- 1Department of Aquatic Ecology and Environmental Biology, Institute for Water and Wetland Research, Radboud University Nijmegen, Nijmegen, Netherlands
- 2Department of Environmental Science, Institute for Water and Wetland Research, Radboud University Nijmegen, Nijmegen, Netherlands
- 3Peel and Maasvallei Regional Water Authority, Venlo, Netherlands
- 4B-WARE Research Centre, Radboud University Nijmegen, Nijmegen, Netherlands
- 5Royal HaskoningDHV, Rotterdam, Netherlands
- 6Community and Conservation Ecology Group, Centre for Ecological and Evolutionary Studies, University of Groningen, Groningen, Netherlands
In wetland soils and underwater sediments of marine, brackish and freshwater systems, the strong phytotoxin sulfide may accumulate as a result of microbial reduction of sulfate during anaerobiosis, its level depending on prevailing edaphic conditions. In this review, we compare an extensive body of literature on phytotoxic effects of this reduced sulfur compound in different ecosystem types, and review the effects of sulfide at multiple ecosystem levels: the ecophysiological functioning of individual plants, plant-microbe associations, and community effects including competition and facilitation interactions. Recent publications on multi-species interactions in the rhizosphere show even more complex mechanisms explaining sulfide resistance. It is concluded that sulfide is a potent phytotoxin, profoundly affecting plant fitness and ecosystem functioning in the full range of wetland types including coastal systems, and at several levels. Traditional toxicity testing including hydroponic approaches generally neglect rhizospheric effects, which makes it difficult to extrapolate results to real ecosystem processes. To explain the differential effects of sulfide at the different organizational levels, profound knowledge about the biogeochemical, plant physiological and ecological rhizosphere processes is vital. This information is even more important, as anthropogenic inputs of sulfur into freshwater ecosystems and organic loads into freshwater and marine systems are still much higher than natural levels, and are steeply increasing in Asia. In addition, higher temperatures as a result of global climate change may lead to higher sulfide production rates in shallow waters.
Introduction: Anaerobiosis and Soil Sulfur Transformations
Although sulfur (S) is one of the six macronutrients for plant growth and low availability of S may therefore limit primary production (Marschner, 1995; Leustek and Saito, 1999), the accumulation of reduced sulfur in sediments of aquatic systems and permanent or riparian wetlands (including estuarine and marine) generally causes physiological toxicity stress for the community involved, including its plants, animals and micro-organisms (Bagarinao, 1992). Unlike the first billion years of life on earth, when sulfide oxidation was an integral part of life generating energy, sulfide accumulation has become much less common as a result of biogenic oxygen production, and sulfide has become toxic to many organisms inhabiting the top layer of soils, including plants (Olsen, 2012). Atmospheric oxygen levels started to increase 2.5 billion years before present (BYBP), and reached levels above 15% since 0.6 BYBP. Oscillations in oxygen and reciprocal oscillations in sulfide levels may even have contributed to mass extinctions (Olsen, 2012).
During flooding and waterlogging of wetland soils, hydrogen sulfide (H2S) is produced as a metabolic end product by prokaryotes that oxidize organic compounds using sulfate as a terminal electron acceptor. This group of dissimilatory sulfate reducers includes both Bacteria (e.g., Desulfovibrio, Desulfobacter) and Archaea (e.g., Archaeoglobus). If the sulfide produced cannot be sufficiently sequestered in the soil by metals such as iron, free (dissolved) sulfide will accumulate. Sulfide concentrations in sediment porewaters show a large range up to 15 mmol L−1 in marine sediments (Bagarinao, 1992). The reduced sulfur compound acts as a potent phytotoxin (equally toxic as cyanide), by inhibiting the activity of cytochrome c oxidase in mitochondria, leading to a subsequent blocking of energy production, and by negatively affecting a range of other metal containing enzymes (Koch et al., 1990; Bagarinao, 1992; Raven and Scrimgeour, 1997). The chemical speciation of sulfide (H2S, HS− and S2−) depends on soil pH (pK1 = 7.2; pK2 = 13.7 for freshwater). Although all forms seem to be equally toxic (Armstrong and Armstrong, 2005), the gaseous H2S will normally prevail over both ionic forms in freshwater systems as the pH of most anaerobic soils is buffered around 6–7 as a result of the HCO−3 - CO2 buffering mechanism, resulting in relative H2S abundances of 95–60%. In marine systems, however, pH is often around 7.5, leading to a relative abundance of only 30% for H2S, and 70% for HS−. As a result of the release of acidic compounds and oxygen from roots, pH in the rhizosphere may, however, be lower than in the bulk soil, and the proportion of H2S consequently be higher.
In marine and brackish ecosystems, sulfate concentrations are 10 to 1000 times higher compared to freshwater systems (Marschner, 1995), stimulating sulfate reducers that play an imminent role in decomposition (Jørgensen, 1982) and concomitant sulfide production. Hence, the role of sulfide as a potential natural toxin in saline sediments has been well-established (Carlson and Forrest, 1982; Ingold and Havill, 1984; Webb and Mendelssohn, 1996; Raven and Scrimgeour, 1997; Koch and Erskine, 2001; Pedersen et al., 2004). Sulfide toxicity may also occur when levels and inputs of sulfur remain unchanged, but increased loading with organic matter boosts sulfate reduction rates by providing electron donors from its decomposition (Jørgensen, 1982; Armstrong and Armstrong, 2001; Ruiz-Halpern et al., 2008; Van der Heide et al., 2012). In many coastal systems worldwide, organic loading has strongly increased as a result of land use change in the catchment of rivers (Ver et al., 1999). In addition, increased inorganic nutrient loading (from rivers, run-off, urbanization, atmospheric deposition) fuels local organic matter production (e.g., Van Beusekom and De Jonge, 2002). This makes sulfide-related questions here even more urgent than in more pristine areas.
As a result of anthropogenic forcing, plants in freshwater wetlands and aquatic systems are facing much higher concentrations of sulfur at a global scale nowadays (Lamers et al., 1998). The emission of sulfur to the atmosphere and airborne inputs of anthropogenically-derived sulfur into freshwater wetlands have increased considerably over the last decades as a result of extensive mining for fossil fuels and associated combustion (Gorham, 1976; Schindler et al., 1980; Benkovitz et al., 1996; Schlesinger, 1997). Although S deposition has decreased in Europe and North America during the last decade as a result of effective legislation, rates are still much higher than natural background levels, and in Asia, South America and South Africa, S emission and deposition rates are still strongly increasing (Shah et al., 2000; Vallack et al., 2001). Moreover, sulfate loading of groundwater has increased due to aerobic oxidation of deposited sulfide minerals as a result of water table lowering for agriculture (Schuurkes et al., 1988; Heathwaite, 1990; Lamers et al., 1998), and from anaerobic oxidation of reduced sulfur compounds by chemolithoautotrophic coupling of sulfide oxidation and nitrate reduction in nitrate-loaded catchments and wetlands (Haaijer et al., 2006; Burgin and Hamilton, 2008; Smolders et al., 2010). As a result of the discharge of this groundwater and run-off from pastures and shores suffering from drought, surface waters have become richer in sulfate too. The S in terrestrial soil and subsoil originates in part from increased anthropogenic airborne inputs (S legacy), but also from natural marine and estuarine deposits in the Quaternary or in earlier periods. In addition, recent hydrological changes such as increased inputs of riverine water to compensate for water shortage in both agricultural areas and nature reserves (Roelofs, 1991; Smolders and Roelofs, 1993; Lamers et al., 1998), as well as the intrusion of seawater (salinization; Fogli et al., 2002; Chambers and Pederson, 2006) have contributed to increased S inputs into freshwater wetlands.
Differential Sensitivity Thresholds for Sulfide
Research on sulfide toxicity and physiological stress originally focused on rice (Oryza sativa) as a crop plant in relation to acid sulfate soils that accumulate high concentrations of sulfide during anaerobiosis (Okajima and Takagi, 1955; Vámos, 1959; Hollis et al., 1972). Seedlings appeared to be particularly sensitive to sulfide (Joshi et al., 1975). Since then, sulfide toxicity has also been reported for many other wetland species in both freshwater and saline systems, with a wide range of threshold levels for different species.
In Table 1, a literature overview is given for sulfide toxicity of different plant species, grouped by ecosystem type, showing the differential threshold levels and ecophysiological responses to sulfide. As high levels of free sulfide are only present in wetland soils (including aquatic systems), dryland species are not represented in this table. In addition, no data are available on phytotoxic effects for macroalgae and phytoplankton. As phytoplankton only occurs in the photic zone of the water column that contains oxygen, sulfide toxicity is very unlikely to play an important role. Sulfide toxicity will be much less common for macroalgae than for vascular plants, because they only possess rhizoids and do not protrude into the anoxic sediment, but are often attached to substrates such as rock and coral. At low tide, however, sulfide may well accumulate under dense mats of macroalgae. As an example, anoxic conditions and high ammonium levels were measured in Cladophora mats, hampering seagrass growth (Hauxwell et al., 2001). Although sulfide was not measured in this study, it can be expected to have led to sulfide accumulation as well, as shown for Ulva mats in coastal lakes (Viaroli et al., 1996). Direct effects of sulfide on macroalgae have, as far as we know, not been tested yet. Algal cover can, however, lead to increased sulfide toxicity to seagrasses (Holmer et al., 2011; Thomsen et al., 2012). Sulfide may only accumulate to high concentrations in the surface water if the water is anoxic and its oxidation is prevented, for instance by the cover of floating-leaved vascular plants such as Eicchornia crassipes, Pistia stratiotes, Lemna spp., and floating ferns such as Salvinia spp. and Azolla spp. Dense layers of these plants effectively block oxygen intrusion from the atmosphere (e.g., Van Kempen et al., 2012). Phytoplankton is lacking in this dark layer, due to photon deficiency.
As can be expected in sulfate-rich environments (particularly when they are permanently submerged), seagrass species are relatively tolerant to sulfide (thresholds generally 2000–6000 μmol L−1), although negative effects on growth rates have also been reported at levels of 200–500 μmol L−1, especially for small species (Table 1). The saltmarsh species Spartina alterniflora is also known to survive high concentrations of sulfide up to 8000 μmol L−1 (Lee, 1999; Van der Heide, unpubl. results), but lower concentrations may already impair its growth (King et al., 1982). For mangroves, Rhizophora seedlings appeared to be more tolerant than those of Avicennia, but adult trees of the latter species tolerate much higher concentrations. The high tolerance of saltmarsh and mangrove species makes sense, as they grow on soils that are rich in both organic electron donors (derived from decomposition of the large flux of litter) and the alternative terminal electron acceptor sulfate. This may also suggest that early-successional species (including a number of seagrass species) may be more sensitive to sulfide than late-successional species, as the latter generally live on sites with higher organic matter accumulation in the sediment. In addition, different ecotypes of the same species can be expected to exist due to strong selection, each adapted to their specific habitat.
Most of the larger freshwater helophyte species such as Phragmites australis and Typha domingensis also show tolerance to relatively high sulfide concentrations (500–1500 μmol L−1; Armstrong et al., 1996; Chambers, 1997; Armstrong and Armstrong, 2001; Adema et al., 2003). Sulfur amendment in order to try to control the unbridled expansion of P. australis in the USA at the expense of other species, led to sulfide concentrations of 1500 μmol L−1, a level that this species demonstrated survival even at higher salinities (Howes et al., 2005).
In contrast, smaller wetland species and aquatic macrophytes show much lower toxicity thresholds between 10 and 250 μmol L−1 (Table 1). Some rootless aquatic macrophytes, growing on highly organic soils, such as Ceratophyllum demersum, tolerate relatively high concentrations up to 500 μmol L−1. Oryza sativa shows intermediate levels of tolerance, although the actual level differs among varieties.
Effects of Experimental Set-Up and Different Field Measurements
Concentrations of dissolved sulfide can be measured colorimetrically, with S2− selective electrodes in immediately fixed and alkalized porewater, in situ using micro-electrodes, or by gas chromatography analysis after gas stripping of acidified porewater. In addition, sulfide-selective optodes, which neither need additional reagents nor consume sulfide, have been developed for direct sulfide measurement (Choi, 1998). As sulfide is easily oxidized and correct sulfide measurements depends on accurate pH measurements for a number of methods, the analytical methods used may show differences in accuracy.
The interpretation of results from literature is strongly confounded by the myriad of methods used in the field and in experiments. For field observations, low sulfide concentrations may also indicate high tolerance to microbial sulfide production due to high oxidation rates supported by oxygen supply from roots. For laboratory tests, the experimental set-up may therefore well-interfere with toxicity levels and attendant effects. As we will discuss, the ability or inability of plants to generate an oxidized rhizosphere strongly determines their sensitivity to reduced phytotoxic compounds including ammonium (NH+4), ferrous iron (Fe2+) and H2S, (Laan et al., 1989, 1991; Lamers et al., 2012). Therefore, great care has to be taken in the interpretation of hydroponic experiments to the actual effect of the suggested stress conditions under natural conditions. To test the potential toxicity of reduced compounds and separate ecophysiological responses from those related to direct anoxia effects, we therefore stress the importance of an experimental set-up using a realistic substrate in which plants are able to potentially realize a protective rhizospheric environment to cope with both primary (anoxia-related) and secondary (toxicity-related) stress during anaerobiosis. Pezeshki (2001), in his review on wetland plant responses to soil flooding, also pleaded for research differentiating between these effects.
On the other hand, the type of soil used in other types of set-up will be very important for the outcome, as this determines the extent of the oxygen sink and diffusion rates. This means that the use of artificial solid substrates, like gels, may also generate experimental artifacts. We therefore suggest using a different approach as an experimental set-up, which includes more realistic edaphic conditions and rhizospheric effects (Figure 1). The actual optimal set-up will depend on the particular questions involved (see, e.g., Van der Heide et al., 2012).
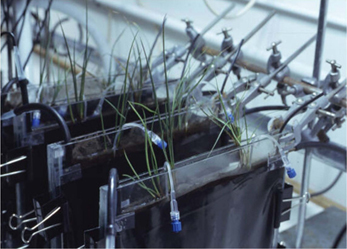
Figure 1. Example of an experimental set-up using rhizotrons showing inflow, outflow, and the tubes of samplers to collect soil porewater (photo: L. Lamers).
Sulfur Uptake and Internal Detoxification
Sulfur concentrations in shoots of terrestrial plants are, on average 30 μmol g−1 (Gruhlke and Slusarenko, 2012), but values may be higher for freshwater wetland plants (35–150 μmol g−1, Van der Welle et al., 2007a,b) and marine plants (100–400 μmol g−1; Holmer and Kendrick, 2013), most probably related to the level of S availability in the different environments, but possibly also as a result of the presence of sulfides in the soil. Sulfate is actively taken up by roots and distributed in the plant, with transport through membranes by proton-sulfate co-transporters driven by a proton gradient (Trust and Fry, 1992; Leustek and Saito, 1999). Studies on the abundance ratios of natural S isotopes in Spartina alterniflora revealed that most of the sulfate in these marsh plants was derived from sulfide that had partly been oxidized within the plant (Carlson and Forrest, 1982). For seagrasses including Zostera marina and Thalassia testudinum, isotopic analysis revealed that 50–96% of the S in plants was derived from different sediment sulfides (Frederiksen et al., 2006; Holmer et al., 2009), even when dissolved sulfide concentrations were low (Holmer and Kendrick, 2013). In small seagrass species, sulfur easily enters the roots, and is transported through rhizomes and stems into the leaves, but in taller species its transport seems to be more limited (Holmer and Kendrick, 2013). It is therefore quite probable that in addition to sulfide oxidation in the rhizosphere and subsequent sulfate uptake, gaseous H2S is transported to the leaves through the aerenchyma, especially during the night (Pedersen et al., 2004; Holmer and Kendrick, 2013). As the uptake of sulfate after rhizospheric and internal sulfide oxidation generates similar δ34S values in plants as direct sulfide uptake, it may be difficult to differentiate between both uptake pathways (Trust and Fry, 1992).
Studies related to S uptake and metabolism have generally been conducted with terrestrial plants species, and differences between sulfide and sulfate uptake and their metabolic pathways are not entirely clear yet. The internal toxicity of sulfide will depend on the species' ability to rapidly metabolize this compound to thiols (organosulfur compound, chemical formula R-SH) such as the amino acids cysteine and methionine and, subsequently, glutathione which is the most abundant thiol in plants (Trust and Fry, 1992; Leustek and Saito, 1999; Hawkesford and De Kok, 2006; Nakamura, 2009). Next, S may be built in a range of different plant tissues. A small number of estuarine plant species, including Spartina spp. and Wollastonia biflora also produce dimethylsulfoniopropionate (DMSP) from methionine, like a number of marine algal species (Stefels, 2000). This compound may act as a constitutive osmoticum, although its concentration in Spartina spp. does not respond to changes in salinity. Alternatively, the production may also provide a mechanism to keep cysteine and methionine levels sufficiently low, and redistribute nitrogen to other amino acids (Stefels, 2000; Otte et al., 2004). In addition, a number of secondary metabolites contain sulfur, including antibiotic substances and odorous compounds (giving flavor to garlic, onions and cabbage) (Leustek and Saito, 1999). The capacity to internally detoxify sulfide is therefore related to cysteine synthesis, catalyzed by the enzyme O-acetylserine(thiol)lyase (OAS-TL) that is present in cytosol, plastids and mitochondria, and an as yet unknown other detoxifying mitochondrial mechanism (Birke et al., 2012). Lee (1999) even hypothesized that low sulfide concentrations might be used by plants to generate energy in mitochondria, similar to the process in microbes and animals. In addition, there is a range of reactive sulfur species next to thiols, such as disulfide-S-oxides (RS(O)xSR), sulfenic acids (RSOH), and thiyl radicals (RS) (Gruhlke and Slusarenko, 2012). Although it seems likely that plant hemoglobin (Hb; Igamberdiev et al., 2005) and other metalloproteins may be related to internal sulfide detoxification, similar to Hb in vertebrates and invertebrates (Beauchamp et al., 1984; Weber and Vinogradow, 2001), this is yet to be studied. Next to the metabolic conversion of sulfide, the emission of sulfide from plants, as shown during the exposition to high sulfide concentrations (roots) or SO2 concentrations (shoots) (Trust and Fry, 1992), may offer protection. For Spartina alterniflora it has been shown that leaves show substantial loss of DMSP during high tide (Pakulski and Kiene, 1992), which provides a mechanism to dissipate excess S. Additionally, the loss of dimethylsulfide (DMS), a volatile metabolite of DMSP, may also offer protection against high S accumulation in a number of estuarine plants (Stefels, 2000).
Effects on Nutrient Uptake
Sulfide is known to be able to hamper plant nutrient uptake, which is not surprising given its basic disturbance of cell metabolism and energy transfer. In addition, root loss due to die-off and concomitantly decreased root to shoot ratios lead to an unbalanced nutrient uptake. Sulfide can impair the uptake of nitrogen (N) (Koch et al., 1990), phosphorus (Van der Heide et al., 2012) and Fe (Smolders and Roelofs, 1996; Armstrong and Armstrong, 2005). Depending on the type of nutrient limitation, growth rates may be impaired, while Fe deficiency may lead to lower photosynthetic rates as a result of hampered chlorophyll synthesis. The effects of sulfide on the uptake of Fe and other metals can, however, also be the result of precipitation (Lamers et al., 2012). Although MgS is highly reactive in water and MgSO4 is quite soluble, it has been shown that Mg and Ca concentrations in acid sulfate soils are generally undersaturated and governed by cation exchange rather than by their activities. It is well-known that this phenomenon can lead to Mg and Ca deficiency of Oryza sativa growing on these soils (Tanaka et al., 1968; Moore and Patrick, 1989). Next, acid production as a result of sulfide oxidation can lead to loss of Mg and Ca from soil cation exchange sites in the rhizosphere, and concomitant lower availability of these macro-ions. For field measurements, however, negative correlations between nutrient uptake and sulfide do not prove sulfide toxicity, as salinity, soil organic matter concentration, and oxygen and nutrient availability are often changing as well along the gradient. Effects of sulfide on soil biogeochemistry affecting plant performance and fitness will be explained further in sections below.
An interesting, but as yet unknown mechanism of sulfide toxicity on plant nutrient uptake might act through its effects of mycorrhizal activity. Although lead sulfides are known to seriously decrease the vitality of ectomycorrhizae (Fomina et al., 2005), the effect of free sulfide on mycorrhizae, and thereby on plant fitness, remains to be elucidated.
Physico-Chemical Protection: Sequestration in the Soil and Volatilization
Even with high rates of sulfate reduction in the field, the accumulation of dissolved sulfide and its phytotoxic effects can be moderate, or largely absent due to metal sequestration, mainly by Fe. For Fe this leads to the formation of FeS and FeS2 (pyrite), detoxifying sulfide (Figure 2; Smolders et al., 1995; Lamers et al., 2002b; Van der Welle et al., 2006, 2007a; Marbà et al., 2007). This mechanism was proposed for Spartina alterniflora already in 1982 by King et al., who showed for marshes on the barrier island Sapelo (GA, USA) that in spite of similar sulfate reduction rates, sulfide accumulation showed large variations related to Fe availability. In the same way, discharge of Fe-rich groundwater in wetlands and aquatic systems effectively protects against sulfide toxicity (Lamers et al., 2002a). In marine systems, where sediment Fe concentrations are generally low, the experimental addition of Fe has been shown to counteract sulfide toxicity to seagrass (Posidonia oceanica) in a similar way (Holmer et al., 2003, 2005; Marbà et al., 2007; Ruiz-Halpern et al., 2008). However, even if total Fe concentrations (i.e., in destruates) in the soil are high, H2S accumulation may still occur if the amorphous Fe pool is sulfide-saturated by present or past high S reduction rates. This is clearly indicated by low total Fe:S ratios of the soil. Other metals, including Mn, Zn, Hg, Pb, Cd and Cu, may also precipitate sulfide, but are quantitatively much less important in S biogeochemistry (Bagarinao, 1992). Finally, the accumulation of dissolved sulfide can also be toned down by the activity of microbial communities using nitrate or ferric iron as electron acceptor (Friedrich et al., 2001; see above).
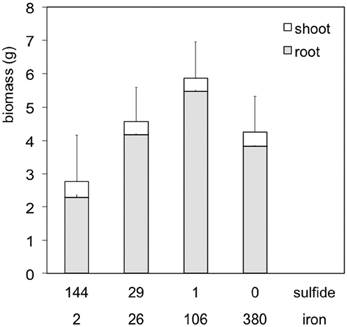
Figure 2. Interacting effects of dissolved sulfide and iron (concentrations in μmol L−1) in the soil porewater on biomass production of Caltha palustris. Although both compounds may be toxic for plant growth, they detoxify one another as a result of FeSx precipitation (quadratic correlation, p = 0.014). Adapted from Van der Welle et al. (2006).
As H2S is a gas, not only sequestration in the soil but also volatilization to the atmosphere determines sulfide concentrations in sediments (Bagarinao, 1992). In addition, sulfide can be methylated in organic marine and freshwater sediments, and released as dimethylsulfide and methanethiol into the atmosphere (Lomans et al., 2002).
Biological Protection: Radial Oxygen Loss From Roots
Many flooded or waterlogged plants show radial oxygen loss (ROL) from their roots, and the level and pattern of ROL is determined by photosynthetic rate, root architecture and root morphology (Armstrong, 1979; Jackson, 1985; McKee et al., 1988; Laan et al., 1991; Jackson and Armstrong, 1999; Visser et al., 2003; Frederiksen and Glud, 2006; Visser and Bögemann, 2006; Voesenek et al., 2006; Deborde et al., 2008). During nighttime, sulfide intrusion into roots and rhizomes is highest (Borum et al., 2005). Rhizosphere oxidation provides an obvious potential defense mechanism against the toxicity of reduced components such as sulfide (Pitts et al., 1972; Mendelssohn and McKee, 1988; Armstrong et al., 1996; Smolders and Roelofs, 1996; Hemminga, 1998; Armstrong and Armstrong, 2001, 2005; Holmer and Storkholm, 2001; Deborde et al., 2008), provided that soil aerobic microbial respiration and concomitant consumption of oxygen do not counteract this effect.
Spatial differences in oxygen release can not only be attributed to differences in aerenchyma structure, but also to lignine and/or suberine in the epidermis of the roots of different species, preventing loss of all oxygen in the upper soil layer. As an example, the rush species Juncus acutiflorus is able to oxidize its rhizosphere, even for the deeper roots, unlike the sedge species Carex disticha (Lamers et al., 2012; Figure 3). Although both species did release oxygen from their roots, the relatively high ROL in the top layer solely proved to be insufficient to detoxify sulfide for C. disticha, leading to almost complete die-off of deeper roots (Lamers, 2001). In contrast, J. acutiflorus was able to completely oxidize its rhizosphere, even in deeper layers where a strong O2 demand results from both soil respiration and S oxidation. Observed root loss correlated well with the differences in spatial ROL patterns for both species (Lamers et al., 2012). Sulfide is even known to induce additional suberization (Armstrong and Armstrong, 2005), which can be either an advantage or a disadvantage depending on the location in the roots. This indicates that the specific pattern of ROL, rather than its overall rate, determines the sensitivity of plant species to reduced phytotoxins such as sulfide. For sufficient ROL, the meristematic oxygen content fuelled by photosynthesis during daytime must also be high enough to prevent oxygen depletion by respiration during nighttime. Particularly at higher temperatures, e.g., as a result of climate change in shallow waters, high respiration rates could exceed photosynthetic O2 production (Greve et al., 2003).
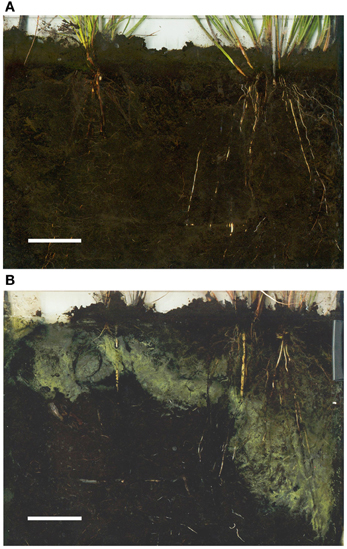
Figure 3. Rhizotron scans for a control (A) and 250 μmol L−1 (B) sulfide treatment (darker soil due to FeSx deposits). The oxidation potential is clearly visible from the yellow-gray halo of metallic sulfur and other products of oxidation. In each scan, Carex disticha is positioned left (only shallow S oxidation halos) and Juncus acutiflorus right (deep S oxidation halos). On (B), two white soil moisture samplers are visible. Bars represent 40 mm. Adapted after Lamers (2001) and Lamers et al. (2012).
Sulfide Oxidizers and Soil Fauna as Rhizospheric Guards
As H2S is readily taken up and causes root toxicity, in contrast to sulfate, the activity of sulfide oxidizing prokaryotes in the rhizosphere is expected to influence both uptake rates and toxicity of S. As the spontaneous chemical oxidation of sulfide is more than 10,000 times slower than biological catalysis (Jørgensen and Revsbech, 1983; Millero, 1986), this rhizosperic sulfur oxidation by prokaryotes (see Friedrich et al., 2001; Ghosh and Roy, 2006) is essential, and the community should comprise large numbers of these organisms living in symbiosis with plants (oxygen supply as a “reward” for detoxification). Sulfur oxidizing microorganisms may be either free living inside or on top of the sediment, in the surface water layer, or associated with roots. Sulfide oxidizers comprise chemolithoautotrophic Proteobacteria such as Beggiatoa and Thiobacillus, photolithoautotrophic bacteria (e.g., Rhodovulum, Chromatium), and chemolitho-autotrophic Archaea (e.g., Sulfolobales) (Ghosh and Dam, 2009). Sulfide-oxidizing prokaryotes may be expected to live inside the root and rhizome aerenchyma as sulfide and oxygen are both present, and Beggiatoa presence has indeed been shown inside the rhizomes of seagrass (Zostera marina) (Elliott et al., 2006). The oxidation of sulfide in the rhizosphere will, however, also generate acidity in the rhizosphere, slowing down sulfate reduction (Starkey, 1966; Connell and Patrick, 1968) even in the layers beyond the influence of radial oxygen loss by proton diffusion. In this way a second “protective shell” against the adverse effects of sulfate reduction is generated. In addition, the availability of Fe, mobilized by partial FeSx oxidation, may be higher as a result of lower pH values even at a higher redox potential, although a large part will re-precipitate with sulfide. On the other hand, strong acidification of the rhizosphere may also be detrimental to plant roots, e.g., via NH+4 toxicity (Lucassen et al., 2003; Van den Berg et al., 2005). The outcome of these different rhizospheric processes is determined by the interplay between the rates of ROL, oxygen consumption, sulfide oxidation and acid buffering in the soil.
In addition, next to prokaryotes, a range of eukaryote animal species including invertebrates and fish, have been shown to be able to oxidize sulfide in their mitochondria (whether or not ancient endosymbionts; Gray et al., 1999; Emelyanov, 2003; Olsen, 2012), or by sulfide-oxidizing prokaryotes on internal organs, generating energy (Bagarinao, 1992; Ghosh and Dam, 2009). As sediment bioturbation leads to higher rates of oxygen intrusion, sulfate reduction rates are suppressed even though the availability of readily decomposable organic matter may increase, as was shown for the burrow-forming marine polychaete Arenicola marina (lugworm; Nielsen et al., 2003). This not only leads to lower concentrations and toxicity of sulfide, but also to higher availability of Fe3+ as an alternative electron acceptor (Nielsen et al., 2003). For this effect, however, the level of bioturbation has to be strong enough to affect rhizospheric sulfide concentrations, especially if organic matter is accumulating in burrows as a result of foraging. Even though fiddler crabs (Uca spp.) were able to oxidize the rhizosphere of young mangrove plants (Laguncularia racemosa), sulfide levels remained similar (Smith et al., 2009).
Recently, it was shown in tropical seagrass systems that mutualisms related to rhizospheric S biogeochemistry can be even more complex. Lucinid bivalves containing sulfide-oxidizing symbionts appear to globally occur in tropical and subtropical seagrass meadows (Fisher and Hand, 1984; Van der Heide et al., 2012) and seem strongly associated with these systems ever since seagrasses evolved in the Cretaceous (Van der Heide et al., 2012 and references herein). These lucinids were experimentally shown to play an essential role in seagrass sulfide tolerance, as the sulfide oxidizing prokaryotes living within the gills of the bivalves detoxify sulfide, stimulating seagrass production (Figure 4; Van der Heide et al., 2012). ROL by the seagrass species Zostera noltii was only able to reduce the added sulfide concentration from 2700 to 2200 μmol L−1, whereas the inclusion of the bivalves led to very low sulfide concentrations of only 15 μmol L−1. Simultaneously, the sulfide oxidizers and their host bivalves benefit from the oxygen supplied by ROL from seagrass, and from its organic matter production. It is very likely that similarly elegant mutualistic symbioses involving multiple species have evolved during evolution enabling other plant species to thrive and have higher fitness under sulfidic conditions. We therefore believe that inclusion of plant-symbiont interactions may be a step forward in our ability to explain sulfide tolerance rather than traditional plant physiology alone.
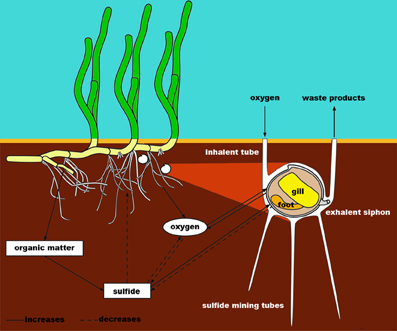
Figure 4. Sulfide-driven coevolution: tripartite mutualistic interactions among seagrasses, lucinid bivalves and sulfide oxidizing bacteria in their gills generate a higher fitness of all species involved under sulfidic conditions. See text for explaining mechanisms. Adapted after Van der Heide et al. (2012).
Indirect Toxicity During Drought of Sulfidic Wetlands
Periodic water level fluctuations and severe droughts lead to profound biogeochemical changes in wetlands, due to the strong temporal variation in oxygen concentrations in the soil. Sulfides (free sulfide and metal sulfides) may become toxic in an indirect way in these riparian systems, as the aerobic microbial and chemical oxidation of sulfides generates sulfuric acid (Smolders et al., 2006; Lamers et al., 2012). The actual balance between acid producing and acid consuming processes determines whether this acidification (proton production) leads to an actual drop in pH (increased proton concentration in the porewater). The acid neutralizing capacity (ANC) of soils is determined by the successive extent of bicarbonate and carbonate buffering, the exchange of base cations such as calcium and magnesium at cation binding sites of organic matter and clay, and dissolution of Fe and Al compounds (Scheffer and Schachtschabel, 2002). The total S/(Ca + Mg) ratio of soils may provide an easy proxy to determine the acidification potential of soils during drought (Lucassen et al., 2002). The strong acidification of coastal acid sulfate soils (high concentrations of iron sulfides, low ANC) during droughts, leading to pH values below 4 and concomitant mobilization of aluminum and iron, is a well-known phenomenon, and a problem for rice production and shrimp farming (Dent, 1986; Sammut et al., 1995). Especially in estuarine systems such as marshes, but also in S-rich freshwater systems, massive plant die-off during drought may therefore not only be caused by water deficiency, but also by strong acidification. For the marsh plant Spartina spp., it has been shown that the combination of proton toxicity and concomitant mobilization of Al may have contributed to die-off events during droughts (McKee et al., 2004). It has been suggested that acid-tolerant arbuscular mycorrhizal fungi may play an important role in the establishment of pioneer species (grasses, forbs and shrubs) on dry acid sulfate soils (Maki et al., 2008).
Other Biogeochemical Processes Related to Sulfide Affecting Plant Growth
The anthropogenically increased availability of sulfate as an electron acceptor in anaerobic freshwater wetland soils potentially results in eutrophication (Lamers et al., 1998). This is not only caused by increased decomposition and nutrient mineralization rates as a result of the increased availability of sulfate as an electron acceptor, but also by the accumulation of sulfide that lowers phosphate binding to iron oxides and iron hydroxide, thereby increasing phosphate availability in the soil (Ohle, 1954; Sperber, 1958; Caraco et al., 1989; Lamers et al., 1998). Enhanced concentrations of ammonium and phosphate may, however, also result from increased decomposition rates due to greater availability of sulfate as an alternative electron acceptor (Roelofs, 1991; Smolders and Roelofs, 1993; Koerselman et al., 1993; Lamers et al., 1998, 2002b; Zak et al., 2006). For Thalassia hemprichii, a seagrass species, it was shown that 80% of its P demand was covered by the activity of sulfate reducers (Holmer et al., 2001). Oxidized sulfur may also be recycled and re-reduced in anaerobic parts of the soil, stimulating decomposition. Under fluctuating oxygen conditions, e.g., in riparian wetlands, reduction and oxidation will therefore alternate (Lucassen et al., 2005).
Sulfate reduction rates can be governed either by the availability of electron donors such as acetate and lactate produced by decomposition of organic matter, or by the availability of sulfate (Lamers et al., 2002b). If, however, high concentrations of a more favorable electron acceptor are available, sulfate-reducing prokaryotes may be partly or completely outcompeted. Wetlands receiving high nitrate loads through discharge of groundwater originating from arable land and fertilized pastures, show low iron and sulfate reduction rates, with concomitantly low phosphate mobilization rates (Lucassen et al., 2004).
In semi-aquatic plants, sulfide toxicity was found to be less pronounced at a higher nutrient availability, possibly as a result of dilution effects by increased growth and increased ROL (Geurts et al., 2009) suggesting that eutrophication may be “masking” sulfide toxicity in polluted areas. For submerged macrophytes, however, eutrophication is expected to aggravate the effects of sulfide, as increased growth of algae and cyanobacteria will directly impair their photosynthetic rates and ability to oxidize the rhizosphere. Filamentous mats of algae on seagrass meadows, resulting from eutrophication, have also been shown to lead to reduced oxygen concentrations in the sediment and increased S uptake and sulfide toxicity in seagrass (Holmer and Nielsen, 2007).
Sulfide and Interspecific Interactions: Competition, Facilitation
Field observations in sulfate-polluted freshwater wetlands suggest that the loss of biodiversity and dominance of a small number of highly competitive plant species may not only be attributed to sulfate-induced eutrophication, but may additionally, or perhaps primarily be triggered by sulfide toxicity (Lamers et al., 2002a). The differential toxicity of hydrogen sulfide provides an additional explanation for changes in competitive strength leading to severe changes in vegetation development in sulfur-loaded wetlands, or in naturally S-rich wetlands that receive higher loads of organic matter. In addition, differences in sulfide accumulation along a gradient may explain vegetation gradients next to salinity effects, e.g., in marshes where Salicornia spp. live at the lower, marshes, and high salt marsh species such as Pucinellia maritime, Atriplex patula and Festuca rubra inhabit less sulfidic spots (Ingold and Havill, 1984). Although Spartina alternifolia lives at higher marshes than Salicornia, the organic content of its sediment is generally higher, potentially leading to higher sulfide accumulation. In freshwater systems, interspecific competition between macrophytes has been shown to depend on the interplay between sulfide and iron in sediments (Van der Welle et al., 2007a). Multiple positive feedback loops therefore increase and stabilize both toxicity and non-toxicity states (Figure 5).
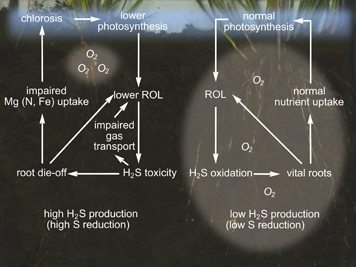
Figure 5. Conceptual model showing positive feedback loops on plant-rhizophere processes under scenarios of sulfide toxicity (left) and non-toxicity (right). See text for further explanation. Unlike in other studies, impaired uptake of N or Fe was not found in this study. Impaired gas transport by sulfide has been shown by Armstrong et al. (1996). Sulfide oxidation is carried out by free-living prokaryotes in sediment and water, symbiotic prokaryotes of roots and soil fauna, and mitochondrial metabolism in soil fauna and plants.
The first constraint on the establishment and competitive strength of wetland plants in sulfur-rich areas, naturally or anthropogenically enhanced, may therefore be sulfide toxicity. As a result, plants that are able to provide their root apices with oxygen without losing all oxygen along the root surface, such as a number of larger graminoids, have a strong competitive advantage, especially if high sulfide oxidation rates are sustained by microbial activity in soils and soil macrofauna. If the growth rate is high, the toxicity effects may be “diluted” and ROL is supported by high photosynthetic rates of the highly competitive species. As a result of these feedbacks, eutrophication and sulfide accumulation in concert may rapidly lead to vegetation changes. From their differential responses to sulfide, Li et al. (2009) argued that the undesirable strong expansion of Typha in the Florida Everglades, at the expense of Cladium, could partially be explained by the high levels of sulfide (250–375 μmol L−1) in this region. These resulted from a combination of high rates of sulfate reduction and low levels of iron to sequester the produced sulfide. For dune slacks it was hypothesized that elevated sulfide concentrations in combination with higher nutrient levels induce a shift to highly productive Phragmites stands (Adema et al., 2003). In a recent study on the biogeochemical drivers of species composition in a groundwater-fed freshwater wetland, sulfide appeared to be the most important explaining variable (Simkin et al., 2013). However, in addition to gaining a higher competitive strength, sulfide-detoxifying plant species might also act as ecosystem engineers (sensu Jones et al., 1994) by their facilitation of sulfide-sensitive plants, provided that the latter group is not outcompeted for light by fast-growing species. However, high sulfide levels, in addition to those of other phytotoxins, may have contributed to the large scale Phragmites die-back in wetlands loaded with organic compounds (Armstrong and Armstrong, 2001), and to large-scale seagrass die-back events (Carlson et al., 1994; Terrados et al., 1999; Borum et al., 2005). Such massive die-off events have also been shown in constructed wetlands receiving high organic fluxes and showing high sulfide concentrations (above 1000 μmol L−1; Wiessner et al., 2008).
Global Effects on Wetlands
The risks of sulfide toxicity are an important issue at a global scale, as sulfur concentrations have risen in many freshwater waters and wetlands, including natural vegetation types and rice paddies, due to high anthropogenic S emissions (Smith et al., 2011) and geochemical oxidation processes including the effect of nitrate pollution (Smolders et al., 2010). Although global emissions decreased between 1970 and 2000 due to legislation, they are now increasing significantly again due to the high S emissions of fast-developing regions such as Asia (particularly China) where SO2 emissions, as a result of the large-scale use of coal as a fuel, may soon equal the combined emissions of North America and Europe (Shah et al., 2000; Smith et al., 2011). In addition, salinization of coastal freshwater wetlands due to the intrusion of saline groundwater or surface water, and salinization due to the increased frequency of drought episodes in more arid regions increase the risk of sulfide-related vegetation changes during anaerobiosis. To determine the exact causes of salinization on vegetation changes, it is, however, important to experimentally test the effects of sulfide and NaCl separately and in concert. In saline systems (in which sulfate is normally not limiting), increased organic loads will stimulate sulfate reduction rates and lead to higher sulfide levels, especially if temperatures become higher (in shallow waters) as a result of global change (Hoffle et al., 2011; Holmer et al., 2011). Accumulated FeSx in riparian wetlands will massively become oxidized to sulfate during drought (Lucassen et al., 2002), which is prone to renewed reduction during flooding. Even in soils that had not been flooded for more than 10 years, an unexpected diversity of sulfate reducers still appeared to be present and become active after one or two weeks of anaerobiosis (Lamers et al., 1998; Miletto et al., 2008). This shows that the microbial community is very persistent with respect to S biogeochemistry, and able to resuscitate although they have to be classified as “delayed responders” (sensu Placella et al., 2012). As a result, the S legacy of a soil is expected to contribute to sudden die-off of plants in riparian wetlands during anaerobic events.
General Conclusion
As our overview shows that even low concentrations of sulfide are able to 1) affect the ecophysiological functioning individual plants, 2) affect plant competition and facilitation, 3) influence complex rhizospheric mutualisms, and 4) interact with nutrient biogeochemistry, it is clear that sulfide can be a strong driver of ecosystem processes and functioning, also in relation to changing global S balances. Future research should include interactions between plants, microbial communities, soil fauna and soil chemistry, to fully understand and explain differences among plant, vegetation and ecosystem responses to sulfide.
Conflict of Interest Statement
The authors declare that the research was conducted in the absence of any commercial or financial relationships that could be construed as a potential conflict of interest.
Acknowledgments
The authors would like to thank Dr. M. A. Vile for her valuable comments on an earlier version of the manuscript, and Mr. J. Eygensteyn for his help with chemical analyses.
References
Adema, E. B., Van Gemerden, H., and Grootjans AP. (2003). Is succession in wet calcareous dune slacks affected by free sulfide? J. Veget. Sc. 14, 153–162. doi: 10.1111/j.1654-1103.2003.tb02140.x
Armstrong, J., AfreenZobayed, F., and Armstrong, W. (1996). Phragmites die-back: sulphide and acetic acid-induced bud and root death, lignifications, and blockages within aeration and vascular systems. New Phytol. 134, 601–614. doi: 10.1111/j.1469-8137.1996.tb04925.x
Armstrong, J., and Armstrong, W. (2001). An overview of the effects of phytotoxins on Phragmites australis in relation to die-back. Aquat. Bot. 69, 251–268. doi: 10.1016/S0304-3770(01)00142-5
Armstrong, J., and Armstrong, W. (2005). Rice: sulfide-induced barriers to root radial oxygen loss, Fe2+ and water uptake, and lateral root emergence. Ann. Bot. Lond. 96, 625–638. doi: 10.1093/aob/mci215
Armstrong, W. (1979). Aeration in higher plants. Adv. Bot. Res. 7, 225–332. doi: 10.1016/S0065-2296(08)60089-0
Armstrong, W., and Boatman, D. J. (1967). Some field observations relating the growth of bog plants to conditions of soil aeration. J. Ecol. 55, 101–110. doi: 10.2307/2257719
Bagarinao, T. (1992). Sulfide as an environmental factor and toxicant – Tolerance and adaptations in aquatic organisms. Aquat. Toxicol. 24, 21–62. doi: 10.1016/0166-445X(92)90015-F
Beauchamp, R. O., Bus, J. S., Popp, J. A., Boreiko, C. J., Andjelkovich, D. A., and Leber, P. (1984). A critical review of the literature on hydrogen sulfide toxicity. Crit. Rev. Toxicol. 13, 25–97. doi: 10.3109/10408448409029321
Benkovitz, C. M., Scholtz, M. T., Pacyna, J., Tarrason, L., Dignon, J., Voldner, E. C., et al. (1996). Global gridded inventories of anthropogenic emissions of sulfur and nitrogen. J. Geophys. Res. 101, 29239–29253. doi: 10.1029/96JD00126
Birke, H., Haas, F. H., De Kok, L. J., Balk, J., Wirtz, M., and Hell, R. (2012). Cysteine biosynthesis, in concert with a novel mechanism, contributes to sulfide detoxification in mitochondria of Arabidopsis thaliana. Biochem. J. 445, 275–283.
Borum, J., Pedersen, O., Greve, T. M., Frankovich, T. A., Zieman, J. C., Fourqurean, J. W., et al. (2005). The potential role of plant oxygen and sulphide dynamics in die-off events of the tropical seagrass, Thalassia testudinum. J. Ecol. 93, 148–158. doi: 10.1111/j.1365-2745.2004.00943.x
Burgin, A. J., and Hamilton, S. K. (2008). NO3−-driven SO42− production in freshwater ecosystems: implications for N and S cycling. Ecosystems 11, 908–922. doi: 10.1007/s10021-008-9169-5
Caraco, N. F., Cole, J. J., and Likens, G. E. (1989). Evidence for sulphate-controlled phosphorus release from sediments of aquatic systems. Nature 341, 316–318 doi: 10.1038/341316a0
Carlson, P. R. Jr., and Forrest, J. (1982). Uptake of dissolved sulfide by Spartina alterniflora: evidence from natural sulfur isotope abundance ratios. Science 7, 633–635. doi: 10.1126/science.216.4546.633
Carlson, P. R., Yarbro, L. A., and Barber, T. R. (1994). Relationship of sediment sulfide to mortality of Thalassia testudinum in Florida Bay. Bull. Mar. Sci. 54, 733–746.
Chambers, R. M. (1997). Porewater chemistry associated with Phragmites and Spartina in a Connecticut tidal marsh. Wetlands 17, 360–367. doi: 10.1007/BF03161425
Chambers, R. M., and Pederson, K. A. (2006). Variation in soil phosphorus, sulfur, and iron pools among south Florida wetlands. Hydrobiologia 569, 63–70. doi: 10.1007/s10750-006-0122-3
Choi, M. M. F. (1998). Fluorimetric optode membrane for sulfide detection. Analyst 123, 1631–1634. doi: 10.1039/A801702F
Connell, W. E., and Patrick, W. H. Jr., (1968). Sulfate reduction in soil: effects of redox potential and pH. Science 159, 86–87. doi: 10.1126/science.159.3810.86
Deborde, J., Gwenael, A., Mouret, A., Jezequel, D., Thouzeau, G., Clavier, J., et al. (2008). Mar. Ecol. Prog. Ser. 355, 59–71. doi: 10.3354/meps07254
Dent, D. (1986). Acid Sulphate Soils: a Baseline for Research and Development. Wageningen: ILRI Publ.
Elliott, J. K., Spear, E., and Wyllie-Echeverria, S. (2006). Mats of Beggiatoa bacteria reveal that organic pollution from lumber mills inhibits growth of Zostera marina. Mar. Ecol. Evol. Persp. 27, 372–380. doi: 10.1111/j.1439-0485.2006.00100.x
Emelyanov, V. V. (2003). Mitochondrial connection to the origin of the eukaryotic cell. Eur. J. Biochem. 270, 1599–1618. doi: 10.1046/j.1432-1033.2003.03499.x
Fisher, C. R., and Hand, S. C. (1984). Chemoautotrophic symbionts in the bivalve Lucina floridana from seagrass beds. Biol. Bull. 167, 445–459. doi: 10.2307/1541289
Fogli, S., Marchesini, R., and Gerdol, R. (2002). Reed (Phragmites australis) decline in a brackish wetland in Italy. Mar. Environ. Res. 53, 465–479. doi: 10.1016/S0141-1136(02)00091-0
Fomina, M. A., Alexander, I. J., Colpaert, J. V., and Gadd, G. M. (2005). Solubilization of toxic metal minerals and metal tolerance of mycorrhizal fungi. Soil Biol. Biochem. 37, 851–866. doi: 10.1016/j.soilbio.2004.10.013
Frederiksen, M. S., Holmer, M., and Perez, M., Invers, O., Ruiz, J. M., and Knudsen, B. B. (2008). Effect of increased sediment sulfide concentrations on the composition of stable sulfur isotopes (delta S-34) and sulfur accumulation in the seagrasses Zostera marina and Posidonia oceanica. J. Exp. Mar. Biol. Ecol. 358, 98–101.
Frederiksen, M. S., and Glud, R. N. (2006). Oxygen dynamics in the rhizosphere of Zostera marina: a two-dimensional planar optode study. Limnol. Oceanogr. 51, 1072–1083. doi: 10.4319/lo.2006.51.2.1072
Frederiksen, M. S., Holmer, M., Borum, J., and Kennedy, H. (2006). Temporal and spatial variation of sulfide invasion in eelgrass (Zostera marina) as reflected by its sulfur isotopic composition. Limnol. Oceanogr. 51, 2308–2318. doi: 10.4319/lo.2006.51.5.2308
Friedrich, C. G., Rother, D., Bardischewsky, F., Quentmeier, A., and Fischer, J. (2001). Oxidation of reduced inorganic sulfur compounds by bacteria: emergence of a common mechanism? Minireview. Appl. Environ. Microb. 67, 2873–2882. doi: 10.1128/AEM.67.7.2873-2882.2001
Geurts, J. J. M., Sarneel, J. M., Willers, B. J. C., Roelofs, J. G. M., Verhoeven, J. T. A., and Lamers, L. P. M. (2009). Interacting effects of sulphate pollution, sulphide toxicity and eutrophication on vegetation development in fens: a mesocosm experiment. Environ. Pollut. 157, 2072–2081. doi: 10.1016/j.envpol.2009.02.024
Ghosh, W., and Dam, B. (2009). Biochemistry and molecular biology of lithotrophic sulfur oxidation by taxonomically and ecologically diverse bacteria and archaea. FEMS Microbiol. Rev. 33, 99–1043. doi: 10.1111/j.1574-6976.2009.00187.x
Ghosh, W., and Roy, P. (2006). Ubiquitous presence and activity of sulfur-oxidizing lithoautotrophic microorganisms in the rhizospheres of tropical plants. Curr. Sci. 91, 159–161.
Goodman, J. L., Moore, K. A., and Dennison, W. C. (1995). Photosynthetic responses of eelgrass (Zostera marina L.) to light and sediment sulfide in a shallow barrier island lagoon. Aquat. Bot. 50, 37–47. doi: 10.1016/0304-3770(94)00444-Q
Gorham, E. (1976). Precipitation and its influence upon aquatic ecosystems – overview. Water Air Soil Poll. 6, 457–481. doi: 10.1007/BF00182886
Gray, M. W., Burger, G., and Lang, B. F. (1999). Mitochondrial evolution. Science 283, 1476–1481. doi: 10.1126/science.283.5407.1476
Greve, T. M., Borum, J., and Pedersen, O. (2003). Meristematic oxygen variability in eelgrass (Zostera marina). Limnol. Oceanogr. 48, 210–216. doi: 10.4319/lo.2003.48.1.0210
Grootjans, A. P., Van den Ende, F. P., and Walsweer, A. F. (1997). The role of microbial mats during primary succession in calcareous dune slacks: an experimental approach. J. Coast. Cons. 3, 95–102. doi: 10.1007/BF02908184
Gruhlke, M. C. H., and Slusarenko, A. J. (2012). The biology of reactive sulfur species (RSS). Plant Physiol. Biochem. 59, 98–107. doi: 10.1016/j.plaphy.2012.03.016
Haaijer, S. C. M., Van der Welle, M. E. W., Schmid, M. C., Lamers, L. P. M., Jetten, M. S. M., and Op den Camp, H. J. M. (2006). Evidence for the involvement of betaproteobacterial thiobacilli in the nitrate-dependent oxidation of iron sulfide minerals. FEMS Microbiol. Ecol. 58, 439–448. doi: 10.1111/j.1574-6941.2006.00178.x
Hauxwell, J., Cebrián, J., Furlong, C., and Valiela, I. (2001). Macroalgal canopies contribute to eelgrass (Zostera marina) decline in temperate estuarine ecosystems. Ecology 82, 1007–1022.
Hawkesford, M. J., and De Kok, L. J. (2006). Managing sulphur metabolism in plants. Plant Cell Environ. 29, 382–395. doi: 10.1111/j.1365-3040.2005.01470.x
Heathwaite, A. L. (1990). The effect of drainage on nutrient release from fen peat and its implications for water quality - a laboratory simulation. Water Air Soil Poll. 49, 159–173. doi: 10.1007/BF00279518
Hemminga, M. A. (1998). The root/rhizome system of seagrasses: an asset and a burden. J. Sea Res. 39, 183–196. doi: 10.1016/S1385-1101(98)00004-5
Hoffle, H., Thomsen, M. S., and Holmer, M. (2011). High mortality of Zostera marina under high temperature regimes but minor effects of the invasive macroalgae Gracilaria vermiculophylla. Estuar. Coast. Shelf Sci. 92, 35–46. doi: 10.1016/j.ecss.2010.12.017
Hollis, J. P., Allam, A. I., and Pitts, G. (1972). Sulfide diseases of rice. Phytopathology 62, 764–765.
Holmer, M., and Nielsen, R. M. (2007). Effects of filamentous algal mats on sulfide invasion in eelgrass (Zostera marina). J. Exp. Mar. Biol. Ecol. 353, 245–252. doi: 10.1016/j.jembe.2007.09.010
Holmer, M., Andersen, F. O., Nielsen, S. L., and Boschker, H. T. S. (2001). The importance of mineralization based on sulfate reduction for nutrient regeneration in tropical seagrass sediments. Aquat. Bot. 71, 1–17. doi: 10.1016/S0304-3770(01)00170-X
Holmer, M., Duarte, C. M., and Marba, N. (2003). Sulfur cycling and seagrass (Posidonia oceanica) status in carbonate sediments. Biogeochemistry 66, 223–229. doi: 10.1023/B:BIOG.0000005326.35071.51
Holmer, M., Duarte, C. M., and Marba, N. (2005). Iron additions reduce sulfate reduction rates and improve seagrass growth on organic-enriched carbonate sediments. Ecosystems 8, 721–730. doi: 10.1007/s10021-003-0180-6
Holmer, M., and Kendrick, G. A. (2013). High sulfide intrusion in five temperate seagrasses growing under contrasting sediment conditions. Estuar. Coast. 36, 116–126. doi: 10.1007/s12237-012-9550-7
Holmer, M., Pedersen, O., Krause-Jensen, D., Olesen, B., Petersen, M. H., Schopmeyer, S., et al. (2009). Sulfide intrusion in the tropical seagrasses Thalassia testudinum and Syringodium filiforme. Estuar. Coast. Shelf S. 85, 319–326. doi: 10.1016/j.ecss.2009.08.015
Holmer, M., and Storkholm, P. (2001). Sulphate reduction and sulphur cycling in lake sediments: a review. Freshw. Biol. 46, 431–451. doi: 10.1046/j.1365-2427.2001.00687.x
Holmer, M., Wirachwong, P., and Thomsen, M. S. (2011). Negative effects of stress-resistant drift algae and high temperature on a small ephemeral seagrass species. Mar. Biol. 158, 297–309. doi: 10.1007/s00227-010-1559-5
Howes, B. L., Teal, J. M., and Peterson, S. (2005). Experimental Phragmites control through enhanced sediment sulfur cycling. Ecol. Eng. 25, 2929–303. doi: 10.1016/j.ecoleng.2005.04.004
Igamberdiev, A. U., Baron, K., Manac'h-Little, N., Stoimenova, M., and Hill, R. D. (2005). The haemoglobin/nitric oxide cycle: involvement in flooding stress and effects on hormone signaling. Ann. Bot. Lond. 96, 557–564. doi: 10.1093/aob/mci210
Ingold, A., and Havill, D. C. (1984). The influence of sulphide on the distribution of higher plants in salt marshes. J. Ecol. 72, 1043–1054. doi: 10.2307/2259550
Jackson, M. B. (1985). Ethylene and responses of plants to soil waterlogging and submergence. Annu. Rev. Plant Physiol. Plant Mol. Biol. 36, 145–174. doi: 10.1146/annurev.arplant.36.1.145
Jackson, M. B., and Armstrong, W. (1999). Formation of aerenchyma and the processes of plant ventilation in relation to soil flooding and submergence. Plant Biol. 1, 274–287. doi: 10.1111/j.1438-8677.1999.tb00253.x
Jones, C. G., Lawton, J. H., and Shachak, M. (1994). Organisms as ecosystem engineers. Oikos 69, 373–386 doi: 10.2307/3545850
Jørgensen, B. B. (1982). Mineralization of organic matter in the sea bed – The role of sulfate reduction. Nature 296, 643–645. doi: 10.1038/296643a0
Jørgensen, B. B., and Revsbech, N. P. (1983). Colorless sulfur bacteria, Beggiatoa spp. and Thiovulum spp., in O2 and H2S microgradients. Appl. Environ. Microbiol. 45, 1261–1270.
Joshi, M. M., Ibrahim, I. K. A., and Hollis, J. P. (1975). Hydrogen-sulfide – effects on physiology of rice plants and relation to straighthead disease. Phytopathology 65, 1165–1170. doi: 10.1094/Phyto-65-1165
King, G. M., Klug, M. J., Wiegert, R. G., and Chalmers, A. G. (1982). Relation of soil water movement and sulfide concentration to Spartina alterniflora production in a Georgia salt marsh. Science 218, 61–63. doi: 10.1126/science.218.4567.61
Koch, M. S., and Erskine, J. M. (2001). Sulfide as a phytotoxin to the tropical seagrass Thalassia testudinum: interactions with light, salinity and temperature. J. Exp. Mar. Biol. Ecol. 266, 81–95. doi: 10.1016/S0022-0981(01)00339-2
Koch, M. S., and Mendelssohn, I. A. (1989). Sulfide as a soil phytotoxin: differential responses in two marsh species. J. Ecol. 77, 565–578. doi: 10.2307/2260770
Koch, M. S., Mendelssohn, I. A., and McKee, K. L. (1990). Mechanism for the hydrogen sulfide-induced growth limitation in wetland macrophytes. Limnol. Oceanogr. 35, 399–408. doi: 10.4319/lo.1990.35.2.0399
Koch, M. S., Schopmeyer, S. A., Kyhn-Hansen, C., and Madden, C. J. (2007). Synergistic effects of high temperature and sulfide on tropical seagrasses. J. Exp. Mar. Biol. Ecol. 341, 91–101. doi: 10.1016/j.jembe.2006.10.004
Koerselman, W., Van Kerkhoven, M. B., and Verhoeven, J. T. A. (1993). Release of inorganic N, P and K in peat soils; effect of temperature, water chemistry and water Level. Biogeochemistry 20, 63–81. doi: 10.1007/BF00004135
Laan, P., Smolders, A., Blom, C. W. P. M., and Armstrong, W. (1989). The relative roles of internal aeration, radial oxygen loss, iron exclusion and nutrient balances in flood tolerance of Rumex species. Acta Bot. Neerl. 38, 131–145.
Laan, P., Smolders, A. J. P., and Blom, C. W. P. M. (1991). The relative importance of anaerobiosis and high iron levels in flood-tolerance of Rumex species. Plant Soil 136, 153–161. doi: 10.1007/BF02150046
Lamers, L. P. M. (2001). Tackling Biogeochemical Problems in Peatlands. Ph.D. thesis, University of Nijmegen, Nijmegen, 161. Available online at: http://webdoc.ubn.kun.nl/mono/l/lamers_l/tackbiqui.pdf
Lamers, L. P. M., Smolders, A. J. P., and Roelofs, J. G. M. (2002a). The restoration of fens in the Netherlands. Hydrobiologia 478, 107–130. doi: 10.1023/A:1021022529475
Lamers, L. P. M., Falla, S. J., Samborska, E. M., Van Dulken, I. A. R., Van Hengstum, G., and Roelofs, J. G. M. (2002b). Factors controlling the extent of eutrophication and toxicity in sulfate-polluted freshwater wetlands. Limnol. Oceanogr. 47, 585–593. doi: 10.4319/lo.2002.47.2.0585
Lamers, L. P. M., Farhoush, C., Van Groenendael, J. M., and Roelofs, J. G. M. (1999). Calcareous groundwater raises bogs; the concept of ombrotrophy revisited. J. Ecol. 87, 639–648. doi: 10.1046/j.1365-2745.1999.00380.x
Lamers, L. P. M., Tomassen, H. B. M., and Roelofs, J. G. M. (1998). Sulfate-induced eutrophication and phytotoxicity in freshwater wetlands. Environ. Sci. Technol. 32, 199–205. doi: 10.1021/es970362f
Lamers, L. P. M., Van Diggelen, J. M. H., Op den Camp, H. J. M., Visser, E. J. W., Lucassen, E. C. H. E. T., Vile, M. A., et al. (2012). Microbial transformations of nitrogen, sulfur, and iron dictate vegetation composition in wetlands: a review. Front. Microbiol. 3:156. doi: 10.3389/fmicb.2012.00156
Lee, R. W. (1999). Oxidation of sulfide by Spartina alterniflora roots. Limnol. Oceanogr. 44, 1155–1159. doi: 10.4319/lo.1999.44.4.1155
Leustek, T., and Saito, K. (1999). Sulfate transport and assimilation in plants. Plant Physiol. 120, 637–644. doi: 10.1104/pp.120.3.637
Li, S., Mendelssohn, I. A., Chen, H., and Orem, W. H. (2009). Does sulphate enrichment promote the expansion of Typha domingensis (cattail) in the Florida Everglades? Freshw. Biol. 54, 1909–1923. doi: 10.1111/j.1365-2427.2009.02242.x
Lomans, B. P., Van der Drift, C., Pol, A., and Op den Camp, H. J. M. (2002). Microbial cycling of volatile organic sulfur compounds. Cell. Mol. Life Sci. 59, 575–588. doi: 10.1007/s00018-002-8450-6
Lucassen, E. C. H. E. T., Bobbink, R., Smolders, A. J. P., Van der Ven, P. J. M., Lamers, L. P. M., and Roelofs, J. G. M. (2003). Interactive effects of low pH and high ammonium levels responsible for the decline of Cirsium dissectum (L.) Hill. Plant Ecol. 165, 45–52. doi: 10.1023/A:1021467320647
Lucassen, E. C. H. E. T., Smolders, A. J. P., and Roelofs, J. G. M. (2002). Potential sensitivity of mires to drought, acidification and mobilisation of heavy metals: the sediment S/(Ca + Mg) ratio as diagnostic tool. Environ. Pollut. 120, 635–646.
Lucassen, E. C. H. E. T., Smolders, A. J. P., and Roelofs, J. G. M. (2005). Effects of temporary desiccation on the mobility of phosphorus and metals in sulphur-rich fens: differential responses of sediments and consequences for water table management. Wetl. Ecol. Manag. 13, 135–148. doi: 10.1007/s11273-004-0314-4
Lucassen, E. C. H. E. T., Smolders, A. J. P., Van der Salm, A. L., and Roelofs, J. G. M. (2004). High groundwater nitrate concentrations inhibit eutrophication of sulphate-rich freshwater wetlands. Biogeochemistry 67, 249–267. doi: 10.1023/B:BIOG.0000015342.40992.cb
Maki, T., Nomachi, M., Yoshida, S., and Ezawa, T. (2008). Plant symbiotic microorganisms in acid sulfate soil: significance in the growth of pioneer plants. Plant Soil 310, 55–65. doi: 10.1007/s11104-008-9628-y
Marbà, N., Calleja, M. Ll., Duarte, M., Álvarez, E., Díaz-Almela, E., and Holmer, M. (2007). Iron additions reduce sulfide intrusion and reverse seagrass (Posidonia oceanica) decline in carbonate sediments. Ecosystems 10, 745–756. doi: 10.1007/s10021-007-9053-8
McKee, K. L. (1993). Soil physicochemical patterns and mangrove species distribution – Reciprocal effects. J. Ecol. 81, 477–487. doi: 10.2307/2261526
McKee, K. L., Mendelssohn, I. A., and Hester, M. W. (1988). Reexamination of pore water sulfide concentrations and redox potentials near the aerial roots of Rhizophora mangle and Avicennia germinans. Am. J. Bot. 75, 1352–1359. doi: 10.2307/2444458
McKee, K. L., Mendelssohn, I. A., and Materne, M. D. (2004). Acute salt marsh dieback in the Mississippi River deltaic plain: a drought-induced phenomenon? Glob. Ecol. Biogeogr. 13, 65–73. doi: 10.1111/j.1466-882X.2004.00075.x
Mendelssohn, I. A., and McKee, K. L. (1988). Spartina alterniflora dieback in Louisiana: time-course investigation of soil waterlogging effects. J. Ecol. 76, 509–521. doi: 10.2307/2260609
Miletto, M., Loy, A., Antheunisse, A. M., Loeb, R., Bodelier, P. L. E., and Laanbroek, H. J. (2008). FEMS Microbiol. Ecol. 64, 395–406. doi: 10.1111/j.1574-6941.2008.00490.x
Millero, F. J. (1986). The thermodynamics and kinetics of the hydrogen sulfide system in natural waters. Mar. Chem. 18, 121–147. doi: 10.1016/0304-4203(86)90003-4
Moore, P. A., and Patrick, W. H. (1989). Calcium and magnesium availability and uptake by Rice in acid sulfate soils. Soil Sci. Soc. Am. J. 53, 816–822. doi: 10.2136/sssaj1989.03615995005300030032x
Nakamura, M. (2009). Increased thiol biosynthesis of transgenic poplar expressing a wheat O-acetylserine(thiol) lyase enhances resistance to hydrogen sulfide and sulfur dioxide toxicity. Plant Cell Rep. 28, 313–323. doi: 10.1007/s00299-008-0635-5
Nielsen, O. I., Kristensen, E., and Holmer, M. (2003). Impact of Arenicola marina (Polychaeta) on sediment sulfur dynamics. Aquat. Microb. Ecol. 33, 95–105. doi: 10.3354/ame033095
Okajima, H., and Takagi, S. (1955). Physiological behavior of hydrogen sulfide in the rice plant. Tohoku J. Agricult. Res. 6, 89–99.
Olsen, K. R. (2012). Mitochondrial adaptations to utilize hydrogen sulfide for energy and signaling. J. Comp. Physiol. B 182, 881–897. doi: 10.1007/s00360-012-0654-y
Otte, M. L., Wilson, G., Morris, J. T., and Moran, B. M. (2004). Dimethylsulphoniopropionate (DMSP) and related compounds in higher plants. J. Exp. Bot. 55, 1919–1925. doi: 10.1093/jxb/erh178
Pakulski, J. D., and Kiene, R. P. (1992). Foliar release of dimethylsulfonioproprionate from Spartina-alterniflora. Mar. Ecol. Prog. Ser. 81, 277–287. doi: 10.3354/meps081277
Pedersen, O., Binzer, T., and Borum, J. (2004). Sulfide intrusion in eelgrass (Zostera marina L.). Plant Cell Environ. 27, 595–602. doi: 10.1111/j.1365-3040.2004.01173.x
Pezeshki, S. R. (2001). Wetland plant responses to soil flooding. Environ. Exp. Bot. 46, 299–312. doi: 10.1016/S0098-8472(01)00107-1
Pitts, G., Allam, I. A., and Hollis, J. P. (1972). Beggiatoa – occurrence in rice rhizosphere. Science 178, 990–991. doi: 10.1126/science.178.4064.990
Placella, S. A., Brodie, E. L., and Firestone, M. K. (2012). Rainfall-induced carbon dioxide pulses result from sequential resuscitation of phylogenetically clustered microbial groups. P. Natl. Acad. Sci U.S.A. 109, 10931–10936. doi: 10.1073/pnas.1204306109
Raven, J. A., and Scrimgeour, C. M. (1997). The influence of anoxia on plants of saline habitats with special reference to the sulphur cycle. Ann. Bot. London 79, 79–86. doi: 10.1093/oxfordjournals.aob.a010309
Roelofs, J. G. M. (1991). Inlet of alkaline river water into peaty lowlands: effects on water quality and on Stratiotes aloides stands. Aquat. Bot. 39, 267–293. doi: 10.1016/0304-3770(91)90004-O
Ruiz-Halpern, S., Macko, S. A., and Fourqurean, J. W. (2008). The effects of manipulation of sedimentary iron and organic matter on sediment biogeochemistry and seagrasses in a subtropical carbonate environment. Biogeochemistry 87, 113–126. doi: 10.1007/s10533-007-9162-7
Sammut, J., Melville, M., Callinan, R., and Fraser, G. (1995). Estuarine acidification: impacts on aquatic biota of draining acid sulphate soils. Aust. Geogr. Stud. 33, 89–100. doi: 10.1111/j.1467-8470.1995.tb00687.x
Scheffer, F., and Schachtschabel, P. (2002). Lehrbuch der Bodenkunde. Heidelberg, Berlin: Spektrum Akademischer Verlag.
Schindler, D. W., Wageman, R., Cook, R. B., Ruszcynski, T., and Prokopowich, J. (1980). Experimental acidification of Lake 223, Experimental Lakes Area: background data and the first three years of acidification. Can. J. Fish. Aquat. Sci. 37, 342–354. doi: 10.1139/f80-048
Schlesinger, W. H. (1997). Biogeochemistry – An Analysis of Global Change. San Diego, CA; London, UK: Academic Press.
Schuurkes, J. A. A. R., Kempers, A. J., and Kok, C. J. (1988). Aspects of biochemical sulphur conversions in sediments of a shallow soft water lake. J. Freshw. Ecol. 4, 369–381. doi: 10.1080/02705060.1988.9665186
Shah, J., Nagpal, T., Johnson, T., Amann, M., Carmichael, G., Foell, W., et al. (2000). Integrated analysis for acid rain in Asia: policy implications and results of RAINS-ASIA model. Annu. Rev. Energy Environ. 25, 339–375. doi: 10.1146/annurev.energy.25.1.339
Simkin, S. M., Bedford, B. L., and Weathers, K. C. (2013). Phytotoxic sulfide more important than nutrients for plants within a groundwater-fed wetland. Ecosystems. doi: 10.1007/s10021-013-9671-2
Smith, N. F., Wilcox, C., and Lessmann, J. M. (2009). Fiddler crab burrowing affects growth and production of the white mangrove (Laguncularia racemosa) in a restored Florida coastal marsh. Mar. Biol. 156, 2255–2266. doi: 10.1007/s00227-009-1253-7
Smith, S. J., Van Aardenne, J., Klimont, Z., Andres, R. J., Volke, A., and Arias, S. D. (2011). Anthropogenic sulfur dioxide emissions: 1850–2005. Atmos. Chem. Phys. 11, 1101–1116. doi: 10.5194/acp-11-1101-2011
Smolders, A. J. P., Lucassen, E. C. H. E. T., Bobbink, R., Roelofs, J. G. M., and Lamers, L. P. M. (2010). How nitrate leaching from agricultural lands provokes phosphate eutrophication in groundwater fed wetlands: the sulphur bridge. Biogeochemistry 98, 1–7. doi: 10.1007/s10533-009-9387-8
Smolders, A. J. P., Moonen, M., Zwaga, K., Lucassen, E. C. H. E. T., Lamers, L. P. M., and Roelofs, J. G. M. (2006). Changes in pore water chemistry of desiccating freshwater sediments with different sulphur contents. Geoderma 132, 372–383. doi: 10.1016/j.geoderma.2005.06.002
Smolders, A. J. P., Nijboer, R. C., and Roelofs, J. G. M. (1995). Prevention of sulphide accumulation and phosphate mobilization by the addition of iron (II) chloride to a reduced sediment: an enclosure experiment. Freshw. Biol. 34, 559–568. doi: 10.1111/j.1365-2427.1995.tb00913.x
Smolders, A. J. P., and Roelofs, J. G. M. (1993). Sulphate mediated iron limitation and eutrophication in aquatic ecosystems. Aquat. Bot. 46, 247–253. doi: 10.1016/0304-3770(93)90005-H
Smolders, A. J. P., and Roelofs, J. G. M. (1996). The roles of internal iron hydroxide precipitation, sulphide toxicity and oxidizing ability in the survival of Stratiotes aloides roots at different iron concentrations in sediment pore water. New Phytol. 133, 253–260. doi: 10.1111/j.1469-8137.1996.tb01892.x
Sperber, J. I. (1958). Release of phosphate from soil minerals by hydrogen sulphide. Nature 181, 934. doi: 10.1038/181934a0
Starkey, R. L. (1966). Oxidation and reduction of sulphur compounds in soils. Soil Sci. 101, 297–306. doi: 10.1097/00010694-196604000-00009
Stefels, J. (2000). Physiological aspects of the production and conversion of DMSP in marine algae and higher plants. Review paper. J. Sea Res. 43, 183–197. doi: 10.1016/S1385-1101(00)00030-7
Tanaka, A., Ranjit, P., Mulleriyawa, R. P., and Yasu, T. (1968). Possibility of hydrogen sulfide induced iron toxicity of the rice plant. Soil Sci. Plant Nutr. 14, 1–6. doi: 10.1080/00380768.1968.10432000
Terrados, J., Duarte, C. M., Kamp-Nielsen, L., Agawin, N. S. R., Gacia, E., Lacap, D., et al. (1999). Are seagrass growth and survival constrained by the reducing conditions of the sediment? Aquat. Bot. 65, 175–197. doi: 10.1016/S0304-3770(99)00039-X
Thomsen, M. S., Wernberg, T., Engelen, A. H., Tuya, F., Vanderklift, M. A., Holmer, M., et al. (2012). A meta-analysis of seaweed impacts on seagrasses: generalities and knowledge gaps. PLoS ONE 7:e28595. doi: 10.1371/journal.pone.0028595
Trust, B. A., and Fry, B. (1992). Stable sulfur isotopes in plants – A review. Plant Cell Environ. 15, 1105–1110. doi: 10.1111/j.1365-3040.1992.tb01661.x
Vallack, H. W., Cinderby, S., Kuylenstierna, J. C. I., and Heaps, C. (2001). Emission inventories for SO2 and NOX in developing country regions in 1995 with projected emissions for 2025 according to two scenarios. Water Air Soil Pollut. 130, 217–222. doi: 10.1023/A:1013802916582
Vámos, R. (1959). ‘Brusone’ disease of rice in Hungary. Plant Soil 11, 65–77. doi: 10.1007/BF01394754
Van Beusekom, J. J. E., and De Jonge, V. N. (2002). Long-term changes in Wadden Sea nutrient cycles: importance of organic matter import from the North Sea. Hydrobiologia 475, 185–194 doi: 10.1023/A:1020361124656
Van den Berg, L. J. L., Dorland, E., Vergeer, P., Hart, M. A. C., Bobbink, R., and Roelofs, J. G. M. (2005). Decline of acid-sensitive plant species in heathland can be attributed to ammonium toxicity in combination with low pH. New Phytol. 166, 551–564. doi: 10.1111/j.1469-8137.2005.01338.x
Van der Heide, T., Govers, L. L., De Fouw, J., Olff, H., Van der Geest, M., Van Katwijk, M. M., et al. (2012). A three-stage symbiosis forms the foundation of seagrass ecosystems. Science 336, 1353–1472.
Van der Welle, M. E. W., Cuppens, M., Lamers, L. P. M., and Roelofs, J. G. M. (2006). Detoxifying toxicants: interactions between sulphide and iron toxicity. Environ. Toxicol. Chem. 25, 1592–1597. doi: 10.1897/05-283R.1
Van der Welle, M. E. W., Smolders, A. J. P., Op den Camp, H. J. M., Roelofs, J. G. M., and Lamers, L. P. M. (2007a). Biogeochemical interactions between iron and sulphate in freshwater wetlands and their implications for interspecific competition between aquatic macrophytes. Freshw. Biol. 52, 434–447. doi: 10.1111/j.1365-2427.2006.01683.x
Van der Welle, M. E. W., Niggebrugge, K., Lamers, L. P. M., and Roelofs, J. G. M. (2007b). Differential responses of the freshwater wetland species Juncus effusus L. and Caltha palustris L. to iron supply in sulfidic environments. Environ. Pollut. 147, 222–230. doi: 10.1016/j.envpol.2006.08.024
Van Diggelen, J., Rozema, J., and Broekman, R. (1987). “Growth and mineral relations of salt-marsh species onnutrient solutions containing various sodium sulphide concentrations,” in Vegetation between Land and Sea, eds A. H. L. Huiskes, C. W. P. M. Blom, and J. Rozema (Dordrecht/Boston/Lancaster: Dr. W. Junk Publishers), 260–269. doi: 10.1007/978-94-009-4065-9_20
Van Kempen, M. M. L., Smolders, A. J. P., Lamers, L. P. M., and Roelofs, J. G. M. (2012). Micro-halocline enabled nutrient recycling may explain extreme Azolla event in the Eocene Arctic Ocean. PLoS ONE 7:e50159. doi: 10.1371/journal.pone.0050159
Ver, L. M. B., Mackenzi, F. T., and Lerman, A. (1999). Carbon cycle in the coastal zone: effects of global perturbations and change in the past three centuries. Chem. Geol. 159, 283–304. doi: 10.1016/S0009-2541(99)00042-X
Viaroli, P., Bartoli, M., Bondavalli, C., Christian, R. R., Giordani, G., and Naldi, M. (1996). Macrophyte communities and their impact on benthic fluxes of oxygen, sulphide and nutrients in shallow eutrophic environments. Hydrobiologia 329, 105–119. doi: 10.1007/BF00034551
Visser, E. J. W., and Bögemann, G. M. (2006). Aerenchyma formation in the wetland plant Juncus effusus is independent of ethylene. New Phytol. 171, 305–314. doi: 10.1111/j.1469-8137.2006.01764.x
Visser, E. J. W., Voesenek, L. A. C. J., Vartapetian, B. B., and Jackson, M. B. (2003). Flooding and plant growth. Ann. Bot. Lond. 91, 107–109. doi: 10.1093/aob/mcg014
Voesenek, L. A. C. J., Colmer, T. D., Pierik, R., Millenaar, E. E., and Peeters, A. J. M. (2006). How plants cope with complete submergence. New Phytol. 170, 213–226. doi: 10.1111/j.1469-8137.2006.01692.x
Webb, E. C., and Mendelssohn, I. A. (1996). Factors affecting Vegetation dieback of an oligohaline marsh in coastal Louisiana: field manipulation of salinity and submergence. Am. J. Bot. 83, 1429–1434. doi: 10.2307/2446098
Weber, R. E., and Vinogradow, S. N. (2001). Nonvertebrate hemoglobins: functions and molecular adaptations. Physiol. Rev. 81, 569–628.
Wiessner, A., Kuschk, P., Jechorek, M., Seidel, H., and Kastner, M. (2008). Sulphur transformation and deposition in the rhizosphere of Juncus effusus in a laboratory-scale constructed wetland. Environ. Pollut. 155, 125–131. doi: 10.1016/j.envpol.2007.10.027
Wu, J., Cheng, S., Liang, W., and Wu, Z. (2009). Effects of organic-rich sediment and below-ground sulfide exposure on submerged macrophyte, Hydrilla verticillata. B. Environ. Contam. Tox. 83, 497–501. doi: 10.1007/s00128-009-9800-y
Keywords: global change, iron, microorganism, oxygen, plant, roots, sulfur, symbiosis
Citation: Lamers LPM, Govers LL, Janssen ICJM, Geurts JJM, Van der Welle MEW, Van Katwijk MM, Van der Heide T, Roelofs JGM and Smolders AJP (2013) Sulfide as a soil phytotoxin—a review. Front. Plant Sci. 4:268. doi: 10.3389/fpls.2013.00268
Received: 16 March 2013; Accepted: 02 July 2013;
Published online: 22 July 2013.
Edited by:
Pierdomenico Perata, Scuola Superiore Sant'Anna, ItalyReviewed by:
Stanislav Kopriva, John Innes Centre, UKMarianne Holmer, University of Southern Denmark, Denmark
Copyright © 2013 Lamers, Govers, Janssen, Geurts, Van der Welle, Van Katwijk, Van der Heide, Roelofs and Smolders. This is an open-access article distributed under the terms of the Creative Commons Attribution License, which permits use, distribution and reproduction in other forums, provided the original authors and source are credited and subject to any copyright notices concerning any third-party graphics etc.
*Correspondence: Leon P. M. Lamers, Department of Aquatic Ecology and Environmental Biology, Institute for Water and Wetland Research, Radboud University Nijmegen, Heyendaalseweg 135, 6525 AJ, Nijmegen, Netherlands e-mail: l.lamers@science.ru.nl