- 1Graduate School, Shandong Sport University, Jinan, China
- 2College of Sports and health, Shandong Sport University, Jinan, China
Purpose: Chronic ankle instability (CAI) causes maladaptive neuroplastic changes in the central nervous system, which may lead to high injury potential under dual-task conditions. This study aims to explore the effects of dual-task paradigm on the injury potential during landing among individuals with CAI.
Methods: Twenty participants with CAI (4 female and 16 male, 12 were affected with their right limbs and 8 were affected with their left limbs, 20.4 ± 1.7 years, 176.9 ± 5.0 cm, and 72.0 ± 11.1 kg) and eighteen without CAI (6 female and 12 male, 20.2 ± 1.5 years, 173.5 ± 7.0 cm, and 70.3 ± 10.8 kg) were recruited. They drop-landed on a trap-door device, with their affected or matched limbs on a flippable platform, under single- (drop-landing only) and dual-task (drop-landing while subtracting of serial threes) conditions. A twelve-camera motion capture system was used to capture the kinematic data. Two-way ANOVA with mixed design (CAI vs non-CAI groups by single-vs dual-task conditions) was used to analyze the data.
Results: Significant group-by-condition interactions were detected in the ankle inversion angle (P = 0.040, η2p = 0.012) and ankle inversion angular velocity (P = 0.038, η2p = 0.114). Both indicators decreased among individuals without CAI from single-to dual-task conditions, while remained unchanged among those with CAI; and they were higher among individuals with CAI under both single- and dual-task conditions, compared to those without CAI.
Conclusion: Individuals with CAI have a reduced ability to limit ankle inversion compared to those without CAI. Under dual-task conditions, individuals without CAI limited their ankle inversion, while those with CAI did not. Drop-landing, especially under dual-task conditions, poses a high risk of excessive ankle inversion for individuals with CAI.
1 Introduction
Ankle sprains are one of the most common sports injuries, accounting for 10%–20% of all sports-related injuries (Fong et al., 2007) and up to 70%–80% among college students with sports experience (Doherty et al., 2014). In the Netherlands, approximately 440,000 ankle sprains occur annually (Kemler et al., 2015), while in the United Kingdom, they constitute 3%–5% of all emergency department visits, resulting in 1–1.5 million cases per year (Gribble et al., 2016b). Acute ankle sprains can lead to repeated injuries and the development of chronic ankle instability (CAI), with a prevalence of about 20%–30% (Konradsen et al., 2002; Herzog et al., 2019). CAI is characterized by pain, instability, recurrent injuries, and persistent dysfunction (Gribble, 2019), significantly impacting an individual’s physical activity levels (Hubbard-Turner and Turner, 2015).
Landing from a height is a common scenario leading to ankle sprains (Doherty et al., 2014; Ardakani et al., 2019). During landing, the foot and ankle complex absorb the impact force from the ground, which can be 2–5 times the body weight (Xu et al., 2024b; Xu et al., 2023). This force is primarily transmitted through the medial aspect of the ankle, may cause sudden and substantial inversion of the ankle joint, which can lead to ankle sprains (Koshino et al., 2017). Larger ankle inversion angles and angular velocities during landing are associated with an increased potential for ankle sprains (Xu et al., 2024a; Zhang et al., 2024). Individuals with CAI have greater ankle inversion angles and angular velocities during landing compared to those without CAI (Simpson et al., 2022; Terrier et al., 2014). Excessive ankle inversion increases the distance between the talus and fibula, stretching the ligaments connecting these bones (Fong et al., 2012). When the ligament is stretched beyond its maximum bearing capacity, it can lead to ligament tears (Medina McKeon and Hoch, 2019).
CAI, a neurophysiological disorder characterized by maladaptive neuroplastic changes in the central nervous system (CNS), may increase potential injury under dual-task conditions. Individuals with CAI often exhibit reduced activation of the dorsal anterior cingulate cortex (Shen et al., 2022), a critical region for integrating cognitive resources (Bush et al., 2000). This reduction in cognitive resources can impair motor performance and elevate injury risk in environments requiring additional cognitive demands, such as dual-task conditions (Rosen et al., 2021; Choi et al., 2023). For example, a volleyball player with CAI aiming to execute a powerful smash must jump as high as possible and extend their upper limb. This constitutes a motor task. Simultaneously, they must consider the optimal landing spot for the ball based on the opposing defender’s position and condition, representing a cognitive task. Together, these actions embody a dual-task condition. Individuals with CAI, who have a limited total capacity of potential cognitive resources, experience reduced allocation of these resources to both cognitive and motor tasks. Consequently, their performance on either task may suffer, potentially leading to tactical errors or unintentional injuries.
There is ongoing controversy regarding the performance of individuals with and without CAI during dual-task conditions, as well as the potential impact of dual-task paradigms on injury risk in individuals with CAI. Some researchers report an increase in injury potential among individuals with FAI when transitioning from single-task to dual-task conditions, attributing this to impaired feedforward and feedback mechanisms of motor control within the CNS (Tavakoli et al., 2016). Conversely, others have observed enhanced postural stability among CAI individuals under dual-task conditions, which they attribute to increased conscious control over body movement due to fear of anticipated pain or reinjury (Shiravi et al., 2017). Moreover, it remains uncertain whether CAI individuals are more susceptible to the effects of dual-task paradigms compared to those without CAI. Some studies have indicated that athletes with FAI exhibit poorer postural stability than healthy controls under dual-task conditions, potentially increasing their injury risk (Rahnama et al., 2010). However, other studies have reported no differences in postural stability between CAI and non-CAI individuals under dual-task conditions (Choi et al., 2023).
Investigating the effects of dual-task paradigm on the injury potential among individuals with CAI may enhancing our understanding on whether the maladaptation of CNS affect the ankle sprain recurrence, and even the tactical arrangements of when and how long the players with CAI would be in the game. Therefore, we hypothesized that: 1) compared to individuals without CAI, those with CAI have higher injury potential, reflected by greater ankle inversion angle and angular velocity. 2) compared to single-task conditions, the injury potential increased among individuals with and without CAI under dual-task conditions.
2 Methods
2.1 Participants
Sample size calculations were conducted using G*Power 3.1 software (University of Düsseldorf, Düsseldorf, Germany). Prior to the formal study, a pilot study was performed with six participants with CAI and another six participants without CAI. The ankle inversion angle and ankle inversion angular velocity were used as outcome measures to estimate the sample size. The effect sizes (η2p) for the group comparison (CAI vs. non-CAI) by condition (single-task vs. dual-task) were 0.064 and 0.087, respectively. Based on these calculations, a total of at least 34 participants (17 in each group) were required to achieve a statistical significance level of 0.05 and a statistical power of 0.80.
Participants were recruited in a local university from April to June 2023 through distributing posters and leaflets. Following the guidelines of the International Ankle Consortium (Gribble et al., 2016a), the inclusion criteria for participants with CAI were: 1) at least one severe ankle sprain a year prior to the recruitment, causing pain, swelling, and other inflammatory symptoms, inhibiting normal participation in daily activities for more than 1 day; 2) aged 18–24 years (Ardakani et al., 2019); 3) at least two episodes of ankle “giving way” in the past 6 months; 4) persistent sense of ankle instability and impaired ability of daily activities and 5) with a score <24 of the Cumberland Ankle Instability Tool (CAIT) (Hiller et al., 2006). Inclusion criteria for participants without CAI were: 1) no previous ankle sprain/injury and no episodes of ankle “giving way” or feeling of ankle instability and 2) CAIT score >28. Exclusion criteria for all participants were: 1) self-reported history of lower limb fractures or surgery; 2) experienced acute injuries such as sprains in the lower limbs 3 months prior to the recruitment; 3) bilateral chronic ankle instability. All participants in this study were regular college students, both with and without CAI, who attended physical education classes three times a week for 45 min per session. They were not sedentary and were in good physical condition. After the assessment, 38 participants met the inclusion criteria, of whom 20 with CAI (4 female and 16 male, 12 were affected with their right limbs and 8 were affected with their left limbs, 20.4 ± 1.7 years, 176.9 ± 5.0 cm, and 72.0 ± 11.1 kg) and 18 without CAI (6 female and 12 male, 20.2 ± 1.5 years, 173.5 ± 7.0 cm, and 70.3 ± 10.8 kg) were recruited. This study was approved by the Institutional Review Board of Shandong Sport University (Approval Number: 2023014) and was in accordance with the Declaration of Helsinki, and all participants signed informed consent forms.
2.2 Protocols
Participants wore uniformed tight shorts and T-shirts, and 36 markers were adhered to their lower limbs, following the protocol of the Oxford Foot Ankle Model (McCahill et al., 2008) (Figure 1). The test limb in the CAI group were the affected limb, and the test limb in the non-CAI group were matched based on the ratio of the right and left affected limb in the CAI group, from which the number of right limbs tested in the non-CAI group was calculated to be: 12/20*18 = 10.8. i.e., 11 participants in the non-CAI group tested the right side, and 7 participants tested the left side. The non-CAI participants were randomized to the computerized array, which was used to determine the side of limb to be tested. Before formal tests, participants familiarized themselves with the procedure by conducting at least 3 drop-landing trials. Then, they conducted formal drop-landing tests under single- and dual-task conditions in a randomized order.
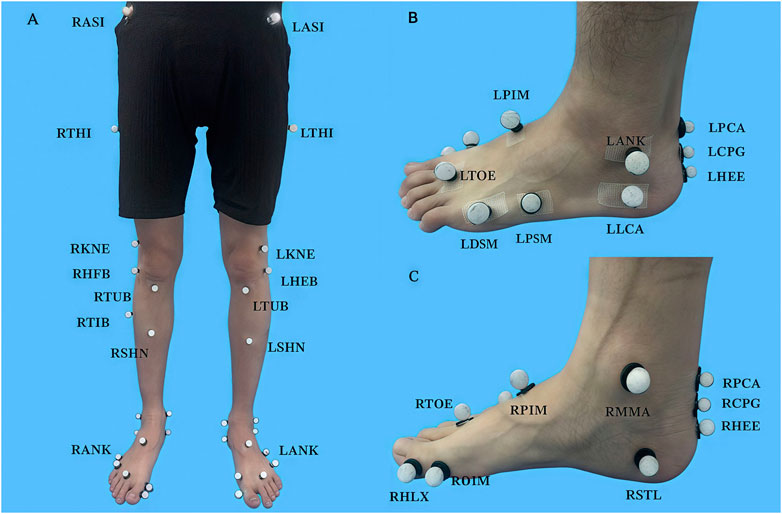
Figure 1. Oxford Foot Ankle Model (A) Front view of full markers. (B) Lateral view of foot markers. (C) Medial view of foot markers.
2.2.1 Drop-landing tests
Participants drop-landed from a height of 30 cm (Mackala et al., 2020) to a custom-made trap-door device consisting of three platforms, namely, take-off, flippable, and supporting platforms (Figure 2A). The height of 30 cm for landing has been proven safe and is widely applied in the literature (Shibata et al., 2023; Mokhtarzadeh et al., 2017; Watanabe et al., 2022; Li et al., 2018; Huang et al., 2024; Lim et al., 2020). The surface of the flippable platform would be flipped when subjected to a force >10 N. A marker was attached to the lateral edge of the flippable platform surface to identify the time point when it flipped. During the drop-landing test, the participants’ kinematic data were recorded by a 12-camera, 3D infrared motion capture system (Vicon Vantage V5, Oxford Metrics Limited, Oxford, United Kingdom), with a frequency of 100 Hz.
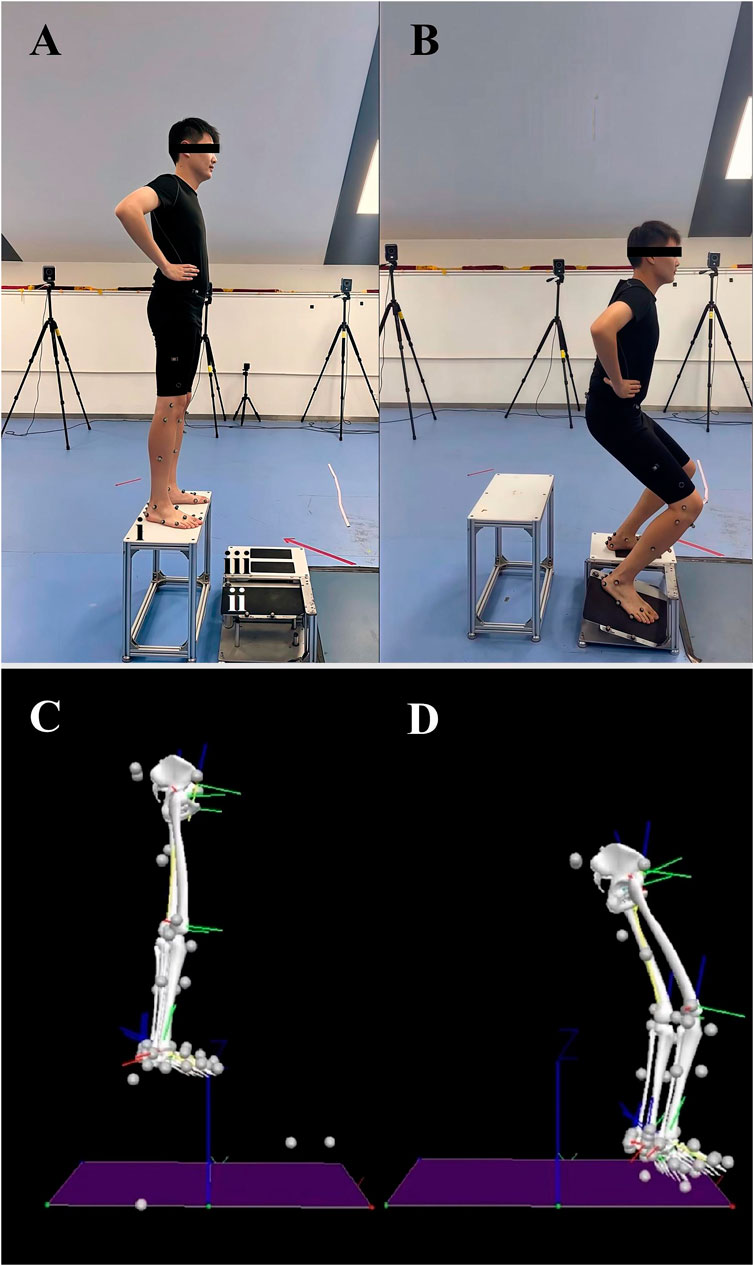
Figure 2. Illustration of drop-landing tests (A) Body position before drop-landing. i, take-off platform; ii. flippable platform; iii, supporting platform; (B) Body position after drop-landing; (C) A free body diagram of body position before drop-landing; (D) A free body diagram of body position after drop-landing.
Single-task conditions: Participants stood on the take-off platform, with their eyes looking straight ahead and hands on hips, and extended their affected or matched feet forward. They moved their bodies forward away from the take-off platform to minimize upward movement and then landed on the flippable and supporting platform with the affected or matched and the contralateral limbs, respectively (Figure 2). Participants performed three successful trials, defined as participants being able to stabilize their body and maintain the body position for at least 3 seconds after landing.
Dual-task conditions: During drop-landing, a subtraction of serial threes from a given three-digit number was performed simultaneously. In each trial, participants subtracted three for three times. Two subtractions were done before drop-landing, and after the second result was given, each participant extended their affected or matched feet forward and performed drop-landing as in single-task conditions, and they gave the result of the third subtraction immediately after landing (Figure 3). To ensure that the subtractions were calculated during landing, the timing of the third subtraction was strictly limited. Prior to the drop-landing test, participants performed consecutive subtractions for three times while sitting in a chair, and the mean time of the three subtractions were recorded as the “sitting subtraction time” (Wrightson et al., 2020). If the time taken for the third subtraction under dual-task conditions during drop-landing exceeded the “sit-subtraction time”, the trial would be deemed unsuccessful. Participants performed three successful trials.
2.3 Data reduction
Kinematic data were imported into Visual 3D (V6 professional, C-Motion, Maryland, United States), and low-pass filtered with a cutoff frequency of 12 Hz (Ford et al., 2007). Based on the marker protocol, an 11-segment Oxford foot ankle model was created and embedded in the motion capture files. The data were collected from the time point at landing to 200 ms after landing, defined by the movement of the markers affixed to the lateral edge of the flippable platform surface (Fu et al., 2014). The landing period was chosen because real ankle sprain occurs within it (Fong et al., 2009). The axis of ankle inversion/eversion is defined as the floating axis, which is the common axis perpendicular to both the Z-axis in the tibia/fibula coordinate system (the line extending from the tip of the medial malleolus to the tip of the lateral malleolus, directed rightward) and the Y-axis in the calcaneus coordinate system (the line aligning with the longitudinal axis of the tibia/fibula in the neutral position, pointing cranially) (Wu et al., 2002).
2.4 Variables
The ankle inversion angle was defined as the angle of rotation of the foot coordinate system relative to the tibial coordinate system in the coronal plane during the landing period.
The ankle inversion angular velocity is defined as the peak rate of the change of ankle inversion angle during the landing period, i.e., the peak value of angle increment per unit time.
2.5 Statistics
The normality of data distribution was examined using Shapiro-Wilk tests. Mixed model two-way ANOVAs were utilized to compare ankle inversion angle and angular velocity between single- and dual-task conditions among participants with and without CAI. If significant group (CAI vs non-CAI) by condition (single-task vs dual-task) interactions were detected, stratified t-tests with Bonferroni adjustment were used to conduct pairwise comparisons. Partial eta squared (η2p) was used to indicate the effect size of the two-way ANOVA’s interactions and main effects with the thresholds: 0.01∼0.06 for small, 0.06∼0.14 for moderate, and > 0.14 for large effect size (Pierce et al., 2004). Cohen’s d was used to indicate the effect size of post hoc pairwise comparison with the thresholds: <0.20 for trivial, 0.21∼0.50 for small, 0.51∼0.80 for medium, and >0.81 for large effect size (Cohen et al., 1988). The significance level was set at 0.05.
3 Results
The Shapiro-Wilk test indicated that all the dependent variables were normally distributed (P > 0.05). Significant group-by-condition interactions were detected in the ankle inversion angle (P = 0.04, η2p = 0.012) and ankle inversion angular velocity (P = 0.038, η2p = 0.114). Post hoc analysis showed that compared to single-task conditions, the ankle inversion angle (P = 0.003, d = 0.84) and ankle inversion angular velocity (P = 0.007, d = 0.91) were significantly decreased among individuals without CAI under dual-task conditions, whereas no significant differences were detected among individuals with CAI. Compared to those without CAI, the individuals with CAI had greater inversion angles under single- (P = 0.045, d = 0.65) and dual-task (P < 0.001, d = 1.13) conditions, and similarly, they had greater ankle inversion angular velocities under single- (P = 0.164, d = 0.46) and dual-task (P = 0.001, d = 1.03) conditions (Figure 4).
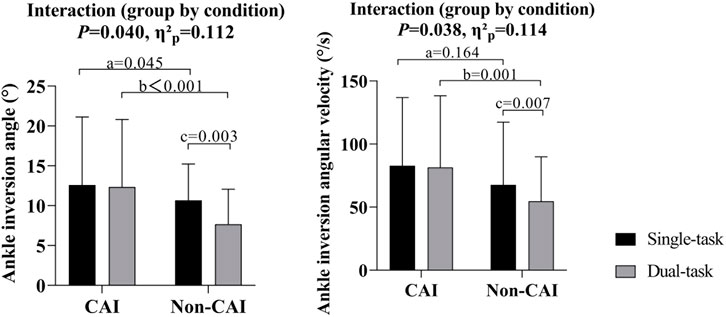
Figure 4. Ankle inversion angle and angular velocity in the CAI and non-CAI populations under single- and dual-task conditions. a. Significant difference between CAI and non-CAI populations under single-task conditions. b. Significant difference between CAI and non-CAI populations under dual-task conditions. c. Significant difference between single- and dual-task conditions in non-CAI populations.
4 Discussion
To our knowledge, this is the first study to determine the effects of the dual-task paradigm on the injury potential during drop-landing among individuals with and without CAI. The results supported Hypothesis 1, which predicted that individuals with CAI would have a higher injury potential compared to those without CAI, under both single-task and dual-task conditions. This was reflected by a greater ankle inversion angle and angular velocity among individuals with CAI. Conversely, Hypotheses 2 was not supported by the results. Hypothesis 2 proposed that, compared to single-task conditions, the injury potential would be higher among individuals with and without CAI under dual-task conditions, but this was not observed.
The results indicated that individuals with CAI exhibited greater ankle inversion angle and angular velocity compared to those without CAI under both conditions. This finding is supported by a previous study, which showed that individuals with CAI exhibited greater ankle inversion angle after initial contact during landing compared to copers (individuals who suffered ankle sprain but did not develop CAI) and healthy controls (Oh et al., 2024). We propose that the inability to limit excessive ankle inversion during landing is linked to dysfunction in ankle eversion muscles and lateral ankle ligaments. The ankle eversion muscles, especially the peroneal muscles, have been proved to have prolonged reaction time during sudden ankle inversion (Menacho Mde et al., 2010), as well as difficulty generating maximum ankle eversion torque in an inverted position among individuals with CAI (Dong et al., 2024). In the case of sudden ankle inversion, the injured anterior talofibular ligament is unable to limit the separation between the talus and fibula, making it difficult to minimize the ankle inversion angle and angular velocity (Rigby et al., 2015). As a result, individuals with CAI are more likely to be injured than individuals without CAI under both single- and dual-task conditions.
Contrary to Hypothesis 2, a surprising finding was that compared to single-task conditions, ankle inversion angle and angular velocity were lower under dual-task conditions among individuals without CAI. This may because participants adopted different movement strategies under dual-task conditions (McNevin and Wulf, 2002; Lacour et al., 2008). In the capacity sharing theory, when participants engage in dual-tasks, their attention shifts partly from the primary motor task to the supplementary task (in our study, a cognitive task). Consequently, cognitive resources are partially allocated to this additional task, the conscious control over body movements reduced (Lacour et al., 2008). To some extent, automatic and reflexive postural control strategies then take precedence to sustain motor performance (McNevin and Wulf, 2002; Lacour et al., 2008). This shift may result in superior motor performance compared to single-task conditions (Wulf et al., 2004; McNevin and Wulf, 2002; Vuillerme et al., 2000), avoiding the occurrence of injuries. For example, the lower ankle inversion angle and angular velocity observed in our study. A smaller ankle inversion angle indicates that the lateral ankle ligaments experience less strain due to inversion (Yıldız and Yalcın, 2013). Similarly, a lower ankle angular velocity indicates that muscles, such as the peroneal muscles, have adequate reaction time to activate and counteract excessive ankle inversion (Ashton-Miller et al., 1996), which all suggest a potential decrease in the risk of ankle sprains (Koshino et al., 2017).
In contrast to individuals without CAI, those with CAI did not show a decrease in injury potential when transitioning from single to dual-task conditions. The maladaptive neuroplastic changes at both spinal and cortical levels follows musculoskeletal injuries like CAI may be the reasons (Bruce et al., 2020), counteracting the injury potential that should be reduced under dual-task conditions. In the spinal level, the protective mechanism for protecting muscles from excessive strains after injuries, i.e., arthrogenic muscle inhibition (Norte et al., 2022), would affect the ability of voluntary muscle activation. This, in turn, may result in muscle weakness around ankle due to heightened activation of inhibitory interneurons synapsing in the motor neuron pool, thus reducing the efficiency of motoneuron recruitment by the CNS after injuries (Dong et al., 2024). Dong et al. demonstrated that arthrogenic muscle inhibition existed in peroneal muscles among individuals with CAI (Dong et al., 2024), which is the dominating muscles to prevent excessive ankle inversion (Ashton-Miller et al., 1996). The functions of the muscles may be inhibited by the protective mechanism at spinal level, leading to unchanged indicators concerning ankle inversion under dual-task conditions compared to single-task conditions among individuals with CAI. In the cortical level, numerous studies showed that the corticomotor excitability decreased in the projection areas of lower limb muscles around ankle in the M1 among individuals with CAI (Pietrosimone and Gribble, 2012; Terada et al., 2022; Nanbancha et al., 2019; Kosik et al., 2017), making them hard to activate the cortical motor neurons (Pietrosimone et al., 2012; McLeod et al., 2015), then leading to muscle weakness and failure of muscle activation (Pietrosimone et al., 2012). Activities of the secondary sensorimotor cortex and other cortical areas would increase to compensate for the declined cortical excitability in M1 to maintain motor performance (Needle et al., 2017; Bruce et al., 2020), but the cortical compensatory mechanism is vulnerable, which tends to collapse under dual-task conditions, leading to poorer motor performance and higher injury potential (Bruce et al., 2020). Similarly, the concurrent subtraction task may break the compensatory mechanisms at cortical level (Burcal et al., 2019), lead to the inability to confront ankle inversion from single-to dual-task conditions among individuals with CAI during landing.
There are three limitations to this study. First, participants were aware that the flippable platform surface of the trap-door device would flip during landing, so this was an anticipatory condition that may differ from a non-anticipatory condition when a real injury occurs. Second, the gender distribution was not uniform across the two groups in this study, which introduces a potential bias in the results due to gender imbalance. Considering that males and females utilize distinct feedforward control strategies during landing, with females typically activating their knee extensors earlier than males to mitigate the deficit in hip extensor rate tension development (Stearns-Reider and Powers, 2018; Di Giminiani et al., 2020), it is advisable for future research to maintain a balanced gender representation within subgroups to mitigate any potential confounding effects of gender on postural control outcomes. Last, this study did not include direct indicators of muscle activity and ligament status, and it is recommended that more attention be paid to neuromuscular factors in future studies to provide evidence through electromyographic measurements of muscle activity and computer simulation modeling calculations of ligament strain to better assess the potential of ankle injury.
5 Conclusion
Individuals with CAI have a reduced ability to limit ankle inversion, inferring increased susceptibility to ankle sprains. Under dual-task conditions, individuals without CAI limited their ankle inversion, while those with CAI did not, inferring a higher injury potential among those with CAI. Drop-landing, especially under dual-task conditions, poses a high risk of excessive ankle inversion for individuals with CAI.
Data availability statement
The raw data supporting the conclusions of this article will be made available by the authors, without undue reservation.
Ethics statement
The studies involving humans were approved by the Institutional Review Board of Shandong Sport University (Approval Number: 2023014) and was in accordance with the Declaration of Helsinki. The studies were conducted in accordance with the local legislation and institutional requirements. The participants provided their written informed consent to participate in this study.
Author contributions
CZ: Methodology, Data curation, Software, Writing–original draft. XL: Writing–original draft, Formal Analysis, Investigation. HG: Writing–original draft, Methodology, Validation. TZ: Methodology, Validation, Writing–original draft. XZ: Writing–original draft, Data curation. XH: Formal analysis, Writing–original draft, Writing–review and editing. PS: Conceptualization, Funding acquisition, Methodology, Project administration, Writing–review and editing.
Funding
The author(s) declare that financial support was received for the research, authorship, and/or publication of this article.
Acknowledgments
The authors would like to thank Yushan Miao, graduate students at Shandong Sport University, and Zhaoyang Yan, undergraduate student at Binzhou Medical University, for their participation in the experiment or data collection.
Conflict of interest
The authors declare that the research was conducted in the absence of any commercial or financial relationships that could be construed as a potential conflict of interest.
Publisher’s note
All claims expressed in this article are solely those of the authors and do not necessarily represent those of their affiliated organizations, or those of the publisher, the editors and the reviewers. Any product that may be evaluated in this article, or claim that may be made by its manufacturer, is not guaranteed or endorsed by the publisher.
References
Ardakani M. K., Wikstrom E. A., Minoonejad H., Rajabi R., Sharifnezhad A. (2019). Hop-stabilization training and landing biomechanics in athletes with chronic ankle instability: a randomized controlled trial. J. Athl. Train. 54, 1296–1303. doi:10.4085/1062-6050-550-17
Ashton-Miller J. A., Ottaviani R. A., Hutchinson C., Wojtys E. M. (1996). What best protects the inverted weightbearing ankle against further inversion? Evertor muscle strength compares favorably with shoe height, athletic tape, and three orthoses. Am. J. Sports Med. 24, 800–809. doi:10.1177/036354659602400616
Bruce A. S., Howard J. S., H V. A. N. W., Mcbride J. M., Needle A. R. (2020). The effects of transcranial direct current stimulation on chronic ankle instability. Med. Sci. Sports Exerc 52, 335–344. doi:10.1249/MSS.0000000000002129
Burcal C. J., Needle A. R., Custer L., Rosen A. B. (2019). The effects of cognitive loading on motor behavior in injured individuals: a systematic review. Sports Med. 49, 1233–1253. doi:10.1007/s40279-019-01116-7
Bush G., Luu P., Posner M. I. (2000). Cognitive and emotional influences in anterior cingulate cortex. Trends Cogn. Sci. 4, 215–222. doi:10.1016/s1364-6613(00)01483-2
Choi J. Y., Yoo T., Burcal C. J., Rosen A. B. (2023). Dual-task differences in individuals with chronic ankle instability: a systematic review with meta-analysis. Gait Posture 106, 28–33. doi:10.1016/j.gaitpost.2023.08.013
Cohen J., Cohen J., Cohen J. W., Cohen J., Cohen J., Cohen J., et al. (1988). Statistical power analysis for the behavioral sciences. Technometrics 31, 499–500. doi:10.2307/1270020
Di Giminiani R., Jozsef T., Francesco M. (2020). Gender differences on neuromuscular strategy during drop jump: a comment on Helm et al. (2019). Eur. J. Appl. Physiol. 120, 2555–2556. doi:10.1007/s00421-020-04465-8
Doherty C., Delahunt E., Caulfield B., Hertel J., Ryan J., Bleakley C. (2014). The incidence and prevalence of ankle sprain injury: a systematic review and meta-analysis of prospective epidemiological studies. Sports Med. 44, 123–140. doi:10.1007/s40279-013-0102-5
Dong S., Liu Y., Liu Z., Shen P., Sun H., Zhang P., et al. (2024). Can arthrogenic muscle inhibition exist in peroneal muscles among people with chronic ankle instability? A cross-sectional study. Sports Med. Open 10, 35. doi:10.1186/s40798-024-00710-y
Fong D. T., Ha S. C., Mok K. M., Chan C. W., Chan K. M. (2012). Kinematics analysis of ankle inversion ligamentous sprain injuries in sports: five cases from televised tennis competitions. Am. J. Sports Med. 40, 2627–2632. doi:10.1177/0363546512458259
Fong D. T., Hong Y., Chan L. K., Yung P. S., Chan K. M. (2007). A systematic review on ankle injury and ankle sprain in sports. Sports Med. 37, 73–94. doi:10.2165/00007256-200737010-00006
Fong D. T. H. Y., Shima Y., Krosshaug T., Yung P. S., Chan K. M. (2009). Biomechanics of supination ankle sprain: a case report of an accidental injury event in the laboratory. Am. J. Sports Med. 37 (4), 822–827. doi:10.1177/0363546508328102
Ford K. R., Myer G. D., Hewett T. E. (2007). Reliability of landing 3D motion analysis: implications for longitudinal analyses. Med. Sci. Sports Exerc 39, 2021–2028. doi:10.1249/mss.0b013e318149332d
Fu W., Fang Y., Liu Y., Hou J. (2014). The effect of high-top and low-top shoes on ankle inversion kinematics and muscle activation in landing on a tilted surface. J. Foot Ankle Res. 7, 14. doi:10.1186/1757-1146-7-14
Gribble P. A. (2019). Evaluating and differentiating ankle instability. J. Athl. Train. 54, 617–627. doi:10.4085/1062-6050-484-17
Gribble P. A., Bleakley C. M., Caulfield B. M., Docherty C. L., Fourchet F., Fong D. T., et al. (2016a). 2016 consensus statement of the International Ankle Consortium: prevalence, impact and long-term consequences of lateral ankle sprains. Br. J. Sports Med. 50, 1493–1495. doi:10.1136/bjsports-2016-096188
Gribble P. A., Bleakley C. M., Caulfield B. M., Docherty C. L., Fourchet F., Fong D. T., et al. (2016b). Evidence review for the 2016 International Ankle Consortium consensus statement on the prevalence, impact and long-term consequences of lateral ankle sprains. Br. J. Sports Med. 50, 1496–1505. doi:10.1136/bjsports-2016-096189
Herzog M. M., Kerr Z. Y., Marshall S. W., Wikstrom E. A. (2019). Epidemiology of ankle sprains and chronic ankle instability. J. Athl. Train. 54, 603–610. doi:10.4085/1062-6050-447-17
Hiller C. E., Refshauge K. M., Bundy A. C., Herbert R. D., Kilbreath S. L. (2006). The Cumberland ankle instability tool: a report of validity and reliability testing. Arch. Phys. Med. Rehabil. 87, 1235–1241. doi:10.1016/j.apmr.2006.05.022
Huang X., Gao H., Fu H. (2024). Effects of transcranial direct current stimulation combined with Bosu ball training on the injury potential during drop landing in people with chronic ankle instability. Front. Physiol. 15, 1451556. doi:10.3389/fphys.2024.1451556
Hubbard-Turner T., Turner M. J. (2015). Physical activity levels in college students with chronic ankle instability. J. Athl. Train. 50, 742–747. doi:10.4085/1062-6050-50.3.05
Kemler E., Van De Port I., Valkenberg H., Hoes A. W., Backx F. J. (2015). Ankle injuries in The Netherlands: trends over 10-25 years. Scand. J. Med. Sci. Sports 25, 331–337. doi:10.1111/sms.12248
Konradsen L., Bech L., Ehrenbjerg M., Nickelsen T. (2002). Seven years follow-up after ankle inversion trauma. Scand. J. Med. Sci. Sports 12, 129–135. doi:10.1034/j.1600-0838.2002.02104.x
Koshino Y., Ishida T., Yamanaka M., Samukawa M., Kobayashi T., Tohyama H. (2017). Toe-in landing increases the ankle inversion angle and moment during single-leg landing: implications in the prevention of lateral ankle sprains. J. Sport Rehabil. 26, 530–535. doi:10.1123/jsr.2016-0004
Kosik K. B., Terada M., Drinkard C. P., Mccann R. S., Gribble P. A. (2017). Potential corticomotor plasticity in those with and without chronic ankle instability. Med. Sci. Sports Exerc 49, 141–149. doi:10.1249/MSS.0000000000001066
Lacour M., Bernard-Demanze L., Dumitrescu M. (2008). Posture control, aging, and attention resources: models and posture-analysis methods. Neurophysiol. Clin. 38, 411–421. doi:10.1016/j.neucli.2008.09.005
Lim Y. Y., Sterzing T., Teo C. J. Y., Alonzo R., Pan J. W., Teng P. S. P., et al. (2020). Between-limb asymmetry in kinetic and temporal characteristics during bilateral plyometric drop jumps from different heights. J. Sports Sci. 38, 1605–1614. doi:10.1080/02640414.2020.1752535
Li Y., Ko J., Walker M. A., Brown C. N., Schmidt J. D., Kim S. H., et al. (2018). Does chronic ankle instability influence lower extremity muscle activation of females during landing? J. Electromyogr. Kinesiol 38, 81–87. doi:10.1016/j.jelekin.2017.11.009
Mackala K., Rauter S., Simenko J., Kreft R., Stodolka J., Krizaj J., et al. (2020). The effect of height on drop jumps in relation to somatic parameters and landing kinetics. Int. J. Environ. Res. Public Health 17, 5886. doi:10.3390/ijerph17165886
Mccahill J., Stebbins J., Theologis T. (2008). Use of the Oxford foot model in clinical practice. J. Foot Ankle Res. 1, O28. doi:10.1186/1757-1146-1-s1-o28
Mcleod M. M., Gribble P. A., Pietrosimone B. G. (2015). Chronic ankle instability and neural excitability of the lower extremity. J. Athl. Train. 50, 847–853. doi:10.4085/1062-6050-50.4.06
Mcnevin N. H., Wulf G. (2002). Attentional focus on supra-postural tasks affects postural control. Hum. Mov. Sci. 21, 187–202. doi:10.1016/s0167-9457(02)00095-7
Medina Mckeon J. M., Hoch M. C. (2019). The ankle-joint complex: a kinesiologic approach to lateral ankle sprains. J. Athl. Train. 54, 589–602. doi:10.4085/1062-6050-472-17
Menacho Mde O., Pereira H. M., Oliveira B. I., Chagas L. M., Toyohara M. T., Cardoso J. R. (2010). The peroneus reaction time during sudden inversion test: systematic review. J. Electromyogr. Kinesiol 20, 559–565. doi:10.1016/j.jelekin.2009.11.007
Mokhtarzadeh H., Yeow C. H., Goh J. C. H., Oetomo D., Ewing K., Lee P. V. S. (2017). Antagonist muscle co-contraction during a double-leg landing maneuver at two heights. Comput. Methods Biomech. Biomed. Engin 20, 1382–1393. doi:10.1080/10255842.2017.1366992
Nanbancha A., Tretriluxana J., Limroongreungrat W., Sinsurin K. (2019). Decreased supraspinal control and neuromuscular function controlling the ankle joint in athletes with chronic ankle instability. Eur. J. Appl. Physiol. 119, 2041–2052. doi:10.1007/s00421-019-04191-w
Needle A. R., Lepley A. S., Grooms D. R. (2017). Central nervous system adaptation after ligamentous injury: a summary of theories, evidence, and clinical interpretation. Sports Med. 47, 1271–1288. doi:10.1007/s40279-016-0666-y
Norte G., Rush J., Sherman D. (2022). Arthrogenic muscle inhibition: best evidence, mechanisms, and theory for treating the unseen in clinical rehabilitation. J. Sport Rehabil. 31, 717–735. doi:10.1123/jsr.2021-0139
Oh M., Lee H., Han S., Hopkins J. T. (2024). Postural control measured before and after simulated ankle inversion landings among individuals with chronic ankle instability, copers, and controls. Gait Posture 107, 17–22. doi:10.1016/j.gaitpost.2023.09.002
Pierce C. A., Block R. A., Aguinis H. (2004). Cautionary note on reporting eta-squared values from multifactor ANOVA designs. Educ. Psychol. Meas. 64, 916–924. doi:10.1177/0013164404264848
Pietrosimone B. G., Gribble P. A. (2012). Chronic ankle instability and corticomotor excitability of the fibularis longus muscle. J. Athl. Train. 47, 621–626. doi:10.4085/1062-6050-47.6.11
Pietrosimone B. G., Mcleod M. M., Lepley A. S. (2012). A theoretical framework for understanding neuromuscular response to lower extremity joint injury. Sports Health 4, 31–35. doi:10.1177/1941738111428251
Rahnama L., Salavati M., Akhbari B., Mazaheri M. (2010). Attentional demands and postural control in athletes with and without functional ankle instability. J. Orthop. Sports Phys. Ther. 40, 180–187. doi:10.2519/jospt.2010.3188
Rigby R., Cottom J. M., Rozin R. (2015). Isolated calcaneofibular ligament injury: a report of two cases. J. Foot Ankle Surg. 54, 487–489. doi:10.1053/j.jfas.2014.08.017
Rosen A. B., Mcgrath M. L., Maerlender A. L. (2021). Males with chronic ankle instability demonstrate deficits in neurocognitive function compared to control and copers. Res. Sports Med. 29, 116–128. doi:10.1080/15438627.2020.1723099
Shen Y., Wang W., Wang Y., Yang L., Yuan C., Yang Y., et al. (2022). Not only in sensorimotor network: local and distant cerebral inherent activity of chronic ankle instability-A resting-state fMRI study. Front. Neurosci. 16, 835538. doi:10.3389/fnins.2022.835538
Shibata S., Takemura M., Miyakawa S. (2023). Kinematics, kinetics and muscle activity analysis during single-leg drop-jump landing followed by an unanticipated task: focusing on differences in neurocognitive function. Int. J. Sports Phys. Ther. 18, 1085–1093. doi:10.26603/001c.86124
Shiravi Z., Talebian Moghadam S., Hadian M. R., Olyaei G. (2017). Effect of cognitive task on postural control of the patients with chronic ankle instability during single and double leg standing. J. Bodyw. Mov. Ther. 21, 58–62. doi:10.1016/j.jbmt.2016.05.001
Simpson J. D., Koldenhoven R. M., Wilson S. J., Stewart E. M., Turner A. J., Chander H., et al. (2022). Lower extremity joint kinematics of a simulated lateral ankle sprain after drop landings in participants with chronic ankle instability. Sports Biomech. 21, 428–446. doi:10.1080/14763141.2021.1908414
Stearns-Reider K. M., Powers C. M. (2018). Rate of torque development and feedforward control of the hip and knee extensors: gender differences. J. Mot. Behav. 50, 321–329. doi:10.1080/00222895.2017.1363692
Tavakoli S., Forghany S., Nester C. (2016). The effect of dual tasking on foot kinematics in people with functional ankle instability. Gait Posture 49, 364–370. doi:10.1016/j.gaitpost.2016.07.302
Terada M., Kosik K. B., Mccann R. S., Drinkard C., Gribble P. A. (2022). Corticospinal activity during a single-leg stance in people with chronic ankle instability. J. Sport Health Sci. 11, 58–66. doi:10.1016/j.jshs.2020.08.008
Terrier R., Rose-Dulcina K., Toschi B., Forestier N. (2014). Impaired control of weight bearing ankle inversion in subjects with chronic ankle instability. Clin. Biomech. (Bristol, Avon) 29, 439–443. doi:10.1016/j.clinbiomech.2014.01.005
Vuillerme N., Nougier V., Teasdale N. (2000). Effects of a reaction time task on postural control in humans. Neurosci. Lett. 291, 77–80. doi:10.1016/s0304-3940(00)01374-4
Watanabe K., Koshino Y., Ishida T., Samukawa M., Tohyama H. (2022). Energy dissipation during single-leg landing from three heights in individuals with and without chronic ankle instability. Sports Biomech. 21, 408–427. doi:10.1080/14763141.2021.2009549
Wrightson J. G., SchäFER L., Smeeton N. J. (2020). Dual-task prioritization during overground and treadmill walking in healthy adults. Gait Posture 75, 109–114. doi:10.1016/j.gaitpost.2019.08.007
Wu G., Siegler S., Allard P., Kirtley C., Leardini A., Rosenbaum D., et al. (2002). ISB recommendation on definitions of joint coordinate system of various joints for the reporting of human joint motion--part I: ankle, hip, and spine. International Society of Biomechanics. J. Biomech. 35, 543–548. doi:10.1016/s0021-9290(01)00222-6
Wulf G., Mercer J., Mcnevin N., Guadagnoli M. A. (2004). Reciprocal influences of attentional focus on postural and suprapostural task performance. J. Mot. Behav. 36, 189–199. doi:10.3200/JMBR.36.2.189-199
Xu D., Zhou H., Quan W., Gusztav F., Wang M., Baker J. S., et al. (2023). Accurately and effectively predict the ACL force: utilizing biomechanical landing pattern before and after-fatigue. Comput. Methods Programs Biomed. 241, 107761. doi:10.1016/j.cmpb.2023.107761
Xu D., Zhou H., Quan W., Ma X., Chon T. E., Fernandez J., et al. (2024a). New insights optimize landing strategies to reduce lower limb injury risk. Cyborg Bionic Syst. 5, 0126. doi:10.34133/cbsystems.0126
Xu D., Zhou H., Wang M., Ma X., Gusztav F., Chon T. E., et al. (2024b). Contribution of ankle motion pattern during landing to reduce the knee-related injury risk. Comput. Biol. Med. 180, 108965. doi:10.1016/j.compbiomed.2024.108965
YıLDıZ S., YalcıN B. (2013). The anterior talofibular and calcaneofibular ligaments: an anatomic study. Surg. Radiol. Anat. 35, 511–516. doi:10.1007/s00276-012-1071-3
Keywords: ankle sprain, dual-task paradigm, drop-landing, ankle inversion angle, ankle inversion angular velocity
Citation: Zhong C, Luo X, Gao H, Zhang T, Zhu X, Huang X and Shen P (2024) Effects of dual-task paradigm on the injury potential during landing among individuals with chronic ankle instability. Front. Physiol. 15:1473844. doi: 10.3389/fphys.2024.1473844
Received: 31 July 2024; Accepted: 07 November 2024;
Published: 28 November 2024.
Edited by:
Pui Wah Kong, Nanyang Technological University, SingaporeReviewed by:
Riccardo Di Giminiani, University of L’Aquila, ItalyKam Ming Mok, The Chinese University of Hong Kong, China
Datao Xu, Ningbo University, China
Copyright © 2024 Zhong, Luo, Gao, Zhang, Zhu, Huang and Shen. This is an open-access article distributed under the terms of the Creative Commons Attribution License (CC BY). The use, distribution or reproduction in other forums is permitted, provided the original author(s) and the copyright owner(s) are credited and that the original publication in this journal is cited, in accordance with accepted academic practice. No use, distribution or reproduction is permitted which does not comply with these terms.
*Correspondence: Peixin Shen, MTgzMjMwMjIwNTRAMTYzLmNvbQ==
†These authors have contributed equally to this work and share first authorship