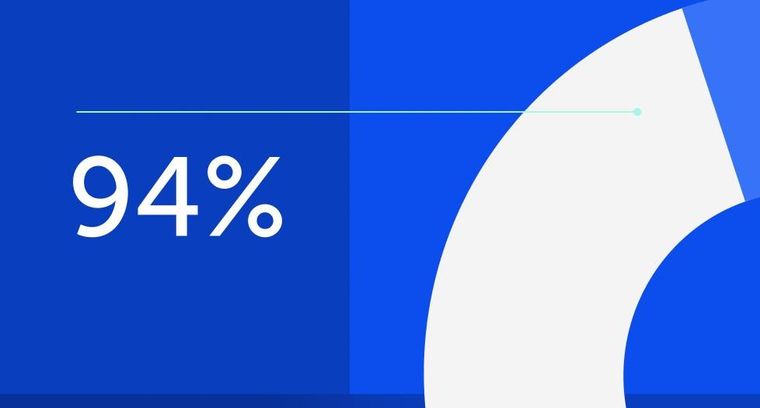
94% of researchers rate our articles as excellent or good
Learn more about the work of our research integrity team to safeguard the quality of each article we publish.
Find out more
TECHNOLOGY REPORT article
Front. Plant Sci., 09 October 2013
Sec. Plant Biophysics and Modeling
Volume 4 - 2013 | https://doi.org/10.3389/fpls.2013.00393
This article is part of the Research TopicApplications of spectroscopy to characterize plant biomassView all 7 articles
In order to understand plant functioning, plant community composition, and terrestrial biogeochemistry, it is decisive to study standing root biomass, (fine) root dynamics, and interactions belowground. While most plant taxa can be identified by visual criteria aboveground, roots show less distinctive features. Furthermore, root systems of neighboring plants are rarely spatially segregated; thus, most soil horizons and samples hold roots of more than one species necessitating root sorting according to taxa. In the last decades, various approaches, ranging from anatomical and morphological analyses to differences in chemical composition and DNA sequencing were applied to discern species’ identity and biomass belowground. Among those methods, a variety of spectroscopic methods was used to detect differences in the chemical composition of roots. In this review, spectroscopic methods used to study root systems of herbaceous and woody species in excised samples or in situ will be discussed. In detail, techniques will be reviewed according to their usability to discern root taxa, to determine root vitality, and to quantify root biomass non-destructively or in soil cores holding mixtures of plant roots. In addition, spectroscopic methods which may be able to play an increasing role in future studies on root biomass and related traits are highlighted.
Studying standing root biomass, root dynamics, and interactions belowground is essential for understanding plant functioning, plant community composition, and terrestrial biogeochemistry (de Kroon and Visser, 2003). Root systems of neighboring plants are rarely spatially segregated, thus, most soil sectors of pristine, agricultural, or silvicultural ecosystems hold roots of more than one species (Hutchings and John, 2003). While most plant taxa can be identified by aboveground criteria, such as flower and leaf morphology, roots show less distinctive features. Because root biomass is the major plant parameter governing water and nutrient uptake (Lambers et al., 2008) and a major sink for plants’ carbohydrates, belowground proportions of species have to be quantified, distinguishing living and dead roots. In the last decades, various approaches, ranging from anatomical and morphological analyses to differences in chemical composition and DNA sequencing were applied to discern species identity and biomass belowground (Rewald et al., 2012). Among those methods, spectroscopic methods were used to detect differences in the chemical composition of roots. Furthermore, root biomass is commonly determined by destructive sampling; to allow for continuous measurements and to reduce the costs of labor intensive root washing procedures, fast and non-destructive methods to determine standing (fine) root biomass and biomass increment are needed.
In this review, essential aspects of spectroscopic methods used to study root systems of herbaceous and woody species in excised samples or in situ will be discussed. In detail, spectroscopic techniques will be reviewed according to their usability to discern root taxa, to determine root vitality, and to quantify root biomass non-destructively or in soil cores holding mixtures of plant roots. Techniques suitable for root analyses on an ultra-structural scale, such as electron energy loss (EEL) spectroscopy (Watteau et al., 2002), are not addressed by this review while spectroscopic methods which are suggested playing an increasing role in future studies on root biomass and related traits are outlined.
Infrared (IR) spectroscopy, especially near-infrared (NIR) and mid-infrared (MIR) spectroscopy, is a standard method to identify and quantify substances. The principle of IR spectroscopy is irradiating a sample and recording the spectral pattern. The chemical composition of a sample determines the spectral print as a function of wavenumber (Chalmers and Griffiths, 2002; Günzler and Gremlich, 2002) and can be utilized for taxa identification. For example, MIR spectroscopy combined with Fourier transformation is able to detect differences in cell-wall composition of leaves which reflect the phylogenetic relationship of plant species (Kim et al., 2004) and was used for species discrimination of fungi and bacteria (Naumann et al., 2005). Today, Fourier transform-infrared (FT-IR) spectroscopy is most commonly used and offers advantages such as short measuring times and high signal-to-noise ratios. While early studies on roots applied IR spectroscopy to determine chemical changes in root tissues of one species (Garrigues et al., 2006; Zhang et al., 2008; Durand et al., 2009). Naumann et al. (2010) achieved a 100% correct discrimination of excised Pisum sativum and Avena sativa roots by FT-MIR spectroscopy with an attenuated total reflectance (ATR) device. One advantage of MIR, compared with NIR, is the more structural spectra and display of the “fingerprint region” (1500-600 cm-1) which is highly characteristic for specific substances and, consequently, beneficial for taxa identification (Skrabal, 2009). FT-MIR–ATR spectra of ground roots of Brassica napus, Triticium aestivum, Apera spica-venti, and Sisymbrium officinale differ in peak location and peak height (Figure 1). Species-specific peaks were especially distinct in the wavenumber region of 1800–400 cm-1. Cluster analysis of the FT-MIR–ATR root spectra allowed for a complete separation according to species (Figure 2). Thus, even the discrimination of closely related crop and weed species within one plant family like T. aestivum and Apera spica-venti as well as Brassica napus and Sisymbrium officinale was possible. The spectral differences between monocotyledons and dicotyledons were more pronounced within one taxonomic group and inter-specific differences of these species’ chemical composition were found higher than the intra-specific heterogeneity. The same distinct differences were also found between the closely related species Zea mays and Echinochloa crus-galli (Poaceae) as well as Beta vulgaris and Chenopodium album (Amaranthaceae; Meinen and Rauber, 2012). Pronounced differences in spectra are the main requirements to distinguish plants on the level of taxonomic groups or species. However, while Naumann et al. (2010) did not find differences in species’ spectral patterns between different environments (i.e., substrate, competitive neighborhood) and root segment positions, Zuo et al. (2007) divided Polygonum cuspidatum root samples from seven geographical origins in China into six classes with principal component analysis (PCA) based on IR fingerprint spectra (Xu et al., 2003). Furthermore, different spectral pattern were detected if roots were inoculated with either rhizobacteria (El Zemrany et al., 2007) or mycorrhizal fungi (Calderón et al., 2009), or treated with environmental pollutants such as benzotriazole (Dokken and Davis, 2011). Thus, because changes in chemical root composition can be caused by changes in abiotic and biotic environments and by secondary growth of roots (White et al., 2011), pure reference samples reflecting the environmental variability and the analyzed “type” of root system are needed to establish more reliable calibration spectra for each species. Beside the need for calibration to local growth conditions, current results demonstrate the ability of IR spectroscopy for distinguishing plant root taxa (Figure 2).
FIGURE 1. FT-MIR–ATR spectra recorded from dried, ground roots of Triticum aestivum (TA), Apera spica-venti (AS), Brassica napus (BN), and Sisymbrium officinale (SO) grown in a greenhouse experiment. Spectra are means of four replications, vector-normalized and offset-corrected (C. Meinen, unpublished data).
FIGURE 2. Cluster analysis of FT-MIR–ATR spectra (Figure 1) recorded from dried, ground roots of Triticum aestivum (TA), Apera spica-venti (AS), Brassica napus (BN), and Sisymbrium officinale (SO) grown in a greenhouse experiment. Data were pre-processed by first derivative and vector normalization. Spectral distances were calculated by Euclidean distance and Ward’s algorithm in the frequency range of 374–3999 cm-1 (mean, n = 4; C. Meinen, unpublished data).
However, spectroscopic techniques to distinguish taxa have only been applied on excised samples. A non-invasive approach using root windows in combination with visible (VIS) and NIR reflectance spectra to distinguish rhizosphere components and root of varying viability (see below) was successfully tested by Nakaji et al. (2008; Figure 3) but did not report species discrimination. Thus, non-destructive approaches for root taxa determination in situ are lacking to date although non-invasive, “remote” IR spectroscopy is common in applied fields such as plastic waste identification (Wienke et al., 1995) and clinical tissue oxygen analyses (Crespi, 2007). While non-invasive IR techniques have the potential to dramatically enhance the application range of (mini-)rhizotrons in mixtures, allowing for species-specific root growth analyses (Pierret, 2008; Rewald and Ephrath, 2013), the different water contents of fresh roots are currently restricting its application. Due to the strong dipole moment of water, which results in a strong signal, applications of IR spectroscopy were for long focused on dry material. Thus an advantage of the ATR techniques is the usability of fresh root material without sample preparation like drying, grinding, or potassium bromide (KBr) pellets. In addition, ATR techniques require only small amounts of sample material which is in many cases limited in root studies. Recently, Meinen and Rauber (2012) noted that fresh rootlets spectra of closely related species (i.e., maize, barnyard grass) showed similar peak distribution when analyzed by FT-MIR–ATR and could not be discriminated by cluster analysis whereas dry rootlets differed in peak location and height. However, distantly related species such as pea and oat could be discriminated by utilizing fresh samples (C. Meinen, unpublished results). While the susceptibility of IR spectroscopy to water content is an intrinsic phenomenon of this technique, a probable solution for closely related species could be the future use of Raman spectroscopy (see below).
FIGURE 3. Classification of Populus spp. rhizosphere components using different combinations of visible (VIS) and near infrared (NIR) wave bands to produce spectral reflectance images at days 20, 55, 70, and 170 after planting (DAP). Wavelengths of the three VIS bands (upper row) and the VIS–NIR bands (lower row) are 650, 550, and 480 nm, and 886, 679, and 522 nm, respectively. A color photo at DAP 70 and the false color classification scheme are given. Source images were taken under wet soil conditions (see Nakaji et al., 2008 for details). Images courtesy of T. Nakaji, K. Noguchi and H. Oguma, Japan.
Dielectric spectroscopy/electrochemical impedance spectroscopy (EIS) methods are widely used to investigate the properties of soils as well as plant and animal tissues (Repo et al., 2012). EIS studies the response to the application of a periodic small amplitude alternating current (AC) signal; measurements are carried out at different AC frequencies and an impedance spectrum is recorded (Lasia, 1999; Cao et al., 2011). A general principle of EIS is that the (root) system is exposed to an alternating electric field that would cause polarization and relaxation phenomena. EIS methods are regarded as an enhancement of earlier electrical measurements at a single frequency (e.g., root capacitance, impedance, and potential; Urban et al., 2011). They have the potential to study (fine) root biomass by the proxy of “active,” i.e., conductive, root surface area (see Repo et al., 2012 for review). Ozier-Lafontaine and Bajazet (2005) used 51 frequencies between 10 Hz and 1 MHz to measure the impedance spectra of soil-grown Solanum lycopersicum. A coefficient of determination of 0.98 was found between the models, based on complex non-linear least squares (CNLS) curve-fitting and including the model variables capacitance C3 and C4, and the increment in root dry weight (see Ozier-Lafontaine and Bajazet, 2005 for details). The high correlation was suggested to be likely based on the proportionality between root capacitance and the quantity of root cells/membranes. In contrast, resistance was found to be the main relevant variable for root length determination, permitting the possibility to distinguish between root biomass and length using EIS. In the same year, Repo et al. (2005) used EIS in a frequency range from 40 Hz to 340 kHz to determine the root biomass of hydroponically grown Salix clones. The most important finding was that the sum of the resistances R1 and R2 (i.e., the resistor of the distributed ZARC-Cole element (Barsoukov and Macdonald, 2005)) in the developed electric model decreased with an increase in root mass of each individual plant (Figure 4). These studies encourage further research on the applicability of EIS methods as a fast way to determine root growth and root biomass of single plant individuals with rather minor destructions (i.e., electrode installation in the shoot). However, future studies are required since environmental parameters such as soil type, and soil and root moisture contents, known to have strong effects on capacitance (Dalton, 1995; Repo et al., 2000), and the influence of the experimental set-up, especially electrode position (Repo et al., 2012), have not been fully understood yet.
FIGURE 4. Fresh weight of hydroponically grown Salix spp. roots and the reciprocal sum of resistances R1 and R2 during growth (mean, n = 3; Repo et al., 2005, modified). Electrochemical impedance spectroscopy (EIS) was used to determine R1 and R2 (see Repo et al., 2005 for details).
While useful for non-destructive root biomass estimation of single plant individuals with sufficiently sized stems, EIS cannot be directly used to determine the proportion of species’ roots in plant mixtures. However, understanding plant community functioning and geochemical consequences (e.g., in terms of C sequestration) requires an accurate assessment of the belowground biomass and the distribution of each species in the community. Similar to the procedure of taxa determination by IR spectroscopy (see above), differences in the chemical composition of mixed root samples can be utilized to determine biomass proportions in excised soil samples. Aboveground, IR spectroscopy has been frequently used to determine the botanical composition of forage mixtures (Coleman et al., 1985, 1990). On roots, NIR spectroscopy was applied first by Rumbaugh et al. (1988) to predict the root biomass proportion of four grass species in binary mixtures with Medicago sativa. Prepared Medicago-grass root mixtures with grass ratios from 0 to 1 were recorded by NIRS, and root spectra were correlated to corresponding root proportion. Coefficients of determination ranged from 0.92 to 0.99 between the actual and the predicted root mass ratio. Similarly, Roumet et al. (2006) tested artificial root mixtures with two and three species (five Poaceae, one Asteraceae), and estimated the proportion of the species in the mixtures using NIR spectra (25000–4000 cm-1) with high accuracy (r = 0.99). Finally, the prediction of species ratios in woody fine-root mixtures (Fagus sylvatica, Quercus petraea, Picea abies, Pseudotsuga menziesii – with two, or up to four tree species plus herbal roots) was successfully demonstrated by Bauhus and colleagues (Bauhus et al., 2007; Lei and Bauhus, 2010). Even in tree root samples with low abundance of specific species, NIR models presented reasonable approximations of the biomass abundance.
To determine the species composition in mixed samples with IR spectroscopy, reference samples of pure material are imperative to create calibration series. Calibration samples are created by mixing pure (ground) material of the species for which the calibration is developed in known proportions and continuously. Roumet et al. (2006) suggested that each calibration required the preparation of >40 artificial mixtures. On fodder mixtures, two different methods have been applied to provide the pure material for calibration series, so called “artificial” and “real” samples (Cougnon et al., 2013). It has been claimed that calibrations based on “artificial” samples, which are attained from pure stands (Coleman et al., 1985; Petersen et al., 1987), have good calibration statistics but fail to predict real validation samples while “real” samples, obtained by hand sorting of species mixtures (Shaffer et al., 1990; Wachendorf et al., 1999), reflect the environmental and thus spectral variation to a greater extent (Pitman et al., 1991; Cougnon et al., 2013). Studying roots, Roumet et al. (2006) used both “artificial” and “real” samples, originating from both natural and environmentally controlled conditions, and found that the predictive equations for calibration were robust. However, the impact of the calibration material origin has not been addressed in greater depth and additional research is necessary. However, regarding the difficult determination of root taxa by visual criteria, which makes hand sorting of grass and herbaceous roots impossible (Rewald et al., 2012), and the high amount of pure sample material needed (~15 g dry weight per species; Roumet et al., 2006), the use of pure “artificial” samples grown under similar environmental conditions as the analyzed sampled might be the only practical and economically feasible option.
In contrast to other studies, which utilized only one bulk sample for each species to generate artificial mixtures (e.g., Roumet et al., 2006), the mixed samples created by Lei and Bauhus (2010) also captured the variation in spectral properties that occur within each species. To capture this variation is very important if species proportions should be predicted in field samples. The quality of prediction of NIR models was assumed to decline with increasing species numbers within samples (Coleman et al., 1990). However, Lei and Bauhus (2010) could show that models based on three components are not more robust than models based on five species. Furthermore, even in highly species diverse forest ecosystems, a single tree has a limited number of inter-specific neighbors. Thus at any given point in space, the actual number of species contained in fine-roots samples from forests will be limited. The same holds true for agricultural mixtures while further research is needed for ecosystems with a high diversity at a small scale such as grasslands. Similar to NIR models, MIR models can predict species proportions in mixtures of herbaceous species. To study the impact of weed roots, a Fourier transform MIR–ATR model for Vicia faba and Matricaria chamomilla was developed (Table 1). Model development and data analysis were carried out with the software OPUS QUANT 2 (Version 7.0; Bruker, 2011). The cross-validation option was used to create the model. The best model with highest coefficient of correlation (R2) and lowest root mean square error of cross validation (RMSECV) was selected by running a procedure for model optimization provided by the software. An independent sample set (n = 16) with known species proportion was used for external validation. This validation revealed a high correlation coefficient (0.99) and a low RMSE of prediction (4.43). Even with a relatively small number of 21 calibration samples the quality of the model was very good. These results indicate that MIR models are a promising tool to quantify species proportions in root mixtures of herbaceous plants.
TABLE 1. Statistical parameters of the two-component FT-IR–ATR model for Vicia faba and Matricaria chamomilla in terms of calibration, validation and external test set validation.
Thus, IR spectroscopy can accurately estimate the botanical composition of moderately diverse root mixtures if sufficient amounts of pure root material are available for calibration. The roots for calibration should be grown and harvested under the same conditions as those in mixed samples.
The ability to determine the vitality of roots, with its extremes “life” and “dead,” is of utmost importance to identify the active (fine) root biomass available for water and nutrient uptake and to determine root longevity. IR spectroscopy has been used to distinguish alive, injured and dead bacteria (Davis and Mauer, 2010) and to discriminate damaged seeds (Agelet et al., 2012) with varying success (see below).
Picon-Cochard et al. (2009) used NIR spectroscopy to predict the percentage of dead versus living roots of five grass species grown in monocultures under field conditions. Root death was induced after total severance of aboveground vegetation. Root samples were collected immediately after this treatment to obtain predominantly live roots, and then 1 and 2 months later to obtain dead roots. NIR spectra of Control samples were different from later samples for four of the five species. The percentage of live and dead roots was significantly predicted by NIR with an error of prediction of 15%. Working in forests, Bauhus et al. (2007) stated that the proportion of live and dead F. sylvatica and Picea abies fine roots can be determined with high accuracy using NIR analysis. The two studies show the potential of NIR to predict the percentage of dead and live roots in excised samples. In the field, Nakaji et al. (2008) used VIS and NIR reflectance spectra of glass-faced rhizoboxes to automatically distinguish Populus spp. roots from soil and leaf mold and to classify roots into four age classes and dead roots (Figure 3) as reported earlier for crop residue (Daughtry et al., 1995). An ethylene vinyl acetate card was used by Nakaji et al. (2008) to standardize their reflectance measurements, an approach likely similar to the calibration model developed by Watari and Ozaki (2005). The reflectance of dead roots was lower than that of mature roots in both VIS and NIR spectral regions. Although the most suitable spectral bands differed between moist and dry soil conditions, and fewer pixels were classified correctly in dryer soil, the spectral bands 17200-14700 and 11800-11200 cm-1 provided reasonable reliability under both conditions. Classification accuracy was higher when using two to five VIS–NIR images (overall accuracy ≤87.8%) than three VIS images (red, green, and blue; accuracy <67.1%). Irrespective of soil moisture condition, the overall accuracy tended to be stable at 92–94% with use of four to five VIS–NIR wavebands; however, the same accuracy could not be obtained for all age classes. The spectral bands effective under wet soil conditions could also be used for classification in dry conditions, with overall accuracies >86.9%. These results suggest that automatic image analysis using combined VIS–NIR images of multiple spectral bands (Figure 3) allows for accurate classification of Populus spp. roots’ live/dead status and relative accurate classification of the age class. While further studies on roots are absent, it is known for bacteria and seeds that spectral differences between live and injured bacteria or heat and freezing stress can be hardly discernible due to the minor compositional differences (Davis and Mauer, 2010; Agelet et al., 2012). Thus, Davis and Mauer (2010), for example, used second derivative pre-processing to increase the number of discriminative features and PCA and soft independent modeling by class analogy (SIMCA) models to correctly classify live and injured bacteria. Similar procedures might be applicable for root vitality analyses with spectroscopic techniques. Further studies on additional species, growing in different soil types and under a larger range of soil moisture conditions are needed to determine the full capacity of VIS–NIR spectroscopy for root viability determination.
Fluorescence is the emission of light subsequent to absorption of ultraviolet (UV) or VIS light by a fluorescent molecule or substructures called fluorophore. Thus, the fluorophore absorbs energy in the form of light at a specific wavelength and emits energy in the form of light emitted at a lower energy level. Fluorescence spectroscopy (FS) is widely used for chemical analyses of auto-fluorescing molecules (Sharma and Schulman, 1999); in plants, chlorophyll fluorescence has been widely used to determine the physiological status of leaves (Zinnert et al., 2013). While in situ root observations on Glycine max showed that nutrient absorption and root elongation rates positively correlate to fluorescence intensity (Dyer and Brown, 1983) and that fluorescence can be influenced by microbial colonization (Gamalero et al., 2004) non-species-specific influences are questioning the applicability of simple fluorescence measurements for root classification. However, more advanced FS approaches obtaining information about the entire fluorescence landscape (or at least >2 excitation wavelength and spectra of emission) in order to find excitation and emission maxima as well as the structure of the curve are likely to allow the application of FS on roots. The utilization of FS is of particular interest because a combined fluorescence and reflectance spectroscopy approach on animal tissues was found to significantly improve signal/noise ratios, disentangling absorption, scattering, and intrinsic fluorescence parameters (Müller et al., 2002).
A variety of X-ray spectroscopic methods has been used to characterize plant tissues in the past as well as plant–environmental interactions such as the biochemistry of the rhizosphere. For example, Agrahari et al. (2010) successfully determined the chemical properties of dried, powdered root tubers with energy dispersive X-ray (EDX) spectroscopy. While EDX has not been used to discriminate among root taxa yet, EDX spectra reflect the composition of the tissue and might thus be used for such purposes similar to IR spectrometry (see above). Frommer et al. (2011) and others used (micro-focused) energy-dispersive X-ray fluorescence (XRF) spectrometry to determine the spatial distribution of certain metal elements. It seem feasible to use this technique to determine plant root taxa in soil thin sections prepared from undisturbed soil samples, although the work load for sample preparation is high and the method is not amendable to study many samples.
Raman spectroscopy (RS) is in general less widely used than IR spectroscopy, mainly due to early difficulties with sample degradation and fluorescence (Allison, 2011). However, a renaissance of Raman spectroscopy is triggered by advanced laser technology, by efficient interference filters to suppress elastically scattered Rayleigh light, by the development of more sensitive detectors and new methodological approaches (e.g., FT-RS; Schmitt and Popp, 2006; Schulz, 2008). These improvements, together with the ability to examine raw samples without any preparation by FT-RS have led to a rapid growth in application (Kizil and Irudayaraj, 2008). While FT-RS has been successfully used as a non-destructive technique for chemotaxonomy on aboveground tissues (Schrader et al., 1991, 1999) and to determine concentrations of specific chemicals within roots (Andreev et al., 2001; Frosch et al., 2007; de Oliveira et al., 2009) it has, to the best of our knowledge, not been used to discriminate among root taxa. Compared to IR spectrometry, RS has two major advantages which make this technique most suitable to perform in situ studies on roots, (i) water content has only weak Raman scattering properties (Gierlinger and Schwanninger, 2007), and (ii) RS does not require optically clear samples making measurements through colored glass and plastic feasible – which are used at the soil interface in (mini-)rhizotron studies (Rewald and Ephrath, 2013).
Nuclear magnetic resonance (NMR) spectroscopy is a non-destructive analytical method that generates data on the presence of a wide range of low molecular weight metabolites in aqueous extracts. Several studies have used NMR-based methods on plant material, demonstrating biochemical differences according to tissue age, geographical location, genetic modification, and response to stress. For example, Shin et al. (2007) performed fingerprinting analyses of fresh, differently old ginseng roots using 1H-NMR spectroscopy and multivariate analysis techniques. The authors detected various distinct peaks in the 1H-NMR spectra of the liquid-state ginseng roots. Two dimensional score plots of a PCA showed clear separations of components at different roots ages, and explained 89.6% of the total variance. These results and others indicate that the combination of 1H-NMR and PCA provides a powerful tool for authenticating root taxa, differentiating between root age classes and to investigate the effects of several environmental stressors (Gavaghan et al., 2011). Other NMR spectroscopic approaches such as 14N-NMR or 13C-NMR, and especially those allowing for solid state analyses, could be similarly suitable to distinguish plant individual or taxa and to determine root viability by metabolic profiling (Farag et al., 2012; Incerti et al., 2013). While solid state (13C)-NMR spectroscopy should be especially useful, its availability is limited to, so far, only few laboratories. Similar, the laborious preparation of liquid extracts and the high costs of NMR in general will limit the broad use of these techniques for non-molecular analysis and prevents high throughput analysis of root samples.
Internal and diffuse external reflectance techniques based on IR are relatively fast and affordable and proved to be able for distinguishing even closely related species, quantifying the biomass proportion of species in mixtures and/or determining the root viability status. However, a plethora of other spectroscopic techniques is available but yet rarely used for these purposes. Thus even though most of the techniques mentioned above provide a powerful tool for the investigation of roots, a most accurate determination can likely be obtained through the integration of complementary approaches. Especially the usability of FT-RS and fluorescence spectrometry should be evaluated further because of the ability of these techniques to analyze roots through glass/plastic windows – as commonly used for (mini-)rhizotron studies – possibly overcoming uncertainties of earlier UV–VIS-based methods to determine root vitality (Wang et al., 1995). Beside the actual techniques, the broad identification of root taxa of single roots and in root mixtures and the determination of viability parameters is hampered by the availability of databases of species- and environmental-specific spectral profiles of roots. This would help researchers to identify even unknown roots in their sample, similar to gene sequence databases for root research (Rewald et al., 2012).
The authors declare that the research was conducted in the absence of any commercial or financial relationships that could be construed as a potential conflict of interest.
The authors wish to thank T. Nakaji, Japan and colleagues for kindly providing the pictures underlying Figure 3. We acknowledge support by the Open Access Publication Funds of the Göttingen University.
Agelet, L. E., Ellis, D. D., Duvick, S., Goggi, A. J. S., Hurburgh, C. R., and Gardner, C. A. (2012). Feasibility of near infrared spectroscopy for analyzing corn kernel damage and viability of soybean and corn kernels. J. Cereal Sci. 55, 160–165. doi: 10.1016/j.jcs.2011.11.002
Agrahari, A. K., Meher, A., and Pradhan, A. R. (2010). Energy dispersive X-ray spectroscopy (EDX) analysis of Curculigo orchioides Gaertn root tubers. Drug Invent. Today 2, 29–30.
Allison, G. G. (2011). “Application of Fourier transform mid-infrared spectroscopy (FTIR) for research into biomass feed-stocks,” in Fourier Transforms – New Analytical Approaches and FTIR Strategies, ed. G. Nikolic (Rijeka: InTech), 71–88.
Andreev, G. N., Schrader, B., Schulz, H., Fuchs, R., Popov, S., and Handjieva, N. (2001). Non-destructive NIR–FT–Raman analyses in practice. Part 1. Analyses of plants and historic textiles. Fresenius J. Anal. Chem. 371, 1009–1017. doi: 10.1007/s00216-001-1109-6
Barsoukov, E., and Macdonald, J. R. (2005). Impedance Spectroscopy: Theory, Experiment, and Applications. Hoboken, NJ: John Wiley & Sons. doi: 10.1002/0471716243
Bauhus, J., Gruselle, M.-C., Nitschke, R., Mohammed, D., Frischbier, N., and Khanna, P. K. (2007). “The use of near-infrared spectroscopy to study ecological interactions in mixed Norway spruce – European beech forests,” in Linking Local Management to Hlobal Change Challenges: The Proceedings of the International Symposium on Forest Soils and Ecosystem Health, eds Z. Xu, C. E. Johnson, C. Chen, and T. J. Blumfield (Brisbane: Centre for Forestry and Horticultural Research, Griffith University), 15–16.
Calderón, F. J., Acosta-Martinez, V., Douds, D. D., Reeves, J. B., and Vigil, M. F. (2009). Mid-infrared and near-infrared spectral properties of mycorrhizal and non-mycorrhizal root cultures. Appl. Spectrosc. 63, 494–500. doi: 10.1366/000370209788346931
Cao, Y., Repo, T., Silvennoinen, R., Lehto, T., and Pelkonen, P. (2011). Analysis of the willow root system by electrical impedance spectroscopy. J. Exp. Bot. 62, 351–358. doi: 10.1093/jxb/erq276
Chalmers, J. M., and Griffiths, P. R. (2002). Handbook of Vibrational Spectroscopy. Chichester: Wiley.
Coleman, S. W., Barton, F. E., and Meyer, R. D. (1985). The use of near infrared reflectance spectroscopy to predict species composition of forage mixtures. Crop Sci. 25, 834–837. doi: 10.2135/cropsci1985.0011183X002500050026x
Coleman, S. W., Christiansen, S., and Shenk, J. S. (1990). Prediction of botanical composition using NIRS calibrations developed from botanically pure samples. Crop Sci. 30, 202–207. doi: 10.2135/cropsci1990.0011183X003000010044x
Cougnon, M., Van Waes, C., Baert, J., and Reheul, D. (2013). “NIRS calibration strategies for the botanical composition of grass-clover mixtures,” in Breeding Strategies for Sustainable Forage and Turf Grass Improvement, eds S. Barthe and D. Milbourne (Dordrecht: Springer), 371–375. doi: 10.1007/978-94-007-4555-1_51
Crespi, F. (2007). Near-infrared spectroscopy (NIRS): a non-invasive in vivo methodology for analysis of brain vascular and metabolic activities in real time in rodents. Curr. Vasc. Pharmacol. 5, 305–321. doi: 10.2174/157016107782023398
Dalton, F. N. (1995). In-situ root extent measurements by electrical capacitance methods. Plant Soil 173, 157–165. doi: 10.1007/BF00155527
Daughtry, C. S. T., Mcmurtrey Iii, J. E., Chappele, E. W., Dulaney, W. P., Irons, J. R., and Satterwhite, M. B. (1995). Potential for discriminating crop residues from soil by reflectance and fluorescence. Agron. J. 87, 165–171. doi: 10.2134/agronj1995.00021962008700020005x
Davis, R., and Mauer, L. J. (2010). “Fourier transform infrared (FT-IR) spectroscopy: a rapid tool for detection and analysis of foodborne pathogenic bacteria,” in Current Research, Technology and Education Topics in Applied Microbiology and Microbial Biotechnology, ed. A. Méndez-Vilas (Badajoz: Formatex), 1582–1594.
de Kroon, H., and Visser, E. J. (2003). Root Ecology. Berlin: Springer. doi: 10.1007/978-3-662-09784-7
de Oliveira, L. F. C., Da Silva Velozo, E., and Edwards, H. G. M. (2009). Raman spectroscopic characterization of the alkaloid dihydrochelerytrine extracted from roots of Zanthoxylum stelligerum (Turcz). Spectrosc. Lett. 42, 194–198. doi: 10.1080/00387010902827635
Dokken, K. M., and Davis, L. C. (2011). Infrared monitoring of dinitrotoluenes in sunflower and maize roots. J. Environ. Qual. 40, 719–730. doi: 10.2134/jeq2010.0143
Durand, C., Vicré-Gibouin, M., Follet-Gueye, M. L., Duponchel, L., Moreau, M., Lerouge, P., et al. (2009). The organization pattern of root border-like cells of Arabidopsis is dependent on cell wall homogalacturonan. Plant Physiol. 150, 1411–1421. doi: 10.1104/pp.109.136382
Dyer, D., and Brown, D. A. (1983). Relationship of fluorescent intensity to ion uptake and elongation rates of soybean roots. Plant Soil 72, 127–134. doi: 10.1007/BF02185102
El Zemrany, H., Czarnes, S., Hallett, P. D., Alamercery, S., Bally, R., and Jocteur Monrozier, L. (2007). Early changes in root characteristics of maize (Zea mays) following seed inoculation with the PGPR Azospirillum lipoferum CRT1. Plant Soil 291, 109–118. doi: 10.1007/s11104-006-9178-0
Farag, M. A., Porzel, A., and Wessjohann, L. A. (2012). Comparative metabolite profiling and fingerprinting of medicinal licorice roots using a multiplex approach of GC–MS, LC–MS and 1D NMR techniques. Phytochemistry 76, 60–72. doi: 10.1016/j.phytochem.2011.12.010
Frommer, J., Voegelin, A., Dittmar, J., Marcus, M., and Kretzschmar, R. (2011). Biogeochemical processes and arsenic enrichment around rice roots in paddy soil: results from micro-focused X-ray spectroscopy. Eur. J. Soil Sci. 62, 305–317. doi: 10.1111/j.1365-2389.2010.01328.x
Frosch, T., Schmitt, M., Noll, T., Bringmann, G., Schenzel, K., and Popp, J. (2007). Ultrasensitive in situ tracing of the alkaloid dioncophylline A in the tropical liana Triphyophyllum peltatum by applying deep-UV resonance Raman microscopy. Anal. Chem. 79, 986–993. doi: 10.1021/ac061526q
Gamalero, E., Lingua, G., Capri, F. G., Fusconi, A., Berta, G., and Lemanceau, P. (2004). Colonization pattern of primary tomato roots by Pseudomonas fluorescens A6RI characterized by dilution plating, flow cytometry, fluorescence, confocal and scanning electron microscopy. FEMS Microbiol. Ecol. 48, 79–87. doi: 10.1016/j.femsec.2003.12.012
Garrigues, E., Doussan, C., and Pierret, A. (2006). Water uptake by plant roots: I – formation and propagation of a water extraction front in mature root systems as evidenced by 2D light transmission imaging. Plant Soil 283, 83–98. doi: 10.1007/s11104-004-7903-0
Gavaghan, C. L., Li, J. V., Hadfield, S. T., Hole, S., Nicholson, J. K., Wilson, I. D., et al. (2011). Application of NMR-based metabolomics to the investigation of salt stress in maize (Zea mays). Phytochem. Anal. 22, 214–224. doi: 10.1002/pca.1268
Gierlinger, N., and Schwanninger, M. (2007). The potential of Raman microscopy and Raman imaging in plant research. Spectroscopy 21, 69–89. doi: 10.1155/2007/498206
Hutchings, M. J., and John, E. A. (2003). “Distribution of roots in soil, and root foraging activity,” in Root Ecology, eds H. de Kroon and E. J. W. Visser (Berlin: Springer), 33–59.
Incerti, G., Romano, A., Termolino, P., and Lanzotti, V. (2013). Metabolomic fingerprinting using nuclear magnetic resonance and multivariate data analysis as a tool for biodiversity informatics: a case study on the classification of Rosa × damascena. Plant Biosyst. doi: 10.1080/11263504.2013.781072 (in press).
Kim, S. W., Ban, S. H., Chung, H., Cho, S., Chung, H. J., Choi, P. S., et al. (2004). Taxonomic discrimination of flowering plants by multivariate analysis of Fourier transform infrared spectroscopy data. Plant Cell Rep. 23, 246–250. doi: 10.1007/s00299-004-0811-1
Kizil, R., and Irudayaraj, J. (2008). “Spectroscopic technique: Fourier transform Raman (FT Raman) spectroscopy,” in Modern Techniques for Food Authentification, ed. D. W. Sun (New York: Elsevier), 185–203.
Lambers, H., Chapin Iii, F. S., and Pons, T. L. (2008). Plant Physiological Ecology. Dordrecht: Springer. doi: 10.1007/978-0-387-78341-3
Lasia, A. (1999). “Electrochemical impedance spectroscopy and its applications,” in Modern Aspects of Electrochemistry, eds B. E. Conway, J. Bockris, and R. E. White (New York: Kluwer), 143–248.
Lei, P., and Bauhus, J. (2010). Use of near-infrared reflectance spectroscopy to predict species composition in tree fine-root mixtures. Plant Soil 333, 93–103. doi: 10.1007/s11104-010-0325-2
Meinen, C., and Rauber, R. (2012). FT-IR–ATR spectroscopy – a new approach in root discrimination of crop and weed species. Julius-Kühn-Archiv 434, 702–707.
Müller, M. G., Wax, A., Georgakoudi, I., Dasari, R. R., and Feld, M. S. (2002). A reflectance spectrofluorometer for real-time spectral diagnosis of disease. Rev. Sci. Instrum. 73, 3933–3937. doi: 10.1063/1.1511795
Nakaji, T., Noguchi, K., and Oguma, H. (2008). Classification of rhizosphere components using visible-near infrared spectral images. Plant Soil 310, 245–261. doi: 10.1007/s11104-007-9478-z
Naumann, A., Heine, G., and Rauber, R. (2010). Efficient discrimination of oat and pea roots by cluster analysis of Fourier transform infrared (FTIR) spectra. Field Crops Res. 119, 78–84. doi: 10.1016/j.fcr.2010.06.017
Naumann, A., Navarro-Gonzalez, M., Peddireddi, S., Kues, U., and Polle, A. (2005). Fourier transform infrared microscopy and imaging: detection of fungi in wood. Fungal Genet. Biol. 42, 829–835. doi: 10.1016/j.fgb.2005.06.003
Ozier-Lafontaine, H., and Bajazet, T. (2005). Analysis of root growth by impedance spectroscopy (EIS). Plant Soil 277, 299–313. doi: 10.1007/s11104-005-7531-3
Petersen, J. C., Barton, F. E., Windham, W. R., and Hoveland, C. S. (1987). Botanical composition definition of tall fescue-white clover mixtures by near infrared reflectance spectroscopy. Crop Sci. 27, 1077–1080. doi: 10.2135/cropsci1987.0011183X002700050050x
Picon-Cochard, C., Pilon, R., Revaillot, S., Jestin, M., and Dawson, L. (2009). Use of near-infrared reflectance spectroscopy to predict the percentage of dead versus living grass roots. Plant Soil 317, 309–320. doi: 10.1007/s11104-008-9810-2
Pierret, A. (2008). Multi-spectral imaging of rhizobox systems: new perspectives for the observation and discrimination of rhizosphere components. Plant Soil 310, 263–268. doi: 10.1007/s11104-008-9651-z
Pitman, W. D., Piacitelli, C. K., Aiken, G. E., and Barton, F. E. (1991). Botanical composition of tropical grass-legume pastures estimated with near-infrared reflectance spectroscopy. Agron. J. 83, 103–107. doi: 10.2134/agronj1991.00021962008300010025x
Repo, T., Cao, Y., Silvennoinen, R., and Ozier-Lafontaine, H. (2012). “Electrical impedance spectroscopy and roots,” in Measuring Roots – An Updated Approach, ed. S. Mancuso (Berlin: Springer), 25–49.
Repo, T., Kalliokoski, T., Domisch, T., Lehto, T., Mannerkoski, H., Sutinen, S., et al. (2005). Effects of timing of soil frost thawing on Scots pine. Tree Physiol. 25, 1053–1062. doi: 10.1093/treephys/25.8.1053
Repo, T., Zhang, G., Ryyppö, A., and Rikala, R. (2000). The electrical impedance spectroscopy of Scots pine (Pinus sylvestris L.) shoots in relation to cold acclimation. J. Exp. Bot. 51, 2095–2107. doi: 10.1093/jexbot/51.353.2095
Rewald, B., and Ephrath, J. E. (2013). “Minirhizotron techniques,” in Plant Roots: The Hidden Half, 4th Edn, eds A. Eshel and T. Beeckman (New York: CRC Press), 1–15.
Rewald, B., Meinen, C., Trockenbrodt, M., Ephrath, J. E., and Rachmilevitch, S. (2012). Root taxa identification in plant mixtures – current techniques and future challenges. Plant Soil 359, 165–182. doi: 10.1007/s11104-012-1164-0
Roumet, C., Picon-Cochard, C., Dawson, L. A., Joffre, R., Mayes, R., Blanchard, A., et al. (2006). Quantifying species composition in root mixtures using two methods: near-infrared reflectance spectroscopy and plant wax markers. New Phytol. 170, 631–638. doi: 10.1111/j.1469-8137.2006.01698.x
Rumbaugh, M. D., Clark, D. H., and Pendery, B. M. (1988). Determination of root mass ratios in alfalfa-grass mixtures using near infrared reflectance spectroscopy. J. Range Manag. 41, 488–490. doi: 10.2307/3899523
Schmitt, M., and Popp, J. (2006). Raman spectroscopy at the beginning of the twenty-first century. J. Raman Spectrosc. 37, 20–28. doi: 10.1002/jrs.1486
Schrader, B., Hoffmann, A., Simon, A., and Sawatzki, J. (1991). Can a Raman renaissance be expected via the near-infrared Fourier-transform technique? Vib. Spectrosc. 1, 239–250. doi: 10.1016/0924-2031(91)85001-4
Schrader, B., Klump, H. H., Schenzel, K., and Schulz, H. (1999). Non-destructive NIR FT Raman analysis of plants. J. Mol. Struct. 509, 201–212. doi: 10.1016/S0022-2860(99)00221-5
Schulz, H. (2008). “Spectroscopic technique: Raman spectroscopy,” in Modern Techniques for Food Authentification, ed. D. W. Sun (New York: Elsevier), 149–184.
Shaffer, J. A., Jung, G. A., Shenk, J. S., and Abrams, S. M. (1990). Estimation of botanical composition in Alfalfa/Ryegrass mixtures by near infrared spectroscopy. Agron. J. 82, 669–673. doi: 10.2134/agronj1990.00021962008200040004x
Shin, Y. S., Bang, K. H., In, D. S., Kim, O. T., Hyun, D. Y., Ahn, I. O., et al. (2007). Fingerprinting analysis of fresh ginseng roots of different ages using 1H-NMR spectroscopy and principal components analysis. Arch. Pharm. Res. 30, 1625–1628. doi: 10.1007/BF02977333
Skrabal, P. M. (2009). Spektroskopie. Eine Methodenübergreifende Darstellung vom UV-bis zum NMR-Bereich. Zurich: UTB.
Urban, J., Bequet, R., and Mainiero, R. (2011). Assessing the applicability of the earth impedance method for in situ studies of tree root systems. J. Exp. Bot. 62, 1857–1869. doi: 10.1093/jxb/erq370
Wachendorf, M., Ingwersen, B., and Taube, F. (1999). Prediction of the clover content of red clover- and white clover-grass mixtures by near-infrared reflectance spectroscopy. Grass Forage Sci. 54, 87–90. doi: 10.1046/j.1365-2494.1999.00150.x
Wang, Z. Q., Burch, W. H., Mou, P., Jones, R. H., and Mitchell, R. J. (1995). Accuracy of visible and ultra-violet light for estimating live root proportions with minirhizotrons. Ecology 76, 2330–2334. doi: 10.2307/1941705
Watari, M., and Ozaki, Y. (2005). Calibration models for the vinyl acetate concentration in ethylene-vinyl acetate copolymers and its on-line monitoring by near-infrared spectroscopy and chemometrics: use of band shifts associated with variations in the vinyl acetate concentration to improve the models. Appl. Spectrosc. 59, 912–919. doi: 10.1366/0003702054411571
Watteau, F., Villemin, G., Ghanbaja, J., Genet, P., and Pargney, J. C. (2002). In situ ageing of fine beech roots (Fagus sylvatica) assessed by transmission electron microscopy and electron energy loss spectroscopy: description of microsites and evolution of polyphenolic substances. Biol. Cell 94, 55–63. doi: 10.1016/S0248-4900(02)01182-6
White, K. E., Reeves, J. B., and Coale, F. J. (2011). Mid-infrared diffuse reflectance spectroscopy for the rapid analysis of plant root composition. Geoderma 167, 197–203. doi: 10.1016/j.geoderma.2011.08.009
Wienke, D., Van Den Broek, W., and Buydens, L. (1995). Identification of plastics among non-plastics in mixed waste by remote sensing near infrared imaging spectroscopy. 2. Multivariate image rank analysis for rapid classification. Anal. Chem. 67, 3760–3766. doi: 10.1021/ac00116a023
Xu, Y. Q., Sun, S. Q., Feng, X. F., and Hu, S. L. (2003). Quick identification of scullcaps in different geographical origins using clustering analysis method and infrared fingerprint spectra [in Chinese with English abstract]. Guang Pu Xue Yu Guang Pu Fen Xi 23, 502–505.
Zhang, X. B., Liu, P., Li, D. T., Xu, G. D., and Jiang, M. J. (2008). FTIR spectroscopic characterization of chromium-induced changes in root cell wall of plants [in Chinese with English abstract]. Guang Pu Xue Yu Guang Pu Fen Xi 28, 1067–1070.
Zinnert, J., Via, S., and Young, D. (2013). Distinguishing natural from anthropogenic stress in plants: physiology, fluorescence and hyperspectral reflectance. Plant Soil 366, 133–141. doi: 10.1007/s11104-012-1414-1
Keywords: electrochemical impedance spectroscopy, fine root, IR spectrometry, root biomass, root taxa, root vitality
Citation: Rewald B and Meinen C (2013) Plant roots and spectroscopic methods – analyzing species, biomass, and vitality. Front. Plant Sci. 4:393. doi: 10.3389/fpls.2013.00393
Received: 26 August 2013; Accepted: 13 September 2013;
Published online: 09 October 2013.
Edited by:
Miguel Vega-Sanchez, Lawrence Berkeley National Laboratory, USAReviewed by:
Andreia Michelle Smith-Moritz, Lawrence Berkeley National Laboratory, USACopyright © 2013 Rewald and Meinen. This is an open-access article distributed under the terms of the Creative Commons Attribution License (CC BY). The use, distribution or reproduction in other forums is permitted, provided the original author(s) or licensor are credited and that the original publication in this journal is cited, in accordance with accepted academic practice. No use, distribution or reproduction is permitted which does not comply with these terms.
*Correspondence: Catharina Meinen, Division of Agronomy, Department of Crop Sciences, Georg-August-Universität Göttingen, Von-Siebold-Str. 8, Göttingen 37075, Germany e-mail:Y2F0aGFyaW5hLm1laW5lbkBhZ3IudW5pLWdvZXR0aW5nZW4uZGU=
Disclaimer: All claims expressed in this article are solely those of the authors and do not necessarily represent those of their affiliated organizations, or those of the publisher, the editors and the reviewers. Any product that may be evaluated in this article or claim that may be made by its manufacturer is not guaranteed or endorsed by the publisher.
Research integrity at Frontiers
Learn more about the work of our research integrity team to safeguard the quality of each article we publish.