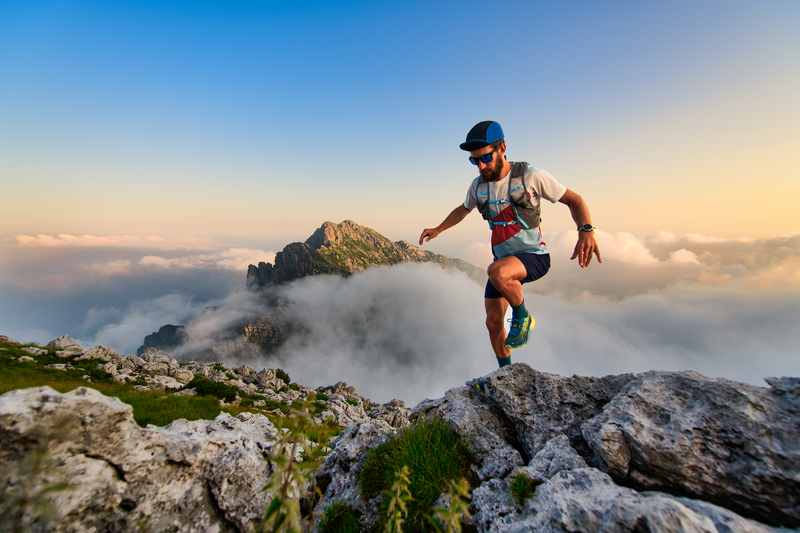
95% of researchers rate our articles as excellent or good
Learn more about the work of our research integrity team to safeguard the quality of each article we publish.
Find out more
OPINION article
Front. Plant Sci. , 05 July 2012
Sec. Plant Physiology
Volume 3 - 2012 | https://doi.org/10.3389/fpls.2012.00141
This article is part of the Research Topic RNA Signaling in Plants View all 8 articles
At the beginning of the last decade, it was believed that animal and plant genomes encoded, on average, 30,000 genes, surrounded by junk non-coding sequences. The dogma “from DNA to RNA to proteins” assigned RNA the role of transducer of genetic information into proteins. Large-scale studies have demonstrated that non-protein-coding RNAs are a massive output of transcription and can be regarded as regulatory RNAs. Following this new understanding, DNA is a static element of genetic information, a hardware, while RNA, in the form of multiple species of short and long non-coding transcripts constitutes the active information, the software (Hayashizaki and Carninci, 2006; Pang et al., 2007). The ability of RNAs to orchestrate chromatin states, DNA transcription, differential splicing, RNA translation, post-transcriptional modification, and protein stability determines a hidden layer of complexity of genetic information.
At the end of the last decade, microRNAs were identified by their role in regulation of proliferation and programmed cell death during the developmental stages of Caenorhabditis elegans (Grishok et al., 2001). In both plants and animals, microRNAs negatively affect their targets through a variety of transcriptional and post-transcriptional mechanisms.
Small RNAs (sRNAs) in plants include microRNAs, siRNAs (small interfering RNAs), tasi-RNA (trans-acting siRNAs), and nat-siRNAs (natural antisense-mediated siRNAs). The presence of methylated 3′ OH ends caused an under-representation of sRNAs in the analysis of RNA composition in plants, producing a bias in RNAs that were sequenced using tag sequencing and next-generation sequencing methods.
Today, sRNAs include an expanding number of 20–40 nt RNAs that function in the regulation of gene expression by affecting mRNA decay and translational inhibition, or lead to DNA methylation and gene silencing. They generally involve double-stranded RNA or stem loops and imply transcriptional or post-transcriptional gene silencing (PTGS). Gene silencing in plants can be mediated by siRNA, microRNA, tasi-RNAs, and nat-siRNAs. These RNAs act as single-stranded filaments incorporated into the effector complexes (RISC, RNA-induced silencing complex) containing one of the Argonaute enzymes degrading the target RNA complementary to sRNA (Bartel, 2009). DNA-dependent RNA polymerase IV (Pol IV)-dependent sRNAs are 24-nt and associated with AGO4, whereas the majority of the potential Pol V-dependent sRNAs are 21-nt and bound to AGO1, suggesting the potential involvement of AGO1 in Pol V-related pathways (Dunoyer et al., 2010a,b; Wang et al., 2011a,b). Their role is in the amplification of signals such as RNA-primed RNA amplification, and in the spread of anti-viral RNA interference mechanisms.
In higher plants, a number of physiological processes are regulated by the transfer of signaling molecules through the phloem. This phloem-mediated remote-control system provides specific and efficient regulation to fine-tune many plant developmental programs. In addition to hormones, proteins, and small peptides, phloem is rich in RNA-binding proteins that function as chaperones for systemic RNA movement.
In 2004, an international consortium within the EU VI Framework Program, “Riboreg”1, supported the production of the first Arabidopsis non-coding RNA macroarrays, that characterized their regulation in different tissues and in plants subjected to environmental stresses (Laporte et al., 2007; Bardou et al., 2011).
Massively Parallel Signature Sequencing (MPSS, Lynx Technologies) was used before the advent of next-generation sequencing as a high-throughput method to identify short transcripts devoid of poly-A tails, as microRNAs and sRNAs (Sunkar and Zhou, 2004). In September 2005, Science published a monograph on non-coding RNAs the first analysis of a large number of small transcribed Arabidopsis RNAs using MPSS (Lu et al., 2005).
In this timeframe, findings on viroids and the movement of viral proteins through the phloem induced a revision of the phloem function. Viruses and other macromolecules are transported through plasmodesmata. Virus movement requires virus-encoded proteins that interact with host factors. Different strategies of movement are exploited by different viruses. These mechanisms were recently reviewed (Atkins et al., 2011).
It appeared evident that RNA-binding proteins in phloem were important in facilitating the movement of RNA interference mediators. Comprehensive analyses of RNA molecules present in the phloem sap were carried out (Yoo et al., 2004; Aoki et al., 2005; Omid et al., 2008; Burgyan and Havelda, 2011). Scientists further identified phloem-specific RNA-binding proteins (19) and a large population of sRNAs including known microRNAs and various endogenous siRNA species (20). For instance, phloem small-RNA-binding protein 1 (PSRP1) was subsequently shown to bind and facilitate movement of single-stranded sRNA molecules between cells (Ham et al., 2009). CmPP16 protein from Cucurbita maxima was shown to possess properties similar to those of viral movement proteins (Aoki et al., 2005). Plants deploy RNA silencing, R-gene-mediated defense and other mechanisms to prevent phloem transport of viruses. Plant viruses use sieve elements in phloem as the route of long-distance movement and systemic infection in plants, and viral RNA-binding proteins are exploited by viruses to counteract the plant RNA silencing machinery (Lakatos et al., 2004; Zhang et al., 2009). Thus, phloem is like a highway that is able to target macromolecules across plant tissues through specific cargo proteins (Takeda and Ding, 2009; Cao et al., 2010) and influences the systemic spread of silencing (Vuorinen et al., 2011). Voinnet and Baulcombe independently contributed to the understanding of RNA silencing, especially in the anti-viral response, by reviewing the difference in the two mechanisms of extensive local spread and system signaling in RNA interference (Voinnet and Baulcombe, 1997; Baulcombe, 2004; Brosnan and Voinnet, 2011; Melnyk et al., 2011). Silencing spreads systemically through the phloem system of the plants, which also translocates metabolites from source to sink tissues. Grafting experiments were essential to allow a clear separation between the silencing signal-producing (source) tissues and the signal-receiving (sink) tissues (Kalantidis et al., 2008). Unlike the short-range silencing signal, there is still little knowledge on the mediators of systemic silencing, which is possibly a cooperative activity mediated by different RNA molecules. Whatever the identity of the systemic signals (siRNA precursors with size ranging form 22 to 40 nt), it is assumed to move through the phloem (Baulcombe, 2004; Moissiard et al., 2007). This was confirmed by studies using a phloem flow tracer (Tournier et al., 2006), which also allowed for a more detailed analysis of the specific properties of RNA spread.
The literature on phloem as a highway for macromolecules is expanding (Lough and Lucas, 2006; Chuck and O’Connor, 2010; Chitwood and Timmermans, 2010; Dinant and Lemoine, 2010). While RNA sequencing and bioinformatic analysis have provided information on microRNAs present in plant phloem (Buhtz et al., 2008; Deeken et al., 2008), several microRNAs have been shown to move through the phloem to exert their activity at a distance. For example, miR166 was shown to act at distance and to induce maize leaf polarity (Juarez et al., 2004), and miR172 is present in sRNA libraries made from phloem exudates, acting as part of a long-distance signaling network (Zeevaart, 2008). It moves between tissues upon grafting and promotes tuberization in potato (Martin et al., 2009). On the other hand, miR319 moves from leaves to roots where it targets a subset of the TCP family of transcription factors that regulates LOX2 expression (Yoo et al., 2004; Schommer et al., 2008; Buhtz et al., 2010).
Phloem-specific sRNAs were also found to travel and inform the roots of the nutrient status of the shoot. The levels of three miRNAs known to respond to nutrient deprivation in non-vascular tissue, miR395 (sulfate), miR398 (copper), and miR399 (phosphate), were increased in phloem sap during the growth of plants under the respective starvation conditions (Buhtz et al., 2008). Plants regulate inorganic phosphate (Pi) homeostasis with cross-talk between roots, stem, and leaves to adapt to environmental changes in Pi availability. During phosphate-limitation conditions, plants respond with increased phosphate uptake from the soil and phosphate mobilization from the leaf. Upon Pi starvation, miR399 is synthesized in leaves and travels along the phloem to the roots, where it cleaves its target gene, PHO2, in A. thaliana, a ubiquitin-conjugating E2 enzyme, thereby releasing several protein targets from ubiquitinylation-dependent degradation and increasing Pi content in the shoots (Franco-Zorrilla et al., 2007). Inhibition of miR399 causes depletion of phosphate in the leaves. MicroRNAs become unable to bind to target mRNAs for the presence of RNA mimics possessing similar target sequences. IPS1 (induced by phosphate starvation1) RNA modulates the availability of miR399 sequestering miR399 releasing from inhibition PHO2 mRNA. A similar mechanism of “target mimicry” was proposed for the anti-mir sponges, able to sequester and inactivate complementary microRNAs. Artificial target mimic sites in plants could indeed reduce translation efficiency in cis (Todesco et al., 2010).
Several messenger RNAs travel a long-distance in the phloem, such as BEL5, LeT6, KNOTTED1, DELLA-GAI, and FLOWERING LOCUS T (FT) mRNA (Kehr and Buhtz, 2010). FT is a signal molecule that acts at a distance, producing flowering in the leaf meristems to induce floral development. FT protein may require FT mRNA together with phloem shuttle proteins to form a complex that is transported through phloem translocation stream to the shoot apical meristem (SAM). This was shown using non-conventional approaches that exploit virus-induced RNA silencing and meristem exclusion of virus infection (Li et al., 2009, 2011). Thus, from the FT mRNA structure and from other RNA sequences, it was shown that the tertiary structure is important to define their function (Zhong et al., 2007; Chitwood and Timmermans, 2010).
Omics and bioinformatics are essential to understanding the molecular systems that underlie various plant functions. Recent sequencing technologies have revitalized sequencing approaches in genomics and have produced opportunities for various emerging analytical applications. A new EU FP7 project now started, AB-Stress, will study the epigenetic regulation and the stress induced sRNA world in legumes during biotic and abiotic stresses2. Several new-omics layers such as the interactome, epigenome, peptidome, and hormonome are taking the lead that will open new perspectives on the complex regulation of communication between plant tissues and in signaling at a distance.
Aoki, K., Suzui, N., Fujimaki, S., Dohmae, N., Yonekura-Sakakibara, K., Fujiwara, T., Hayashi, H., Yamaya, T., and Sakakibara, H. (2005). Destination-selective long-distance movement of phloem proteins. Plant Cell 17, 1801–1814.
Atkins, C. A., Smith, P. M., and Rodriguez-Medina, C. (2011). Macromolecules in phloem exudates – a review. Protoplasma 248, 165–172.
Bardou, F., Merchan, F., Ariel, F., and Crespi, M. (2011). Dual RNAs in plants. Biochimie 93, 1950–1954.
Brosnan, C. A., and Voinnet, O. (2011). Cell-to-cell and long-distance siRNA movement in plants: mechanisms and biological implications. Curr. Opin. Plant Biol. 14, 580–587.
Buhtz, A., Pieritz, J., Springer, F., and Kehr, J. (2010). Phloem small RNAs, nutrient stress responses, and systemic mobility. BMC Plant Biol. 10, 64. doi: 10.1186/1471-2229-10-64
Buhtz, A., Springer, F., Chappell, L., Baulcombe, D. C., and Kehr, J. (2008). Identification and characterization of small RNAs from the phloem of Brassica napus. Plant J. 53, 739–749.
Burgyan, J., and Havelda, Z. (2011). Viral suppressors of RNA silencing. Trends Plant Sci. 16, 265–272.
Cao, M., Ye, X., Willie, K., Lin, J., Zhang, X., Redinbaugh, M. G., Simon, A. E., Morris, T. J., and Qu, F. (2010). The capsid protein of Turnip crinkle virus overcomes two separate defense barriers to facilitate systemic movement of the virus in Arabidopsis. J. Virol. 84, 7793–7802.
Chuck, G., and O’Connor, D. (2010). Small RNAs going the distance during plant development. Curr. Opin. Plant Biol. 13, 40–45.
Deeken, R., Ache, P., Kajahn, I., Klinkenberg, J., Bringmann, G., and Hedrich, R. (2008). Identification of Arabidopsis thaliana phloem RNAs provides a search criterion for phloem-based transcripts hidden in complex datasets of microarray experiments. Plant J. 55, 746–759.
Dinant, S., and Lemoine, R. (2010). The phloem pathway: new issues and old debates. C. R. Biol. 333, 307–319.
Dunoyer, P., Brosnan, C. A., Schott, G., Wang, Y., Jay, F., Alioua, A., Himber, C., and Voinnet, O. (2010a). An endogenous, systemic RNAi pathway in plants. EMBO J. 29, 1699–1712.
Dunoyer, P., Schott, G., Himber, C., Meyer, D., Takeda, A., Carrington, J. C., and Voinnet, O. (2010b). Small RNA duplexes function as mobile silencing signals between plant cells. Science 328; 912–916
Franco-Zorrilla, J. M., Valli, A., Todesco, M., Mateos, I., Puga, M. I., Rubio-Somoza, I., Leyva, A., Weigel, D., García, J. A., and Paz-Ares, J. (2007). Target mimicry provides a new mechanism for regulation of microRNA activity. Nat. Genet. 39, 1033–1037.
Grishok, A., Pasquinelli, A. E., Conte, D., Parrish, S., Ha, I., Baillie, D. L., Fire, A., Ruvkun, G., and Mello, C. C. (2001). Genes and mechanisms related to RNA interference regulate expression of the small temporal RNAs that control C. elegans developmental timing. Cell 106, 23–34.
Ham, B. K., Brandom, J. L., Xoconostle-Cázares, B., Ringgold, V., Lough, T. J., and Lucas, W. J. (2009). A polypyrimidine tract binding protein, pumpkin RBP50, forms the basis of a phloem-mobile ribonucleoprotein complex. Plant Cell 21, 197–215.
Hayashizaki, Y., and Carninci, P. (2006). Genome network and FANTOM3: assessing the complexity of the transcriptome. PLoS Genet. 2, e63, 492–497. doi:10.1371/journal.pgen.0020063
Juarez, M. T., Kui, J. S., Thomas, J., Heller, B. A., and Timmermans, M. C. P. (2004). microRNA-mediated repression of rolled leaf1 specifies maize leaf polarity. Nature 428, 84–88.
Kalantidis, K., Schumacher, H. T., Alexiadis, T., and Hem, J. M. (2008). RNA silencing movement in plants. Biol. Cell 100, 13–26.
Kehr, J., and Buhtz, A. (2010). Long distance transport and movement of RNA through the phloem. J. Exp. Bot. 59, 85–92.
Lakatos, L., Szittya, G., Silhavy, D., and Burgyán, J. (2004). Molecular mechanism of RNA silencing suppression mediated by p19 protein of tombusviruses. EMBO J. 23, 876–884.
Laporte, P., Merchan, F., Amor, B. B., Wirth, S., and Crespi, M. (2007). Riboregulators in plant development. Biochem. Soc. Trans. 35, 1638–1642.
Li, C., Gu, M., Shi, N., Zhang, H., Yang, X., Osman, T., Liu, Y., Wang, H., Vatish, M., Jackson, S., and Hong, Y. (2011). Mobile FT mRNA contributes to the systemic florigen signalling in floral induction. Sci. Rep. 1, 73.
Li, C., Zhang, K., Zeng, X., Jackson, S., Zhou, Y., and Hong, Y. (2009). A cis Element within Flowering Locus T mRNA determines its mobility and facilitates trafficking of heterologous viral RNA. J. Virol. 83, 3540–3548.
Lough, T. J., and Lucas, W. J. (2006). Integrative plant biology: role of phloem long-distance macromolecular trafficking. Annu. Rev. Plant Biol. 53, 203–232.
Lu, C., Tej, S. S., Luo, S., Haudenschild, C. D., Meyers, B. C., and Green, P. J. (2005). Elucidation of the small RNA component of the transcriptome. Science 309, 1567–1569.
Martin, A., Adam, H., Díaz-Mendoza, M., Zurczak, M., González-Schain, N. D., and Suárez-López, P. (2009). Graft-transmissible induction of potato tuberization by the microRNA miR172. Development 136, 2873–2881.
Melnyk, C. W., Molnar, A., and Baulcombe, D. C. (2011). Intercellular and systemic movement of RNA silencing signals. EMBO J. 30, 3553–3563.
Moissiard, G., Parizotto, E. A., Himber, C., and Voinnet, O. (2007). Transitivity in Arabidopsis can be primed, requires the redundant action of the antiviral Dicer-like 4 and Dicer-like 2, and is compromised by viral-encoded suppressor proteins. RNA 13, 1268–1278.
Omid, A., Malter, D., Peleg, G., and Wolf, S. (2008). Long-distance trafficking of macromolecules in the phloem. Plant Signal. Behav. 3, 260–262.
Pang, K. C., Stephen, S., Dinger, M.E., Engrstrom, P.G., Lehnard, B., and Mattick, J. S. (2007). RNAdb 2.0 – an expanded database of mammalian non-coding RNAs. Nucleic Acid Res. 35, D178–D182.
Schommer, C., Palatnik, J., Aggarwal, P., Chetelat, A., Cubas, P., Farmer, E. E., Nath, U., and Weigel, D. (2008). Control of jasmonate biosynthesis and senescence by miR319 targets. PLoS Biol. 6, e230. doi: 10.1371/journal.pbio.0060230
Sunkar, R., and Zhou, J. K. (2004). Novel and stress-regulated microRNAs and other small RNAs from Arabidopsis. Plant Cell 16, 2001–2019.
Takeda, R., and Ding, B. (2009). Viroid intercellular trafficking: RNA motifs, cellular factors and broad impacts. Viruses 1, 210–221.
Todesco, M., Rubio-Somoza, I., Paz-Ares, J., and Weigel, D. (2010). A collection of target mimics for comprehensive analysis of microRNA function in Arabidopsis thaliana. PLoS Genet. 6, e1001031. doi: 10.1371/journal.pgen.1001031
Tournier, B., Tabler, M., and Kalantidis, K. (2006). Phloem flow strongly influences the systemic spread of silencing in GFP Nicotiana benthamiana plants. Plant J. 47, 383–394.
Vuorinen, A. L., Kelloniemi, J., and Valkonen, J. P. (2011). Why do viruses need phloem for systemic invasion of plants? Plant Sci. 181, 355–363.
Wang, H., Zhang, X., Liu, J., Kiba, T., Woo, J., Ojo, T., Hafner, M., Tuschl, T., Chua, N. H., and Wang, X. J. (2011a). Deep sequencing of small RNAs specifically associated with Arabidopsis AGO1 and AGO4 uncovers new AGO functions. Plant J. 67, 292–304.
Wang, X. B., Jovel, J., Udomporn, P., Wang, Y., Wu, Q., Li, W. X., Gasciolli, V., Vaucheret, H., and Ding, S. W. (2011b). The 21-nucleotide, but not 22-nucleotide, viral secondary small interfering RNAs direct potent antiviral defense by two cooperative Argonautes in Arabidopsis thaliana. Plant Cell 23, 625–638.
Xoconostle-Cázares, B., Xiang, Y., Ruiz-Medrano, R., Wang, H. L., Monzer, J., Yoo, B. C., McFarland, K. C., Franceschi, V. R., and Lucas, W. J. (1999). Plant paralog to viral movement protein that potentiates transport of mRNA into the phloem. Science 283, 94–98.
Yoo, B. C., Kragler, F., Varkonyi-Gasic, E., Haywood, V., Archer-Evans, S., Lee, Y. M., Lough, T. J., and Lucas, W. J. (2004). A systemic small RNA signaling system in plants. Plant Cell 16, 1979–2000.
Zhang, S., Sun, L., and Kragler, F. (2009). The phloem-delivered RNA pool contains small noncoding RNAs and interferes with translation. Plant Physiol. 150, 378–387.
Citation: Poltronieri P and Santino A (2012) Non-coding RNAs in intercellular and systemic signaling. Front. Plant Sci. 3:141. doi: 10.3389/fpls.2012.00141
Received: 17 May 2012; Accepted: 11 June 2012;
Published online: 05 July 2012.
Edited by:
Yiguo Hong, Hangzhou Normal University, ChinaReviewed by:
Yiguo Hong, Hangzhou Normal University, ChinaCopyright: © 2012 Poltronieri and Santino. This is an open-access article distributed under the terms of the Creative Commons Attribution License, which permits use, distribution and reproduction in other forums, provided the original authors and source are credited and subject to any copyright notices concerning any third-party graphics etc.
*Correspondence:cGFsbWlyby5wb2x0cm9uaWVyaUBpc3BhLmNuci5pdA==
Disclaimer: All claims expressed in this article are solely those of the authors and do not necessarily represent those of their affiliated organizations, or those of the publisher, the editors and the reviewers. Any product that may be evaluated in this article or claim that may be made by its manufacturer is not guaranteed or endorsed by the publisher.
Research integrity at Frontiers
Learn more about the work of our research integrity team to safeguard the quality of each article we publish.