- 1Biomedical Engineering, University of Connecticut, Storrs, CT, United States
- 2Analog Devices, Wilmington, MA, United States
- 3Naval Submarine Medical Research Laboratory, Groton, CT, United States
- 4Leidos, Reston, VA, United States
Introduction: This study investigated the differences between males and females in autonomic functions and cognitive performance during cold-air exposure and cold-water partial-immersion compared to a room temperature-air environment. Although several studies have investigated the effects of cold-air or cold-water exposures on autonomic function and cognitive performance, biological sex differences are often under-researched.
Methods: Twenty-two males and nineteen females participated in the current study. Subjects completed a battery of cognitive tasks based upon those used within the Defense Automated Neurobehavioral Assessment (DANA), consisting of five subtasks that assess simple and procedural reaction time, spatial manipulation, attention, and immediate memory. In total, subjects took the battery within a 15-minute period across 30-minute intervals throughout the duration of environmental exposure. Across three separate days, subjects were exposed to three different environmental conditions: room temperature air (23°C), cold air (10°C), and cold water (15°C; in which subjects were immersed up to their necks). Room temperature and cold-air conditions consisted of five sessions (about 2.5 h), and the cold-water condition consisted of three sessions (about 1.5 h). During each experimental condition, physiological data were collected to assess autonomic function, including electrodermal activity (EDA) data and heart rate variability (HRV) derived from electrocardiogram signals.
Results: Females showed slower reaction time in spatial manipulation tasks, immediate memory, and attention during cold-air exposures compared to room temperature air, whereas the performance of males were similar or better during cold-air exposures compared to room temperature air. Cold-water immersion affected the immediate memory performance of males. Both males and females exhibited smaller EDA amplitudes during cold-air and cold-water conditions compared to room temperature air. For HRV, only male subjects exhibited significantly greater values in low-frequency and very-low-frequency components during cold air exposure compared to the normal condition.
Discussion: Sex introduces important differences in cognitive performance and autonomic functions during exposure to cold-air and cold-water. Therefore, sex should be considered when assessing the autonomic nervous system in cold environments and when establishing optimal thermal clothing for performance in operational environments. Our findings can assist with determination of operational clothing, temperature in operating environment, and personnel deployment to operational sites, particularly in settings involving both males and females.
Introduction
Prolonged exposure to cold temperatures can have negative effects on cognitive performance such as attention, mental processing speed, executive function, and memory (Pilcher et al., 2002; Falla et al., 2021; Yang et al., 2021). These effects are likely associated with the body’s natural physiological and thermoregulatory responses. When humans are exposed to cold air or immersed in cold water, their body undergoes thermoregulatory responses to maintain their core temperature within a narrow range (Osilla et al., 2018). Additionally, immersion in cold water is influenced by an elevated hydrostatic pressure due to the density of water, which is 800 times higher than that of air (Jimenez et al., 2010). The sympathetic nervous system (SNS) activates vasoconstriction in the peripheral blood vessels, reducing blood flow to the skin and other superficial tissues, which decreases overall heat loss (Campbell, 2008). The SNS also activates brown adipose tissue capable of converting energy into heat (Lee et al., 2013). Studies have also shown increases in parasympathetic nervous system (PNS) activity after initial SNS response, and also during cold habituation (Harinath et al., 2005; Mäkinen et al., 2008; Castellani and Young, 2016; Lundell et al., 2020). The mechanisms and interplay of SNS and PNS functions employed during thermoregulation are unclear; assessing the physiological signals of the autonomic nervous system (ANS) may help clarify these mechanisms and their associations with cognitive performance in cold-air exposures and cold-water immersion.
The ANS is often assessed via heart rate variability (HRV), which is typically derived from data collected through an electrocardiogram (ECG), a non-invasive measure of the electrical activity generated by the heart (Shaffer and Ginsberg, 2017). HRV can provide insights into the interplay of cardiac SNS and PNS functions during cold exposure, although it is often difficult to distinguish the exact mechanism behind each function/system. Using HRV analysis, researchers found a significant increase in PNS activity from subjects who underwent cold-air exposure or cold-water immersion (Mäkinen et al., 2008; Lundell et al., 2020). Despite their insightful outcomes, these studies were limited to assessing cardiac PNS, as none of the HRV indices provided exclusive assessment of cardiac SNS. For example, the high frequency component of HRV is primarily influenced by cardiac PNS, whereas both cardiac SNS and PNS have been found to affect the low frequency components of HRV (Shaffer and Ginsberg, 2017).
Electrodermal activity (EDA) has shown promising ability to exclusively assess SNS activity (Posada-Quintero and Chon, 2020). EDA is a non-invasive measure of sudomotor activity, which is activated by the sympathetic function. EDA devices measure the variation in the electrical conductance of the skin predominantly affected by the SNS (Shields et al., 1987). This advantage enables researchers to get insights into the ANS, with more reliable assessments of SNS activity using EDA alone or in complement with HRV (Visnovcova et al., 2013; Posada-Quintero and Bolkhovsky, 2019; Ghiasi et al., 2020). EDA is often decomposed into two distinct components: phasic and tonic. The phasic component reflects the rapid EDA changes in response to a startle-like stimulus or event, while the tonic component reflects the overall level of electrical conductance of the skin, influenced by factors such as baseline arousal, hydration levels, and skin temperature (Posada-Quintero and Chon, 2020). Additionally, the time-and-frequency-domain analysis, such as the time-varying index of EDA (TVSymp), has shown high sensitivities in assessing SNS activity (Posada-Quintero et al., 2016; Kong et al., 2021b; Tran et al., 2022; Kong et al., 2023).
Although the effects of cold air exposure or cold-water immersion on autonomic function and cognitive performance have been studied, many of these studies have not considered biological sex as a confounding factor. Sex is an important factor in both the thermoregulation mechanism and the assessment of autonomic function (Tikuisis et al., 2000; Kaikaew et al., 2018; Mengel et al., 2020). Males and females generally have different body compositions that can affect thermoregulation, such as body fat distribution, muscle mass, hormonal differences, and body size (Kaciuba-Uscilko and Grucza, 2001; Castellani and Young, 2016; Bredella, 2017). Furthermore, a meta-analysis by Koenig and Thayer concluded that sex differences in HRV should be taken into account in HRV analysis (Koenig and Thayer, 2016). Studies have shown that EDA has different dynamics between males and females (Bari, 2020; Kong et al., 2021a), but these studies did not exhibit statistically significant differences in EDA metrics. Given that males and females can react to cold exposure differently, we hypothesized that there are sex differences in both EDA and HRV metrics and cognitive performance during cold environments. The current study investigated differences in cognitive function and autonomic function during cold-air exposure and cold-water immersion of males and females. Understanding sex differences in cold environments can be beneficial for operational conditions where both males and females work, such as military settings.
Materials and methods
Subjects
Data were collected from 41 subjects, whose mean age was 24.32 ± 5.03 years old. Subjects were recruited from around the University of Connecticut (UConn). Subjects were asked to come to the experiment site for three different days, during which they performed a cognitive task battery while exposed to one of three different environmental conditions: room temperature air (i.e., “normal”) (23°C), cold-air (10°C), and cold-water (15°C). Subjects were compensated $50 (USD) a day and given an extra $150 (USD) if they completed all 3 days/conditions. Subjects underwent an informed consent procedure and completed a questionnaire of demographic information prior to participating in the experiment.
Stimuli and materials
Task battery for the assessment of cognitive performance
Used within the experimental cognitive task battery were five tasks derived from the Defense Automated Neurobehavioral Assessment (DANA), a battery designed to evaluate cognitive and psychological performance in operational environments (Lathan et al., 2013). This included 1) go/no-go (GNG), 2) spatial discrimination (SPD), 3) procedural reaction time (PRO), 4) simple reaction time (SRT), and 5) code substitution (CDS). This set of cognitive task battery evaluates abilities ranging from simple reaction time (SRT) and executive functioning with easy decision-making capabilities (PRO) to measure of sustained attention and impulsivity (GNG), visuospatial analytic ability (SPD), and short-term memory (CDS) (Lathan et al., 2013). Note that DANA has shown consistency and reliability across simulated environmental conditions, including a cold condition (around 3°C) (Haran et al., 2015). The battery was administered on a Galaxy Tab S7 tablet device (Samsung, S. Korea), using the Inquisit Millisecond software (Millisecond Software, LLC). Supplementary Figure S1 shows an example of screenshots of the task battery.
Go/No-go (GNG)
Subjects were shown a rectangle on the screen in either a horizontal or vertical orientation. They were then instructed to press the space bar button as fast as possible if the rectangle turned green, indicating a “go” signal, regardless of its orientation. They were also told not to respond if the rectangle turned blue, indicating a “no-go” signal. The goal of this task, which took approximately 2 min and 30 s to complete, was to assess subjects’ speed and accuracy of selecting targets, including their ability to make commissions and omissions. Subjects’ average reaction time and the number of incorrect responses were used to evaluate their performance.
Spatial discrimination (SPD)
Subjects were shown a set of two histograms, each consisting of 4 bars, on the screen. After the initial presentation of the first set, a second set of histograms was shown, which were rotated by either 0°, 90°, 180°, or 270° angles. The subjects were then required to determine whether the two sets of histograms were congruent or not. The purpose of this task was to assess the subjects’ spatial manipulation abilities, and it took approximately 3 min to complete. Subjects’ performance was measured by calculating their average reaction time and the number of incorrect responses.
Procedural reaction time (PRO)
Subjects were shown a horizontal row of four boxes onscreen. When a box turned red, subjects were asked to tap on buttons presented at the bottom of the screen that corresponded to each of those boxes. The objective of this task was to assess the subjects’ reaction time and their ability to accurately respond while inhibiting premature responses. The task took approximately 90 s to complete, depending on the individual’s reaction time. The subjects’ average reaction time, the number of incorrect responses, and the number of premature responses were calculated to evaluate their performance.
Simple reaction time (SRT)
Subjects were instructed to tap anywhere onscreen as soon as a red circle appeared. The task took approximately 90 s to complete and was designed to assess the subjects’ reaction time. The subjects’ average reaction time and the number of lapses (defined as instances where the reaction time exceeded 0.5 s) were calculated to evaluate the subjects’ ability to sustain attention and quickly respond to stimuli.
Code substitution (CDS)
Subjects were shown a matrix of symbols and were instructed to enter the corresponding digits as quickly and accurately as possible, based on a key of symbol-digit pairs. The matrix of symbols was randomized for each trial. Subjects were given 2 min to complete as much of the matrix as they could. The purpose of this task was to evaluate the subjects’ immediate memory and attention abilities. Subject performance was evaluated by calculating the average reaction time of each input and the error rate, which was determined by dividing the number of incorrect responses by the total number of responses.
Experimental conditions
The experiment assessed cognitive performance and physiological response in three different environmental conditions: 1) the “normal-air condition” which had a room temperature of 23°C, 2) the “cold-air condition” which had a room temperature of 10°C, and 3) the “cold-water condition” which required subjects to be immersed up to the neck in water with a temperature of 15°C. The relative humidity was maintained at 35% ± 1% for the normal-air condition; while it was 57% ± 1% for the cold-air condition. The subjects experienced each condition on three different days at a similar time, with the order of the environmental conditions pseudo-randomly assigned. To control for clothing insulation across subjects, subjects were provided with clothing to wear in each condition. Clothing was worn over all physiological sensors. For the normal-air and cold-air conditions, subjects were given a long sleeve cotton shirt, long cotton pants, and cotton ankle socks, which provided a clothing insulation (Clo) rating slightly less than 1, in which 1 Clo = 0.155 m2K/W. To perform the task battery, subjects used a smart tablet while seated at a desk. For the cold-water condition, subjects wore a 3 mm Henderson Aqua Lock dive wetsuit and 3 mm Aqua Lung diving boots (approximately 0.25 Clo) (Tikuisis and Keefe, 1996). During the cold-water sessions, subjects sat in water filled up to the neck, in the Whitehall S110M Mobile Whirlpool bathtub, and a bathtub tray was provided for the subjects to use while performing the tablet-based cognitive task battery.
Mean body temperature
Skin temperature was calculated from three different body regions. Dermal patch sensors (VitalSense, Philips Respironics, Bend, Oregon, United States) were used to collect the skin temperature data from the back of the dominant hand, the left calf, and the forehead. Also, subjects’ core temperature was obtained using an ingestible capsule sensor (VitalSense, Philips Respironics, Bend, Oregon, United States) (Travers et al., 2016). These patches and ingestible sensors transmitted data to the Equivital EQ02+ LifeMonitor’s Sensor Electronic Modules (Equivital, Cambridge, United Kingdom) every 5 seconds. Using ISO Standard weighting coefficients (ISO 9886:2004 en, 2004), the area-weighted skin temperature (Tskin) was calculated from the three skin temperature measurements. Since only Tskin data were collected from three locations, the weighting coefficients were proportionally adjusted to have a sum of 1 (Supplementary Table S1). Finally, the mean body temperature (MBT) was calculated using Burton’s formula based on Tskin and Tcore (Burton, 1935; Lenhardt and Sessler, 2006), as follows:
Electrodermal activity (EDA) and heart rate variability (HRV)
Raw EDA signals were recorded at a frequency of 4 Hz using the Empatica E4 (Empatica, Boston, MA, United States), which was placed on the non-dominant wrist. Ag/AgCl electrodes were attached to the index and middle fingers and connected to Empatica E4 to record EDA signals. The raw EDA signals were then decomposed into phasic (PhEDA) and tonic (TonEDA) components using the cvxEDA technique (Greco et al., 2015). PhEDA and TonEDA correspond to fast and slow components of EDA, respectively. The time-varying index of sympathetic activity (TVSymp) was derived from the raw EDA signals. TVSymp is derived from reconstructed signals using components within a frequency range of 0.08–0.24 Hz, and has shown high sensitivity to SNS activity, such as noxious stimuli, stress, and dental pain (Posada-Quintero et al., 2016; Posada-Quintero et al., 2020; Kong et al., 2021c; Kong et al., 2023; Tran et al., 2022). Non-specific skin conductance responses (NSSCR) were calculated by counting the number of skin conductance responses that met a set threshold in a given time period. The threshold is normally set to 0.05 (Posada-Quintero and Chon, 2020). However, three different threshold values were assessed, which are 0.05, 0.01, and 0.005 μS, as cold acclimatization can reduce perspiration rates (Mcmullen et al., 2013).
To obtain HRV, lead-2 electrocardiogram (ECG) signals were recorded using multiple devices, to minimize the effect of data corruption. For normal and cold-air conditions, the ScottCare Chroma Holter Monitor (ScottCare, Cleveland, OH, United States) was used with Ag/AgCl electrodes, which were attached to the upper chest and the left and right lower rib areas. For the cold-water condition, Rozinn RZ153+ Holter Recorder (Cardiac Direct, Ventura, CA) was used with reusable hydrophobic electrodes comprised of carbon black powder and polydimethylsiloxane (CB/PDMS), which were attached to the upper chest and the left lower rib areas (Reyes et al., 2014). Also, ECG signals were collected using the Equivital EQ02+ LifeMonitor for all conditions. Finally, photoplethysmogram (PPG) signals were collected from the non-dominant wrist using Empatica E4.
HRV was calculated from R-peak intervals obtained using an approach based on the complete ensemble empirical mode decomposition (CEEMD) (Torres et al., 2011; Hossain et al., 2019). If R peaks were not identifiable due to artifacts, HRV was calculated using PPG signals (provided they were devoid of noise artifacts). Pulse peaks of PPG signals were obtained using an accurate peak detection technique only when the PPG quality was sufficiently high (Han et al., 2022). The following HRV indices were calculated: high- (HF), low- (LF), and very-low-frequency (vLF) power, LF/HF ratio, heart rate (HR), the root mean square of successive differences (RMSSD) between normal heartbeats, and the standard deviation of the interbeat intervals of normal sinus beats (SDNN).
Protocol
Subjects were instructed to get a minimum of 8 h of sleep the night before each testing day. They were also asked to avoid consuming any depressants and stimulants, such as nicotine, alcohol, and caffeine, for at least 24-h before a testing session. Finally, subjects were instructed to consume food no less than 3 h before each testing day. All condition testing was conducted at similar times of day on the fourth floor of the Engineering and Science Building at UConn Storrs Campus. On the first day of participation subjects completed a demographic questionnaire and provided information including age, sex, height (in), weight (lbs), waist circumferences (cm), smoking, and fitness (rated on a scale from 1–3: sedentary, moderately, vigorously active); skinfold measurements (mm) were also taken (Jackson and Pollock, 1985). Note that the units for height and weight were then converted to centimeters and kilograms. Different body areas were measured between male and female subjects for skinfold measurements. Skinfold measurements of the chest, abdomen, and thigh were obtained for male subjects, whereas thigh, triceps, and suprailium measurements were collected from female subjects (Jackson and Pollock, 1978; Jackson and Pollock, 1985; Jackson et al., 1980). Body mass index (BMI) was calculated using height and weight. Also body fat was estimated for both male and female subjects using Jackson and Pollock’s 3-site skinfold equations and Siri’s formula (Siri, 1956; Jackson and Pollock, 1978; Jackson et al., 1980).
Subjects were provided clothing, a capsule sensor with a cup of water, three dermal patch sensors, and the Equivital EQ02+ LifeMonitor vest. Before the sensors were placed, the skin areas were cleaned with 70% isopropyl alcohol. They were then asked to attach all electrodes to their respective, aforementioned placements for EDA and ECG recording. They were asked to wear the Empatica E4 on their non-dominant wrist. For the cold-water condition, subjects wore a nitrile glove on the non-dominant forearm to protect the Empatica E4 sensors and electrodes from water.
Figure 1 shows the sequence of all environmental conditions. At the start of each condition, 5 min were provided to participants for hemodynamic stabilization before starting the battery of cognitive tasks. Subjects completed two practice sessions of the task battery to minimize the effect of learning on the first day, because a mild learning effect has been observed during the first and second trials of DANA from which our cognitive task battery was derived (Lathan et al., 2013). A total of five experimental sessions of the cognitive task battery were conducted for the normal and cold-air conditions, whereas the cold-water condition consisted of three sessions to minimize the risk of hypothermia. Each session of the battery took approximately 15 min, and the sessions were repeated every 30 min. During each session for the cold-water condition, subjects were immersed in water up to the upper chest to perform the battery of cognitive tasks and up to the neck when not required to conduct the tasks. This research complied with tenets of the Declaration of Helsinki and was approved by the Institutional Review Board at the University of Connecticut (UConn) and the Naval Submarine Medical Research Laboratory (NSMRL).
Statistics
For EDA and HRV indices, 5-min segments were used from the middle portion of each session (2.5 min forwards and backwards from the midpoint). For EDA indices, mean values for each index were used. R’s LMER function was used to perform a three-way analysis of variance (ANOVA) with a random intercept for participant (Bates, 2005; Bates et al., 2015) to see if there was a significant effect in sex, between conditions and across exposure time, on cognitive task battery performance and physiological metrics. Note that the LMER function treats missing data as missing at random (MAR) by removing rows containing missing responses and by fitting models based on maximum likelihood estimation (De Boeck et al., 2011). If a significant effect was observed, estimated marginal means (i.e., least square means) of the fitted LMER models were obtained, using R’s emmeans function with the Tukey method to perform a pairwise post hoc test (Lenth et al., 2019). To compare the normal and cold-air conditions, segments from the five sessions during the task battery were compared (i.e., five-session analysis). To compare the cold-water condition with the other conditions, segments from the first three sessions of the task battery were analyzed (i.e., three-session analysis). For comparisons of demographic data, a t-test, chi-square test, and Mann-Whitney U test were used accordingly to see if there were significant demographic differences between male and female subjects.
Results
Demographic characteristics
A total of 41 subjects was recruited, including 22 male (aged 25.7 ± 5.9) and 19 female subjects (aged 22.7 ± 3.3). Among them, a male subject participated in sessions involving the cold-water condition and withdrew from participation in the other conditions. The inter-condition interval was 4.3 ± 9.3 days. There was no missing EDA data, whereas a portion of temperature and HRV data were missing due to sensor malfunctions. For the cognitive task, SPD and CDS had missing data due to software errors. Supplementary Table S2 summarizes the number of available data segments between male and female subjects.
Table 1 shows demographic information between male and female subjects. The male and female subjects did not have detectable significant differences in age, BMI, smoking, and exercise frequency. However, there were significant differences in height, weight, waist circumferences, estimated body fat, and thigh skinfolds. Note that these differences have been commonly observed (Durnin and Womersley, 1974; Flegal et al., 2009; Fryar et al., 2018). Supplementary Table S3; Supplementary Figure S2 shows the distribution of BMI and estimated body fat differences between male and female subjects.
Sex difference of mean body temperature, autonomic functions, and cognitive performance
Tables 2, 3 summarize our statistical analyses of male and female differences in five-session (normal vs. cold-air) and three-session (all conditions) comparisons, respectively. Note that five-session analyses compare normal and cold-air conditions, while three-session analyses compare cold-water condition with other conditions in the result and discussion sections.
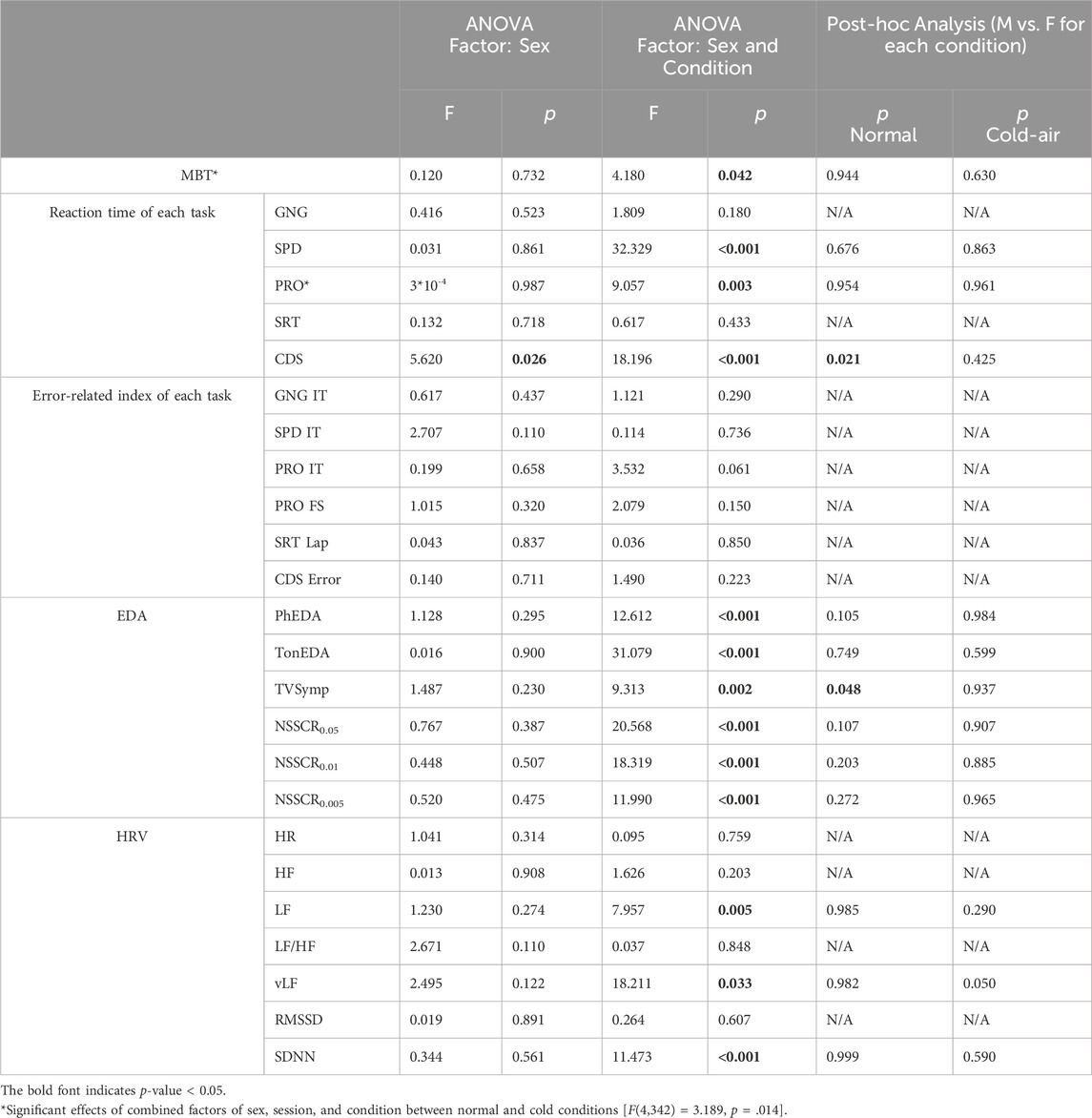
Table 2. Statistical analysis of male and female differences (normal and cold-air conditions, 5 sessions).
Mean body temperature
Both cold-air and cold-water conditions affected physiological changes to both males and females. Both male and female subjects showed significantly higher MBT in the normal condition compared to the cold-air condition (5 sessions, p < .001) (Supplementary Figure S3).
However, no statistical difference in MBT was observed between males and females. Note that core temperature showed no significant effect of sex or combined factors of sex and condition per se, although it was marginally greater in females compared to males in the three-session analysis [F (1,36) = 3.824, p = 0.058].
Cognitive performance
Cognitive performance exhibited distinct patterns between males and females (Figure 2). Females showed significantly slower reaction times in the cold air-condition compared to the normal condition for SPD (p = .002) and for PRO (p < .001), whereas males showed significantly faster reaction times of SPD in the cold-air condition (compared to the normal condition, p < .001).
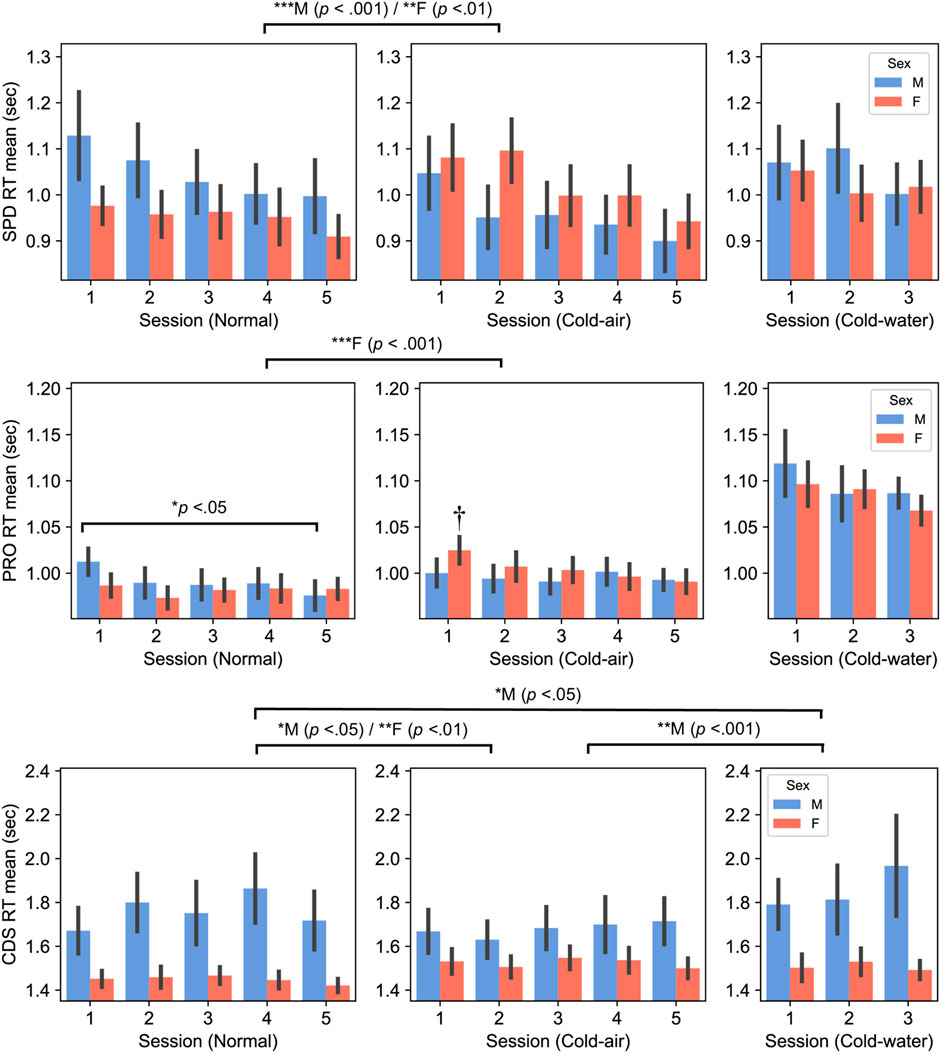
Figure 2. Spatial discrimination (SPD) (top panel), Procedural reaction time (PRO) (middle panel), and Code Substitution (CDS) (bottom panel), RT between males and females (Mean ± SEM). P-values displayed at the top of the figure indicate significant differences between the normal and cold-air/water conditions for each sex. † indicates significant difference with normal 1–5 sessions of female subjects (p < 0.001 for session 2 and p < 0.05 for others).
Interestingly, male subjects’ PRO reaction time significantly decreased in session 5 compared to session 1 (p < .05) in the normal condition, while female subjects showed significantly slower reaction time in the first session in the cold-air condition compared to all the other sessions in the normal condition (p < .05). The former is likely due to a learning effect, despite the inclusion of the practice sessions, and the latter may indicate that cold environment affects performance of females during the initial exposure, while the combination of learning effect and acclimation in the cold environment mitigates the affected performance.
Similarly, CDS reaction time exhibited opposite patterns between males and females. Female subjects exhibited significantly slower reaction time in the cold-air condition compared to the normal condition (five-session analysis, p = .008). Conversely, male subjects showed significantly faster reaction time in the cold-air condition compared to the normal condition (five-session analysis, p = .022). Additionally, male subjects showed a significantly increased reaction time in the cold-water condition compared to the normal (p < .05) and cold-air conditions (p < .001). Nevertheless, female subjects showed significantly faster reaction time than male subjects in normal condition in five-session analysis (p = .021) and in cold-water condition in three-session analysis (p = .039).
Electrodermal activity (EDA)
All EDA indices showed similar patterns between males and females, with decreased amplitudes during cold-air and cold-water conditions compared to the normal condition (Figure 3; Supplementary Figure S4). Both male and female subjects showed significantly higher PhEDA,TonEDA, and TVSymp in the normal condition compared to the water condition (p < .001). Additionally, both male and female subjects showed significantly higher PhEDA and TVSymp in the normal condition compared to the cold condition (p < .001). Both male and female subjects showed significantly lower TonEDA in cold water compared to the other conditions (p < .001). For NSSCR with all thresholds, both male and female subjects showed significantly higher values in the normal condition than in the other conditions (p < .001), and also higher values in the cold-air condition than in the cold-water condition (p < .01).
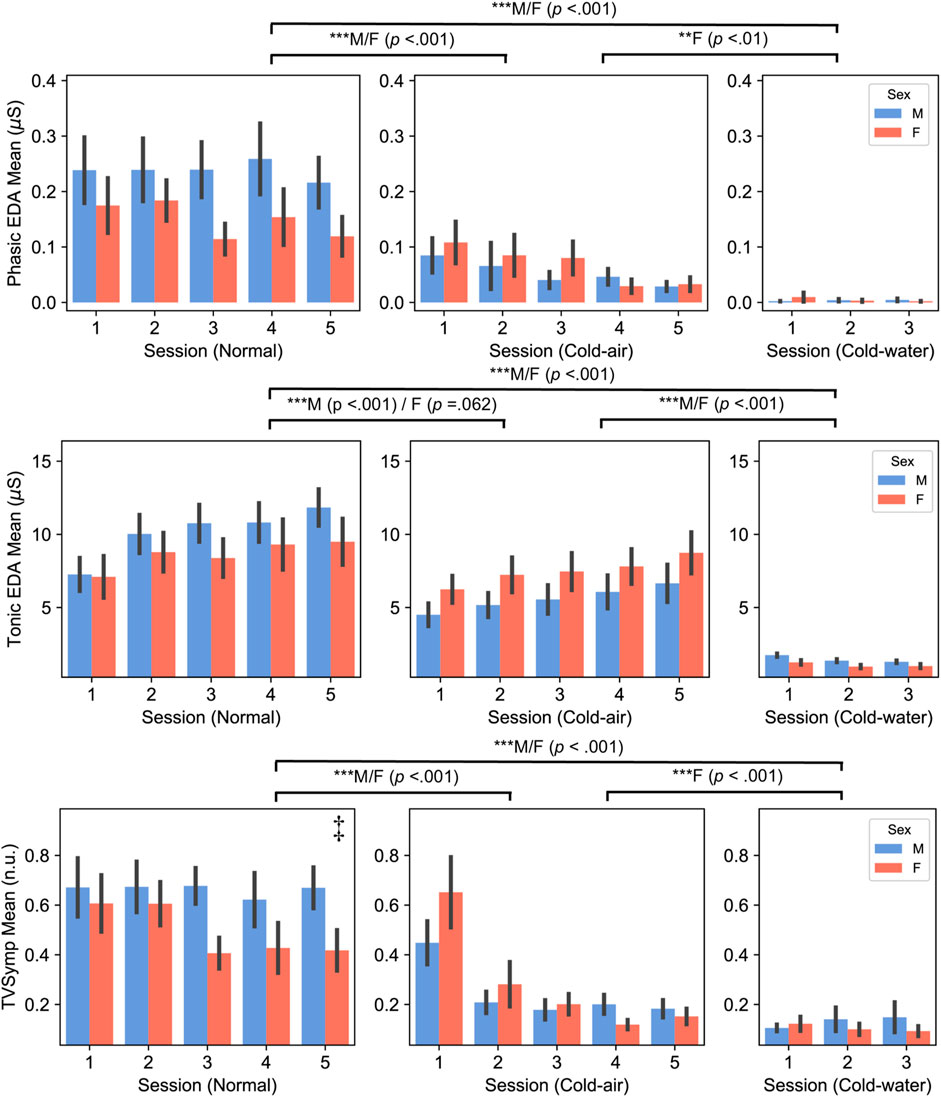
Figure 3. Phasic (top panel) and Tonic (middle panel) components of EDA, and TVSymp (bottom panel) between male and female subjects (Mean ± SEM). P-values displayed at the top of the figure indicate significant differences between the normal and cold-air/water conditions for each sex. ‡ indicates there was significant difference between males and females in the normal condition.
On the other hand, PhEDA, TonEDA, and TVSymp also showed different patterns between males and females. In three-session analysis, only female subjects showed significantly lower PhEDA and TVSymp in the cold-water condition compared to cold-air condition (p < .01), whereas males did not. Additionally, only male subjects showed a significantly lower TonEDA in the cold-air condition compared to the normal condition (p < .001), whereas females did not. Interestingly, a significant difference in TVSymp was found between male and female subjects in the normal condition (five-session analysis, p = .048). Sex did not have a significant effect on the NSSCR features.
Heart rate variability (HRV)
SDNN was significantly higher in the cold-air condition than in the normal condition for both males and females (five-session, p < .01) (Supplementary Figure S5). LF/HF, LF, and vLF showed different patterns between males and females (Figure 4). LF/HF showed higher values in males compared to females [three-session analysis, F (1,38) = 5.370, p = .026]. Additionally, male subjects showed significantly higher LF and vLF in the cold-air condition compared to the normal condition (p < .001). Time-domain HRV indices (RMSSD and SDNN) and HR did not show statistical difference between males and females.
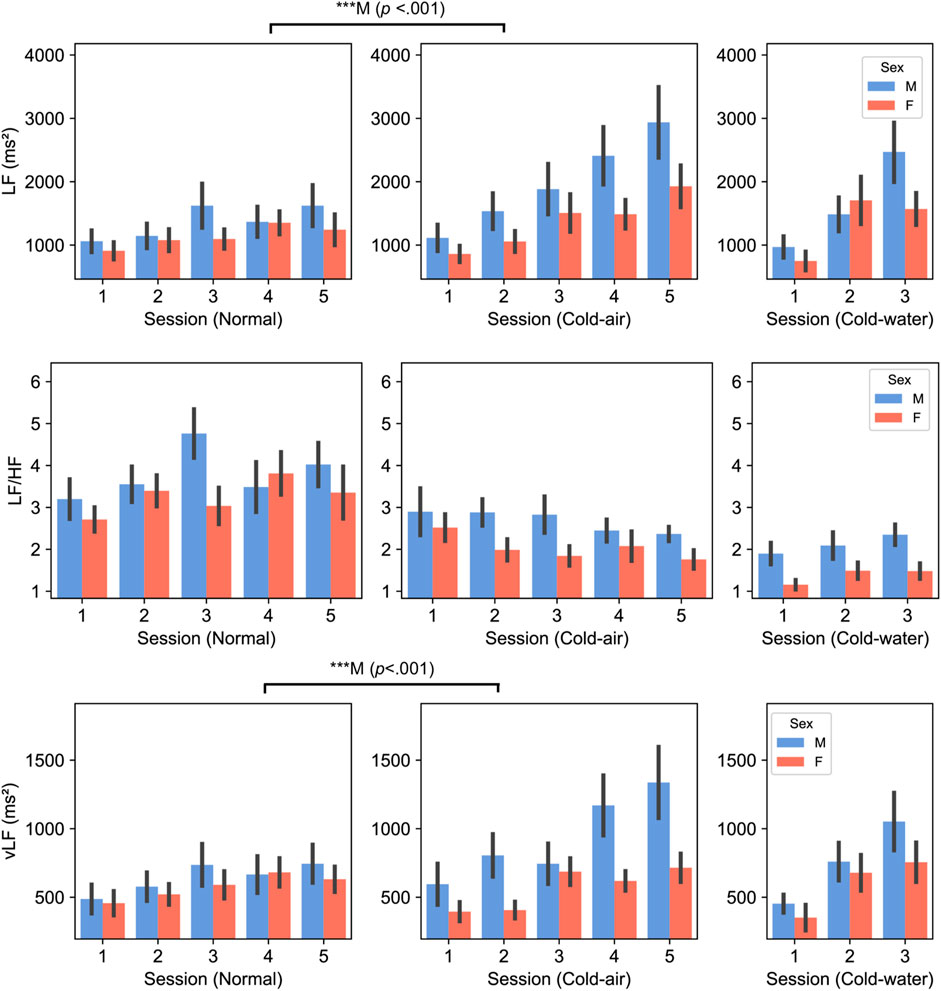
Figure 4. LF (top panel), LF/HF (middle panel), and vLF (bottom panel) between male and female subjects. P-values displayed at the top of the figure indicate significant differences between the normal and cold-air conditions for each sex.
Discussion
We studied the differences between male and female subjects in their cognitive performance and autonomic functions during exposure to three environments, including room-temperature-air/normal, cold-air, and cold-water conditions. Twenty-two male and nineteen female subjects performed a battery of cognitive tasks in these conditions, while HRV and EDA data were collected passively. In our study, subjects were exposed to three different environmental conditions that were pseudo-randomly assigned, based on the availability of the experiential units. However, the comparison between the expected (i.e., uniform) frequency of each permutation of the three conditions and our pseudo-random frequency was not statistically significant (Chi-square, p = .193). In future studies, true randomization should be considered to minimize potential bias.
Regarding cognitive performance, the reaction times of SPD, PRO, and CDS tasks showed differences between normal and cold-air conditions for either or both sexes, while none of the error-related indices did. Interestingly, in the cold-air condition compared to the normal condition, male subjects showed significantly improved SPD and CDS performance while female subjects exhibited significantly deteriorated SPD, PRO, and CDS performance. The reaction time of PRO in female subjects was significantly higher in the first session (i.e., around 10 min of exposures) of cold-air condition compared to all sessions in the normal condition. This may suggest that cold-air exposure female subjects showed deteriorated performance in the beginning period of cold-air exposures, but the combination of learning effect and habituation in the cold-air condition mitigates the affected performance. The reaction time of CDS also showed significantly deteriorated performance for female subjects in the cold-air condition compared to the normal condition. These performance decrements in females are possibly due to females typically feeling less comfortable than males in cold environments (Kaikaew et al., 2018; Maykot et al., 2018). Alternatively, it may be due to a result of cooling of the extremities of the body. Studies showed that females generally experience lower hand blood flows when exposed to cold attributed by increased vascular reactivity (Daanen, 2003). For example, pioneers of this field revealed that finger temperature was significantly warmer in males compared to females during exposure to 0°C cold air for 0–40 min, followed by stabilization between 40 and 75 min of exposures with no significant difference between males and females (Reading et al., 1997). Despite showing decreased performance due to cold environments, female subjects showed faster reaction time than male subjects in memory and attention tasks (i.e., CDS) for the normal and cold-water conditions. Our results are in agreement with other studies which have demonstrated that females outperform males across various short-term memory tasks (Kaushanskaya et al., 2011; Gabriel and Sridevi, 2016; Adyalkar, 2019; Theofilidis et al., 2020). Our findings provide a novel perspective that cold-air exposure may exert an influence on this sex-based disparity.
Our EDA analysis also revealed sex differences in response to cold-air exposures and cold-water immersion. Both male and female subjects showed significantly lower PhEDA and TVSymp in the cold-air and cold-water conditions compared to the normal condition, while only female subjects showed significantly lower values in the cold-water condition than in the cold-air condition. Both male and female subjects showed significantly higher TonEDA in the normal condition compared to the other conditions, while only male subjects showed significantly decreased values in the cold condition compared to the normal condition. The decreased TonEDA in female subjects was marginally significant in the cold air condition compared to the normal condition (p = 0.062). In summary, both males and females showed generally lower values in EDA during the cold-air and cold-water conditions compared to the normal condition, and lower values in the cold-water condition compared to the cold-air conditions. Most EDA indices did not show a significant difference between males and females for any conditions. Bari also found no significant difference in both PhEDA and TonEDA from subjects who underwent sympathetic stimuli (Bari, 2020). Our findings provide further evidence that vasoconstriction in the skin results in decreased openings of sweat glands, subsequently leading to decreased skin conductivity (Edelberg, 1964; Deltombe et al., 1998). Interestingly, TVSymp during the normal condition was significantly higher in male subjects than in female subjects, which has shown higher sensitivity in response to sympathetic stimuli compared to other EDA indices including PhEDA and TonEDA (Posada-Quintero et al., 2016; Kong et al., 2021c). This higher degree of sensitivity capable of differentiating SNS activation between males and females, possibly due to the cognitive tasks, may be diminished by the cold environment.
For HRV analysis, we found differences in the LF/HF ratio, LF, and vLF indices. LF/HF in male subjects was significantly higher than in female subjects across all conditions, which is consistent with other studies (Koenig and Thayer, 2016; Ramalho et al., 2017). This generally indicates a relatively greater cardiac sympathetic tone or reduced cardiac parasympathetic tone in males. Regarding LF and vLF, male subjects showed significant increases in LF and vLF in the cold-air condition compared to the normal condition, while female subjects did not exhibit any differences. Mäkinen et al. also showed significant increases of LF in ten male subjects who underwent cold (10°C) environment exposure for 2 h (Mäkinen et al., 2008). Additionally, Lundell et al. (2020) also showed increased LF and vLF in four male Navy divers who underwent 0°C cold-water dives (Lundell et al., 2020). While we did not find significant effects in female subjects, Matsumoto et al. found a significant increase in LF and vLF in twelve female subjects who underwent control (25°C) or cold-air exposure (10°C) conditions for 15 min (Matsumoto et al., 1999). Interestingly, they reported no significant increase in LF and vLF for another 12 subjects in an obese group. This may be because our group’s BMI is closer to the obese group than the non-obese group (Table 4). Several studies have shown that obesity is associated with reduced SNS activity, which is inversely correlated with reduced brown adipose tissue activity, contributing to impaired thermogenesis in response to cold exposure (Jung et al., 1979; Himms-Hagen, 1989; Nielsen et al., 1993; Matsumoto et al., 1999). It is thought that vLF is closely connected to the thermoregulation mechanism (Fleisher et al., 1996; Thayer et al., 1997). The vLF feature may be a key indicator of the fact that only female subjects showed impaired cold-stressed cognitive performance in our analysis.

Table 4. BMI and Fisher’s Ratio (Ciortan, 2019) of obese and non-obese groups in (Matsumoto et al., 1999).
In addition to our observations of differences in autonomic mechanisms, there are other factors that can influence sex differences in respond to cold exposures. Males tend to have more lean mass, while females tend to have more fat mass in comparison (Bredella, 2017). Our results also showed significantly higher fat percentage in female subjects compared to male subjects. Additionally, males generally accumulate adipose tissue around the trunk and abdomen, whereas females typically accumulate tissue around the hips and thighs (Bredella, 2017), which was shown in our demographic information with thicker thigh skinfolds in female subjects. This difference in adipose tissue distribution may lead to variation in thermoregulation between males and females, as studies found different regional distributions of brown adipose tissue (Heaton, 1972; Leitner et al., 2017). Furthermore, females have a higher proportion of estrogen than males, which can affect their response to cold temperatures (Charkoudian and Stachenfeld, 2016; Herz et al., 2021). For example, increased estrogen augments vasodilation by directly affecting peripheral blood vessels, which increases blood flow to the skin and thus helps maintain warmer skin temperatures in cold environments (Charkoudian and Stachenfeld, 2016). Moreover, some studies showed that estrogen can influence memory and spatial performance (Patel et al., 2022; Joue et al., 2024). In this study, however, we did not consider these factors, including effect of hormones. These should be considered, along with menstruation cycle, in future studies.
We demonstrated that sex differences play an impactful role in autonomic functions and cognitive performance during cold-air exposures and cold-water immersion. However, there was no significant effect of sex and environmental conditions on short-term exposure time, except for one reaction time task. One of our findings is that females may require habituation time (around 30 min) in cold environments for tasks that require fast reaction time, or sufficient training for the task. Another finding is that males may have better reaction time performance in cold-air exposure than in normal conditions. Therefore, different environmental strategies should be provided depending on sex, to achieve optimized performance in reaction time, such as thermal clothing to mitigate different environmental temperatures. Additionally, sex should be considered as one of the main factors when analyzing cognitive performance obtained during cold environment. Individual variations are also important to consider, as response to cold can vary widely depending on a person’s body composition, genetics, and other factors.
Limitations
One limitation of this study is the absence of blood pressure measurements. Blood pressure can provide insights into the effects on arterial and central venous pressure, such as vasoconstriction and hydrostatic pressure. Additionally, we did not account for the potential of baroreflex resetting under different conditions. For instance, a previous study demonstrated that cold exposure can affect baroreflex sensitivity and overall vagal activity (Pikkarainen et al., 2023). Our study did not consider the potential impact of different temperature conditions on baroreflex resetting, which may have affected HRV indices. These should be investigated with blood pressure measurements in future studies. Another limitation is complexity of EDA analysis associated with cold exposure. The increase in skin sympathetic nerve activity during cold exposure is thought to be primarily noradrenergic (Charkoudian, 2010), while EDA is primarily mediated by acetylcholine (Posada-Quintero and Chon, 2020). Muscarinic receptors, which respond to acetylcholine, are mainly associated with the PNS but also play a significant role in stimulating the sweat glands under the control of the SNS (Kudlak and Tadi, 2023). A rat study exhibited decreased acetylcholine responding to short-term cold stress, followed by gradual recovery (Stillman et al., 1997). Similarly, our tonic EDA index showed an increasing trend for both males and females over time in cold-air conditions (Figure 3; Supplementary Table S4). The decreasing trend in Phasic EDA, NSSCR, and TVSymp may be due in a larger part to subjects becoming accustomed to the cold stress and the series of cognitive tasks. (Figure 3; Supplementary Table S4). Interestingly, cold-water condition showed decreased tonic EDA over time, while other metrics increased for males but decreased for females (Figure 3; Supplementary Table S5). This suggests that there may be sex difference in hydrostatic effect to EDA. However, this should be investigated further, as no significant combined effect of exposure time, conditions, and sex was observed in the EDA indices (except for TVSymp in the normal condition). Thus, EDA measured during the early phase of cold exposure may need to have additional considerations made during analysis compared to EDA measured at normal temperatures. EDA might have been affected by the direct impact of cooling on the skin, even though a study showed no correlation between the degree of cooling and the time of onset or intensity of sweating (Glaser and Lee, 1953). However, their study is limited in assessing the effect on the phasic component of EDA and also tested only males. These should be further investigated in future studies. We did not account for the effect of smoking (despite no significant difference between males and females, Chi-square test, p = .284), hydration (at least a cup of water for the core temperature pill), shivering during cold exposures, and thermal perception and comfort. The effects of these factors should be investigated in future studies.
Another significant limitation of this study is the lack of investigation into the menstrual cycle. The menstrual cycle, an integral component of the female reproductive system, involves regular cyclic changes that prepare the body for pregnancy and fertilization, regulated by hormones such as follicle-stimulating hormone, luteinizing hormone, estrogen, and progesterone (Thiyagarajan et al., 2022). One notable physiological effect associated with hormonal fluctuations during the menstrual cycle is the alteration in thermoregulatory responses, with an increased core body temperature during the luteal phase compared to the follicular phase (Baker et al., 2020). Specifically, females in the luteal phase exposed to rapid cold air have a constantly higher core body temperature compared to those in the follicular phase, along with a higher threshold for shivering, sweating, and cutaneous vasodilation (Hessemer and Bruck, 1985; Charkoudian and Johnson, 1999; Baker et al., 2020). Moreover, the menstrual cycle influences cognitive function (Farage et al., 2008; Baker et al., 2020). For instance, researchers have found that performance on simple reaction tasks during the luteal phase is better when core body temperature is higher (Baker et al., 2020). Another notable pattern is that performance on mental rotation tests (spatial ability) peaks during menstruation but is lowest during the mid-luteal phase (Farage et al., 2008). Furthermore, the menstrual cycle affects both EDA (Gómez-Amor et al., 1990) and HRV (Brar et al., 2015). Gómez-Amor et al. showed that females in the ovulatory phase exhibited significantly greater values in both SCR and SCL to auditory stimuli, than did those in menstrual, luteal, and premenstrual phases (Gómez-Amor et al., 1990). For HRV, Brar et al. demonstrated that HRV indices, including LF/HF, LF, and vLF, which showed sex differences in our analysis, were statistically significant across the menstrual cycle (Brar et al., 2015). In their study, LF, vLF, and LF/HF ratio were higher during the luteal phase compared to the follicular phase. As the menstrual cycle can influence thermoregulatory responses, cognitive and autonomic functions, future studies should include female participants across various stages of the menstrual cycle.
Data availability statement
The raw data supporting the conclusions of this article will be made available by the authors, without undue reservation.
Ethics statement
The studies involving humans were approved by the Institutional Review Boards at the University of Connecticut (UConn) and the Naval Submarine Medical Research Laboratory (NSMRL). The studies were conducted in accordance with the local legislation and institutional requirements. The participants provided their written informed consent to participate in this study. No potentially identifiable images or data are presented in this study.
Author contributions
YK: Conceptualization, Data curation, Formal Analysis, Investigation, Methodology, Writing–original draft, Writing–review and editing. MH: Formal Analysis, Writing–review and editing. RM: Investigation, Writing–review and editing. HP-Q: Conceptualization, Writing–review and editing. MD: Investigation, Writing–review and editing. KD: Investigation, Writing–review and editing. KC: Conceptualization, Funding acquisition, Supervision, Writing–review and editing. JB: Conceptualization, Funding acquisition, Supervision, Writing–review and editing.
Funding
The author(s) declare that financial support was received for the research, authorship, and/or publication of this article. This work was supported by Military Operational Medicine Research Program/Joint Program Committee 5 (JPC5), work unit number F1103.
Acknowledgments
During the preparation of this work the author(s) used ChatGPT in order to check grammar and polish the wordings. After using this tool/service, the author(s) reviewed and edited the content as needed and take(s) full responsibility for the content of the publication.
Conflict of interest
Author MH was employed by Analog Devices. Authors MD and KD was employed by Leidos.
The remaining authors declare that the research was conducted in the absence of any commercial or financial relationships that could be construed as a potential conflict of interest.
The author(s) declared that they were an editorial board member of Frontiers, at the time of submission. This had no impact on the peer review process and the final decision.
Publisher’s note
All claims expressed in this article are solely those of the authors and do not necessarily represent those of their affiliated organizations, or those of the publisher, the editors and the reviewers. Any product that may be evaluated in this article, or claim that may be made by its manufacturer, is not guaranteed or endorsed by the publisher.
Author disclaimer
The views expressed in this article reflect the results of research conducted by the authors and do not necessarily reflect the official policy or position of the Department of the Navy, Department of Defense, nor the United States Government. The study protocol was approved by the Institutional Review Boards at the University of Connecticut (UConn) and the Naval Submarine Medical Research Laboratory (NSMRL), in compliance with all applicable Federal regulations governing the protection of human subjects (protocol #NSMRL.2022.0002). One of the authors is an employee of the U.S. Government. This work was prepared as part of their official duties. Title 17, U.S.C., §105 provides that copyright protection under this title is not available for any work of the U.S. Government. Title 17, U.S.C., §101 defines a U.S. Government work as a work prepared by a military Service member or employee of the U.S. Government as part of that person’s official duties. Regarding the use of ScottCare, Cardiac Direct, Equivital, Empatica, Inquisit, and similar products in this article: reference to any specific commercial product, process or service or the use of any trade, firm or corporation name is for the information and convenience of the public, and does not constitute endorsement, recommendation, or favoring by the Department of the Navy.
Supplementary material
The Supplementary Material for this article can be found online at: https://www.frontiersin.org/articles/10.3389/fphys.2024.1463784/full#supplementary-material
References
Adyalkar S. (2019). Relationship between short-term memory and gender of 18-20 years. Int. J. Indian Psychȯlogy 7. doi:10.25215/0703.004
Baker F. C., Siboza F., Fuller A. (2020). Temperature regulation in women: effects of the menstrual cycle. Temperature 7, 226–262. doi:10.1080/23328940.2020.1735927
Bari D. S. (2020). Gender differences in tonic and phasic electrodermal activity components. Sci. J. Univ. Zakho 8, 29–33. doi:10.25271/sjuoz.2020.8.1.670
Bates D., Mächler M., Bolker B., Walker S. (2015). Fitting linear mixed-effects models using lme4. J. Stat. Softw. 67, 1–48. doi:10.18637/jss.v067.i01
Brar T. K., Singh K. D., Kumar A. (2015). Effect of different phases of menstrual cycle on heart rate variability (HRV). J. Clin. diagnostic Res. JCDR 9, CC01–CC04. doi:10.7860/JCDR/2015/13795.6592
Bredella M. A. (2017). Sex differences in body composition. Sex Gend. factors Affect. metabolic Homeost. diabetes Obes. 1043, 9–27. doi:10.1007/978-3-319-70178-3_2
Burton A. C. (1935). The average temperature of the body tissues. J. Nutr. 9, 261–180. doi:10.1093/jn/9.3.261
Campbell I. (2008). Body temperature and its regulation. Anaesth. and Intensive Care Med. 9, 259–263. doi:10.1016/j.mpaic.2008.04.009
Castellani J. W., Young A. J. (2016). Human physiological responses to cold exposure: acute responses and acclimatization to prolonged exposure. Aut. Neurosci. 196, 63–74. doi:10.1016/j.autneu.2016.02.009
Charkoudian N. (2010). Mechanisms and modifiers of reflex induced cutaneous vasodilation and vasoconstriction in humans. J. Appl. Physiology 109, 1221–1228. doi:10.1152/japplphysiol.00298.2010
Charkoudian N., Johnson J. M. (1999). Altered reflex control of cutaneous circulation by female sex steroids is independent of prostaglandins. Am. J. Physiology-Heart Circulatory Physiology 276, H1634–H1640. doi:10.1152/ajpheart.1999.276.5.H1634
Charkoudian N., Stachenfeld N. (2016). Sex hormone effects on autonomic mechanisms of thermoregulation in humans. Aut. Neurosci. 196, 75–80. doi:10.1016/j.autneu.2015.11.004
Ciortan M. (2019). Overview of feature selection methods. Medium. Available at: https://towardsdatascience.com/overview-of-feature-selection-methods-a2d115c7a8f7 (Accessed July 18, 2020).
Daanen H. A. M. (2003). Finger cold-induced vasodilation: a review. Eur. J. Appl. Physiol. 89, 411–426. doi:10.1007/s00421-003-0818-2
De Boeck P., Bakker M., Zwitser R., Nivard M., Hofman A., Tuerlinckx F., et al. (2011). The estimation of item response models with the lmer function from the lme4 package in R. J. Stat. Softw. 39, 1–28. doi:10.18637/jss.v039.i12
Deltombe T., Hanson P., Jamart J., Clérin M. (1998). The influence of skin temperature on latency and amplitude of the sympathetic skin response in normal subjects. Muscle and Nerve Official J. Am. Assoc. Electrodiagn. Med. 21, 34–39. doi:10.1002/(sici)1097-4598(199801)21:1<34::aid-mus5>3.0.co;2-h
Durnin J. V., Womersley J. (1974). Body fat assessed from total body density and its estimation from skinfold thickness: measurements on 481 men and women aged from 16 to 72 years. Br. J. Nutr. 32, 77–97. doi:10.1079/bjn19740060
Edelberg R. (1964). Effect of vasoconstriction on galvanic skin response amplitude. J. Appl. physiology 19, 427–430. doi:10.1152/jappl.1964.19.3.427
Falla M., Micarelli A., Hüfner K., Strapazzon G. (2021). The effect of cold exposure on cognitive performance in healthy adults: a systematic review. Int. J. Environ. Res. Public Health 18, 9725. doi:10.3390/ijerph18189725
Farage M. A., Osborn T. W., MacLean A. B. (2008). Cognitive, sensory, and emotional changes associated with the menstrual cycle: a review. Archives Gynecol. obstetrics 278, 299–307. doi:10.1007/s00404-008-0708-2
Flegal K. M., Shepherd J. A., Looker A. C., Graubard B. I., Borrud L. G., Ogden C. L., et al. (2009). Comparisons of percentage body fat, body mass index, waist circumference, and waist-stature ratio in adults. Am. J. Clin. Nutr. 89, 500–508. doi:10.3945/ajcn.2008.26847
Fleisher L. A., Frank S. M., Sessler D. I., Cheng C., Matsukawa T., Vannier C. A. (1996). Thermoregulation and heart rate variability. Clin. Sci. 90, 97–103. doi:10.1042/cs0900097
Fryar C. D., Kruszan-Moran D., Gu Q., Ogden C. L. (2018). Mean body weight, weight, waist circumference, and body mass index among adults: United States, 1999–2000 through 2015–2016.
Gabriel S., Sridevi G. (2016). Gender differences in short term memory and perception. Int. J. Dev. Res. 06 (7), 8478–8480.
Ghiasi S., Greco A., Barbieri R., Scilingo E. P., Valenza G. (2020). Assessing autonomic function from electrodermal activity and heart rate variability during cold-pressor test and emotional challenge. Sci. Rep. 10, 5406. doi:10.1038/s41598-020-62225-2
Glaser E. M., Lee T. S. (1953). Activity of human sweat glands during exposure to cold. J. Physiology 122, 59–65. doi:10.1113/jphysiol.1953.sp004978
Gómez-Amor J., Martínez-Selva J., Román F., Zamora S. (1990). Electrodermal activity in menstrual cycle phases: a comparison of within-and between-subjects designs. Int. J. Psychophysiol. 9, 39–47. doi:10.1016/0167-8760(90)90006-y
Greco A., Valenza G., Lanata A., Scilingo E. P., Citi L. (2015). cvxEDA: a convex optimization approach to electrodermal activity processing. IEEE Trans. Biomed. Eng. 63, 797–804. doi:10.1109/TBME.2015.2474131
Han D., Bashar S. K., Lázaro J., Mohagheghian F., Peitzsch A., Nishita N., et al. (2022). A real-time ppg peak detection method for accurate determination of heart rate during sinus rhythm and cardiac arrhythmia. Biosensors 12, 82. doi:10.3390/bios12020082
Haran F. J., Lovelace A., Florian J. (2015). Reliability assessment of the Defense automated neurobehavioral assessment (dana) in extreme operational environments. Available at: https://apps.dtic.mil/sti/tr/pdf/AD1003616.pdf.
Harinath K., Malhotra A. S., Pal K., Prasad R., Kumar R., Sawhney R. C. (2005). Autonomic nervous system and adrenal response to cold in man at Antarctica. Wilderness and Environ. Med. 16, 81–91. doi:10.1580/pr30-04.1
Herz C. T., Kulterer O. C., Prager M., Marculescu R., Langer F. B., Prager G., et al. (2021). Sex differences in brown adipose tissue activity and cold-induced thermogenesis. Mol. Cell. Endocrinol. 534, 111365. doi:10.1016/j.mce.2021.111365
Hessemer V., Bruck K. (1985). Influence of menstrual cycle on shivering, skin blood flow, and sweating responses measured at night. J. Appl. Physiology 59, 1902–1910. doi:10.1152/jappl.1985.59.6.1902
Himms-Hagen J. (1989). Brown adipose tissue thermogenesis and obesity. Prog. lipid Res. 28, 67–115. doi:10.1016/0163-7827(89)90009-x
Hossain M. B., Bashar S. K., Walkey A. J., McManus D. D., Chon K. H. (2019). An accurate QRS complex and P wave detection in ECG signals using complete ensemble empirical mode decomposition with adaptive noise approach. IEEE Access 7, 128869–128880. doi:10.1109/access.2019.2939943
ISO 9886:2004 (en) (2004). Ergonomics — evaluation of thermal strain by physiological measurements. Available at: https://www.iso.org/obp/ui/#iso:std:iso:9886:ed-2:v1:en (Accessed March 5, 2023).
Jackson A. S., Pollock M. L. (1978). Generalized equations for predicting body density of men. Br. J. Nutr. 40, 497–504. doi:10.1079/bjn19780152
Jackson A. S., Pollock M. L. (1985). Practical assessment of body composition. Physician Sportsmed. 13, 76–90. doi:10.1080/00913847.1985.11708790
Jackson A. S., Pollock M. L., Ward A. N. N. (1980). Generalized equations for predicting body density of women. Med. Sci. sports Exerc. 12, 175–182. doi:10.1249/00005768-198023000-00009
Jimenez C., Regnard J., Robinet C., Mourot L., Gomez-Merino D., Chennaoui M., et al. (2010). Whole body immersion and hydromineral homeostasis: effect of water temperature. Eur. J. Appl. Physiol. 108, 49–58. doi:10.1007/s00421-009-1187-2
Joue G., Navarro-Schröder T., Achtzehn J., Moffat S., Hennies N., Fuß J., et al. (2024). Effects of estrogen on spatial navigation and memory. Psychopharmacology 241, 1037–1063. doi:10.1007/s00213-024-06539-3
Jung R. T., Shetty P. S., James W. P. T., Barrand M. A., Callingham B. A. (1979). Reduced thermogenesis in obesity. Nature 279, 322–323. doi:10.1038/279322a0
Kaciuba-Uscilko H., Grucza R. (2001). Gender differences in thermoregulation. Curr. Opin. Clin. Nutr. and Metabolic Care 4, 533–536. doi:10.1097/00075197-200111000-00012
Kaikaew K., van den Beukel J. C., Neggers S. J., Themmen A. P., Visser J. A., Grefhorst A. (2018). Sex difference in cold perception and shivering onset upon gradual cold exposure. J. Therm. Biol. 77, 137–144. doi:10.1016/j.jtherbio.2018.08.016
Kaushanskaya M., Marian V., Yoo J. (2011). Gender differences in adult word learning. Acta Psychol. 137, 24–35. doi:10.1016/j.actpsy.2011.02.002
Koenig J., Thayer J. F. (2016). Sex differences in healthy human heart rate variability: a meta-analysis. Neurosci. and Biobehav. Rev. 64, 288–310. doi:10.1016/j.neubiorev.2016.03.007
Kong Y., Posada-Quintero H. F., Chon K. H. (2021a). “Female–male differences should be considered in physical pain quantification based on electrodermal activity: preliminary study,” in 2021 43rd annual international conference of the IEEE engineering in medicine and biology society (EMBC) (IEEE), 6941–6944.
Kong Y., Posada-Quintero H. F., Chon K. H. (2021b). Real-time high-level acute pain detection using a smartphone and a wrist-worn electrodermal activity sensor. Sensors 21, 3956. doi:10.3390/s21123956
Kong Y., Posada-Quintero H. F., Chon K. H. (2021c). Sensitive physiological indices of pain based on differential characteristics of electrodermal activity. IEEE Trans. Biomed. Eng. 68, 3122–3130. doi:10.1109/TBME.2021.3065218
Kong Y., Posada-Quintero H. F., Tran H., Talati A., Acquista T. J., Chen I.-P., et al. (2023). Differentiating between stress-and EPT-induced electrodermal activity during dental examination. Comput. Biol. Med. 155, 106695. doi:10.1016/j.compbiomed.2023.106695
Kudlak M., Tadi P. (2023). “Physiology, muscarinic receptor,” in StatPearls (Treasure Island, FL: StatPearls Publishing). Available at: https://www.ncbi.nlm.nih.gov/books/NBK555909/(Accessed September 1, 2024).
Lathan C., Spira J. L., Bleiberg J., Vice J., Tsao J. W. (2013). Defense automated neurobehavioral assessment (DANA)—psychometric properties of a new field-deployable neurocognitive assessment tool. Mil. Med. 178, 365–371. doi:10.7205/MILMED-D-12-00438
Lee P., Swarbrick M. M., Ho K. K. (2013). Brown adipose tissue in adult humans: a metabolic renaissance. Endocr. Rev. 34, 413–438. doi:10.1210/er.2012-1081
Leitner B. P., Huang S., Brychta R. J., Duckworth C. J., Baskin A. S., McGehee S., et al. (2017). Mapping of human brown adipose tissue in lean and obese young men. Proc. Natl. Acad. Sci. U.S.A. 114, 8649–8654. doi:10.1073/pnas.1705287114
Lenhardt R., Sessler D. I. (2006). Estimation of mean body temperature from mean skin and core temperature. J. Am. Soc. Anesthesiol. 105, 1117–1121. doi:10.1097/00000542-200612000-00011
Lundell R. V., Räisänen-Sokolowski A. K., Wuorimaa T. K., Ojanen T., Parkkola K. I. (2020). Diving in the arctic: cold water immersion’s effects on heart rate variability in navy divers. Front. physiology 10, 1600. doi:10.3389/fphys.2019.01600
Mäkinen T. M., Mäntysaari M., Pääkkönen T., Jokelainen J., Palinkas L. A., Hassi J., et al. (2008). Autonomic nervous function during whole-body cold exposure before and after cold acclimation. Aviat. space, Environ. Med. 79, 875–882. doi:10.3357/asem.2235.2008
Matsumoto T., Miyawaki T., Ue H., Kanda T., Zenji C., Moritani T. (1999). Autonomic responsiveness to acute cold exposure in obese and non-obese young women. Int. J. Obes. 23, 793–800. doi:10.1038/sj.ijo.0800928
Maykot J. K., Rupp R. F., Ghisi E. (2018). A field study about gender and thermal comfort temperatures in office buildings. Energy Build. 178, 254–264. doi:10.1016/j.enbuild.2018.08.033
Mcmullen R. L., Gillece T., Lu G., Laura D., Chen S. (2013). Influence of various environmental parameters on sweat gland activity. J. Cosmet. Sci. 64, 243–260.
Mengel L. A., Seidl H., Brandl B., Skurk T., Holzapfel C., Stecher L., et al. (2020). Gender differences in the response to short-term cold exposure in young adults. J. Clin. Endocrinol. and Metabolism 105, dgaa110–e1948. doi:10.1210/clinem/dgaa110
Nielsen B., Astrup A., Samuelsen P., Wengholt H., Christensen N. J. (1993). Effect of physical training on thermogenic responses to cold and ephedrine in obesity. Int. J. Obes. Relat. Metabolic Disord. J. Int. Assoc. Study Obes. 17, 383–390.
Patel S. A., Frick K. M., Newhouse P. A., Astur R. S. (2022). Estradiol effects on spatial memory in women. Behav. Brain Res. 417, 113592. doi:10.1016/j.bbr.2021.113592
Pikkarainen K., Valtonen R. I., Hintsala H. E., Kiviniemi A., Crandall C. G., Perkiömäki J., et al. (2023). Baroreflex sensitivity following acute upper-body exercise in the cold among stable coronary artery disease patients. Front. Physiology 14, 1184378. doi:10.3389/fphys.2023.1184378
Pilcher J. J., Nadler E., Busch C. (2002). Effects of hot and cold temperature exposure on performance: a meta-analytic review. Ergonomics 45, 682–698. doi:10.1080/00140130210158419
Posada-Quintero H. F., Bolkhovsky J. B. (2019). Machine learning models for the identification of cognitive tasks using autonomic reactions from heart rate variability and electrodermal activity. Behav. Sci. 9, 45. doi:10.3390/bs9040045
Posada-Quintero H. F., Chon K. H. (2020). Innovations in electrodermal activity data collection and signal processing: a systematic review. Sensors 20, 479. doi:10.3390/s20020479
Posada-Quintero H. F., Florian J. P., Orjuela-Cañón Á. D., Chon K. H. (2016). Highly sensitive index of sympathetic activity based on time-frequency spectral analysis of electrodermal activity. Am. J. Physiology-Regulatory, Integr. Comp. Physiology 311, R582–R591. doi:10.1152/ajpregu.00180.2016
Posada-Quintero H. F., Kong Y., Nguyen K., Tran C., Beardslee L., Chen L., et al. (2020). Using electrodermal activity to validate multilevel pain stimulation in healthy volunteers evoked by thermal grills. Am. J. Physiology-Regulatory, Integr. Comp. Physiology 319, R366-R375–R375. doi:10.1152/ajpregu.00102.2020
Ramalho E. S., Souza-Junior E. L., Magnani M., Braga V. A. (2017). Gender differences in heart rate variability among individuals undergoing regular resistance training preliminary observations. Sultan Qaboos Univ. Med. J. 17, e209–e212. doi:10.18295/squmj.2016.17.02.012
Reading J. E., Roberts D. E., Prusaczyk W. K. (1997). Gender differences in finger temperatures during cold air exposure. Fort Belvoir, VA: Defense Technical Information Center. doi:10.21236/ADA359127
Reyes B. A., Posada-Quintero H. F., Bales J. R., Clement A. L., Pins G. D., Swiston A., et al. (2014). Novel electrodes for underwater ECG monitoring. IEEE Trans. Biomed. Eng. 61, 1863–1876. doi:10.1109/TBME.2014.2309293
Shaffer F., Ginsberg J. P. (2017). An overview of heart rate variability metrics and norms. Front. public health 258, 258. doi:10.3389/fpubh.2017.00258
Shields S. A., MacDowell K. A., Fairchild S. B., Campbell M. L. (1987). Is mediation of sweating cholinergic, adrenergic, or both? A comment on the literature. Psychophysiology 24, 312–319. doi:10.1111/j.1469-8986.1987.tb00301.x
Siri W. E. (1956). In advances in biological and medical physics. London and New York: Academic press Inc. 4, 239–280.
Stillman M. J., Shukitt-Hale B., Coffey B. P., Levy A., Lieberman H. R. (1997). In vivo hippocampal acetylcholine release during exposure to acute stress. Stress 1, 191–200. doi:10.3109/10253899709013740
Thayer J. F., Nabors-Oberg R., Sollers J. J. (1997). Thermoregulation and cardiac variability: a time-frequency analysis. Biomed. Sci. Instrum. 34, 252–256.
Theofilidis A., Karakasi M.-V., Kevrekidis D.-P., Pavlidis P., Sofologi M., Trypsiannis G., et al. (2020). Gender differences in short-term memory related to music genres. Neuroscience 448, 266–271. doi:10.1016/j.neuroscience.2020.08.035
Thiyagarajan D. K., Basit H., Jeanmonod R. (2022). “Physiology, menstrual cycle,” in StatPearls (Treasure Island, FL: StatPearls Publishing). Available at: https://www.ncbi.nlm.nih.gov/books/NBK500020/(Accessed September 29, 2024).
Tikuisis P., Jacobs I., Moroz D., Vallerand A. L., Martineau L. (2000). Comparison of thermoregulatory responses between men and women immersed in cold water. J. Appl. Physiology 89, 1403–1411. doi:10.1152/jappl.2000.89.4.1403
Tikuisis P., Keefe A. (1996). Prediction of sea survival time. North York, ON: Defence and Civil Institute of Environmental Medicine.
Torres M. E., Colominas M. A., Schlotthauer G., Flandrin P. (2011). “A complete ensemble empirical mode decomposition with adaptive noise,” in 2011 IEEE international conference on acoustics, speech and signal processing (ICASSP) (IEEE), 4144–4147.
Tran H., Kong Y., Talati A., Posada-Quintero H., Chon K., Chen I.-P. (2022). The use of electrodermal activity in pulpal diagnosis and dental pain assessment. Int. Endod. J. 56, 356–368. doi:10.1111/iej.13868
Travers G. J. S., Nichols D. S., Farooq A., Racinais S., Périard J. D. (2016). Validation of an ingestible temperature data logging and telemetry system during exercise in the heat. Temp. (Austin) 3, 208–219. doi:10.1080/23328940.2016.1171281
Visnovcova Z., Calkovska A., Tonhajzerova I. (2013). Heart rate variability and electrodermal activity as noninvasive indices of sympathovagal balance in response to stress. Acta Medica Martiniana 13, 5–13. doi:10.2478/acm-2013-0006
Keywords: sex differences, cold, autonomic functions, cognitive performance, electrodermal activity, heart rate variability, memory, reaction time
Citation: Kong Y, Hossain MB, McNaboe R, Posada-Quintero HF, Daley M, Diaz K, Chon KH and Bolkhovsky J (2024) Sex differences in autonomic functions and cognitive performance during cold-air exposure and cold-water partial immersion. Front. Physiol. 15:1463784. doi: 10.3389/fphys.2024.1463784
Received: 12 July 2024; Accepted: 01 October 2024;
Published: 16 October 2024.
Edited by:
Pieter-Jan A. M. Van Ooij, Royal Netherlands Navy, NetherlandsReviewed by:
Thad E. Wilson, University of Kentucky, United StatesAdam W Potter, US Army Research Institute of Environmental Medicine (USARIEM), United States
Copyright © 2024 Kong, Hossain, McNaboe, Posada-Quintero, Daley, Diaz, Chon and Bolkhovsky. This is an open-access article distributed under the terms of the Creative Commons Attribution License (CC BY). The use, distribution or reproduction in other forums is permitted, provided the original author(s) and the copyright owner(s) are credited and that the original publication in this journal is cited, in accordance with accepted academic practice. No use, distribution or reproduction is permitted which does not comply with these terms.
*Correspondence: Youngsun Kong, eW91bmdzdW4ua29uZ0B1Y29ubi5lZHU=