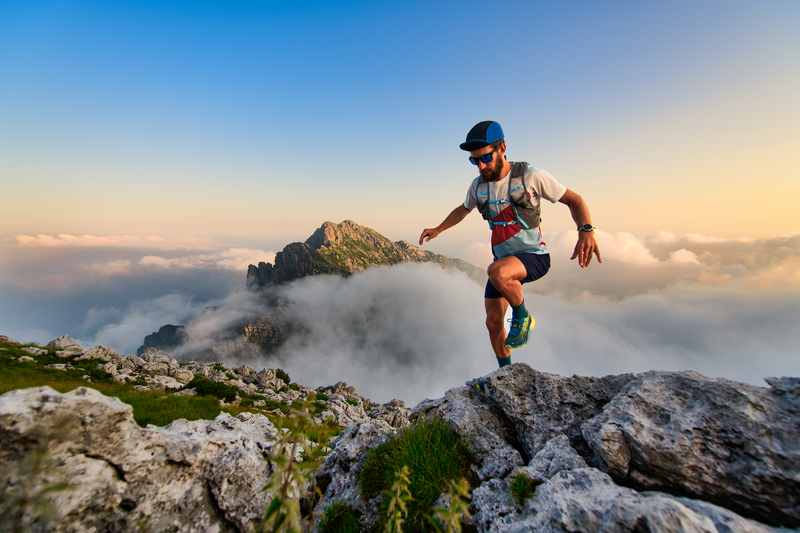
95% of researchers rate our articles as excellent or good
Learn more about the work of our research integrity team to safeguard the quality of each article we publish.
Find out more
MINI REVIEW article
Front. Plant Sci. , 21 May 2013
Sec. Plant Physiology
Volume 4 - 2013 | https://doi.org/10.3389/fpls.2013.00146
This article is part of the Research Topic Current challenges in photosynthesis: From natural to artificial View all 11 articles
The photosystem II reaction center mediates the light-induced transfer of electrons from water to plastoquinone, with concomitant production of O2. Water oxidation chemistry occurs in the oxygen-evolving complex (OEC), which consists of an inorganic Mn4CaO5 cluster and its surrounding protein matrix. Light-induced Fourier transform infrared (FTIR) difference spectroscopy has been successfully used to study the molecular mechanism of photosynthetic water oxidation. This powerful technique has enabled the characterization of the dynamic structural changes in active water molecules, the Mn4CaO5 cluster, and its surrounding protein matrix during the catalytic cycle. This mini-review presents an overview of recent important progress in FTIR studies of the OEC and implications for revealing the molecular mechanism of photosynthetic water oxidation.
Photosynthetic water oxidation is catalyzed by a Mn4Ca cluster and its surrounding protein matrix in photosystem II (PSII; Ferreira et al., 2004; Loll et al., 2005; Yano et al., 2006; Umena et al., 2011). The oxygen-evolving complex (OEC) accumulates oxidizing equivalents from the photochemical reactions within PSII and cycles through five oxidation states, termed Sn (n = 0–4, n representing the storage of oxidizing equivalents in the OEC; Kok et al., 1970; Figure 1A). The molecule O2 is produced in the transition of S3-(S4)-S0. One of the recent major breakthroughs in PSII research was the report of the crystal structure of oxygen-evolving PSII at 1.9 Å resolution (Umena et al., 2011). The structure of the Mn4CaO5 cluster is shown in Figure 1B. Three Mn, one Ca, and four oxygen atoms form a cubane-like structure; the fourth Mn connects to the cubic structure by two μ-oxo-bridges. The Mn4CaO5 cluster is connected with four water molecules: two are ligated to Ca and two to Mn4 (Umena et al., 2011; Figure 1B). These water molecules are candidates for substrates in photosynthetic water oxidation. Another distinct feature in the structure is the apparently longer bond distances between the O5-bridging oxygen atom and neighboring metal ions, which indicates weak bonding of this oxygen atom in the cluster. O5 was proposed as a candidate for one of the substrates in dioxygen formation (Umena et al., 2011). More recent studies have suggested that the structure of the X-ray diffraction (XRD) model of PSII is modified by radiation-induced reduction of the Mn cluster (Luber et al., 2011; Grundmeier and Dau, 2012). Despite this problem, the 1.9 Å XRD structure is the crucial foundation for spectroscopic and mechanistic studies of photosynthetic water oxidation.
FIGURE 1. (A) S-state cycle of photosynthetic water oxidation. (B) Structure of the Mn4CaO5 cluster as revealed by the 1.9 Å XRD study (Umena et al., 2011). Images generated in the PyMOL program using Protein Data Bank entry 3ARC.
Several structural models of photosynthetic water oxidation have been proposed (Hoganson and Babcock, 1997; Pecoraro et al., 1998; McEvoy and Brudvig, 2006; Kusunoki, 2007; Pushkar et al., 2008; Siegbahn, 2009; Grundmeier and Dau, 2012), with differing locations and molecular structures of S-state intermediates (i.e., terminal water molecules or water-derived metal ligands). Several molecular spectroscopic techniques, including advanced electron paramagnetic resonance (EPR) spectroscopy (Britt et al., 2004; McConnell et al., 2011; Rapatskiy et al., 2012) and light-induced Fourier transform infrared (FTIR) difference spectroscopy (see below) have been extensively used to resolve this issue.
Fourier transform infrared difference spectroscopy has been widely used to study the structural changes in the OEC during the S-state catalytic cycle. The S2-minus-S1 mid-frequency (1800–1000 cm-1) FTIR difference spectrum was first reported in 1992 (Noguchi et al., 1992). The S3-minus-S2 spectrum of the PSII/OEC was reported in 2000 (Chu et al., 2000b), and spectra of flash-induced S-state transitions (S1 → S2, S2 → S3, S3 → S0, and S0 → S1) during the complete S-state cycle were reported 1 year later (Hillier and Babcock, 2001; Noguchi and Sugiura, 2001). Many FTIR studies of the OEC focused on the mid-frequency region (1800–1000 cm-1) of the IR spectrum, which contains information on structural changes of protein backbones and amino acid side-chains associated with S-state transitions of the OEC. One very important development in FTIR studies of the OEC were reports of high-frequency spectra (3700–3500 cm-1) of the OEC, which contain information on structural changes of the weakly H-bonded OH-stretching of active water molecules during S-state transitions of the OEC (Noguchi and Sugiura, 2000, 2002a,b). The other important developments were reports of low-frequency spectra (<1000 cm-1), which contain information on metal-ligand and manganese-substrate vibration modes of the OEC (Chu et al., 1999, 2000a,c; Yamanari et al., 2004; Kimura et al., 2005b).
This mini-review gives an overview of recent important progress in FTIR studies of the OEC, combined with new spectroscopic and XRD structural information, to understand the chemical mechanism of photosynthetic water oxidation. More comprehensive reviews on FTIR studies of the OEC are available (Noguchi, 2007, 2008a,b; Debus, 2008).
The reactions of substrate water during the S-state catalytic cycle of the OEC are of paramount importance to understand the chemical mechanism of photosynthetic water oxidation. In the new XRD structure of the Mn4CaO5 cluster, the four water molecules connected to the OEC are involved in a hydrogen-bonded network linking the Mn4CaO5-cluster and YZ (Umena et al., 2011). The bond distances (2.8–3.3 Å) between oxygen atoms of coordinated water molecules and their neighboring water molecules indicate that most of the O–H groups of the water molecules are weakly hydrogen bonded and will appear in the weakly hydrogen-bonded OH-stretch (3750–3500 cm-1) region of the FTIR spectra. Noguchi and colleagues reported flash-induced difference spectra of S-state transitions in the weakly H-bonded OH-stretching region (Noguchi and Sugiura, 2000, 2002a,b). One active water molecule on the OEC, which gave rise to the S1 band at ~3585 cm-1 and the S2 band at ~3618 cm-1, was identified at 250 K in light-induced S2/S1 FTIR difference spectrum (Noguchi and Sugiura, 2000) and during the S-state cycle at 10°C (Noguchi and Sugiura, 2002a,b) in PSII core complexes from Thermosynechococcus elongatus. The results indicated a weakened hydrogen bond of the OH group for one water molecule connected to the OEC during the S1 → S2 transition. In contrast to the S1 → S2 transition, the S2 → S3, S3 → S0, and S0 → S1 transitions all showed a negative OH-stretching mode at different frequencies, which indicates that these water (or hydroxide) molecules were involved in proton release reactions of the OEC or formed strong hydrogen-bonding interactions during these transitions (Noguchi and Sugiura, 2002a,b). In addition, these observations are consistent with a recent FTIR study which concluded that the proton release pattern from the substrate water on the OEC is in 1:0:1:2 stoichiometry for the S0 → S1 → S2 → S3 → S0 transition (Suzuki et al., 2009). One of the important issues is the exact location of these active water molecules detected by FTIR difference spectra on the OEC (e.g., associated with Mn or Ca) in the new XRD structure.
From studies of Mn model compound, Mn-ligand and Mn-substrate vibration modes of the PSII/OEC are expected to show up in the low-frequency region (<1000 cm-1) of the IR spectrum (Chu et al., 2001c). In low-frequency S2/S1 FTIR difference spectra of octyl-β-D-thioglucopyranoside (OTG) PSII core preparations of spinach, a positive mode at 606 cm-1 in 16O water clearly downshifted to 596 cm-1 in 18O water (Chu et al., 2000c; Figure 2A). With double-difference (S2/S1 and 16O minus 18O) spectra, the 606 cm-1 mode was assigned to an S2 mode, and a corresponding S1 mode at about 625 cm-1 was identified (Chu et al., 2000c). In addition, this 606-cm-1 mode was up-shifted to about 618 cm-1 with Sr2+ substitution but not significantly affected by 44Ca isotope substitution (Chu et al., 2000c; Kimura et al., 2005a; Figure 2B). From these results and studies of Mn model compounds, this vibrational mode at 606 cm-1 in the S2 state was assigned to a Mn–O–Mn cluster vibration in the OEC (Chu et al., 2000c). The structure of this Mn–O–Mn cluster very likely includes additional oxo and carboxylate bridges(s). IR modes for υ(Mn=O) and υasy(Mn–O–Mn) for a singly oxo-bridged Mn cluster usually occur at >700 cm-1 and typically have a 30–40 cm-1 downshift (Chu et al., 2001c). They are unlikely to be the origin of the 606-cm-1 mode. Furthermore, this 606-cm-1 mode was altered in S2/S1 FTIR difference spectra of Ala344D1Gly, Glu189Gln, and Asp170HisD1 Synechocystis mutant PSII particles (Chu et al., 2001a; Mizusawa et al., 2004; Kimura et al., 2005c). All the above amino acid residues are direct ligands for the Mn4Ca cluster. Therefore, the structure of the Mn–O–Mn cluster is structurally coupled to its surrounding ligand environment.
FIGURE 2. Comparison of the low-frequency S2/S1 FTIR difference spectra of spinach PSII core complexes (A) in buffered H216O (solid line) or H218O (dotted line), (B) with Sr2+-reconstitution (solid line) or Ca2+-reconstitution (dashed line), and (C) S2QA–/S1QA FTIR difference spectra with 50 mM NaCl (blue line), 50 mM 14NH4Cl (red line), or 50 mM 15NH4Cl (green line). The sample temperature was 250 K (reprinted with permission from Chu et al., 2000c, copyright 2000 and 2011, by the American Chemical Society).
Low-frequency S3/S2 spectra were reported in OTG PSII core preparations of spinach, in which intense bands at 604(-) and 621 (+) cm-1 were sensitive to 18O water exchange (Chu et al., 2001b). The S3 mode at ~621 cm-1 was attributed to the Mn–O–Mn cluster mode of the S3 state. Kimura et al. (2005b) reported on 16O/18O- and/or H/D water-sensitive low-frequency vibrations of the OEC during the complete S-state cycle in PSII core particles from T. elongatus. The S2 mode at ~606 cm-1 changed their sign and intensity during S-state cycling, which indicates S-state-dependent changes in the core structure of the Mn4CaO5 cluster. In addition, several IR bands sensitive to both 16O/18O and H/D exchanges were attributed to S-state intermediates during the S-state cycling (Kimura et al., 2005b). Furthermore, an intense 577(-) cm-1 band in the S2/S1 spectra was found insensitive to universal 15N- and 13C-isotope labeling and assigned to the skeletal vibration of the Mn cluster or stretching vibrational modes of the Mn ligand (Kimura et al., 2003).
Low-frequency FTIR results demonstrate that one bridged oxygen atom in the Mn–O–Mn cluster of the OEC is accessible to and can be exchanged with bulky-phase water. This exchange occurs within minutes or faster because it is complete within 30 min (Chu et al., 2000c). A recent study involving W-band 17O electron–electron double resonance-detected nuclear magnetic resonance (NMR) spectroscopy reported that one μ-oxo bridge of the OEC can exchange with H217O on a time scale (≤15 s) similar to that of substrate water on the OEC (Rapatskiy et al., 2012). This study also suggested that the exchangeable μ-oxo bridge links the outer Mn to the Mn3O3Ca open-cuboidal unit (O4 and O5 in Figure 1). The authors of this study favored the Ca-linked O5 oxygen assignment (Rapatskiy et al., 2012). Low-frequency FTIR results showed that the Mn–O–Mn cluster mode at 606 cm-1 is sensitive to Sr2+ substitution but not 44Ca substitution (Chu et al., 2000c; Kimura et al., 2005a). Considering the structure of O5 in the Mn4CaO5 cluster (Umena et al., 2011; Figure 1B), the 44Ca-induced isotopic shift of the Mn–O–Mn cluster mode may have been too small to be detected by previous FTIR studies. Thus, the O5-bridging oxygen atom is a good candidate for the exchangeable-bridged oxygen atom in the Mn–O–Mn cluster identified by FTIR. A recent continue-wave Q-band electron nuclear double resonance (ENDOR) study reported a much slower 17O exchange rate (on the time scale of hours) with 17O-labeled water into the μ-oxo bridge of the OEC (McConnell et al., 2011). Future study is required to resolve this discrepancy.
Because of the structural similarity between NH3 and H2O and the ability of NH3 to inhibit photosynthetic water oxidation, the NH3 binding site on the OEC might occur at the substrate water-binding site. Previous EPR studies of NH3-treated PSII samples demonstrated that the S2-state multiline EPR signal is altered when samples illuminated at 200 K are subsequently “annealed” above 250 K (Beck et al., 1986; Britt et al., 1989). FTIR studies showed that NH3 induced characteristic spectral changes in the S2/S1 spectra at 250 K (Chu et al., 2004a; Fang et al., 2005). Among them, the S2-state symmetric carboxylate stretching mode at 1365 cm-1 in the S2/S1 spectrum of control samples up-shifted to ~1379 cm-1 in NH3-treated samples. This carboxylate mode was also altered by Sr2+ substitution (Strickler et al., 2005; Suzuki et al., 2006), which indicates that the action site of NH3 on the OEC is near the Ca2+ site. In addition, the conditions that give rise to the NH3-induced up-shift of this S2-state carboxylate stretching mode at 1365 cm-1 are strongly correlated with those producing the modified S2-state multiline EPR signal (Chu et al., 2004a; Fang et al., 2005). Furthermore, a recent FTIR result showed that NH3 did not replace the active water molecule connected to the OEC during the S1-to-S2 transition at 250 K, whereas the Mn–O–Mn cluster vibrational mode at 606 cm-1 was diminished or underwent a large shift (Hou et al., 2011; Figure 2C). The above results are consistent with the proposal that NH3 may replace one of the bridging oxygen atoms, presumably O5, in the Mn4CaO5 cluster during the S1-to-S2 transition (Britt et al., 1989).
The other intriguing FTIR finding is that the effect of NH3-induced up-shift of 1365 cm-1 mode in the S2/S1 spectrum was diminished at temperatures above 0°C (Huang et al., 2008). The results indicate that the interaction of NH3 with the OEC is attenuated at temperatures above 0°C (Huang et al., 2008). In addition, a recent FTIR study reported an inhibitory effect of the ammonium cation on the PSII/OEC at 283 K (Tsuno et al., 2011). The results suggested that the ammonium cation perturbs some carboxylate residues coupled to the Mn cluster during the S1-to-S2 transition and inhibits the oxygen evolution reaction at 283 K (Tsuno et al., 2011).
Fourier transform infrared studies involving isotopic labeling and site-directed mutagenesis have provided a wealth of information on dynamic structural changes of the protein backbones and amino acid side-chains during the S-state transitions of the OEC (Debus, 2008; Noguchi, 2008a; Shimada et al., 2011). An isotope-edited FTIR study identified the L-[1-13C]alanine-sensitive symmetric carboxylate stretching modes in S2/S1 difference spectra to the α-COO- group of D1-Ala344 (Chu et al., 2004b). This mode appears at ~1356 cm-1 in the S1 state and at ~1339 or ~1320 cm-1 in the S2 state in unlabeled wild-type PSII particles but not in D1-Ala344Gly and D1-Ala344Ser mutant PSII particles. These frequencies are consistent with unidentate ligation of the α-COO- group of D1-Ala344 to the Mn4Ca cluster in both the S1 and S2 state (Chu et al., 2004b; Strickler et al., 2005). In addition, substituting Sr for Ca did not alter the symmetric carboxylate stretching modes of D1-Ala344 (Strickler et al., 2005). The results suggested that the α-COO- group of D1-Ala344 did not ligate Ca. In the 1.9 Å XRD structure, the α-COO- group of D1-Ala344 shows very asymmetrical bridging between Mn2 and Ca in the cluster, with the Mn–O distance 2.0 Å and Ca–O distance 2.6 Å (Kawakami et al., 2011). In addition, the isotopic bands for the α-COO- group of D1-Ala344 showed characteristic changes during S-state cycling (Kimura et al., 2005d). These results indicated that the C-terminal Ala 344D1 is structurally coupled, presumably directly ligated, to the Mn ion that undergoes oxidation of Mn(III) to Mn(IV) during the S1-to-S2 transition and is reduced in reverse with the S3-to-S0 transition (Chu et al., 2004b; Kimura et al., 2005d). In contrast, mutations of D1-Asp170, D1-Glu189, and D1-Asp342 did not eliminate any carboxylate vibrational stretching modes during S-state cycling of the OEC (Debus et al., 2005; Strickler et al., 2006, 2007). Recent computational studies suggested that vibrations of carboxylate ligands can be quite insensitive to Mn oxidation, if they are not coordinated along the Jahn–Teller axis (Sproviero et al., 2008). In their model, the only amino acid residue that is ligated along the Jahn–Teller axis of a MnIII ion is CP43-E354.
Of note, CP43-E354Q mutant PSII particles gave rise to characteristic spectral changes in the amide and carboxylate stretch regions of FTIR difference spectra during S-state transitions (Strickler et al., 2008; Shimada et al., 2009; Service et al., 2010). In addition, the weakly H-bonded O–H stretching modes of the active water molecule associated with the OEC were significantly altered in S2/S1 FTIR difference spectra of CP43-E354Q mutant PSII particles (Shimada et al., 2009). Furthermore, H218O exchange mass spectrometry experiments showed that the CP43-E354Q mutation weakened the binding of both substrate-water molecules (or water-derived ligands), particularly affecting the one with faster exchange in the S3 state (Service et al., 2010). The XRD structure of the OEC showed that coordinated water molecules were on Ca2+ and Mn4, which were both not ligated by CP43-E354 (Umena et al., 2011). Presumably, CP43-E354Q mutation may induce significant structural changes to the Mn4CaO5 core that affects associated active water molecule(s) on the OEC during the S1-to-S2 transition.
A recent time-resolved infrared study revealed the proton and protein dynamics associated with the OEC during the S-state transitions (Noguchi et al., 2012). The results suggest that during the S3-to-S0 transition, protons are greatly rearranged to form a transient state before the oxidation of the Mn4CaO5 cluster that leads to O2 formation. In addition, an early proton movement was detected during the S2 → S3 transition, indicating a proton release coupled with the electron transfer reaction. Furthermore, a relatively slow carboxylate movement occurred in the S0 → S1 transition, which might reflect the protein relaxation process to stabilize the S1 state (Noguchi et al., 2012). This study demonstrates that time-resolved infrared technique is extremely useful to monitor proton and protein dynamics of the OEC during photosynthetic oxygen evolution.
Vibrational data from model compounds relevant to the OEC is crucial to interpret FTIR data of the OEC during S-state cycling. However, vibrational data for synthetic multinuclear Mn complexes are still limited (Cua et al., 2003; Berggren et al., 2012). Particularly, vibrational data are needed for the Ca–Mn multinuclear cluster that models the Mn4CaO5 cluster (Kanady et al., 2011; Mukherjee et al., 2012). One previous study reported IR spectra and normal mode analysis of the adamantine-like complex [Mn4O6(bpea)4]n+ (Visser et al., 2002). By using the electrochemical method to record the difference IR spectrum and 18O isotopic labeling, the authors identified Mn–O vibrational modes for [MnIV4] and [MnIV3MnIII]. Comparison with OEC data ruled out the adamantine-like complex as the possible structure intermediate. Nevertheless, this approach is very powerful for interpreting FTIR data for the OEC during S-state cycling.
Light-induced FTIR difference spectroscopy has become a fruitful structural technique to study the molecular mechanism of photosynthetic water oxidation. The new high-resolution XRD structure of the OEC has served as a crucial foundation for designing FTIR experiments and interpreting FTIR data. Combined with isotopic labeling, site-directed mutagenesis, model compound studies, and normal mode analysis, FTIR difference spectroscopy will continue to provide important structural and mechanistic insights into the water-splitting process in PSII.
The author declares that the research was conducted in the absence of any commercial or financial relationships that could be construed as a potential conflict of interest.
The author is grateful to Prof. Richard J. Debus for helpful suggestions on the manuscript. This work was supported by National Science Council in Taiwan (NSC 101-2627-M-001-001) and by Academia Sinica.
EPR, electron paramagnetic resonance; FTIR, Fourier transform infrared; OEC, oxygen-evolving complex; OTG, octyl-β-D-thioglucopyranoside; PSII, photosystem II; QA, primary quinone electron acceptor in PSII; XRD, X-ray diffraction.
Beck, W. F., De Paula, J. C., and Brudvig, G. W. (1986). Ammonia binds to the manganese site of the oxygen-evolving complex of photosystem II in the S2 state. J. Am. Chem. Soc. 108, 4018–4022.
Berggren, G., Anderlund, M. F., Styring, S., and Thapper, A. (2012). FTIR study of manganese dimers with carboxylate donors as model complexes for the water oxidation complex in photosystem II. Inorg. Chem. 51, 2332–2337.
Britt, R. D., Campbell, K. A., Peloquin, J. M., Gilchrist, M. L., Aznar, C. P., Dicus, M. M., et al. (2004). Recent pulsed EPR studies of the photosystem II oxygen-evolving complex: implications as to water oxidation mechanisms. Biochim. Biophys. Acta 1655, 158–171.
Britt, R. D., Zimmermann, J. L., Sauer, K., and Klein, M. P. (1989). Ammonia binds to the catalytic manganese of the oxygen-evolving complex of photosystem II. Evidence by electron spin-echo envelope modulation spectroscopy. J. Am. Chem. Soc. 111, 3522–3532.
Chu, H.-A., Debus, R. J., and Babcock, G. T. (2001a). D1-Asp170 is structurally coupled to the oxygen evolving complex in photosystem II as revealed by light-induced Fourier transform infrared difference spectroscopy. Biochemistry 40, 2312–2316.
Chu, H.-A., Hillier, W., Law, N. A., and Babcock, G. T. (2001b). “Identification of a possible Mn–O–Mn cluster vibrational mode of the S3 state in the oxygen-evolving complex of photosystem II by the low-frequency FTIR spectroscopy,” in PS2001 proceedings: 12th Intenational Congress on Photosynthesis, S13-027, CSIRO Publishing, Collingwood, Australia.
Chu, H.-A., Hillier, W., Law, N. A., and Babcock, G. T. (2001c). Vibrational spectroscopy of the oxygen-evolving complex and of manganese model compounds. Biochim. Biophys. Acta 1503, 69–82.
Chu, H.-A., Feng, Y.-W., Wang, C.-M., Chiang, K.-A., and Ke, S.-C. (2004a). Ammonia-induced structural changes of the oxygen-evolving complex in photosystem II as revealed by light-induced FTIR difference spectroscopy. Biochemistry 43, 10877–10885.
Chu, H.-A., Hillier, W., and Debus, R. J. (2004b). Evidence that the C-terminus of the D1 polypeptide of photosystem II is ligated to the manganese ion that undergoes oxidation during the S1 to S2 transition: an isotope-edited FTIR study. Biochemistry 43, 3152–3166.
Chu, H.-A., Gardner, M., Hillier, W., and Babcock, G. (2000a). Low-frequency Fourier transform infrared spectroscopy of the oxygen-evolving complex in photosystem II. Photosynth. Res. 66, 57–63.
Chu, H.-A., Hillier, W., Law, N. A., Sackett, H., Haymond, S., and Babcock, G. T. (2000b). Light-induced FTIR difference spectroscopy of the S2-to-S3 state transition of the oxygen-evolving complex in photosystem II. Biochim. Biophys. Acta 1459, 528–532.
Chu, H.-A., Sackett, H., and Babcock, G. T. (2000c). Identification of a Mn–O–Mn cluster vibrational mode of the oxygen-evolving complex in photosystem II by low-frequency FTIR spectroscopy. Biochemistry 39, 14371–14376.
Chu, H.-A., Gardner, M. T., O’Brie, J. P., and Babcock, G. T. (1999). Low-frequency Fourier transform infrared spectroscopy of the oxygen-evolving and quinone acceptor complexes in photosystem II. Biochemistry 38, 4533–4541.
Cua, A., Vrettos, J., Paula, J., Brudvig, G., and Bocian, D. (2003). Raman spectra and normal coordinate analyses of low-frequency vibrations of oxo-bridged manganese complexes. J. Biol. Inorg. Chem. 8, 439–451.
Debus, R. J. (2008). Protein ligation of the photosynthetic oxygen-evolving center. Coord. Chem. Rev. 252, 244–258.
Debus, R. J., Strickler, M. A., Walker, L. M., and Hillier, W. (2005). No evidence from FTIR difference spectroscopy that aspartate-170 of the D1 polypeptide ligates a manganese ion that undergoes oxidation during the S0 to S1, S1 to S2, or S2 to S3 transitions in photosystem II. Biochemistry 44, 1367–1374.
Fang, C.-H., Chiang, K.-A., Hung, C.-H., Chang, K., Ke, S.-C., and Chu, H.-A. (2005). Effects of ethylene glycol and methanol on ammonia-induced structural changes of the oxygen-evolving complex in photosystem II. Biochemistry 44, 9758–9765.
Ferreira, K. N., Iverson, T. M., Maghlaoui, K., Barber, J., and Iwata, S. (2004). Architecture of the photosynthetic oxygen-evolving center. Science 303, 1831–1838.
Grundmeier, A., and Dau, H. (2012). Structural models of the manganese complex of photosystem II and mechanistic implications. Biochim. Biophys. Acta 1817, 88–105.
Hillier, W., and Babcock, G. T. (2001). S-state dependent Fourier transform infrared difference spectra for the photosystem II oxygen evolving complex. Biochemistry 40, 1503–1509.
Hoganson, C. W., and Babcock, G. T. (1997). A metalloradical mechanism for the generation of oxygen from water in photosynthesis. Science 277, 1953–1956.
Hou, L.-H., Wu, C.-M., Huang, H.-H., and Chu, H.-A. (2011). Effects of ammonia on the structure of the oxygen-evolving complex in photosystem II as revealed by light-induced FTIR difference spectroscopy. Biochemistry 50, 9248–9254.
Huang, H.-H., Wang, T.-H., and Chu, H.-A. (2008). “Ammonia-induced structural changes of the oxygen-evolving complex in photosystem II diminished at 277 K as revealed by light-induced FTIR difference Spectroscopy,” in Photosynthesis. Energy from the Sun, eds J. Allen, E. Gantt, J. Golbeck, and B. Osmond (Dordrecht: Springer), 389–391.
Kanady, J. S., Tsui, E. Y., Day, M. W., and Agapie, T. (2011). A synthetic model of the Mn3Ca subsite of the oxygen-evolving complex in photosystem II. Science 333, 733–736.
Kawakami, K., Umena, Y., Kamiya, N., and Shen, J.-R. (2011). Structure of the catalytic, inorganic core of oxygen-evolving photosystem II at 1.9 Å resolution. J. Photochem. Photobiol. B Biol. 104, 9–18.
Kimura, Y., Hasegawa, K., Yamanari, T., and Ono, T.-A. (2005a). Studies on photosynthetic oxygen-evolving complex by means of Fourier transform infrared spectroscopy: calcium and chloride cofactors. Photosynth. Res. 84, 245–250.
Kimura, Y., Ishii, A., Yamanari, T., and Ono, T.-A. (2005b). Water-sensitive Low-frequency vibrations of reaction intermediates during S-state cycling in photosynthetic water oxidation. Biochemistry 44, 7613–7622.
Kimura, Y., Mizusawa, N., Ishii, A., Nakazawa, S., and Ono, T. A. (2005c). Changes in structural and functional properties of oxygen-evolving complex induced by replacement of D1-glutamate 189 with glutamine in photosystem II: ligation of glutamate 189 carboxylate to the manganese cluster. J. Biol. Chem. 280, 37895–37900.
Kimura, Y., Mizusawa, N., Yamanari, T., Ishii, A., and Ono, T. A. (2005d). Structural changes of D1 C-terminal alpha-carboxylate during S-state cycling in photosynthetic oxygen evolution. J. Biol. Chem. 280, 2078–2083.
Kimura, Y., Mizusawa, N., Ishii, A., Yamanari, T., and Ono, T.-A. (2003). Changes of low-frequency vibrational modes induced by universal 15N- and 13C-isotope labeling in S2/S1 FTIR difference spectrum of oxygen-evolving complex. Biochemistry 42, 13170–13177.
Kok, B., Forbush, B., and McGloin, M. (1970). Cooperation of charges in photosynthetic O2 evolution-I. A linear four step mechanism. Photochem. Photobiol. 11, 457–475.
Kusunoki, M. (2007). Mono-manganese mechanism of the photosystem II water splitting reaction by a unique Mn4Ca cluster. Biochim. Biophys. Acta 1767, 484–492.
Loll, B., Kern, J., Saenger, W., Zouni, A., and Biesiadka, J. (2005). Towards complete cofactor arrangement in the 3.0 Å resolution structure of photosystem II. Nature 438, 1040–1044.
Luber, S., Rivalta, I., Umena, Y., Kawakami, K., Shen, J.-R., Kamiya, N., et al. (2011). S1-state model of the O2-evolving complex of photosystem II. Biochemistry 50, 6308–6311.
McConnell, I. L., Grigoryants, V. M., Scholes, C. P., Myers, W. K., Chen, P.-Y., Whittaker, J. W., et al. (2011). EPR–ENDOR characterization of (17O, 1H, 2H) water in manganese catalase and its relevance to the oxygen-evolving complex of photosystem II. J. Am. Chem. Soc. 134, 1504–1512.
McEvoy, J. P., and Brudvig, G. W. (2006). Water-splitting chemistry of photosystem II. Chem. Rev. 106, 4455–4483.
Mizusawa, N., Kimura, Y., Ishii, A., Yamanari, T., Nakazawa, S., Teramoto, H., et al. (2004). Impact of replacement of D1 C-terminal alanine with glycine on structure and function of photosynthetic oxygen-evolving complex. J. Biol. Chem. 279, 29622–29627.
Mukherjee, S., Stull, J. A., Yano, J., Stamatatos, T. C., Pringouri, K., Stich, T. A., et al. (2012). Synthetic model of the asymmetric [Mn3CaO4] cubane core of the oxygen-evolving complex of photosystem II. Proc. Natl. Acad. Sci. U.S.A. 109, 2257–2262.
Noguchi, T. (2007). Light-induced FTIR difference spectroscopy as a powerful tool toward understanding the molecular mechanism of photosynthetic oxygen evolution. Photosynth. Res. 91, 59–69.
Noguchi, T. (2008a). Fourier transform infrared analysis of the photosynthetic oxygen-evolving center. Coord. Chem. Rev. 252, 336–346.
Noguchi, T. (2008b). FTIR detection of water reactions in the oxygen-evolving centre of photosystem II. Philos. Trans. R. Soc. Lond. B Biol. Sci. 363, 1189–1194.
Noguchi, T., Ono, T., and Inoue, Y. (1992). Detection of structural changes upon S1-to-S2 transition in the oxygen-evolving manganese cluster in photosystem II by light-induced Fourier transform infrared difference spectroscopy. Biochemistry 31, 5953–5956.
Noguchi, T., and Sugiura, M. (2000). Structure of an active water molecule in the water-oxidizing complex of photosystem II as studied by FTIR spectroscopy. Biochemistry 39, 10943–10949.
Noguchi, T., and Sugiura, M. (2001). Flash-induced Fourier transform infrared detection of the structural changes during the S-state cycle of the oxygen-evolving complex in photosystem II. Biochemistry 40, 1497–1502.
Noguchi, T., and Sugiura, M. (2002a). Flash-induced FTIR difference spectra of the water oxidizing complex in moderately hydrated photosystem II core films: effect of hydration extent on S-state transitions. Biochemistry 41, 2322–2330.
Noguchi, T., and Sugiura, M. (2002b). FTIR detection of water reactions during the flash-induced S-state cycle of the photosynthetic water-oxidizing complex. Biochemistry 41, 15706–15712.
Noguchi, T., Suzuki, H., Tsuno, M., Sugiura, M., and Kato, C. (2012). Time-resolved infrared detection of the proton and protein dynamics during photosynthetic oxygen evolution. Biochemistry 51, 3205–3214.
Pecoraro, V., Baldwin, M., Caudle, M., Hsieh, W.-Y., and Law, N. (1998). A proposal for water oxidation in photosystem II. Pure Appl. Chem. 70, 925–930.
Pushkar, Y., Yano, J., Sauer, K., Boussac, A., and Yachandra, V. K. (2008). Structural changes in the Mn4Ca cluster and the mechanism of photosynthetic water splitting. Proc. Natl. Acad. Sci. U.S.A. 105, 1879–1884.
Rapatskiy, L., Cox, N., Savitsky, A., Ames, W. M., Sander, J., Nowaczyk, M. M., et al. (2012). Detection of the water-binding sites of the oxygen-evolving complex of photosystem II using W-band 17O electron–electron double resonance-detected NMR spectroscopy. J. Am. Chem. Soc. 134, 16619–16634.
Service, R., Yano, J., McConnell, I., Hwang, H. J., Niks, D., Hille, R., et al. (2010). Participation of glutamate-354 of the CP43 polypeptide in the ligation of Mn and the binding of substrate water in photosystem II. Biochemistry 50, 63–81.
Shimada, Y., Suzuki, H., Tsuchiya, T., Mimuro, M., and Noguchi, T. (2011). Structural coupling of an arginine side chain with the oxygen-evolving Mn4Ca cluster in photosystem II as revealed by isotope-edited Fourier transform infrared spectroscopy. J. Am. Chem. Soc. 133, 3808–3811.
Shimada, Y., Suzuki, H., Tsuchiya, T., Tomo, T., Noguchi, T., and Mimuro, M. (2009). Effect of a single-amino acid substitution of the 43 kDa chlorophyll protein on the oxygen-evolving reaction of the cyanobacterium Synechocystis sp. PCC 6803: analysis of the Glu354Gln mutation. Biochemistry 48, 6095–6103.
Siegbahn, P. E. M. (2009). Structures and energetics for O2 formation in photosystem II. Acc. Chem. Res. 42, 1871–1880.
Sproviero, E. M., Gascón, J. A., Mcevoy, J. P., Brudvig, G. W., and Batista, V. S. (2008). Computational studies of the O2-evolving complex of photosystem II and biomimetic oxomanganese complexes. Coord. Chem. Rev. 252, 395–415.
Strickler, M. A., Hillier, W., and Debus, R. J. (2006). No evidence from FTIR difference spectroscopy that glutamate-189 of the D1 polypeptide ligates a Mn ion that undergoes oxidation during the S0 to S1, S1 to S2, or S2 to S3 transitions in photosystem II. Biochemistry 45, 8801–8811.
Strickler, M. A., Hwang, H. J., Burnap, R. L., Yano, J., Walker, L. M., Service, R. J., et al. (2008). Glutamate-354 of the CP43 polypeptide interacts with the oxygen-evolving Mn4Ca cluster of photosystem II: a preliminary characterization of the Glu354Gln mutant. Philos. Trans. R. Soc. Lond. B Biol. Sci. 363, 1179–1187.
Strickler, M. A., Walker, L. M., Hillier, W., Britt, R. D., and Debus, R. J. (2007). No evidence from FTIR difference spectroscopy that aspartate-342 of the D1 polypeptide ligates a Mn ion that undergoes oxidation during the S0 to S1, S1 to S2, or S2 to S3 transitions in photosystem II. Biochemistry 46, 3151–3160.
Strickler, M. A., Walker, L. M., Hillier, W., and Debus, R. J. (2005). Evidence from biosynthetically incorporated strontium and FTIR difference spectroscopy that the C-terminus of the D1 polypeptide of photosystem II does not ligate calcium. Biochemistry 44, 8571–8577.
Suzuki, H., Sugiura, M., and Noguchi, T. (2009). Monitoring proton release during photosynthetic water oxidation in photosystem II by means of isotope-edited infrared spectroscopy. J. Am. Chem. Soc. 131, 7849–7857.
Suzuki, H., Taguchi, Y., Sugiura, M., Boussac, A., and Noguchi, T. (2006). Structural perturbation of the carboxylate ligands to the manganese cluster upon Ca2+/Sr2+ exchange in the S-state cycle of photosynthetic oxygen evolution as studied by flash-induced FTIR difference spectroscopy. Biochemistry 45, 13454–13464.
Tsuno, M., Suzuki, H., Kondo, T., Mino, H., and Noguchi, T. (2011). Interaction and inhibitory effect of ammonium cation in the oxygen evolving center of photosystem II. Biochemistry 50, 2506–2514.
Umena, Y., Kawakami, K., Shen, J. R., and Kamiya, N. (2011). Crystal structure of oxygen-evolving photosystem II at a resolution of 1.9 Å. Nature 473, 55–60.
Visser, H., Dubé, C. E., Armstrong, W. H., Sauer, K., and Yachandra, V. K. (2002). FTIR spectra and normal-mode analysis of a tetranuclear manganese adamantane-like complex in two electrochemically prepared oxidation states: relevance to the oxygen-evolving complex of photosystem II. J. Am. Chem. Soc. 124, 11008–11017.
Yamanari, T., Kimura, Y., Mizusawa, N., Ishii, A., and Ono, T.-A. (2004). Mid-to low-frequency Fourier transform infrared spectra of S-state cycle for photosynthetic water oxidation in Synechocystis sp. PCC 6803. Biochemistry 43, 7479–7490.
Keywords: photosystem II, oxygen evolution, FTIR, manganese cluster, infrared spectroscopy
Citation: Chu H-A (2013) Fourier transform infrared difference spectroscopy for studying the molecular mechanism of photosynthetic water oxidation. Front. Plant Sci. 4:146. doi: 10.3389/fpls.2013.00146
Received: 31 March 2013; Paper pending published: 26 April 2013;
Accepted: 29 April 2013; Published online: 21 May 2013.
Edited by:
Harvey J. M. Hou, Alabama State University, USAReviewed by:
Takumi Noguchi, Nagoya University, JapanCopyright: © 2013 Chu. This is an open-access article distributed under the terms of the Creative Commons Attribution License, which permits use, distribution and reproduction in other forums, provided the original authors and source are credited and subject to any copyright notices concerning any third-party graphics etc.
*Correspondence: Hsiu-An Chu, Institute of Plant and Microbial Biology, Academia Sinica, Taipei 11529, Taiwan. e-mail:Y2h1aGFAZ2F0ZS5zaW5pY2EuZWR1LnR3
Disclaimer: All claims expressed in this article are solely those of the authors and do not necessarily represent those of their affiliated organizations, or those of the publisher, the editors and the reviewers. Any product that may be evaluated in this article or claim that may be made by its manufacturer is not guaranteed or endorsed by the publisher.
Research integrity at Frontiers
Learn more about the work of our research integrity team to safeguard the quality of each article we publish.