- 1Department of Biology, The Pennsylvania State University, University Park, PA, USA
- 2Center for Lignocellulose Structure and Formation, The Pennsylvania State University, University Park, PA, USA
Pectin is a component of the cell walls of plants that is composed of acidic sugar-containing backbones with neutral sugar-containing side chains. It functions in cell adhesion and wall hydration, and pectin crosslinking influences wall porosity and plant morphogenesis. Despite its low abundance in the secondary cell walls that make up the majority of lignocellulosic biomass, recent results have indicated that pectin influences secondary wall formation in addition to its roles in primary wall biosynthesis and modification. This mini-review will examine these and other recent results in the context of biomass yield and digestibility and discuss how these traits might be enhanced by the genetic and molecular modification of pectin. The utility of pectin as a high-value, renewable biomass co-product will also be highlighted.
Introduction
In a society with an increasing demand for renewable energy, plant species as diverse as switchgrass, sugarcane, Miscanthus, Jatropha, poplar, willow, and Agave have been put forward as candidates for lignocellulosic feedstocks to produce liquid biofuels with low net greenhouse gas emissions (Carroll and Somerville, 2009; Somerville et al., 2010). However, many challenges and limitations remain for the economical and efficient conversion of biomass to biofuel (Somerville et al., 2010). Two central challenges are the recalcitrance of biomass to degradation by enzymes into its component sugars, and the fact that plant biomass contains many different hexose and pentose monosaccharides, all of which must be converted into useful products in order to capture the full energy content and value of lignocellulosic feedstocks.
Pectin is a major component of the primary cell walls of dicotyledonous plants and is also present in smaller amounts in the secondary walls of dicots and both types of cell walls in monocots (Vogel, 2008). Pectins are highly complex polysaccharides and are composed of at least four subclasses: homogalacturonan (HG), rhamnogalacturonan (RG-I), RG-II, and xylogalacturonan (XGA; Mohnen, 2008). The backbones of HG, RG-II, and XGA consist of α-1,4-linked galacturonic acid (GalA) residues that can be methyl-esterified at the C6 carboxyl group and/or acetylated at O2 or O3, whereas the backbone of RG-I is composed of alternating rhamnose and GalA residues. RG-II possesses complex side chains with at least 12 different types of sugars, RG-I contains structurally diverse side chains consisting mainly of arabinose and galactose along with other sugars, and XGA is essentially HG with added β-1,3-xylosyl side groups (Mohnen, 2008). The synthesis of pectic polysaccharides is estimated to involve at least 67 different enzyme activities, including glycosyltransferases, methyltransferases, and acetyltransferases (Mohnen, 2008; Harholt et al., 2010). Several excellent reviews discuss the details of pectin structure and biosynthesis (Ridley et al., 2001; Willats et al., 2001; Mohnen, 2008; Harholt et al., 2010), which will not be further elaborated upon here.
Roles of Pectin in Plant Development and Biomass Yield
Pectin biosynthesis, function, modification, and degradation are involved in several key processes during plant development, including cell wall expansion, cell adhesion, organ formation, cell separation, and phyllotactic patterning (Wolf et al., 2009). Pectin is synthesized in the Golgi apparatus (Moore and Raine, 1988; Moore et al., 1991), which in plants is also the assembly site for glycoproteins, proteoglycans, and other complex polysaccharides (Parsons et al., 2012). Pectin is secreted into the apoplast (the extracellular space that contains the cell wall) in a highly methyl-esterified form (Driouich et al., 2012). One unanswered question is the extent to which pectin and other wall components are sorted during synthesis and trafficking, and whether they first interact with one another before or after secretion.
In the apoplast, pectin can be de-methyl-esterified by the activity of pectin methylesterases (PMEs; Micheli, 2001), and the carboxyl groups of GalA residues can then form intermolecular Ca2+-mediated crosslinks (Vincken et al., 2003). Additionally, borate diesters can form between the apiose groups of different RG-II molecules, causing them to dimerize (Kobayashi et al., 1996). These crosslinks are generally thought to increase cell wall stiffness: for example, premature de-methyl-esterification restricts hypocotyl elongation in dark-grown Arabidopsis thaliana (Arabidopsis) seedlings (Derbyshire et al., 2007), and digestion by fungal pectinases or chelation of Ca2+ by ethylene glycol tetraacetic acid (EGTA) restores the susceptibility of cucumber hypocotyls to the activity of wall-loosening expansins in vitro (Zhao et al., 2008). However, recent research has suggested that pectin de-methyl-esterification might also increase its susceptibility to enzymatic degradation, loosening the wall: for instance, pectin de-methyl-esterification facilitates organ primordium initiation in Arabidopsis shoot apical meristems (Peaucelle et al., 2011), and overexpression of PMEI4 delays the growth acceleration of dark-grown Arabidopsis hypocotyls (Pelletier et al., 2010). Depending on its consequences, the methyl-esterification status of pectin can thus have complex effects on plant growth (Peaucelle et al., 2012).
Intriguingly, overexpression of a PME inhibitor (PMEI) has resulted in increased biomass in transgenic Arabidopsis, as well as slightly increased biomass in transgenic wheat, although the latter difference was not significant (Lionetti et al., 2010). Taken together, the above results suggest that the timing and extent of pectin crosslinking likely influence the growth rate, persistence of expansion, final size, and/or growth robustness of plant tissues, which could in turn influence overall crop yields. Further analysis and manipulation of the links between pectin modification and biomass yield will be an important future research avenue.
Pectin and Secondary Wall Formation
In addition to its well-established role in primary wall biosynthesis and expansion, some studies have provided evidence for the importance of pectin in secondary cell wall biosynthesis and modification. PME genes are expressed in the expanding wood cells of poplar (Siedlecka et al., 2008) and in the stem, phloem, and xylem of southern blue gum (Eucalyptus globulus; Goulao et al., 2011). In E. pilularis, single-nucleotide polymorphism (SNP) alleles of PME6 associate with cellulose, lignin, and pulp yield, whereas alleles of PME7 associate with cellulose, pulp yield, and wood shrinkage (Sexton et al., 2012). Pectin-associated β-1,4-galactans have also been detected in the secondary walls of tension and compression wood (Mellerowicz and Gorshkova, 2012), and upregulation of both pectin-modifying and secondary wall biosynthetic genes has been detected in Arabidopsis plants placed under mechanical load (Koizumi et al., 2009). However, these analyses only provide correlative evidence, and genetic, biochemical, and mechanical experiments are required to establish a clearer link between pectin modification and secondary wall formation. In a pioneering study along these lines, Arabidopsis mutants lacking PME35 gene function displayed reduced mechanical integrity in their stem interfascicular fibers (Hongo et al., 2012). Interestingly, all of the above studies highlight pectin-modifying or -degrading genes rather than pectin biosynthetic genes, implying that pectin modification, instead of its synthesis, is an important aspect of secondary wall development.
Among plant lineages, the presence of RG-II correlates with upright growth, and an increased amount of borate crosslinked RG-II in the cell walls has been postulated to have facilitated the evolution of lignified secondary walls in vascular plants (Matsunaga et al., 2004), implying that pectin might continue to play a role in the early stages of secondary wall deposition. Finally, lignin polymerization, which is an important phase of secondary wall formation in many cell types, has been postulated to initiate in the pectin-rich middle lamella that lies between the walls of adjacent cells (Figure 1A), suggesting that there may be a functional connection between these polymers (Westermark et al., 1986). Support for this hypothesis is provided by the finding that addition of pectin affects the in vitro dispersion and polymerization of lignin in cellulose networks produced by Gluconacetobacter xylinus (Touzel et al., 2003). However, additional evidence will be required to establish a clear and direct connection between pectin biosynthesis and/or modification and secondary wall formation.
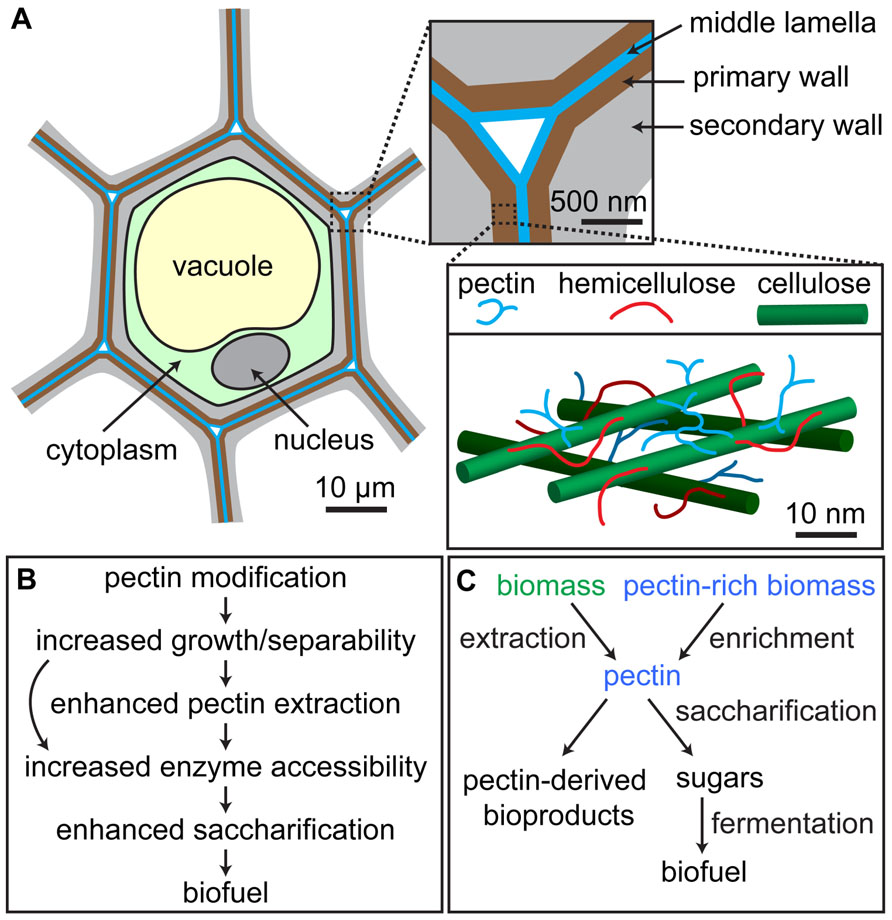
FIGURE 1. Location and roles of pectins in biomass. (A) Schematic of plant cell showing arrangement of cell walls; pectin is abundant in the primary walls synthesized by growing cells (brown) and the middle lamella that adheres adjacent cells (blue), but is also present in lower amounts in secondary walls produced after the cessation of growth (gray). Inset at lower right is a simplified model of the primary cell wall showing one possible arrangement of cellulose microfibrils (green), hemicellulose (red), and pectin (blue). (B) Pectin-rich biomass can be derived from lignocellulosic feedstocks or naturally pectin-rich plant material, after which it can be processed into pectin-derived high-value bioproducts and/or saccharified and fermented into biofuel. (C) Potential positive impacts of pectin modification in bioenergy crop plants on biomass processing. In some cases, pectin modification might allow for the elimination of processing steps, such as pectin extraction (curved arrow in B).
Pectin and Cell Adhesion
Intercellular adhesion is a basic feature of plant development and contributes to plant morphogenesis (Knox, 1992). Cell adhesion occurs primarily at the middle lamella, which contains abundant pectins, especially in the reinforcing zones (Jarvis et al., 2003). However, the exact makeup of pectin in the middle lamella is unclear, with some evidence indicating that pectin in this region is mainly composed of RG-I (Moore and Raine, 1988) and other work describing a preponderance of HG (Knox et al., 1990; Willats et al., 1999; Bush et al., 2001). HG chains might also contribute to cell adhesion by crosslinking to other wall components via uronyl esters (Sobry et al., 2005). Antibody labeling of pectin epitopes has provided circumstantial evidence for the function of pectin in cell adhesion (Parker et al., 2001; Sobry et al., 2005), but additional evidence that directly extrapolates the adhesive forces between individual pectin molecules to those between adjacent cells would be informative.
Defective cell adhesion in several mutants has been attributed to insufficient HG–Ca2+ complexes, branched RG-I polysaccharides, and/or RG-II dimerization (Rhee and Somerville, 1998; Thompson et al., 1999; Shevell et al., 2000; Neumetzler et al., 2012). Arabidopsis mutants lacking functional copies of the QUASIMODO1 (QUA1) gene, which encodes the putative GalA transferase GALACTURONOSYLTRANSFERASE 8 (GAUT8), display reduced stature, pectin content, and cell adhesion (Bouton et al., 2002; Leboeuf et al., 2005). Mutants lacking another Arabidopsis putative glycosyltransferase, ECTOPICALLY PARTING CELLS 1 (EPC1), also display defective cell adhesion (Singh et al., 2005). However, direct evidence of the role of EPC1 in pectin biosynthesis and cell adhesion is lacking. Mutation in a putative pectin methyltransferase gene, QUA2/TUMOROUS SHOOT DEVELOPMENT2 (TSD2), causes reduced cell adhesion and inhibition of shoot development (Krupkova et al., 2007; Mouille et al., 2007). In addition, it has also been shown that polygalacturonases (PGs), which cleave de-methyl-esterified HG, can affect cell adhesion: overexpression of a PG gene in apple trees led to altered cell wall adhesion, resulting in abnormal cell separation and plant morphology (Atkinson et al., 2002).
The opposite of cell adhesion, controlled cell separation, occurs in specific tissues and developmental stages in plants and involves the selective degradation of pectin in the middle lamella (Lewis et al., 2006). Artificially controlling cell separation processes might enhance the degradability of engineered biomass feedstocks by increasing the ease with which their cells can be separated by mechanical and/or enzymatic treatments, exposing more surface area to wall-degrading enzymes. However, plants displaying increased cell separability must also maintain growth robustness and disease resistance; thus, inducibly controlled cell separation might be preferable to constitutive activation of this process in future biomass feedstocks (Figure 1B).
Pectin and Biomass Processing
To efficiently produce biofuels from raw biomass feedstocks, the optimization of methods for pectin extraction and degradation is necessary (Fissore et al., 2011; Min et al., 2011). This is true for two reasons: first, pectin can affect the accessibility of other cell wall components to enzymatic degradation, and second, the sugars contained in pectin itself represent captured photosynthetic energy. In most biomass processing schemes, biomass is first pretreated to disrupt cell wall structure, then saccharified by enzymatic, chemical, or thermal treatment. However, the architectural properties of cell walls, which have been modeled as a cellulose–hemicellulose network embedded in a pectin matrix (Figure 1A; Cosgrove, 2000; Dick-Perez et al., 2011), suggest that pectins might mask cellulose and/or hemicellulose (Marcus et al., 2008, 2010), blocking their exposure to degradative enzymes. In fiber hemp processing, pectinase treatment has recently been shown to increase yields of GalA and neutral monosaccharides, and removal of pectin led to increased cell wall surface, improving the accessibility of cellulose to degradative enzymes (Pakarinen et al., 2012). Moreover, modification of pectin by expressing a PG or a PMEI to reduce the total amount of de-methyl-esterified HG in Arabidopsis, tobacco, or wheat significantly increased the efficiency of enzymatic saccharification (Lionetti et al., 2010), although PG expression, but not PMEI expression, also led to reduced biomass accumulation in transgenic plants.
The acetyl groups contained in pectin are generally thought to increase biomass recalcitrance by reducing the susceptibility of pectin to enzymatic degradation (Gille and Pauly, 2012). However, surprising results in a recent study (Gou et al., 2012) showed that reduction of pectin acetylation in tobacco by overexpression of a poplar (Populus trichocarpa) pectin acetylesterase (Pt PAE1) in fact led to lower susceptibility of pectin to degradation, throwing the conventional view into question. Interestingly, the floral styles and filaments of transgenic plants displayed reductions in monosaccharides associated with pectins and increases in monosaccharides associated with cellulose and hemicelluloses (Gou et al., 2012), suggesting that compensatory changes in cell wall composition took place in these tissues. In another study, heterologous expression of a mung bean PAE in potato tubers resulted in stiffer tuber tissue, implying that the cell walls of transgenic tubers were mechanically stronger (Orfila et al., 2012). The generation and analysis of biomass crop plants overexpressing PAEs should indicate whether manipulating pectin acetylation levels will in fact enhance biomass for biofuel production. The accumulation of acetate in saccharified biomass, which is derived mainly from de-acetylation of xylans but also arises partly from pectin de-acetylation, can act as a potent inhibitor of biofuel conversion (Gille and Pauly, 2012), and the partial reduction of cell wall acetylation by modulating pectin acetyltransferase and/or acetylesterase activities might therefore improve microbial viability during fermentation and enhance the conversion efficiency of biomass to biofuel (Figure 1B).
Because of its crosslinking and water complexation properties, pectin is also a determinant of cell wall porosity (Willats et al., 2001). In one study, treatment with pectin-degrading enzymes such as endo-PGs increased wall pore size and the ability of larger molecules to pass through the wall (Baron-Epel et al., 1988); however, treatment with cellulysin or protease did not affect porosity, implying that pectin rather than cellulose is a major mediator of wall porosity. Wall porosity is also regulated by borate diester-coupled RG-II linkages (O’Neill et al., 1996; Fleischer et al., 1999). In the walls of pollen tubes, which have unique composition and mechanical properties, pectin influences both cell wall porosity and mechanical strength (Derksen et al., 2011). Because the average pore size in cell walls is similar to that of many globular proteins (Carpita et al., 1979), increased wall porosity should correlate with higher diffusion rates and accessibility to wall components for degradative enzymes during biomass processing. A relatively unexplored idea is the extent to which the aforementioned effects of pectin on wall rigidity might influence the physical properties of biomass during pretreatment. Conceivably, stiffening cell walls by the manipulation of Ca2+-mediated pectin crosslinks might enhance the fracturability of biomass, but experimental support for this idea is currently lacking.
Biofuels from Pectin-Rich Feedstocks
Although lignocellulosic biofuels are a promising renewable energy resource, the recalcitrance of biomass to degradation presents a major roadblock to their production. To increase biofuel yields, one strategy is to improve the conversion efficiency of plant cell walls to bioethanol (Jordan et al., 2012). The conversion process can be simplified by altering lignocellulose composition in bioenergy crop plants through genetic and molecular engineering (Demura and Ye, 2010; Pauly and Keegstra, 2010). Another strategy is to exploit existing plants with large amounts of easily digestible biomass (Somerville et al., 2010). At present, bioethanol is mainly produced from corn in the United States (Jordan et al., 2012), where the government has set a goal to produce 30% of liquid transportation fuels from biomass by 2030 (Demura and Ye, 2010). Like starch, pectins are largely water-soluble and relatively easy to degrade in comparison to other wall components. Pectins are abundant in waste residues of fruits and vegetables, which could be used as feedstocks for ethanol production. These pectin-rich residues have in many cases already been pretreated or processed and contain low lignin levels, which should facilitate the deconstruction of their cell walls and reduce the usage of degradative enzymes (Edwards and Doran-Peterson, 2012). So far, several pectin-rich materials, including sugar beet pulp (Rorick et al., 2011), citrus waste (Lopez et al., 2010; Pourbafrani et al., 2010), and apple pomace (Canteri-Schemin et al., 2005) have been analyzed as bioenergy feedstocks. Recent research has also indicated that potato pulp is an attractive raw material for bioethanol production since it contains abundant polysaccharides (Lesiecki et al., 2012). The use of pectin-rich resources as bioenergy feedstocks will require saccharification and fermentation methods that are optimized for the suite of sugars they contain, and efforts are already underway to generate microbial bioprocessing strains tailored to these materials (Edwards et al., 2011).
Pectin as a High-Value Biomass Co-Product
As a natural complex polysaccharide, pectin plays important industrial roles in several fields. Its physical and chemical properties make it a valuable material in the food and pharmaceutical industries (May, 1990). As a food additive, pectin is mainly used as a gelling agent in jams, a thickening and stabilizing agent in drinks, and as a gelatin substitute in baked foods (Srivastava and Malviya, 2011). Recent work has shown that the field application of pectin-derived oligosaccharides (PDOs) improves the coloration and anthocyanin content of seedless grapes (Ochoa-Villarreal et al., 2011), and recombinant PME has been used to increase the hardness of fruit products and reduce the turbidity of fruit juices (Jiang et al., 2012b).
Pectin is part of the soluble dietary fiber that exists in all fruits and vegetables and is thus beneficial for human health. Pectin consumption has been demonstrated to reduce blood cholesterol levels in humans, although the pectins used in these studies were administered at high doses and were not precisely characterized (Brouns et al., 2012). Modified citrus pectin (MCP) has been shown to enhance the immune system’s ability to prevent metastasis (Hurd, 1999) and inhibit cancer cell growth (Nangia-Makker et al., 2002; Jackson et al., 2007; Yan and Katz, 2010; Maxwell et al., 2012). The MCP functions synergistically with other compounds in inhibiting cancer cell growth (Jiang et al., 2012a), which is a promising result for the development of anti-metastatic drugs (Glinsky and Raz, 2009). Specifically, the RG-I component of pectin might contribute to its anticancer activity (Cheng et al., 2012). Because of its structural malleability, biodegradability, and tunable porosity, pectin is also used as a surface modifier for medical devices (Morra et al., 2004) and a material for biomedical applications including drug delivery, gene delivery, and tissue engineering (Munarin et al., 2011, 2012). These applications make pectin, either in its unmodified or derivatized forms, a potentially high-value component of biomass (Figure 1C).
Conclusion
Pectins are one of the most structurally complex classes of molecules in nature, and it is perhaps due to this complexity that they serve a multitude of functions during plant growth and development. Depending on the feedstock, processing regime, and desired end products, pectin can be viewed either as a hindrance to biomass degradability, a source of fermentable sugars in its own right, or a potentially valuable co-product of biofuel production. A more comprehensive understanding of pectin structure and the mechanisms of its synthesis, modification, and degradation will allow for the enhancement of efforts to grow and utilize plants as renewable sources of food, materials, and energy.
Conflict of Interest Statement
The authors declare that the research was conducted in the absence of any commercial or financial relationships that could be construed as a potential conflict of interest.
Acknowledgments
Thanks to Thomas McCarthy and Daniel McClosky for helpful comments. Support for this work was provided as part of the Center for Lignocellulose Structure and Formation, an Energy Frontier Research Center funded by the U.S. Department of Energy, Office of Science, Office of Basic Energy Sciences under Award Number DE-SC0001090.
References
Atkinson, R. G., Schroder, R., Hallett, I. C., Cohen, D., and Macrae, E. A. (2002). Overexpression of polygalacturonase in transgenic apple trees leads to a range of novel phenotypes involving changes in cell adhesion. Plant Physiol. 129, 122–133.
Baron-Epel, O., Gharyal, P. K., and Schindler, M. (1988). Pectins as mediators of wall porosity in soybean cells. Planta 175, 389–395.
Bouton, S., Leboeuf, E., Mouille, G., Leydecker, M. T., Talbotec, J., Granier, F., et al. (2002). Quasimodo1 encodes a putative membrane-bound glycosyltransferase required for normal pectin synthesis and cell adhesion in Arabidopsis. Plant Cell 14, 2577–2590.
Brouns, F., Theuwissen, E., Adam, A., Bell, M., Berger, A., and Mensink, R. P. (2012). Cholesterol-lowering properties of different pectin types in mildly hyper-cholesterolemic men and women. Eur. J. Clin. Nutr. 66, 591–599.
Bush, M. S., Marry, M., Huxham, I. M., Jarvis, M. C., and Mccann, M. C. (2001). Developmental regulation of pectic epitopes during potato tuberisation. Planta 213, 869–880.
Canteri-Schemin, M. H., Fertonani, H. C. R., Waszczynskyj, N., and Wosiacki, G. (2005). Extraction of pectin from apple pomace. Braz. Arch. Biol. Technol. 48, 259–266.
Carpita, N., Sabularse, D., Montezinos, D., and Delmer, D. P. (1979). Determination of the pore size of cell walls of living plant cells. Science 205, 1144–1147.
Cheng, H., Zhang, Z., Leng, J., Liu, D., Hao, M., Gao, X., et al. (2012). The inhibitory effects and mechanisms of rhamnogalacturonan I pectin from potato on HT-29 colon cancer cell proliferation and cell cycle progression. Int. J. Food Sci. Nutr. 64, 36–43.
Demura, T., and Ye, Z. H. (2010). Regulation of plant biomass production. Curr. Opin. Plant Biol. 13, 299–304.
Derbyshire, P., Mccann, M. C., and Roberts, K. (2007). Restricted cell elongation in Arabidopsis hypocotyls is associated with a reduced average pectin esterification level. BMC Plant Biol. 7:31. doi: 10.1186/1471-2229-7-31
Derksen, J., Janssen, G. J., Wolters-Arts, M., Lichtscheidl, I., Adlassnig, W., Ovecka, M., et al. (2011). Wall architecture with high porosity is established at the tip and maintained in growing pollen tubes of Nicotiana tabacum. Plant J. 68, 495–506.
Dick-Perez, M., Zhang, Y. A., Hayes, J., Salazar, A., Zabotina, O. A., and Hong, M. (2011). Structure and interactions of plant cell-wall polysaccharides by two- and three-dimensional magic-angle-spinning solid-state NMR. Biochemistry 50, 989–1000.
Driouich, A., Follet-Gueye, M. L., Bernard, S., Kousar, S., Chevalier, L., Vicre-Gibouin, M., et al. (2012). Golgi-mediated synthesis and secretion of matrix polysaccharides of the primary cell wall of higher plants. Front. Plant Sci. 3:79. doi: 10.3389/fpls.2012.00079
Edwards, M. C., and Doran-Peterson, J. (2012). Pectin-rich biomass as feedstock for fuel ethanol production. Appl. Microbiol. Biotechnol. 95, 565–575.
Edwards, M. C., Henriksen, E. D., Yomano, L. P., Gardner, B. C., Sharma, L. N., Ingram, L. O., et al. (2011). Addition of genes for cellobiase and pectinolytic activity in Escherichia coli for fuel ethanol production from pectin-rich lignocellulosic biomass. Appl. Environ. Microbiol. 77, 5184–5191.
Fissore, E. N., Ponce, N. M. A., Matkovic, L., Stortz, C. A., Rojas, A. M., and Gerschenson, L. N. (2011). Isolation of pectin-enriched products from red beet (Beta vulgaris L. var. conditiva) wastes: composition and functional properties. Food Sci. Technol. Int. 17, 517–527.
Fleischer, A., O’Neill, M. A., and Ehwald, R. (1999). The pore size of non-graminaceous plant cell walls is rapidly decreased by borate ester cross-linking of the pectic polysaccharide rhamnogalacturonan II. Plant Physiol. 121, 829–838.
Gille, S., and Pauly, M. (2012). O-acetylation of plant cell wall polysaccharides. Front. Plant Sci. 3:12. doi: 10.3389/fpls.2012.00012
Glinsky, V. V., and Raz, A. (2009). Modified citrus pectin anti-metastatic properties: one bullet, multiple targets. Carbohydr. Res. 344, 1788–1791.
Gou, J. Y., Miller, L. M., Hou, G., Yu, X. H., Chen, X. Y., and Liu, C. J. (2012). Acetylesterase-mediated deacetylation of pectin impairs cell elongation, pollen germination, and plant reproduction. Plant Cell 24, 50–65.
Goulao, L. F., Vieira-Silva, S., and Jackson, P. A. (2011). Association of hemicellulose- and pectin-modifying gene expression with Eucalyptus globulus secondary growth. Plant Physiol. Biochem. 49, 873–881.
Harholt, J., Suttangkakul, A., and Vibe Scheller, H. (2010). Biosynthesis of pectin. Plant Physiol. 153, 384–395.
Hongo, S., Sato, K., Yokoyama, R., and Nishitani, K. (2012). Demethylesterification of the primary wall by PECTIN METHYLESTERASE35 provides mechanical support to the Arabidopsis stem. Plant Cell 24, 2624–2634.
Jackson, C. L., Dreaden, T. M., Theobald, L. K., Tran, N. M., Beal, T. L., Eid, M., et al. (2007). Pectin induces apoptosis in human prostate cancer cells: correlation of apoptotic function with pectin structure. Glycobiology 17, 805–819.
Jarvis, M. C., Briggs, S. P. H., and Knox, J. P. (2003). Intercellular adhesion and cell separation in plants. Plant Cell Environ. 26, 977–989.
Jiang, J., Eliaz, I., and Sliva, D. (2012a). Synergistic and additive effects of modified citrus pectin with two polybotanical compounds, in the suppression of invasive behavior of human breast and prostate cancer cells. Integr. Cancer Ther. 12, 145–152.
Jiang, X., Chen, P., Yin, M., and Yang, Q. (2012b). Constitutive expression, purification and characterisation of pectin methylesterase from Aspergillus niger in Pichia pastoris for potential application in the fruit juice industry. J. Sci. Food Agric. 93, 375–381.
Jordan, D. B., Bowman, M. J., Braker, J. D., Dien, B. S., Hector, R. E., Lee, C. C., et al. (2012). Plant cell walls to ethanol. Biochem. J. 442, 241–252.
Knox, J. P., Linstead, P. J., King, J., Cooper, C., and Roberts, K. (1990). Pectin esterification is spatially regulated both within cell-walls and between developing-tissues of root apices. Planta 181, 512–521.
Kobayashi, M., Matoh, T., and Azuma, J. (1996). Two chains of rhamnogalacturonan II are cross-linked by borate-diol ester bonds in higher plant cell walls. Plant Physiol. 110, 1017–1020.
Koizumi, K., Yokoyama, R., and Nishitani, K. (2009). Mechanical load induces upregulation of transcripts for a set of genes implicated in secondary wall formation in the supporting tissue of Arabidopsis thaliana. J. Plant Res. 122, 651–659.
Krupkova, E., Immerzeel, P., Pauly, M., and Schmulling, T. (2007). The TUMOROUS SHOOT DEVELOPMENT2 gene of Arabidopsis encoding a putative methyltransferase is required for cell adhesion and co-ordinated plant development. Plant J. 50, 735–750.
Leboeuf, E., Guillon, F., Thoiron, S., and Lahaye, M. (2005). Biochemical and immunohistochemical analysis of pectic polysaccharides in the cell walls of Arabidopsis mutant QUASIMODO 1 suspension-cultured cells: implications for cell adhesion. J. Exp. Bot. 56, 3171–3182.
Lesiecki, M., Bialas, W., and Lewandowicz, G. (2012). Enzymatic hydrolysis of potato pulp. Acta Sci. Pol. Technol. Aliment. 11, 53–59.
Lewis, M. W., Leslie, M. E., and Liljegren, S. J. (2006). Plant separation: 50 ways to leave your mother. Curr. Opin. Plant Biol. 9, 59–65.
Lionetti, V., Francocci, F., Ferrari, S., Volpi, C., Bellincampi, D., Galletti, R., et al. (2010). Engineering the cell wall by reducing de-methyl-esterified homogalacturonan improves saccharification of plant tissues for bioconversion. Proc. Natl. Acad. Sci. U.S.A. 107, 616–621.
Lopez, J. A. S., Li, Q., and Thompson, I. P. (2010). Biorefinery of waste orange peel. Crit. Rev. Biotechnol. 30, 63–69.
Marcus, S. E., Blake, A. W., Benians, T. A., Lee, K. J., Poyser, C., Donaldson, L., et al. (2010). Restricted access of proteins to mannan polysaccharides in intact plant cell walls. Plant J. 64, 191–203.
Marcus, S. E., Verhertbruggen, Y., Herve, C., Ordaz-Ortiz, J. J., Farkas, V., Pedersen, H. L., et al. (2008). Pectic homogalacturonan masks abundant sets of xyloglucan epitopes in plant cell walls. BMC Plant Biol. 8:60. doi: 10.1186/1471-2229-8-60
Matsunaga, T., Ishii, T., Matsumoto, S., Higuchi, M., Darvill, A., Albersheim, P., et al. (2004). Occurrence of the primary cell wall polysaccharide rhamnogalacturonan II in pteridophytes, lycophytes, and bryophytes. Implications for the evolution of vascular plants. Plant Physiol. 134, 339–351.
Maxwell, E. G., Belshaw, N. J., Waldron, K. W., and Morris, V. J. (2012). Pectin – an emerging new bioactive food polysaccharide. Trends Food Sci. Technol. 24, 64–73.
May, C. D. (1990). Industrial pectins – sources, production and applications. Carbohydr. Polym. 12, 79–99.
Mellerowicz, E. J., and Gorshkova, T. A. (2012). Tensional stress generation in gelatinous fibres: a review and possible mechanism based on cell-wall structure and composition. J. Exp. Bot. 63, 551–565.
Micheli, F. (2001). Pectin methylesterases: cell wall enzymes with important roles in plant physiology. Trends Plant Sci. 6, 414–419.
Min, B., Lim, J., Ko, S., Lee, K. G., Lee, S. H., and Lee, S. (2011). Environmentally friendly preparation of pectins from agricultural byproducts and their structural/rheological characterization. Bioresour. Technol. 102, 3855–3860.
Moore, G. R., and Raine, C. S. (1988). Immunogold localization and analysis of IgG during immune-mediated demyelination. Lab. Invest. 59, 641–648.
Moore, P. J., Swords, K. M., Lynch, M. A., and Staehelin, L. A. (1991). Spatial organization of the assembly pathways of glycoproteins and complex polysaccharides in the Golgi apparatus of plants. J. Cell Biol. 112, 589–602.
Morra, M., Cassinelli, C., Cascardo, G., Nagel, M. D., Della Volpe, C., Siboni, S., et al. (2004). Effects on interfacial properties and cell adhesion of surface modification by pectic hairy regions. Biomacromolecules 5, 2094–2104.
Mouille, G., Ralet, M. C., Cavelier, C., Eland, C., Effroy, D., Hematy, K., et al. (2007). Homogalacturonan synthesis in Arabidopsis thaliana requires a Golgi-localized protein with a putative methyltransferase domain. Plant J. 50, 605–614.
Munarin, F., Guerreiro, S. G., Grellier, M. A., Tanzi, M. C., Barbosa, M. A., Petrini, P., et al. (2011). Pectin-based injectable biomaterials for bone tissue engineering. Biomacromolecules 12, 568–577.
Munarin, F., Tanzi, M. C., and Petrini, P. (2012). Advances in biomedical applications of pectin gels. Int. J. Biol. Macromol. 51, 681–689.
Nangia-Makker, P., Hogan, V., Honjo, Y., Baccarini, S., Tait, L., Bresalier, R., et al. (2002). Inhibition of human cancer cell growth and metastasis in nude mice by oral intake of modified citrus pectin. J. Natl. Cancer Inst. 94, 1854–1862.
Neumetzler, L., Humphrey, T., Lumba, S., Snyder, S., Yeats, T. H., Usadel, B., et al. (2012). The FRIABLE1 gene product affects cell adhesion in Arabidopsis. PLoS ONE 7:e42914. doi: 10.1371/journal.pone.0042914
Ochoa-Villarreal, M., Vargas-Arispuro, I., Islas-Osuna, M. A., Gonzalez-Aguilar, G., and Martinez-Tellez, M. A. (2011). Pectin-derived oligosaccharides increase color and anthocyanin content in Flame Seedless grapes. J. Sci. Food Agric. 91, 1928–1930.
O’Neill, M. A., Warrenfeltz, D., Kates, K., Pellerin, P., Doco, T., Darvill, A. G., et al. (1996). Rhamnogalacturonan-II, a pectic polysaccharide in the walls of growing plant cell, forms a dimer that is covalently cross-linked by a borate ester. In vitro conditions for the formation and hydrolysis of the dimer. J. Biol. Chem. 271, 22923–22930.
Orfila, C., Dal Degan, F., Jorgensen, B., Scheller, H. V., Ray, P. M., and Ulvskov, P. (2012). Expression of mung bean pectin acetyl esterase in potato tubers: effect on acetylation of cell wall polymers and tuber mechanical properties. Planta 236, 185–196.
Pakarinen, A., Zhang, J., Brock, T., Maijala, P., and Viikari, L. (2012). Enzymatic accessibility of fiber hemp is enhanced by enzymatic or chemical removal of pectin. Bioresour. Technol. 107, 275–281.
Parker, C. C., Parker, M. L., Smith, A. C., and Waldron, K. W. (2001). Pectin distribution at the surface of potato parenchyma cells in relation to cell–cell adhesion. J. Agric. Food Chem. 49, 4364–4371.
Parsons, H. T., Christiansen, K., Knierim, B., Carroll, A., Ito, J., Batth, T. S., et al. (2012). Isolation and proteomic characterization of the Arabidopsis Golgi defines functional and novel components involved in plant cell wall biosynthesis. Plant Physiol. 159, 12–26.
Pauly, M., and Keegstra, K. (2010). Plant cell wall polymers as precursors for biofuels. Curr. Opin. Plant Biol. 13, 305–312.
Peaucelle, A., Braybrook, S., and Hofte, H. (2012). Cell wall mechanics and growth control in plants: the role of pectins revisited. Front. Plant Sci. 3:121. doi: 10.3389/fpls.2012.00121
Peaucelle, A., Braybrook, S. A., Le Guillou, L., Bron, E., Kuhlemeier, C., and Hofte, H. (2011). Pectin-induced changes in cell wall mechanics underlie organ initiation in Arabidopsis. Curr. Biol. 21, 1720–1726.
Pelletier, S., Van Orden, J., Wolf, S., Vissenberg, K., Delacourt, J., Ndong, Y. A., et al. (2010). A role for pectin de-methylesterification in a developmentally regulated growth acceleration in dark-grown Arabidopsis hypocotyls. New Phytol. 188, 726–739.
Pourbafrani, M., Forgacs, G., Horvath, I. S., Niklasson, C., and Taherzadeh, M. J. (2010). Production of biofuels, limonene and pectin from citrus wastes. Bioresour. Technol. 101, 4246–4250.
Rhee, S. Y., and Somerville, C. R. (1998). Tetrad pollen formation in quartet mutants of Arabidopsis thaliana is associated with persistence of pectic polysaccharides of the pollen mother cell wall. Plant J. 15, 79–88.
Ridley, B. L., O’Neill, M. A., and Mohnen, D. (2001). Pectins: structure, biosynthesis, and oligogalacturonide-related signaling. Phytochemistry 57, 929–967.
Rorick, R., Nahar, N., and Pryor, S. W. (2011). Ethanol production from sugar beet pulp using Escherichia coli KO11 and Saccharomyces cerevisiae. Biol. Eng. 3, 199–209.
Sexton, T. R., Henry, R. J., Harwood, C. E., Thomas, D. S., Mcmanus, L. J., Raymond, C., et al. (2012). Pectin methylesterase genes influence solid wood properties of Eucalyptus pilularis. Plant Physiol. 158, 531–541.
Shevell, D. E., Kunkel, T., and Chua, N. H. (2000). Cell wall alterations in the Arabidopsis emb30 mutant. Plant Cell 12, 2047–2060.
Siedlecka, A., Wiklund, S., Peronne, M. A., Micheli, F., Lesniewska, J., Sethson, I., et al. (2008). Pectin methyl esterase inhibits intrusive and symplastic cell growth in developing wood cells of Populus. Plant Physiol. 146, 554–565.
Singh, S. K., Eland, C., Harholt, J., Scheller, H. V., and Marchant, A. (2005). Cell adhesion in Arabidopsis thaliana is mediated by ECTOPICALLY PARTING CELLS 1 – a glycosyltransferase (GT64) related to the animal exostosins. Plant J. 43, 384–397.
Sobry, S., Havelange, A., and Van Cutsem, P. (2005). Immunocytochemistry of pectins in shoot apical meristems: consequences for intercellular adhesion. Protoplasma 225, 15–22.
Somerville, C., Youngs, H., Taylor, C., Davis, S. C., and Long, S. P. (2010). Feedstocks for lignocellulosic biofuels. Science 329, 790–792.
Srivastava, P., and Malviya, R. (2011). Sources of pectin, extraction and its applications in pharmaceutical industry – an overview. Indian J. Nat. Prod. Resour. 2, 11–18.
Thompson, A. J., Tor, M., Barry, C. S., Vrebalov, J., Orfila, C., Jarvis, M. C., et al. (1999). Molecular and genetic characterization of a novel pleiotropic tomato-ripening mutant. Plant Physiol. 120, 383–389.
Touzel, J. P., Chabbert, B., Monties, B., Debeire, P., and Cathala, B. (2003). Synthesis and characterization of dehydrogenation polymers in Gluconacetobacter xylinus cellulose and cellulose/pectin composite. J. Agric. Food Chem. 51, 981–986.
Vincken, J. P., Schols, H. A., Oomen, R. J., Mccann, M. C., Ulvskov, P., Voragen, A. G., et al. (2003). If homogalacturonan were a side chain of rhamnogalacturonan I. Implications for cell wall architecture. Plant Physiol. 132, 1781–1789.
Westermark, U., Hardell, H. L., and Iversen, T. (1986). The content of protein and pectin in the lignified middle lamella primary wall from spruce fibers. Holzforschung 40, 65–68.
Willats, W. G., Gilmartin, P. M., Mikkelsen, J. D., and Knox, J. P. (1999). Cell wall antibodies without immunization: generation and use of de-esterified homogalacturonan block-specific antibodies from a naive phage display library. Plant J. 18, 57–65.
Willats, W. G., Mccartney, L., Mackie, W., and Knox, J. P. (2001). Pectin: cell biology and prospects for functional analysis. Plant Mol. Biol. 47, 9–27.
Wolf, S., Mouille, G., and Pelloux, J. (2009). Homogalacturonan methyl-esterification and plant development. Mol. Plant 2, 851–860.
Yan, J., and Katz, A. (2010). PectaSol-C modified citrus pectin induces apoptosis and inhibition of proliferation in human and mouse androgen-dependent and -independent prostate cancer cells. Integr. Cancer Ther. 9, 197–203.
Keywords: pectin, cell wall, cell adhesion, gelling, biomass, lignocellulosic biofuel
Citation: Xiao C and Anderson CT (2013) Roles of pectin in biomass yield and processing for biofuels. Front. Plant Sci. 4:67. doi: 10.3389/fpls.2013.00067
Received: 14 January 2013; Accepted: 10 March 2013;
Published online: 27 March 2013.
Edited by:
Samuel P. Hazen, University of Massachusetts, USAReviewed by:
Henrik Scheller, Lawrence Berkeley National Laboratory, USAHerman Höfte, Institut National de la Recherche Agronomique, France
Copyright: © 2013 Xiao and Anderson. This is an open-access article distributed under the terms of the Creative Commons Attribution License, which permits use, distribution and reproduction in other forums, provided the original authors and source are credited and subject to any copyright notices concerning any third-party graphics etc.
*Correspondence: Charles T. Anderson, Department of Biology, The Pennsylvania State University, 201 Huck Life Sciences Building, University Park, PA 16802, USA. e-mail: cta3@psu.edu