- Department of Biotechnology, Agricultural Research Institute for South-East Region, Saratov, Russia
Heritable changes of phenotype arising in plant ontogenesis by the influence of environmental factors belong to the most intriguing genetic phenomena. An unusual inheritance pattern was detected during examination of male fertility restoration in the CMS-inducing “9E” type cytoplasm of sorghum: Rf-genes were functional in self-pollinated progeny of F1 hybrids yet were either not expressed or poorly expressed in backcrosses of these hybrids to CMS-lines with the same cytoplasm type. In experiments on parallel growing of the same F1 hybrid combinations in the “dry plot” and in the “irrigated plot,” it was found that high level of plant water availability during panicle and pollen developmental stages significantly increased male fertility of F1 and test-cross hybrid populations, in which fertility-restoring genes were in heterozygote state, whereas in F2 populations the influences of water availability conditions cause less pronounce effects. Similarly, male-sterile F1 plants, being transferred from the “dry plot” to greenhouse, produced male-fertile panicles. In addition, male-sterile plants from F2 families, which segregated-out as recessives, being transferred to greenhouse also produced male-fertile panicles. In the progenies of these revertants that were grown in field conditions and in the “dry plot,” stable inheritance of male fertility for three cycles of self-pollination was observed, and a number of stable fertile lines in the “9E” cytoplasm were obtained. However, in test-crosses of these fertile lines to CMS-lines with the “9E” cytoplasm restoration of male fertility was not observed, except the progeny of one revertant that behaved as fertility-restorer line. These data suggest that the functional state of fertility-restoring genes for the “9E” sorghum cytoplasm is epigenetically regulated trait established by the influence of environmental factors and is transmitted to sexual generations.
Introduction
Interaction of plant genotype with environment is one of the central problems of plant genetics. According to modern data, environmental factors, such as, temperature, water availability, lighting conditions, mineral nutrition regulate expression of plant genes affecting diverse epigenetic processes – DNA methylation, histone modification, micro-RNA formation (Grant-Downton and Dickinson, 2005; Pfluger and Wagner, 2007; Phillips et al., 2007; King et al., 2010; Fisher and Franklin, 2011; Wang et al., 2011). These effects in certain cases, may be stable and are maintained both in vegetative propagation and in sexual reproduction (Grant-Downton and Dickinson, 2006; Takeda and Paszkowski, 2006; Daxinger and Whitelaw, 2010; D:Hauser:2011]).
Nuclear and cytoplasmic (mitochondrial) genes involved in genetic control of cytoplasmic male sterility (CMS) – maternally heritable failure to develop fertile pollen – belong to genetic systems, which are strongly sensitive to environmental factors. It is generally accepted that CMS is caused be expression of specific mitochondrial genes originating from high recombination activity peculiar to mitochondrial genome (Chase and Gabay-Laughnan, 2004; Hanson and Bentolila, 2004; Fujii and Toriyama, 2008). These genes encode proteins impairing mitochondrial functions at the stage of microsporogenesis and/or microgametogenesis. However, expression of these genes takes place only in hybrid combinations, when they interact with alien nuclear genomes. In fertile lines-donors of CMS-inducing cytoplasms (euplasmic lines), functioning of CMS-inducing genes is inhibited by nuclear fertility-restoring genes, which suppress expression of these genes at the transcriptional or post-transcriptional level. Such fertility-restoring genes usually have dominant mode of expression.
Recently obtained experimental data also suggest CMS to be caused by retrograde regulation from mitochondrial genome of expression of nuclear genes involved in formation of fertile pollen (Fujii and Toriyama, 2008; Yang et al., 2008). This hypothesis seems to be true for those CMS types, which originate from interaction of genetically remote nuclear and cytoplasmic genomes. Such CMS types were obtained, in particular, in sorghum [Sorghum bicolor (L.) Moench], which presents great number of subspecies and races that can be crossed giving rise to progeny that combines nuclear and cytoplasmic genomes of genetically remote parents. These types of CMS differ by mechanisms of pollen degeneration, phenotypes of sterile anthers, reaction to fertility-restorer lines, mode of action of fertility-restoring genes, types of mitochondrial, and chloroplast DNAs etc. (Pring et al., 1995). Assuming significant role of retrograde signaling from chloroplasts (Fernandez and Strand, 2008; Chan et al., 2010) and mitochondria (Atkin and Macherel, 2009), such genetically remote nuclear-cytoplasmic combinations may disturb cross-talk between nuclear and cytoplasmic genomes that may result in sensitivity to biotic and abiotic stresses, in particular, in drought stress response. In this connection, one may suggest that such impacts of environmental factors could down-regulate specific nuclear gene(s) involved in genetic control of pollen development.
In our investigations on genetics of male fertility restoration in some types of CMS-inducing cytoplasms of sorghum (9E, A4, M35-1A) we found an unusual inheritance pattern: the Rf-genes function in the self-pollinated progenies of F1 hybrids but are not expressed or poorly expressed in backcrosses of these hybrids to parental CMS-lines or in test-crosses to CMS-lines with the same cytoplasm type (Elkonin et al., 1998, 2005). Similar phenomenon was also described for the A3 cytoplasm and it was explained by paramutation of the Rf-genes caused by sterility-maintaining alleles (Tang et al., 2007). In our experiments, we observed that the level of male fertility of the F1 hybrids in the 9E and M35-1A cytoplasms correlates with the level of plant water availability during panicle development stage (Elkonin et al., 2005). On the basis of these data we suggested hypothesis on epigenetic regulation of fertility-restoring genes for the 9E cytoplasm (Elkonin et al., 2006).
In this paper, we report experimental results on heritable “activation” of fertility-restoring genes by “inductive” environmental conditions (plant water availability conditions) and expression of “activated” genes in self-pollinated progenies of fertile hybrids and in test-crosses with CMS-lines in “non-inductive” conditions that support hypothesis on epigenetic regulation of a dominant status of fertility-restoring alleles for the “9E” CMS-inducing cytoplasm of sorghum.
Materials and Methods
The CMS-lines used in this study were [9E] Tx398, [9E] Milo-10, and [9E] Volzhskoe-615 (V-615). These lines contain CMS-inducing cytoplasm “9E” (Webster and Singh, 1964). The lines [9E] Milo-10 and [9E] V-615 were obtained by backcrossing of the line [9E] Tx398 with the lines Milo-10 and Volzhskoe-615. The seeds of the line [9E] Tx398 were generously provided by the late Dr K. F. Schertz (Texas Agricultural Experimental Station, USA). Grain sorghum cv. Perspectivnoe-1 (Pers-1) and fertile (euplasmic) line in the “9E” cytoplasm, KVV-263, were used as lines-fertility restorers. KVV-263 was obtained by self-pollination of the fertile F1 hybrids, [9E] Tx398/KVV-112 grown in greenhouse. The F2 progeny and subsequent generations were grown in field conditions.
To test the hypothesis of heritable activation of fertility-restoring genes by plant water availability conditions one and the same F1, F2, and BC1 hybrids (i.e., the hybrids obtained by pollination of maternal CMS-lines with the pollen of F1 hybrids) were grown in “dry plot,” which has a roof from translucent polycarbonate to prevent irrigation of plants by rain precipitation (the roof was put on the plot at the booting stage of plant development), and in “irrigated plot,” which was artificially irrigated starting from the same developmental stage (the total amount of watering was 70 l/m2). In addition, sterile hybrids from F1 and F2 families grown in the “dry plot” and in the “irrigated plot” were transferred into greenhouse and were grown with regular irrigation. The progenies of fertile F1 hybrids and of fertile panicles developed on sterile plants, transferred to the greenhouse, were grown in the “dry plot” and in experimental field.
To evaluate level of male fertility the first panicle of each plant was bagged before anthesis. The level of male fertility was estimated at anthesis by cytological analysis of pollen and at maturity by determining the percent seed set. On the basis of the percent seed set on the panicles the plants were classified as sterile (s) (0% seed setting or a few, no more than 1% seeds), partially sterile (ps; <50%; usually 5–20% in the basal part of the panicle), or fertile (f; >50%; usually 90–100%). For cytological analysis of pollen fertility the spikelets from different parts of the panicle were fixed in acetic alcohol (1:3), and stored in 75% alcohol. For pollen analysis the anthers from 10 to 15 spikelets were placed on a glass slide and macerated. Pollen grains (PGs) were stained with 1% iodine–potassium iodide stain for the estimation of starch accumulation. A minimum of 400 PGs per plant were analyzed.
The χ2-test and exact binomial test (McDonald, 2009) were used to determine the fit of observed ratios of sterile and fertile plants to the expected segregation ratio. The number of fertile and ps-plants in different progenies was also compared by the Fisher method using F-criteria (Zaitsev, 1984). All experiments were replicated.
Results
Experiments on Parallel Growth of Hybrid Populations in “dry plot” and in “irrigated plot”
Results of experiments on parallel growth of hybrid populations in the “dry plot” and in the “irrigated plot” clearly demonstrate that water availability strongly affects the male fertility level of sorghum hybrids with the “9E” cytoplasm (Table 1). In the majority of the combinations analyzed sterile plants appeared in the F1 and BC1 generations in the “dry plot” ([9E] Rannee-7/KVV-263; [9E] Milo-10/Pers-1; [9E] P-614/Pers-1), whereas only fertile ([9E] Milo-10/Pers-1) or fertile and ps-plants were observed in the “irrigated plot” ([9E] Rannee-7/KVV-263; [9E] P-614/Pers-1). In other hybrid combinations the frequency of ps-plants significantly increased in the “irrigated plot” in contrast to complete or almost complete male sterility of the “dry plot.” In this connection, the most significant results were obtained in the hybrid combination [9E] Milo-10/KVV-263, in which F1 plants have almost completely sterile phenotype in drought conditions. However, after artificial watering ps-plants appeared among these hybrids. In the [9E] Tx398/KVV-263, the F1 hybrids were completely fertile in the “irrigated plot,” while both fertile and ps-plants were found in the “dry plot.” Remarkably, fertile line in the “9E” cytoplasm, KVV-263, which was one of the sources of fertility-restoring genes was fertile both in the “irrigated plot” and in the “dry plot.” Similar results were observed in different seasons (2007–2009; Table 1). These data demonstrate that fertility-restoring genes, in homozygous state, can function in drought conditions, however, in heterozygous state, these genes need in higher level of water availability for their expression.
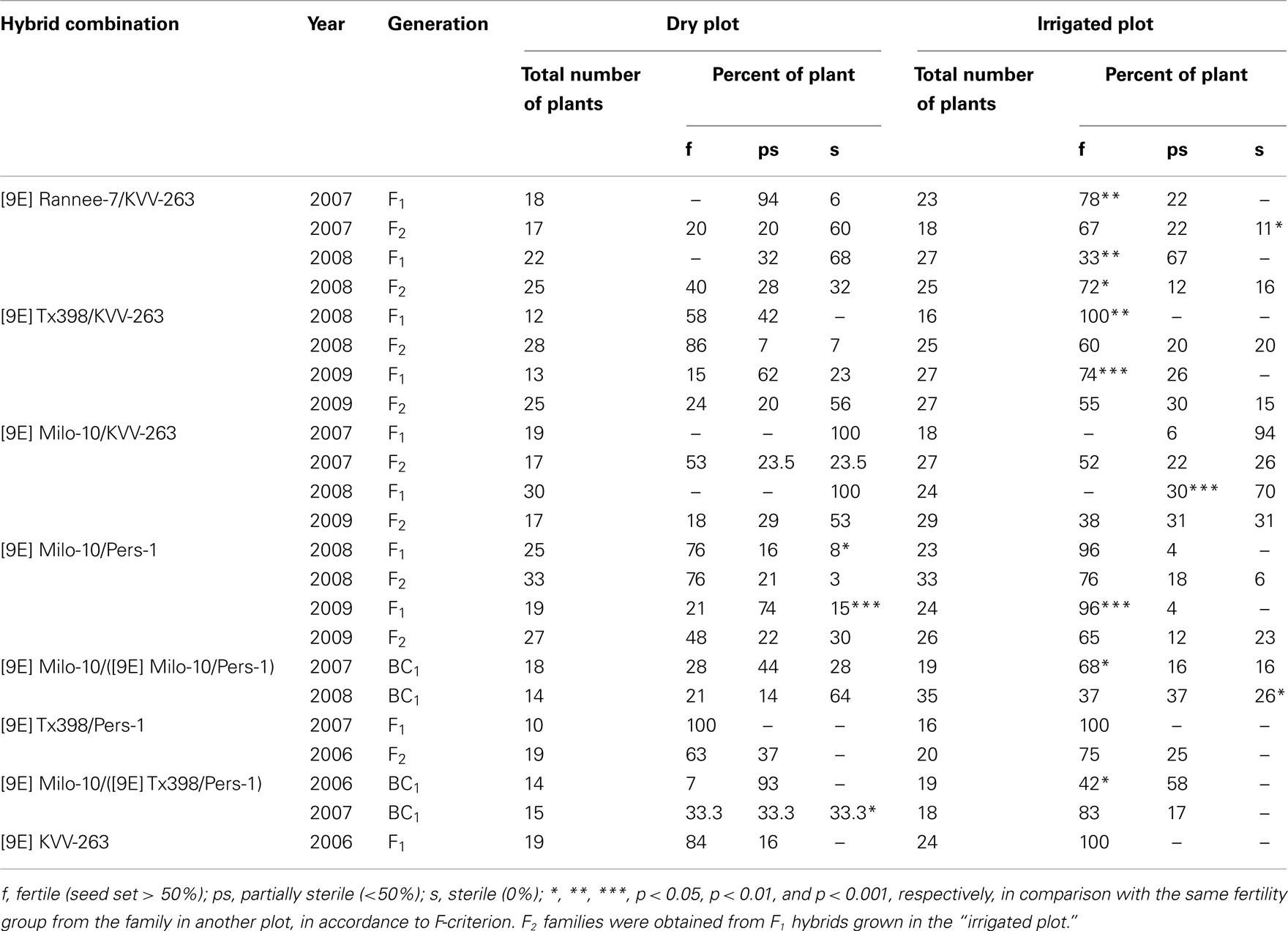
Table 1. Effect of plant water availability at panicle development stage on level of male fertility of sorghum hybrids in the “9E” type of CMS-inducing cytoplasm.
Cytological analysis of pollen of F1 hybrids from the “dry plot” and from the “irrigated plot” revealed a number of disturbances in starch accumulation, developmental delay at the stages of one- or two-nuclear gametophyte, and PGs with completely degenerated content (Figures 1B,C). The frequency of fertile PGs did not exceed 44% and in most cases varied from 13 to 22% in plants with completely restored seed fertility (Table 2). These data demonstrate that fertility-restoring genes did not normalize pollen development completely. No significant differences were observed in percent of fertile PGs between the “dry plot” and “irrigated plot” except ps-plants from the [9E] Tx398/KVV-263, but such differences have been found between plants with different seed set level within the same plot. Remarkably, sterile plants also contained some portion of fertile PGs, as well as plants of original stable CMS-lines ([9E] Milo-10: 7.17 ± 2.17%; [9E] Tx398: 15.26 ± 5.74%; Figure 1A), that is characteristic feature of the “9E” cytoplasm (Pring et al., 1995). However, they did not set seed as a result of anther indehiscence. It is noteworthy that in the [9E] Tx398/KVV-263, in 2009 season, the plants with different seed set level grown in the “dry plot,” did not differed significantly by proportion of fertile PGs (Table 2). Such failure of seed set in the presence of fertile PGs was resulted from anther indehiscence. Therefore, sterilization of F1 hybrids in the “dry plot” is due not only to disturbances of pollen formation but also to anther indehiscence.
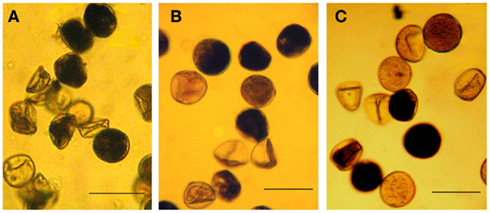
Figure 1. Pollen of male-sterile and fertility restored plants in the “9E” cytoplasm: (A) [9E] Milo-10, (B) F1 [9E] Milo-10/Perspectivnoe-1, (C) F1 [9E] Milo-10/KVV-263/. Bar: 50 mkm.
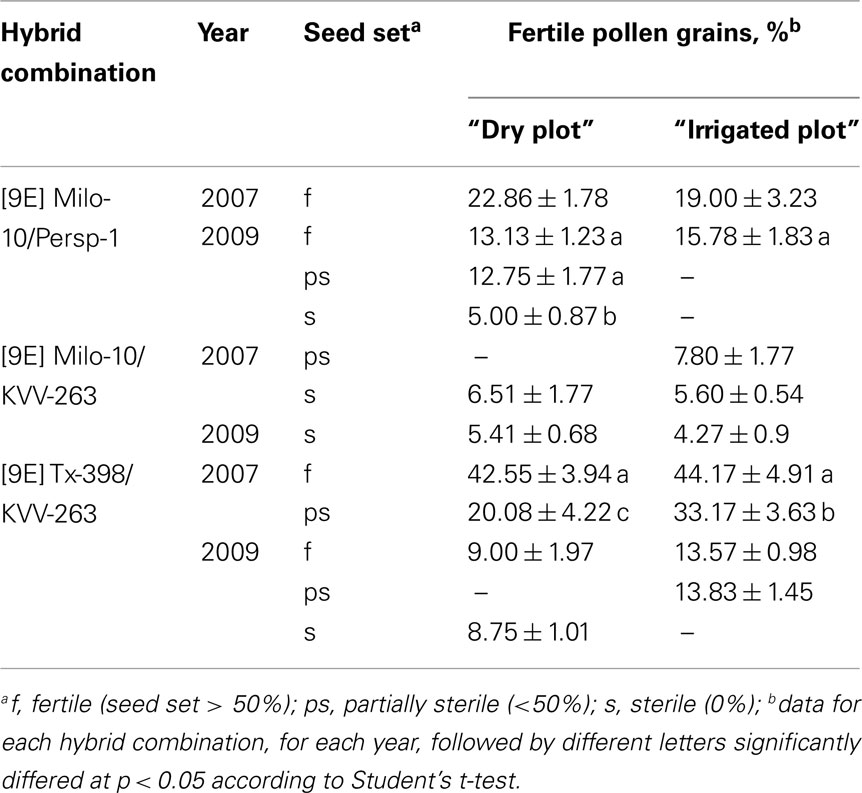
Table 2. Pollen fertility of plants from different F1 hybrid combinations in the “9E” CMS-inducing cytoplasm of sorghum.
In majority of F2 families obtained from the F1 hybrids developed in “irrigated plot,” the proportion of fertile and sterile plants in conditions of “dry” and “irrigated plot” did not differ significantly (Table 1), except [9E] Rannee-7/KVV-263, in which the percent of sterile plants in the “dry plot” was significantly higher than in the “irrigated plot.” Predominance of fertile plants in F2 families analyzed, both in the “irrigated plot” and in the field (Table 3) points to a dominant nature of fertility-restoring genes for the “9E” CMS-inducing cytoplasm of sorghum. Analysis of segregation pattern in large F2 populations grown in field conditions revealed that both lines-fertility restorers used in our experiments (Persp-1 and KVV-263) have two fertility-restoring genes, Rf 9E1 and Rf 9E2, however in different hybrid combinations function either one or two genes, depending on genotype of CMS-lines. Remarkably, in the same hybrid combination, in the wet seasons (2003, 2008), two nuclear genes participated in restoration of male fertility, while in the drought seasons (2001, 2009) – one fertility-restorer gene (Table 3).
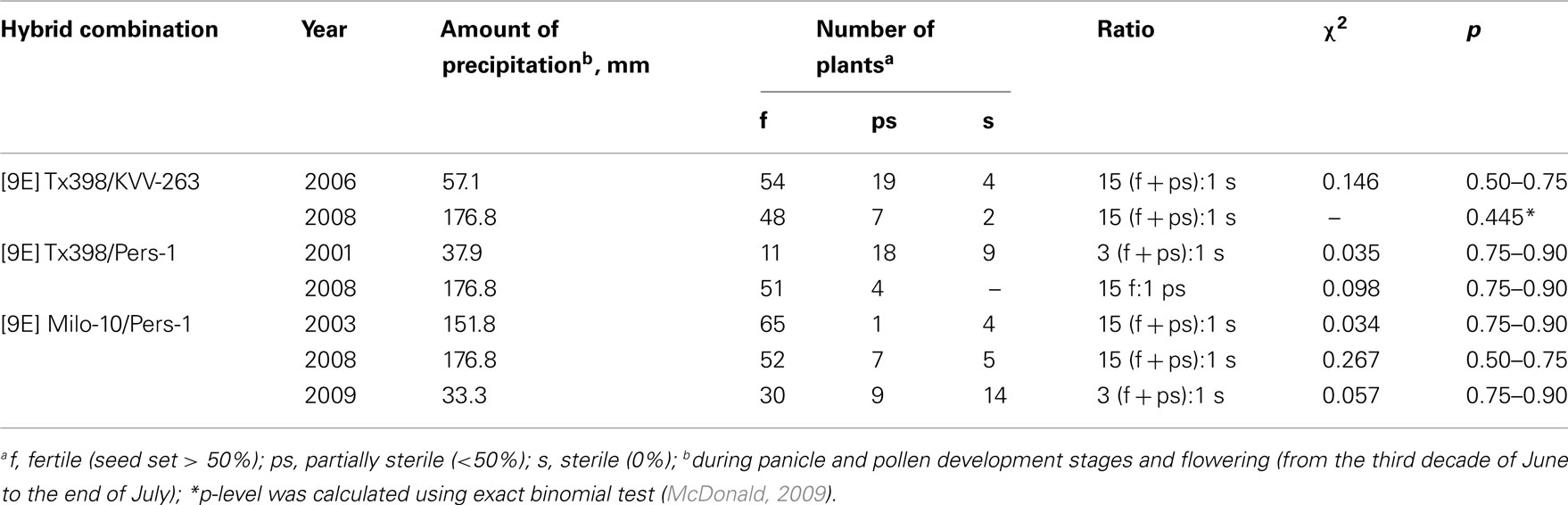
Table 3. Segregation in F2 families from crosses of different CMS-lines in the “9E” cytoplasm with fertility-restoring lines.
Reversions to Male Fertility in Male-sterile Hybrids in Greenhouse Conditions
To test the hypothesis on an environmental effect on “switching on” fertility-restoring genes for the “9E” CMS-inducing cytoplasm, male-sterile plants from different F1 and F2 hybrid populations that were grown both in the “dry plot” and in the “irrigated plot,” were transferred to the greenhouse and were grown under artificial watering (“inductive” conditions). In such conditions, the majority of the transferred male-sterile plants produced fertile panicles with high seed set (Table 4). For example, sterile F1 hybrid [9E] Tx398/KVV-263, which in the “dry plot” had 8.3% fertile PGs in greenhouse conditions had 54.7% fertile PGs and set seeds; the sterile F1 hybrid [9E] Milo-10/KVV-263 from the “dry plot,” which was characterized by 7.3% fertile PGs, in greenhouse conditions had 31.7% fertile PGs and also set seeds.
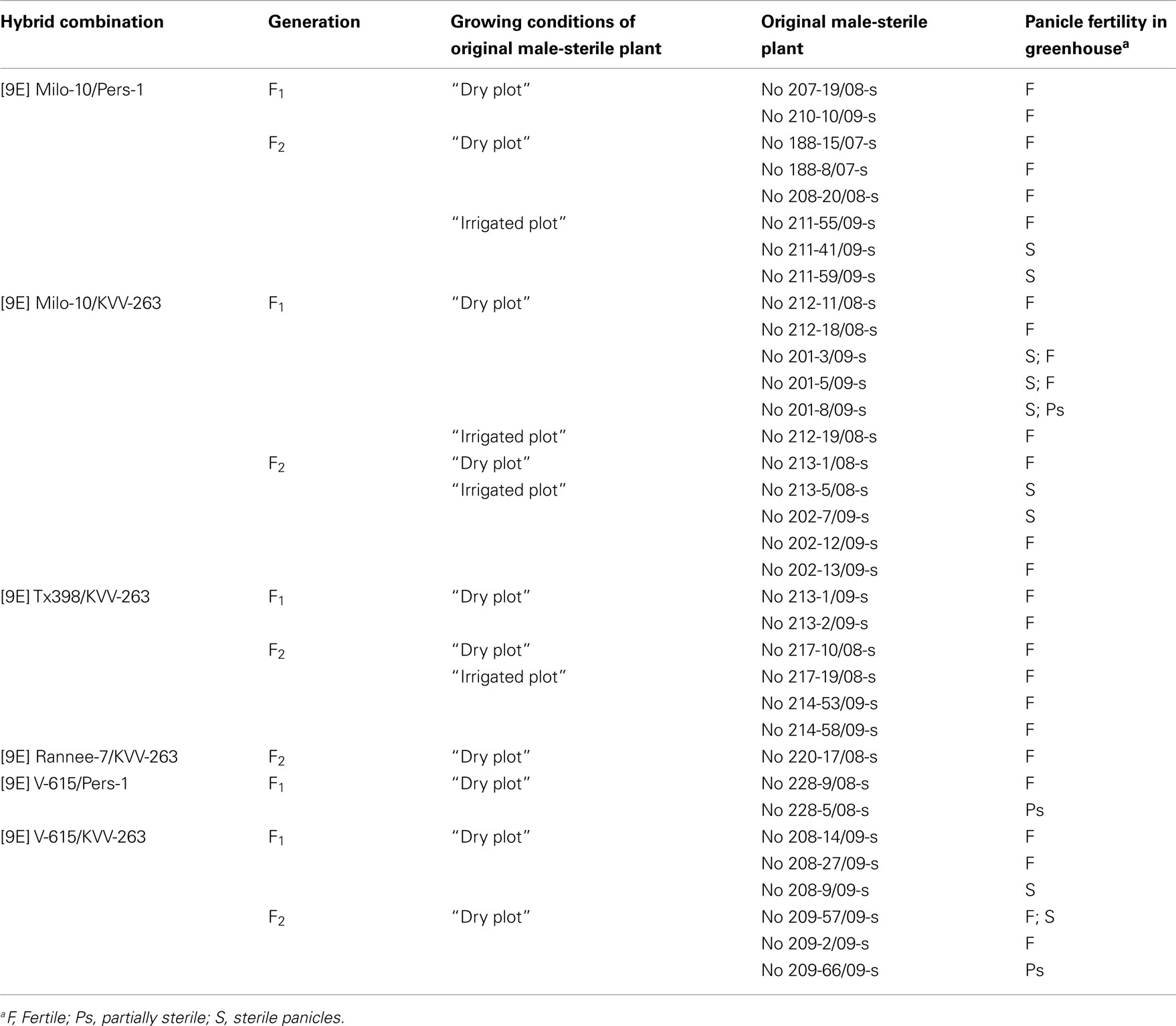
Table 4. Examples of reversions to male fertility in male-sterile plants, which were transferred to the greenhouse.
Remarkably, reversion to male fertility was observed not only in the F1 hybrids, which were heterozygous for fertility-restoring genes but also in the male-sterile plants segregating-out in the F2 families as recessives, presumably devoid of dominant fertility-restoring genes. Few sterile plants from the F2 families grown in the “irrigated plot” were stable and did not reverted to male fertility; in some F2 plants in greenhouse conditions both fertile and sterile panicles developed.
Heritability of Reversion to Male Fertility Induced by Environmental Conditions
To study the inheritance of “switched on” fertility-restoring genes, seeds from ps-plants from the “irrigated plot” (“inductive” conditions) from those combinations, which were completely sterile in the “dry plot,” were sown in the field and in the “dry plot” (in “non-inductive” conditions). In addition, the seeds from fertile tillers developed in the greenhouse (“inductive” conditions) were also sown in the field (in “non-inductive” conditions).
Few semi-sterile plants, appeared in the “irrigated plot” in the F1 [9E] Milo-10/KVV-263 yielded in their progeny (F2) fertile, ps, and sterile plants, “induced” fertility manifesting both in the “irrigated plot” and in the “dry plot” (Figure 2A). Similarly, male fertility induced in F1 plants from hybrid combination [9E] V-615/KVV-263, which were grown in the “irrigated plot” inherited for three cycles of self-pollination (Figure 2B). In F2 generation, segregation pattern in the “irrigated plot” and in the “dry plot” differed significantly in the progeny of plant no 1: in the “irrigated plot” predominated fertile plants while in the “dry plot” – sterile plants. No differences were recorded in the progeny of plant no 13. In the progeny (F3) of fertile plants from both “irrigated plot” and from the “dry plot” homozygous fertile families were found. In the F4 families grown in the “dry plot” conditions male fertility manifested in 100% plants. However, test-crosses of these plants to CMS-line [9E] Milo-10 expressed complete male sterility.
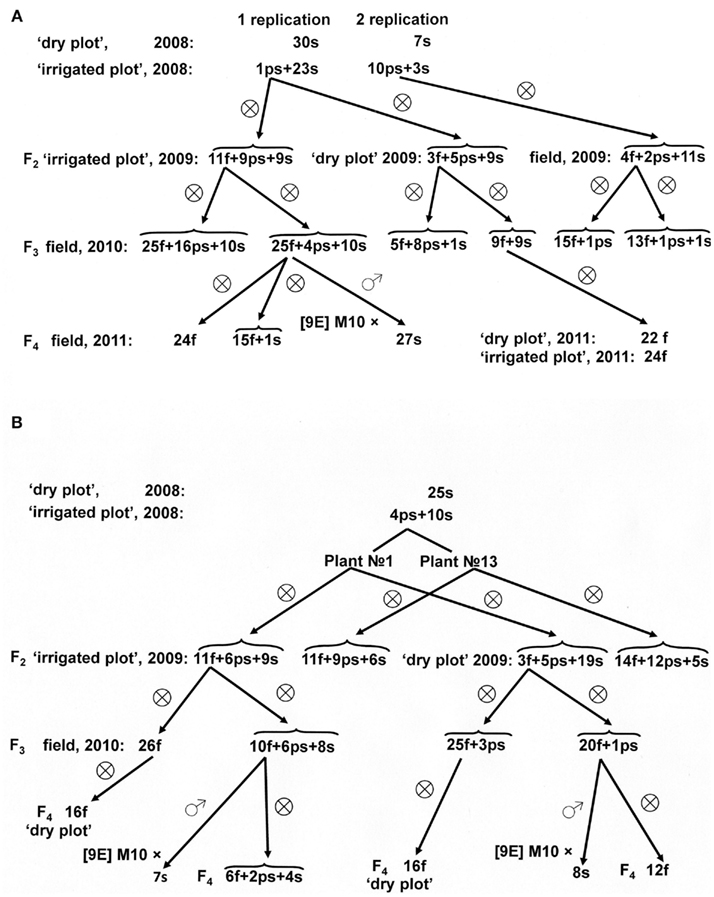
Figure 2. Inheritance of reversion to male fertility induced in “irrigated plot” in F1 hybrids (A) [9E] Milo-10/KVV-263 and (B) [9E] Volzhskoe-615/KVV-263. f, fertile (seed set > 50%); ps, partially sterile (<50%); s, sterile (0%). The crossed circles mean self-pollinated progenies.
Similarly, fertile panicles developed on male-sterile F1 hybrids [9E] Milo-10/KVV-263 transferred from the “dry plot” to the greenhouse also yielded fertile plants in their progeny grown in field conditions (Figure 3). “Induced” male fertility inherited for two cycles of self-pollination. Remarkably, sterile plants from self-pollinated progenies of fertile revertants, i.e., from the F2 and F3 generations, being transferred into greenhouse conditions also reverted to male fertility, and such “induced” fertility also stably inherited in the progeny of revertants. However, test-crosses with CMS-line [9E] Milo-10 were male-sterile.
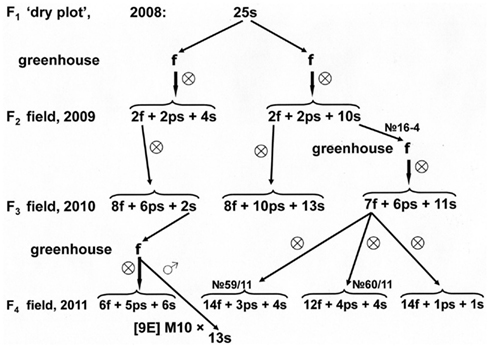
Figure 3. Inheritance of reversion to male fertility induced in greenhouse in male-sterile plants of F1 hybrids [9E] Milo-10/KVV-263 and in their progeny.
Thus, growing of the F1 hybrids in conditions of increased water availability allowed to obtain fertile lines in different hybrid combinations, [9E] V-615/KVV-263 and [9E] Milo-10/KVV-263, that would be impossible if the F1 hybrids were grown in drought conditions: in both combinations, in the “dry plot,” complete male sterility was observed in the F1 plants. However, these fertile lines were unable to restore male fertility of CMS-lines with their own cytoplasm type.
The seeds set on fertile tillers that developed on sterile plants from the F2 families in greenhouse conditions, also gave fertile progeny. For example, the F2 families of the [9E] Milo-10/Persp-1 hybrid combination grown in the “dry plot” segregated-out the sterile plants, their ratio to plants with restored male fertility corresponded to monogenic segregation 3 (f + ps):1 s (χ2 = 0.899; 0.50 > p > 0.25; Figure 4A). Similar segregation, 3:1, was observed in this family also in the “irrigated plot” (χ2 = 0.545; 0.50 > p > 0.25). In sterile plants from the “dry plot,” no 188-8 and no 188-15, after their transfer to the greenhouse developed fertile panicles. In the progeny of these panicles, which were grown in field conditions, fertile and sterile individuals were noticed. After two cycles of self-pollination of fertile individuals from the progeny of 188-15 plant, the line no 21/10 was obtained. This line manifested complete male fertility in conditions of both “irrigated plot” and “dry plot” (Figure 4A). However, backcross hybrids of this line to maternal CMS-line [9E] Milo-10 were characterized by complete male sterility.
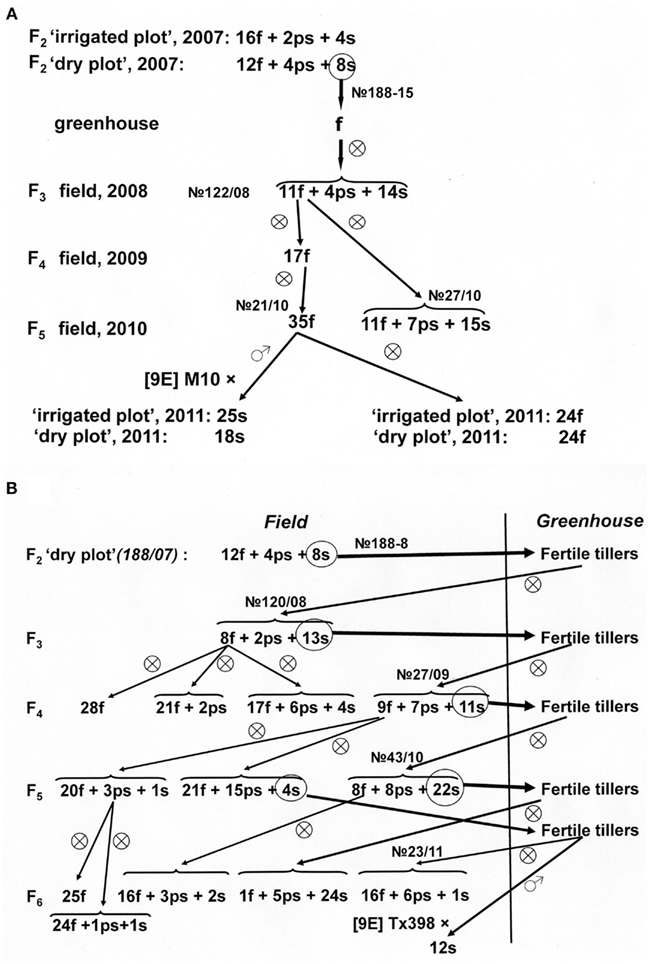
Figure 4. Inheritance of reversion to male fertility induced in greenhouse in male-sterile plants 188-15 (A) and 188–8 (B) from F2 family of [9E] Milo-10/Perspectivnoe-1.
Inheritance of reversion to male fertility in the progeny of fertile panicles developed on another male-sterile plant from this family, no 188-8 is of special interest (Figure 4B). In the self-pollinated progeny of fertile panicles (F3), segregation for plant male fertility was observed. Fertile plants from this progeny were either homozygous or produced families with few sterile or ps-plants. Sterile plants, being transferred to greenhouse, again developed fertile panicles, in their progeny (F4) grown in field conditions segregation was observed again. The progeny obtained from sterile plants from this family after their transfer to greenhouse (F5) was not homozygous but segregated again for male fertility; the self-pollinated progeny of fertile plants consisted almost completely from fertile individuals, while sterile plants again reverted in the greenhouse to male fertility. It should be noted that in the self-pollinated progeny of ps-plants, as well as in the progeny of fertile plants, either only fertile, or fertile and few ps or sterile individuals were found that point on identity of genotype of fertile and ps-plants. Remarkably, revertant panicles that produced fertile progeny after self-pollination, did not restore male fertility of test-cross hybrids with CMS-line [9E] Tx398.
Principally different result was noticed in test-crosses of fertile line no 14/10 that was obtained after self-pollination of fertile panicles developed in male-sterile plant from F2 family of [9E] Tx398/Persp-1 transferred from the “dry plot” to greenhouse (Figure 5). This line was able to restore male fertility of test-cross hybrids with the CMS-line [9E] Milo-10. Fertility of the line 14/10 and its test-cross hybrids expressed both in the “irrigated plot” and in the “dry plot.” Thus, in this case, after reversion in greenhouse conditions the line-fertility restorer was obtained on the basis of CMS-plant.
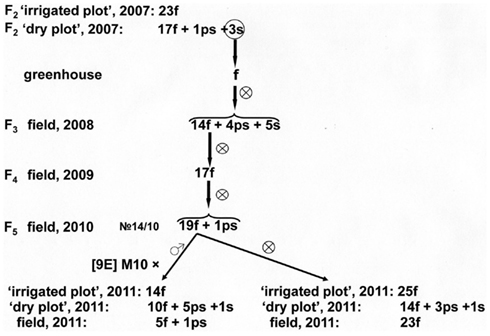
Figure 5. Inheritance of reversion to male fertility induced in greenhouse in male-sterile plant from F2 family of [9E] Tx398/Perspectivnoe-1.
Discussion
Experimental data presented above clearly demonstrate that environmental conditions, such as water availability during panicle and pollen developmental stages, affect functioning of the fertility-restoring genes for the “9E” CMS-inducing cytoplasm of sorghum. Its influence most strongly affects male fertility of F1 and test-cross hybrid populations, in which fertility-restoring genes were in heterozygote state. In some hybrid combinations ([9E] Milo-10/KVV-263, [9E] V-615/KVV-263), water-dependence of fertility-restoring genes is extremely strong, so that in drought conditions the F1 heterozygous plants were completely male-sterile. Growing in conditions of high level of water availability or transferring into greenhouse conditions allowed obtaining male-fertile F1 hybrids in these combinations, and, on their base, fertile lines. However, male fertility “induced” by this way, as a rule, did not transmit through the pollen in test-crosses with CMS-lines in the “9E” cytoplasm, with the only exception of revertant from the male-sterile plant from the F2 [9E] Tx398/Persp-1 family.
Such absence of fertility transmission through the pollen can not be explained by cytoplasmic nature of fertility reversions because previously we have found that the reciprocal hybrids, KVV-263/Tx398, obtained by pollination of emasculated panicle of fertile line KVV-263, which derived from the F1 hybrid [9E] Tx398/KVV-112 grown in greenhouse conditions, with the pollen of the line-sterility maintainer of the “9E” type CMS – Tx398 – were male-sterile in drought conditions as well as direct hybrids, [9E] Tx398/KVV-263 (Elkonin et al., 2005).
The following hypotheses can be suggested to explain the above-described phenomena.
Perhaps, fertility-restoring genes are sensitive to water availability of plants during panicle development stage, and in heterozygous state produce small amount of product in drought conditions. Therefore, F1 or BC1 plants in drought conditions are sterile. In wet conditions, fertility-restoring genes function more effectively, and heterozygous plants produce fertile pollen. After self-pollination and recombination, the homozygous plants for fertility-restoring genes would appear in the progeny of such heterozygotes, and these plants may be fertile in drought conditions if expression level in homozygous state is sufficient for restoration of male fertility. In other words, according to this hypothesis, water availability conditions change dominance in the nuclear loci governing fertility restoration: in drought conditions sterility-maintaining alleles are dominant, while in wet conditions fertility-restoring alleles are dominant. As follows from this hypothesis, in the F2 families grown in the “irrigated plot,” plants with restored male fertility (f- and ps-phenotypes) should be homozygous and heterozygous, while sterile plants (s-phenotype) should be only homozygous. In the “dry plot,” controversially, sterile plants should be heterozygous and homozygous, while plants with restored male fertility should be homozygous. Segregation observed in the F2 progeny of ps-plant no 1 [9E] V-615/KVV-263 (Figure 2B) grown in the “irrigated plot” [17 (f + ps):9 s; χ23:1 = 0.167; 0.50 > p > 0.25] and in the “dry” plot [8 (f + ps):19 s; χ21:3 = 0.309; 0.75 > p > 0.50] confirmed this hypothesis.
However, in the F2 progeny of ps-plant no 13 from the same hybrid combination no differences were observed between the “irrigated plot” and the “dry plot” (Figure 2B). In both cases, the frequency of plants with restored male fertility prevailed over sterile plants; the ratio fitted monogenic segregation 3:1 (χ2 = 0.051; 0.90 > p > 0.75 and χ2 = 1.301; 0.50 > p > 0.25; respectively). In addition, this hypothesis can not explain reversion to male fertility of male-sterile individuals segregating in the F2 families as recessives.
Therefore, more likely is another hypothesis that assumes epigenetic activation of fertility-restoring alleles by high water-availability conditions. The sensitivity of epigenetic events (such as DNA methylation, histone modifications) to plant water availability conditions, is well-documented (Lukens and Zhan, 2007; Chinnusamy and Zhu, 2009; Verhoeven et al., 2010). Perhaps, increased water availability conditions cause modification of histone proteins – such a modification (dimethylation of H3 at lysine 4) associated with active gene expression was found to be high during the wet season in perennial desert plant Zygophyllum dumosum Boiss. (Granot et al., 2009), or prevent methylation of fertility-restoring gene sequences that may take place in drought conditions (Wang et al., 2011) and this methylation state is transmitted to the next sexual generations. Such transgenerational inheritance of methylation state is well-known phenomenon (Kakutani, 2002; Takeda and Paszkowski, 2006; Daxinger and Whitelaw, 2010; D:Hauser:2011]). According to this hypothesis fertility-restoring genes are “activated” in wet conditions (Rf/rf → Rf*/rf; rf/rf → rf*/rf, where the asterisk means activated allele) and then are transmitted to the progeny in their active state. However, taking into account complete male sterility of test-cross plants one should suppose that activated state of fertility-restoring genes inherited only under self-pollination, whereas in “new” genetic background (F1 hybrid genome) the epigenetic marks caused activation of fertility-restoring genes are erased and the test-cross plants become male-sterile. Such inheritance is typical for epigenetically determined traits, which are caused, in particular, by changes of DNA methylation pattern. Data on remodeling of the parental methylation patterns in the F1 hybrids are well-documented (Xiong et al., 1999; Shaked et al., 2001; Zhang et al., 2007; Zhao et al., 2007).
It should be stressed that after such activation the fertility-restoring genes become expressive not only in wet conditions but also under drought stress (Figures 2A, 4A, and 5).
Segregation in the progeny of fertility revertants may be due to their heterozygosity. To explain these segregation patterns, one should take into account the data from Table 3. As follows from these data, both fertility-restorers, which were used in our experiments, KVV-263 and Persp-1, possess two pairs of fertility-restoring genes. In hybrid combinations with Persp-1, in the wet seasons (2003, 2008) both genes functioned and segregation corresponded to 15:1 ratio, whereas in the dry seasons (2001, 2009) only one fertility-restorer gene expressed, and segregation ratio corresponded to 3:1 ratio. Therefore, sterile plants which were taken from F2 families from the “dry plot” may have genotype rf1rf1rf2rf2 or rf1rf1Rf2−. After activation in the greenhouse conditions, the heterozygotes for two pairs of fertility-restoring alleles may appear (rf1*rf1Rf2rf2 and/or rf1*rf1rf2 *rf2). Therefore, segregation in the progeny of such fertility revertants should correspond to digenic ratio. Indeed, segregation in the progeny of fertility revertant from sterile plants 188-15 (F2 [9E] M-10/Persp-1; Figure 4A) and 16-4 (F2 [9E] M-10/KVV-263; Figure 3) presumably corresponds to 9 (f + ps):7 s ratio (χ2 = 0.241; 0.75 > p > 0.50; and χ2 = 0.042; 0.90 > p > 0.75; respectively). In the self-pollinated progenies of fertile plants from these families male fertility inherited as a dominant trait, and segregation fitted to either 3:1 (families 59/11, 60/11, Figure 3: χ2 = 0.659; 0.50 > p > 0.25, for total data) or 9:7 (family 27/10, Figure 4A: χ2 = 0.039; 0.90 > p > 0.75) ratios, perhaps, depending on genotype of self-pollinated plants (mono- or di-heterozygotes).
Ratio of fertile and male-sterile individuals in the progeny of fertility revertants from another sterile plant from this hybrid combination, 188-8 (Figure 4B), also resembled segregation for two pairs of genes, although in this case activated fertility-restoring alleles behaved as recessives and segregation fitted to 7 (f + ps):9 s ratio (χ2 = 0.001; 0.99 > p > 0.95). This type of segregation means that sterility should be caused by two complementary dominant sterility-maintaining genes. In this case, self-pollinated progeny of fertile plants should be also fertile. Indeed, self-pollinated progeny of fertile plants were either fertile or contained only few sterile or ps individuals. These sterile individuals could be reverted again to fertile plants in greenhouse conditions. But contrary to “primary” revertants (families no 120/08, 27/09, 43/10, Figure 4B) the revertants from sterile individuals from the progeny of self-pollinated fertile plants produced predominantly fertile off-spring (family no 23/11, Figure 4B) as it was observed in self-pollinated progenies of fertile plants.
Thus, the functional state of fertility-restoring genes in the “9E” CMS-inducing cytoplasm of sorghum is conditioned by epigenetic changes appearing by the influence of sufficient level of water availability. This state is transmitted to self-pollination progenies but in the “new” genetic background – in F1 or BC1 hybrid genome – it might be established de novo and only under sufficient level of water availability.
At present time we can only speculate about the molecular mechanisms involved in suppression of fertility-restoring genes for the “9E” cytoplasm in drought conditions. Recent studies on retrograde signaling from chloroplast, which are the primary targets of drought stress (Chan et al., 2010) suggest that any molecules accumulating within these organelles under water deficit, such as reactive oxygen species, ROS (Suzuki et al., 2011), or 3′-phosphoadenosine 5′-phosphate, PAP (Estavillo et al., 2011), may down-regulate nuclear gene(s) involved in pollen maturation and/or anther dehiscence. In addition to chloroplasts, mitochondria play also an important role in drought response by supplying chloroplasts with ATP, controlling the ROS accumulation and regulating proline concentration (Atkin and Macherel, 2009). Perhaps, withdrawal of water deficit in plants grown in the “irrigated plot” or in greenhouse conditions prevents accumulation of ROS molecules within chloroplasts and by mechanism of retrograde signaling activate expression of nuclear genes restoring male fertility. However, such activation would not be inherited in subsequent sexual generation, unless inherent changes would occur in nuclear loci controlling pollen development, taking into account that heritable restoration of male fertility did not result from cytoplasmic mutation(s) (Elkonin et al., 2005).
Therefore, to test the outlined hypothesis on activation of fertility-restoring genes by high water availability conditions it is crucially important to study the nature of molecular changes that may occur in the nuclear loci controlling fertility restoration in the “9E” cytoplasm. Future investigations, therefore, should be conducted with identification of nucleotide sequences of genes located in these loci, and investigation of epigenetic changes occurring in these loci in different plant water availability conditions. Assuming that almost all of the fertility-restoring nuclear genes studied up to date encode proteins with PPR motif (Fujii and Toriyama, 2008), and that the PPR proteins involved in post-transcriptional regulation of gene expression in chloroplasts and mitochondria, were also found to be involved in abiotic stress response (Zsigmond et al., 2008; Liu et al. 2010; Yuan and Liu 2012), one should suppose that “environmentally sensitive” fertility-restoring genes in our revertants also encode a protein(s) belonging to PPR family. Therefore, another approach that would be helpful in investigation of hypothesis on activation of fertility-restoring genes by high water availability is to study chloroplast and mitochondria proteome in water-stressed and irrigated plants, with special attention to PPR proteins.
Summarizing, the F1 hybrids in the “9E” cytoplasm represent a model systems for studying regulation of plant response to drought stress. Further investigation of this system as well as the molecular bases of epigenetic events in the fertility-restoring loci occurring under high water-availability conditions are needed to shed light on fundamental genetic problems, such as anterograde and retrograde signaling, gene dominance and interaction of genes with their nuclear background, and on possible application of these findings in sorghum genetic improvement.
Conflict of Interest Statement
The authors declare that the research was conducted in the absence of any commercial or financial relationships that could be construed as a potential conflict of interest.
Acknowledgments
We are grateful to reviewers for valuable comments promoting to improve the manuscript of this paper.
References
Atkin, O. K., and Macherel, D. (2009). The crucial role of plant mitochondria in orchestrating drought tolerance. Ann. Bot. 103, 581–597.
Chan, K. X., Crisp, P. A., Estavillo, G. M., and Pogson, B. I. (2010). Chloroplast-to-nucleus communication: current knowledge, experimental strategies and relationship to drought stress signaling. Plant Signal. Behav. 5, 1575–1582.
Chase, C. D., and Gabay-Laughnan, S. (2004). “Cytoplasmic male sterility and fertility restoration by nuclear genes”, in Molecular Biology and Biotechnology of Plant Organelle, eds H. Daniell and C. D. Chase (Dordrecht: Springer), 593–621.
Chinnusamy, V., and Zhu, J.-K. (2009). Epigenetic regulation of stress responses in plants. Curr. Opin. Plant Biol. 12, 133–139.
Daxinger, L., and Whitelaw, E. (2010). Transgenerational epigenetic inheritance: more questions than answers. Genome Res. 20, 1623–1628.
Elkonin, L. A., Kozhemyakin, V. V., and Ishin, A. G. (1998). Nuclear-cytoplasmic interactions in restoration of male fertility in the ‘9E’ and A4 CMS-inducing cytoplasms of sorghum. Theor. Appl. Genet. 97, 626–632.
Elkonin, L. A., Kozhemyakin, V. V., and Ishin, A. G. (2005). Influence of water availability on fertility restoration of CMS lines with the ‘M35’, A4 and ‘9E’ CMS-inducing cytoplasms of sorghum. Plant Breed. 134, 565–571.
Elkonin, L. A., Kozhemyakin, V. V., and Kibalnik, O. P. (2006). Genetic and epigenetic regulation of male fertility restoration in the ‘9E’, A4 and ‘M35’ CMS-inducing cytoplasms of sorghum. Acta Agron. Hung. 54, 281–289.
Estavillo, G. M., Crisp, P. A., Pornsiriwong, W., Wirtz, M., Collinge, D., Carrie, C., Giraud, E., Whelan, J., David, P., Javot, H., Brearley, C., Hell, R., Marin, E., and Pogson, B. J. (2011). Evidence for a SAL1-PAP chloroplast retrograde pathway that functions in drought and high light signaling in Arabidopsis. Plant Cell 23, 3992–4012.
Fernandez, A. P., and Strand, A. (2008). Retrograde signaling and plant stress: plastid signals initiate cellular stress responses. Curr. Opin. Plant Biol. 11, 509–513.
Fisher, A. J., and Franklin, K. A. (2011). Chromatin remodelling in plant light signaling. Physiol. Plant. 142, 305–313.
Fujii, S., and Toriyama, K. (2008). Genome barriers between nuclei and mitochondria exemplified by cytoplasmic male sterility. Plant Cell Physiol. 49, 1484–1494.
Granot, G., Sikron-Persi, N., Gaspan, O., Florentin, A., Talwara, S., Paul, L. K., Morgenstern, Y., Granot, Y., and Grafi, G. (2009). Histone modifications associated with drought tolerance in the desert plant Zygophyllum dumosum Boiss. Planta 231, 27–34.
Grant-Downton, R. T., and Dickinson, H. G. (2005). Epigenetics and its implications for plant biology. 1. The epigenetic network in plants. Ann. Bot. 96, 1143–1164.
Grant-Downton, R. T., and Dickinson, H. G. (2006). Epigenetics and its implications for plant biology 2. The “epigenetic epiphany”: epigenetics, evolution and beyond. Ann. Bot. 97, 11–27.
Hanson, M. R., and Bentolila, S. (2004). Interactions of mitochondrial and nuclear genes that affect male gametophyte development. Plant Cell 16, 154–169.
Hauser, M. T., Aufsatz, W., Jonak, C., and Luschnig, C. (2011). Transgenerational epigenetic inheritance in plants. Biochim. Biophys. Acta 1809, 459–468.
Kakutani, T. (2002). Epi-alleles in plants: inheritance of epigenetic information over generations. Plant Cell Physiol. 43, 1106–1111.
King, G. J., Amoah, S., and Kurup, S. (2010). Exploring and exploiting epigenetic variation in crops. Genome 53, 856–868.
Liu, Y., He, J., Chen, Z., Ren, X., Hong, X., and Gong, Z. (2010). ABA overly-sensitive 5 (ABO5), encoding a pentatricopeptide repeat protein required for cis-splicing of mitochondrial nad2 intron 3, is involved in the abscisic acid response in Arabidopsis. Plant J. 63, 749–765.
Lukens, L. N., and Zhan, S. H. (2007). The plant genome’s methylation status and response to stress, implications for plant improvement. Curr. Opin. Plant Biol. 10, 317–322.
McDonald, J. H. (2009). Handbook of Biological Statistics, 2nd Edn. Baltimore, MD: Sparky House Publishing.
Pfluger, J., and Wagner, D. (2007). Histone modifications and dynamic regulation of genome accessibility in plants. Curr. Opin. Plant Biol. 6, 645–652.
Phillips, J. R., Dalmay, T., and Bartels, D. (2007). The role of small RNAs in abiotic stress. FEBS Lett. 581, 3592–3597.
Pring, D. R., Tang, H. V., and Schertz, K. F. (1995). “Cytoplasmic male sterility and organelle DNAs of sorghum”, in Molecular Biology of Plant Mitochondria, eds C. S. Levings, III, and I. K. Vasil (Boston, MA: Kluwer Academic Publishers), 461–495.
Shaked, H., Kashkush, K., Ozkan, H., Feldman, M., and Levy, A. A. (2001). Sequence elimination and cytosine methylation are rapid and reproducible responses of the genome to wide hybridization and allopolyploidy in wheat. Plant Cell 13, 1749–1759.
Suzuki, N., Koussevitzky, S., Mittler, R., and Miller, G. (2011). ROS and redox signaling in the response of plants to abiotic stress. Plant Cell Environ. 35, 259–270.
Takeda, S., and Paszkowski, J. (2006). DNA methylation and epigenetic inheritance during plant gametogenesis. Chromosoma 115, 27–35.
Tang, H. V., Pedersen, J. F., Chase, C. D., and Pring, D. R. (2007). Fertility restoration of the sorghum A3 male-sterile cytoplasm through a sporophytic mechanism derived from sudangrass. Crop Sci. 47, 943–950.
Verhoeven, K. J., Jansen, J. J., van Dijk, P. J., and Biere, A. (2010). Stress-induced DNA methylation changes and their heritability in asexual dandelions. New Phytol. 185, 1108–1118.
Wang, W.-S., Pan, Y. J., Zhao, X.-Q., Dwivedi, D., Zhu, L.-H., Ali, J., Fu, B.-Y., and Li, Z.-K. (2011). Drought-induced site-specific DNA methylation and its association with drought tolerance in rice (Oryza sativa L.). J. Exp. Bot. 62, 1951–1960.
Webster, O. J., and Singh, S. P. (1964). Breeding behavior and histological structure of a non-dehiscent anther character in Sorghum vulgare Pers. Crop Sci. 4, 656–658.
Xiong, L. Z., Xu, C. G., Shagi-Maroof, M. A., and Zhang, Q. (1999). Patterns of cytosine methyaltion in an elite rice hybrid and its parental lines, detected by a methylation-sensitive amplification polymorphism technique. Mol. Genet. Genomics 261, 439–446.
Yang, J., Zhang, M., and Yu, J. (2008). Mitochondrial retrograde regulation tuning fork in nuclear genes expressions of higher plants. J. Genet. Genomics 35, 65–71.
Yuan, H., and Liu, D. (2012). Functional disruption of the PPR protein SLG1 affects mitochondrial RNA editing, plant development, and responses to abiotic stresses in Arabidopsis. Plant J. 70, 432–444.
Zhang, M. S., Yan, H. Y., Zhao, N., Lin, X. Y., Pang, J. S., Xu, K. Z., Liu, L. X., and Liu, B. (2007). Endosperm-specific hypomethylation, and meiotic inheritance and variation of DNA methylation level and pattern in sorghum (Sorghum bicolor L.) inter-strain hybrids. Theor. Appl. Genet. 115, 195–207.
Keywords: epigenetic inheritance, cytoplasmic male sterility, drought, Sorghum bicolor (L.) Moench
Citation: Elkonin LA and Tsvetova MI (2012) Heritable effect of plant water availability conditions on restoration of male fertility in the “9E” CMS-inducing cytoplasm of sorghum. Front. Plant Sci. 3:91. doi: 10.3389/fpls.2012.00091
Received: 30 November 2011; Accepted: 21 April 2012;
Published online: 11 May 2012.
Edited by:
Richard A. Jorgensen, National Laboratory of Genomics for Biodiversity, MexicoReviewed by:
Richard A. Jorgensen, National Laboratory of Genomics for Biodiversity, MexicoIgor Kovalchuk, University of Lethbridge, Canada
Copyright: © 2012 Elkonin and Tsvetova. This is an open-access article distributed under the terms of the Creative Commons Attribution Non Commercial License, which permits non-commercial use, distribution, and reproduction in other forums, provided the original authors and source are credited.
*Correspondence: L. A. Elkonin, Department of Biotechnology, Agricultural Research Institute for South-East Region, Tulaikova street, 7, Saratov, 410010, Russia e-mail:bGVsa29uaW5AZ21haWwuY29t