responses in plant roots. Plant Cell Environ. 32, 682–693. doi: 10.1111/j.1365-3040.2008.01927.x
Gautier, L., Cope, L., Bolstad, B. M., and Irizarry, R. A. (2004). affy—analysis of Affymetrix GeneChip data at the probe level. Bioinformatics 20, 307–315. doi: 10.1093/bioinformatics/btg405
Gifford, M. L., Dean, A., Gutierrez, R. A., Coruzzi, G. M., and Birnbaum, K. D. (2008). Cell-specific nitrogen responses mediate developmental plasticity. Proc. Natl. Acad. Sci. U.S.A. 105, 803–808. doi: 10.1073/pnas.0709559105
Gilroy, S., and Jones, D. L. (2001). Through form to function: root hair development and nutrient uptake. Trends Plant Sci. 5, 56–60. doi: 10.1016/S1360-1385(99)01551-4
Gómez-Maldonado, J., Avila, C., Torre, F., Cañas, R., Cánovas, F. M., and Campbell, M. M. (2004). Functional interactions between a glutamine synthetase promoter and MYB proteins. Plant J. 39, 513–526. doi: 10.1111/j.1365-313X.2004.02153.x
Gutiérrez, R. A. (2012). Systems biology for enhanced plant nitrogen nutrition. Science 336, 1673–1675. doi: 10.1126/science.1217620
Gutiérrez, R. A., Lejay, L. V., Dean, A., Chiaromonte, F., Shasha, D. E., and Coruzzi, G. M. (2007a). Qualitative network models and genome-wide expression data define carbon/nitrogen-responsive molecular machines in Arabidopsis. Genome Biol. 8:R7. doi: 10.1186/gb-2007-8-1-r7
Gutiérrez, R. A., Gifford, M. L., Poultney, C., Wang, R., Shasha, D. E., Coruzzi, G. M., et al. (2007b). Insights into the genomic nitrate response using genetics and the Sungear Software System. J. Exp. Bot. 58, 2359–2367. doi: 10.1093/jxb/erm079
Gutiérrez, R. A., Stokes, T. L., Thum, K., Xu, X., Obertello, M., Katari, M. S., et al. (2008). Systems approach identifies an organic nitrogen-responsive gene network that is regulated by the master clock control gene CCA1. Proc. Natl. Acad. Sci. U.S.A. 105, 4939–4944. doi: 10.1073/pnas.0800211105
Hanson, J., Hanssen, M., Wiese, A., Hendriks, M. M., and Smeekens, S. (2011). The sucrose regulated transcription factor bZIP11 affects amino acid metabolism by regulating the expression of ASPARAGINE SYNTHETASE1 and PROLINE DEHYDROGENASE2. Plant J. 53, 935–949. doi: 10.1111/j.1365-313X.2007.03385.x
Heidari, B., Matre, P., Nemie-Feyissa, D., Meyer, C., Rognli, O. A., Møller, S. G., et al. (2011). Protein phosphatase 2A B55 and A regulatory subunits interact with nitrate reductase and are essential for nitrate reductase activation. Plant Physiol. 156, 165–172. doi: 10.1104/pp.111.172734
Ho, C. H., Lin, S. H., Hu, H. C., and Tsay, Y. F. (2009). CHL1 functions as a nitrate sensor in plants. Cell 138, 1184–1194. doi: 10.1016/j.cell.2009.07.004
Hong, F., and Breitling, R. (2008). A comparison of meta-analysis methods for detecting differentially expressed genes in microarray experiments. Bioinformatics 24, 374–382. doi: 10.1093/bioinformatics/btm620
Hu, H. C., Wang, Y. Y., and Tsay, Y. F. (2009). AtCIPK8, a CBL-interacting protein kinase, regulates the low-affinity phase of the primary nitrate response. Plant J. 57, 264–278. doi: 10.1111/j.1365-313X.2008.03685.x
Irizarry, R. A., Hobbs, B., Collin, F., Beazer-Barclay, Y. D., Antonellis, K. J., Scherf, U., et al. (2003). Exploration, normalization, and summaries of high density oligonucleotide array probe level data. Biostatistics 4, 249–264. doi: 10.1093/biostatistics/4.2.249
Jones, M. A., Raymond, M. J., and Smirnoff, N. (2006). Analysis of the root-hair morphogenesis transcriptome reveals the molecular identity of six genes with roles in root-hair development in Arabidopsis. Plant J. 45, 83–100. doi: 10.1111/j.1365-313X.2005.02609.x
Katari, M. S., Nowicki, S. D., Aceituno, F. F., Nero, D., Kelfer, J., Thompson, L. P., et al. (2010). VirtualPlant: a software platform to support systems biology research. Plant Physiol. 152, 500–515. doi: 10.1104/pp.109.147025
Kiba, T., Kudo, T., Kojima, M., and Sakakibara, H. (2011). Hormonal control of nitrogen acquisition: roles of auxin, abscisic acid, and cytokinin. J. Exp. Bot. 62, 1399–1409. doi: 10.1093/jxb/erq410
Kim, K. N., Cheong, Y. H., Grant, J. J., Pandey, G. K., and Luan, S. (2003). CIPK3, a calcium sensor-associated protein kinase that regulates abscisic acid and cold signal transduction in Arabidopsis. Plant Cell 15, 411–423. doi: 10.1105/tpc.006858
Konishi, M., and Yanagisawa, S. (2013). Arabidopsis NIN-like transcription factors have a central role in nitrate signalling. Nat. Commun. 4, 1617. doi: 10.1038/ncomms2621
Krouk, G., Mirowski, P., LeCun, Y., Shasha, D. E., and Coruzzi, G. M. (2010a). Predictive network modeling of the high-resolution dynamic plant transcriptome in response to nitrate. Genome Biol. 11:R123. doi: 10.1186/gb-2010-11-12-r123
Krouk, G., Crawford, N. M., Coruzzi, G. M., and Tsay, Y. F. (2010b). Nitrate signaling: adaptation to fluctuating environments. Curr. Opin. Plant Biol. 13, 266–273. doi: 10.1016/j.pbi.2009.12.003
Kwasniewski, M., Chwialkowska, K., Kwasniewska, J., Kusak, J., Siwinski, K., and Szarejko, I. (2013). Accumulation of peroxidase-related reactive oxygen species in trichoblasts correlates with root hair initiation in barley. J. Plant Physiol. 170, 185–195. doi: 10.1016/j.jplph.2012.09.017
Lan, P., Li, W., Lin, W. D., Santi, S., and Schmidt, W. (2013). Mapping gene activity of Arabidopsis root hairs. Genome Biol. 14:R67. doi: 10.1186/gb-2013-14-6-r67
Langfelder, P., and Horvath, S. (2008). WGCNA: an R package for weighted correlation network analysis. BMC Bioinformatics 9:559. doi: 10.1186/1471-2105-9-559
Langfelder, P., Zhang, B., and Horvath, S. (2008). Defining clusters from a hierarchical cluster tree: the dynamic tree cut package for R. Bioinformatics 24, 719–720. doi: 10.1093/bioinformatics/btm563
Lea, U. S., Slimestad, R., Smedvig, P., and Lillo, C. (2007). Nitrogen deficiency enhances expression of specific MYB and bHLH transcription factors and accumulation of end products in the flavonoid pathway. Planta 225, 1245–1253. doi: 10.1007/s00425-006-0414-x
Lee, R. D., and Cho, H. T. (2013). Auxin, the organizer of the hormonal/environmental signals for root hair growth. Front. Plant Sci. 4:448. doi: 10.3389/fpls.2013.00448
Libault, M., Brechenmacher, L., Cheng, J., Xu, D., and Stacey, G. (2010). Root hair systems biology. Trends Plant Sci. 15, 641–650. doi: 10.1016/j.tplants.2010.08.010
Lillo, C. (2008). Signalling cascades integrating light-enhanced nitrate metabolism. Biochem. J. 415, 11–19. doi: 10.1042/BJ20081115
Lin, C., Choi, H. S., and Cho, H. T. (2011a). Root hair-specific EXPANSIN A7 is required for root hair elongation in Arabidopsis. Mol. Cells 31, 393–397. doi: 10.1007/s10059-011-0046-2
Lin, W. D., Liao, Y. Y., Yang, T. J., Pan, C. Y., Buckhout, T. J., and Schmidt, W. (2011b). Coexpression-based clustering of Arabidopsis root genes predicts functional modules in early phosphate deficiency signaling. Plant Physiol. 155, 1383–1402. doi: 10.1104/pp.110.166520
Liu, H., Yang, H., Wu, C., Feng, J., Liu, X., Qin, H., et al. (2009). Overexpressing HRS1 confers hypersensitivity to low phosphate-elicited inhibition of primary root growth in Arabidopsis thaliana. J. Integr. Plant Biol. 51, 382–392. doi: 10.1111/j.1744-7909.2009.00819.x
Ljung, K., Hull, A. K., Celenza, J., Yamada, M., Estelle, M., Normanly, J., et al. (2005). Sites and regulation of auxin biosynthesis in Arabidopsis roots. Plant Cell 17, 1090–1104. doi: 10.1105/tpc.104.029272
Luan, S., Lan, W., and Chul Lee, S. (2009). Potassium nutrition, sodium toxicity, and calcium signaling: connections through the CBL-CIPK network. Curr. Opin. Plant Biol. 12, 339–346. doi: 10.1016/j.pbi.2009.05.003
Mano, Y., and Nemoto, K. (2012). The pathway of auxin biosynthesis in plants. J. Exp. Bot. 63, 2853–2872. doi: 10.1093/jxb/ers091
Marchive, C., Roudier, F., Castaings, L., Bréhaut, V., Blondet, E., Colot, V., et al. (2013). Nuclear retention of the transcription factor NLP7 orchestrates the early response to nitrate in plants. Nat. Commun. 4, 1713. doi: 10.1038/ncomms2650
Maris, A., Suslov, D., Fry, S. C., Verbelen, J. P., and Vissenberg, K. (2009). Enzymic characterization of two recombinant xyloglucan endotransglucosylase/hydrolase (XTH) proteins of Arabidopsis and their effect on root growth and cell wall extension. J. Exp. Bot. 60, 3959–3972. doi: 10.1093/jxb/erp229
Miller, A. J., and Cramer, M. D. (2005). Root nitrogen acquisition and assimilation. Plant Soil 274, 1–36. doi: 10.1007/s11104-004-0965-1
Mizoi, J., Shinozaki, K., and Yamaguchi-Shinozaki, K. (2012). AP2/ERF family transcription factors in plant abiotic stress responses. Biochim. Biophys. Acta 1819, 86–96. doi: 10.1016/j.bbagrm.2011.08.004
Muday, G. K., Rahman, A., and Binder, B. M. (2012). Auxin and ethylene: collaborators or competitors? Trends Plant Sci. 17, 181–195. doi: 10.1016/j.tplants.2012.02.001
Niu, Y. F., Chai, R. S., Jin, G. L., Wang, H., Tang, C. X., and Zhang, Y. S. (2013). Responses of root architecture development to low phosphorus availability: a review. Ann. Bot. 112, 391–408. doi: 10.1093/aob/mcs285
Patterson, K., Cakmak, T., Cooper, A., Lager, I., Rasmusson, A. G., and Escobar, M. A. (2010). Distinct signalling pathways and transcriptome response signatures differentiate ammonium- and nitrate-supplied plants. Plant Cell Environ. 33, 1486–1501. doi: 10.1111/j.1365-3040.2010.02158.x
Rubin, G., Tohge, T., Matsuda, F., Saito, K., and Scheible, W. R. (2009). Members of the LBD family of transcription factors repress anthocyanin synthesis and affect additional nitrogen responses in Arabidopsis. Plant Cell 11, 3567–3584. doi: 10.1105/tpc.109.067041
Ruffel, S., Krouk, G., Ristova, D., Shasha, D., Birnbaum, K. D., and Coruzzi, G. M. (2011). Nitrogen economics of root foraging: transitive closure of the nitrate-cytokinin relay and distinct systemic signaling for N supply vs. demand. Proc. Natl. Acad. Sci. U.S.A. 108, 18524–18529. doi: 10.1073/pnas.1108684108
Rushton, P. J., Somssich, I. E., Ringler, P., and Shen, Q. J. (2010). WRKY transcription factors. Trends Plant Sci. 15, 247–258. doi: 10.1016/j.tplants.2010.02.006
Sawaki, N., Tsujimoto, R., Shigyo, M., Konishi, M., Toki, S., Fujiwara, T., et al. (2013). A nitrate-inducible GARP family gene encodes an auto-repressible transcriptional repressor in rice. Plant Cell Physiol. 54, 506–517. doi: 10.1093/pcp/pct007
Shearer, H. L., Cheng, Y. T., Wang, L., Liu, J., Boyle, P., Després, C., et al. (2012). Arabidopsis clade I TGA transcription factors regulate plant defenses in an NPR1-independent fashion. Mol. Plant Microbe Interact. 25, 1459–1468. doi: 10.1094/MPMI-09-11-0256
Slabaugh, E., Held, M., and Brandizzi, F. (2011). Control of root hair development in Arabidopsis thaliana by an endoplasmic reticulum anchored member of the R2R3-MYB transcription factor family. Plant J. 67, 395–405. doi: 10.1111/j.1365-313X.2011.04602.x
Stepanova, A. N., Robertson-Hoyt, J., Yun, J., Benavente, L. M., Xie, D. Y., Dolezal, K., et al. (2008). TAA1-mediated auxin biosynthesis is essential for hormone crosstalk and plant development. Cell 133, 177–191. doi: 10.1016/j.cell.2008.01.047
Supek, F., Bošnjak, M., Škunca, N., and Šmuc, T. (2011). REVIGO summarizes and visualizes long lists of gene ontology terms. PLoS ONE 6:e21800. doi: 10.1371/journal.pone.0021800
Vidal, E. A., Araus, V., Lu, C., Parry, G., Green, P. J., Coruzzi, G. M., et al. (2010). Nitrate-responsive miR393/AFB3 regulatory module controls root system architecture in Arabidopsis thaliana. Proc. Natl. Acad. Sci. U.S.A. 107, 4477–4482. doi: 10.1073/pnas.0909571107
Vidal, E. A., and Gutiérrez, R. A. (2008). A systems view of nitrogen nutrient and metabolite responses in Arabidopsis. Curr. Opin. Plant Biol. 11, 521–529. doi: 10.1016/j.pbi.2008.07.003
Vidal, E. A., Moyano, T. C., Riveras, E., Contreras-López, O., and Gutiérrez, R. A. (2013). Systems approaches map regulatory networks downstream of the auxin receptor AFB3 in the nitrate response of Arabidopsis thaliana roots. Proc. Natl. Acad. Sci. U.S.A. 110, 12840–12845. doi: 10.1073/pnas.1310937110
Wang, R., Okamoto, M., Xing, X., and Crawford, N. M. (2003). Microarray analysis of the nitrate response in Arabidopsis roots and shoots reveals over 1,000 rapidly responding genes and new linkages to glucose, trehalose-6-phosphate, iron, and sulfate metabolism. Plant Physiol. 132, 556–567. doi: 10.1104/pp.103.021253
Wang, R., Tischner, R., Gutiérrez, R. A., Hoffman, M., Xing, X., Chen, M., et al. (2004). Genomic analysis of the nitrate response using a nitrate reductase-null mutant of Arabidopsis. Plant Physiol. 136, 2512–2522. doi: 10.1104/pp.104.044610
Wang, R., Xing, X., and Crawford, N. (2007). Nitrite acts as a transcriptome signal at micromolar concentrations in Arabidopsis roots. Plant Physiol. 145, 1735–1745. doi: 10.1104/pp.107.108944
Wang, X., Wu, P., Xia, M., Wu, Z., Chen, Q., and Liu, F. (2002). Identification of genes enriched in rice roots of the local nitrate treatment and their expression patterns in split-root treatment. Gene 297, 93–102. doi: 10.1016/S0378-1119(02)00870-3
Whitacre, J. M. (2012). Biological robustness: paradigms, mechanisms, and systems principles. Front. Genet. 3:67. doi: 10.3389/fgene.2012.00067
Winter, D., Vinegar, B., Nahal, H., Ammar, R., Wilson, G. V., and Provart, N. J. (2007). An “Electronic Fluorescent Pictograph” browser for exploring and analyzing large-scale biological data sets. PLoS ONE 2:e718. doi: 10.1371/journal.pone.0000718
Yi, X., Du, Z., and Su, Z. (2013). PlantGSEA: a gene set enrichment analysis toolkit for plant community. Nucleic Acids Res. 41, W98–W103. doi: 10.1093/nar/gkt281
Yip, A. M., and Horvath, S. (2007). Gene network interconnectedness and the generalized topological overlap measure. BMC Bioinformatics 8:22. doi: 10.1186/1471-2105-8-22
Zhang, B., and Horvath, S. (2005). A general framework for weighted gene co-expression network analysis. Stat. Appl. Genet. Mol. Biol. 4, Article17. doi: 10.2202/1544-6115.1128
Zhang, H., Jennings, A., Barlow, P. W., and Forde, B. G. (1999). Dual pathways for regulation of root branching by nitrate. Proc. Natl. Acad. Sci. U.S.A. 96, 6529–6534. doi: 10.1073/pnas.96.11.6529
Zhang, H., Jin, J., Tang, L., Zhao, Y., Gu, X., Gao, G., et al. (2011). PlantTFDB 2.0: update and improvement of the comprehensive plant transcription factor database. Nucleic Acids Res. 39, D1114–D1117. doi: 10.1093/nar/gkq1141
Zhao, Y., Hull, A. K., Gupta, N. R., Goss, K. A., Alonso, J., Ecker, J. R., et al. (2002). Trp-dependent auxin biosynthesis in Arabidopsis: involvement of cytochrome P450s CYP79B2 and CYP79B3. Genes Dev. 16, 3100–3112. doi: 10.1101/gad.1035402
ZhiMing, Y., Bo, K., XiaoWei, H., ShaoLei, L., YouHuang, B., WoNa, D., et al. (2011). Root hair-specific expansins modulate root hair elongation in rice. Plant J. 66, 725–734. doi: 10.1111/j.1365-313X.2011.04533.x
Keywords: meta-analysis, root hairs, nitrate, systems biology, gene co-expression analysis, transcription factors, Gene Ontology (GO)
Citation: Canales J, Moyano TC, Villarroel E and Gutiérrez RA (2014) Systems analysis of transcriptome data provides new hypotheses about Arabidopsis root response to nitrate treatments. Front. Plant Sci. 5:22. doi: 10.3389/fpls.2014.00022
Received: 24 November 2013; Accepted: 20 January 2014;
Published online: 07 February 2014.
Edited by:
Wolfgang Schmidt, Academia Sinica, TaiwanReviewed by:
Miyako Kusano, RIKEN Plant Science Center, JapanMiriam L. Gifford, The University of Warwick, UK
Copyright © 2014 Canales, Moyano, Villarroel and Gutiérrez. This is an open-access article distributed under the terms of the Creative Commons Attribution License (CC BY). The use, distribution or reproduction in other forums is permitted, provided the original author(s) or licensor are credited and that the original publication in this journal is cited, in accordance with accepted academic practice. No use, distribution or reproduction is permitted which does not comply with these terms.
*Correspondence: Rodrigo A. Gutiérrez, Department of Molecular Genetics and Microbiology, Faculty of Biological Sciences, FONDAP Center for Genome Regulation, Millennium Nucleus Center for Plant Functional Genomics, Pontifical Catholic University of Chile, Avda. Libertador Bernardo O'Higgins 340, Santiago 8331150, Chile e-mail:cmd1dGllcnJlekBiaW8ucHVjLmNs