- 1Department of Orthodontics, Peking University School and Hospital of Stomatology, National Center for Stomatology, National Clinical Research Center for Oral Diseases, National Engineering Research Center of Oral Biomaterials and Digital Medical Devices, Beijing Key Laboratory of Digital Stomatology, NHC Key Laboratory of Digital Stomatology, NMPA Key Laboratory for Dental Materials, Beijing, China
- 2National Center of Stomatology, National Clinical Research Center for Oral Diseases, National Engineering Laboratory for Digital and Material Technology of Stomatology, Beijing Key Laboratory for Digital Stomatology, Research Center of Engineering and Technology for Computerized Dentistry Ministry of Health, NMPA Key Laboratory for Dental Materials, Beijing, China
- 3Central laboratory, Peking University School and Hospital of Stomatology, Beijing, China
Orthodontic treatment in adults is often related to longer treatment time as well as higher periodontal risks compared to adolescents. The aim of this review is to explore the influence of age-related chages on orthodontic tooth movement (OTM) from macro and micro perspectives. Adults tend to show slower tooth movement speed compared to adolescence, especially during the early phase. Under orthodontic forces, the biological responses of the periodontal ligament (PDL) and alveolar bone is different between adult and adolescents. The adult PDL shows extended disorganization time, increased cell senescence, less cell signaling and a more inflammatory microenvironment than the adolescent PDL. In addition, the blood vessel surface area is reduced during the late movement phase, and fiber elasticity decreases. At the same time, adult alveolar bone shows a higher density, as well as a reduced osteoblast and osteoclast activation, under orthodontic forces. The local cytokine expression also differs between adults and adolescents. Side-effects, such as excessive root resorption, greater orthodontic pain, and reduced pulpal blood flow, also occur more frequently in adults than in adolescents.
1 Introduction
Malocclusion is the deviation from normal or ideal occlusion, which can affect oral health, function, and facial aesthetics of patients. Malocclusion occurs in all populations; its prevalence among children and adolescents ranges from 48% to 83.5% (Lombardo et al., 2020; Yin et al., 2023), and 45.6%–68% in the adult population (Jonsson et al., 2007; Claudino and Traebert, 2013; Asiri et al., 2019). With the recent focus on quality of life and the increased emphasis on appearance, adults pay more attention to having an “ideal” appearance. Therefore, the demand for orthodontic treatment in adults has been substantially increasing.
Clinically, orthodontic treatment in adults is often related to longer treatment times, as well as higher periodontal risks than in adolescents, which may hinder the application of orthodontic treatment in adults (Ren et al., 2003; Alikhani et al., 2018). This is related to the maturation and aging process. As the human body experience aging, there is a time-dependent decline in function, which is the result of the cumulative effect of various molecular and cellular damages at the microscopic level (Li et al., 2016; Calcinotto et al., 2019; Mohanakumar et al., 2021). Thus, tissue properties and biological responses are altered. As a biological process, orthodontic tooth movement (OTM) is also impacted by age-related changes. During orthodontic treatment, the tooth moves within the alveolar bone under mechanical forces. This process is essentially a localized aseptic inflammatory response in the periodontal ligament (PDL) and alveolar bone, mediated by mechanical forces, which results in bone resorption on the pressure side and bone deposition on the tension side (Li et al., 2021). The senescence related changes of the tooth, surrounding periodontium and the alveolar bone may all contribute to the age-related changes of OTM (Figure 1).
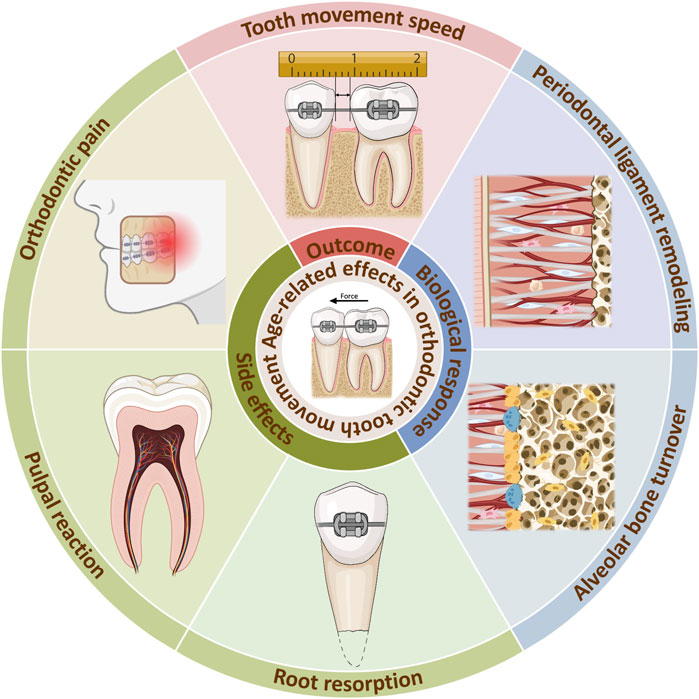
Figure 1. Age-related effects in orthodontic tooth movement. Age-related changes occurs during orthodontic treatment, reducing tooth movement speed. The internal reason can be explained by alterations of biological responses in the PDL and alveolar bone. Meanwhile, side effects such as orthodontic pain, pulpal reaction, root resorption can be aggrevated. Created with BioRender.com.
This review focuses on the difference in biological reactions to orthodontic treatment between adults and adolescents. With a comprehensive understanding of the age-related biologic and mechanistic changes in the OTM process, key regulatory targets may be identified. This can improve the clinical outcomes of orthodontic treatment in adults and provide insight on future research directions. Hence, the aim of this review is to explore the influence of age-related changes on OTM from macro and micro perspectives, as well as the involved inner mechanisms.
2 Reduced tooth movement speed in adults during orthodontic treatment
The movement speed is an important factor in OTM as it determines the course of the treatment. Many researchers have discovered that tooth movement speed in adult human is slower than that in adolescents. For instance, during the maxillary canine distalization, after 7 days of traction, a longer movement distance of canines was found for adolescents than for adults (Kawasaki et al., 2006). The faster movement of canines in adolescents continues even up to 56 days of canine distalization, especially during the 28–56 days period (Alikhani et al., 2018). The same result was found in animal models. Molar movement distance in old rats was significantly smaller than that observed in young rats over a total of 12 weeks. This tooth movement speed appears to be significantly higher in young rats than in older rats during the early phase (0–3 weeks), but gradually becomes similar during the late phase (4–12 weeks) (Ren et al., 2003). Similar results of tooth movement being more rapid in adolescents than in adults were confirmed by many in both human and animals (Kyomen and Tanne, 1997; Von Böhl et al., 2016). However, Li’s study found a tendency for decreasing molar movement distance in old (18–20 weeks) rats compared to young (4–5 weeks) rats, after applying 7 days of orthodontic force; however, no significant difference was found (Li et al., 2016). This may be due to the minimal age gap between the two groups or the short duration of the experiment.
When teeth are subjected to orthodontic forces, the physiological process of their movement can be divided into four phases according to speed: initial phase, lag phase, acceleration phase, and linear phase (Pilon et al., 1996). In the initial phase, the tooth undergoes an instantaneous movement in its socket, pressuring the PDL. Therefore, its movement distance is limited by the compression and viscoelastic properties of the PDL. Subsequently, OTM enters the lag phase, in which its speed decelerates and tooth movement arrests. This could be attributed to the hyalinization of the PDL and blockage of the alveolar bone. Under compressive force, phagocytes and osteoclasts locally accumulated, removing hyalinized areas and initiating PDL turnover and bone remodeling. As accumulation and activity of these cells increase, the tooth movement speed elevates, leading to the acceleration phase. When local cellular activities reach homeostasis, PDL turnover and bone remodeling achieve maximum capacity, resulting in constant tooth movement speed as in the linear phase.
As described above, a significant faster tooth movement velocity occurred in young rats only during the early phase of OTM (Ren et al., 2003), suggesting that the biological difference between adolescences and adults takes place at the early phase. This may be related to the speed of the initial response of the PDL and alveolar bone cells.
3 Reduced regenerative capacity and enhanced inflammatory response in aged PDL during orthodontic treatment
As the connective tissue between teeth and alveolar bone, the PDL is composed of a heterogeneous cell population, including fibroblastic lineages, osteoblastic lineages, multipotent stem cells etc. as well as fibrous structures and blood vessels (Nanci and Bosshardt, 2006). The PDL plays a crucial role in OTM with the ability of mechanotransduction, cellular signaling, self-renewal and direct or indirect regulation of periodontal tissue remodeling. Various components and their function experience noticeable changes with aging (Figure 2) (Li et al., 2021). Cell proliferation ability, migratory potential and differentiation capacity declines as age development occurs (Lim et al., 2014; Chen et al., 2012). Meanwhile, cytokine expression is also altered in adults (Table 1) (Wu et al., 2015). Other components, including blood vessels and collagen content, undergo degenerative changes influenced by aging (Sims et al., 1996; Lim et al., 2014).
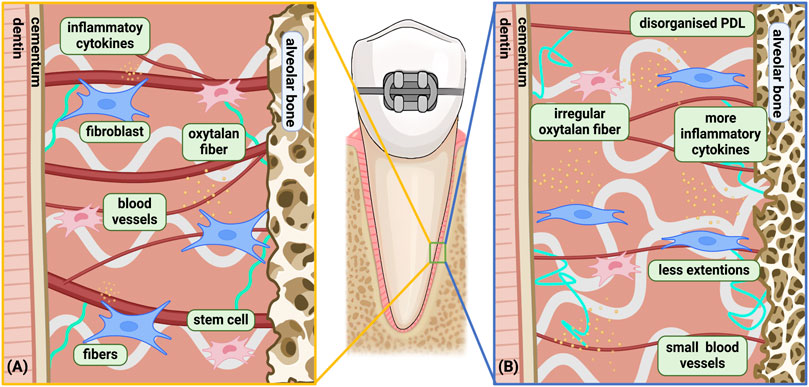
Figure 2. Age-related changes in the biological response of PDL under orthodontic force. (A) Young PDLC under orthodontic force; (B) aged PDLC under orthodontic force. Differences between the Young and Aged PDLC was shown: a) Slower biological reaction and recovery with increased disorganization time; b) Cell senescence; c) More inflammatory microenvironment; d) Fewer extensions in cells leading to deteriorated signaling activity; e) More complex and irregular oxytalan fibers with loss of elasticity; f) Reduced blood vessel surface area during late phase, with solely proliferation of small blood vessels instead of large blood vessel. Among the above differences, a only occurs on the compression side, while b, c, d, e, f occurs on both the compression side and the tension side. Created with BioRender.com.
3.1 Age-related changes in periodontal ligament cells (PDLCs)
Age-related changes in the PDL have also been observed under orthodontic forces. When tooth movement occurs, surrounding PDLCs are compressed on the pressure side, forming degenerated tissue while they extended on the tension side (Shimpo et al., 2003). From a phenotypic perspective, mouse PDL showed little difference between aged and young rats on the tension side, with abundant collagen fibers, PDLCs and osteoblasts (Ren et al., 2008c). However, a slower biological reaction and recovery were found in the PDL of aged rats on the pressure side. While young rats experienced PDL disorganization after 1–2 weeks of force application and rearranged at 8–12 weeks, the disorganized status of the PDL extended to 12 weeks in aged rats, as recovery was not observed within the 12-week trial (Ren et al., 2008c). This corresponds with higher proliferative activity of PDLCs in young rats than in adult rats during the first week of OTM on both the pressure side and tension side (Kyomen and Tanne, 1997). The reduction in physiological response and reconstruction capability of the PDL with age corresponds with the decreased movement speed.
Furthermore, adult human PDLCs (hPDLCs) exhibited increased senescence with higher beta-galactosidase activity as well as lower α-Klotho expression (Mohanakumar et al., 2021). Senescence-associated beta-galactosidase (SA-β-GAL) serves as a commonly used biomarker to detect and quantify the aging status of cells, with its activity significantly increases in senescent cells. α-Klotho is the co-receptor for Fibroblast Growth Factor-23 (FGF23) and is an important anti-aging protein associated with phosphate regulation, vitamin D metabolism, oxidative stress reduction and insulin signaling. During orthodontic movement, both adult and young hPDLCs showed decreased SA-β-GAL level and increased α-Klotho expression. However, adult hPDLCs still had higher SA-β-GAL level and lower α-Klotho expression compared with young hPDLCs(Mohanakumar et al., 2021). Furthermore, the SA-β-GAL level in adult PDLCs ascended before descending, with the peak at day 7, while it exhibited a straightforward decrease in young hPDLCs (George et al., 2020). Therefore, adult hPDLCs still exhibit higher levels of senescence and lower anti-aging protein expression compared to younger cells, even during orthodontic movement.
Inflammatory changes also take place in adult hPDLCs, with a significant increase in PGE2 and IL-1β mRNA expression level higher than young hPDLCs during the first month of OTM (Mayahara et al., 2007; George et al., 2020). PGE2 is a critical inflammatory cytokine and osteoclastogenic mediator during the OTM process, which is expressed in hPDLCs under mechanotransduction and related with osteoclastogenesis and regulation of osteo-related cytokines (Kang et al., 2010; Ullrich et al., 2019). IL-1β is also associated with acute inflammation and bone resorption during tooth movement (Alhashimi et al., 2001; Kapoor et al., 2014). These differences suggest a stronger inflammatory microenvironment under mechanical stress in adult PDL than in young PDL, along with more catabolic changes. Subsequently, relating changes occurred in PGE2 and IL-1β concentration in the gingival cervical fluid (GCF) of adult and young patients. PGE2 concentration experiences a significant increase followed by a decline with the peak at 21 days of OTM in young patients, while it maintains a high concentration in adult patients (Ren et al., 2002; Chibebe et al., 2010). Additionally, GCF PGE2 concentration of young patients is lower than adult patients at all times except at peak, demonstrating a more inflammatory periodontal microenvironment in adults at all time. Likewise, IL-1β concentration is significantly higher in adult human GCF after 7 days of OTM(Alikhani et al., 2018). PGE2 can be synthesized by COX-2, a prostaglandin endoperoxidase, which is stimulated under mechanical stress and a critical component of the orthodontic movement process (Mitsui et al., 2005; de Carlos et al., 2006). A rapid increase in COX-2 mRNA expression and cell expression ratio after 48 h of compressive force has also been found in adult hPDLCs compared with young hPDLCs(Mayahara et al., 2007). IL-6 and GM-CSF are also important inflammatory mediators involved in the orthodontic process (Bakker et al., 2014; Nunes et al., 2017; Feng et al., 2022). Similarly, IL-6 and GM-CSF concentrations were higher in adult human GCF than adolescents during OTM (Ren et al., 2002). Adult hPDLCs experience more pronounced inflammatory responses and catabolic changes, suggesting a more inflammatory environment in adult PDL under mechanical stress.
3.2 Age-related changes in periodontal fibers and blood vessels
Age-related changes also take place in the extracellular matrix, including fibers and the vascularis. Oxytalan fibers is an important component of the PDL, as a kind of elastic fiber, it is composed of microfibrils and can provide tissue resilience and flexibility. Oxytalan fibers are suggested to support and regulate the vascular system, guide fibroblast migration and contribute to the mechanical properties of the PDL (Strydom et al., 2012). Under tension strain, microfibril synthesis is upregulated in PDL fibroblasts, resulting in oxytalan fiber bundle thickening (Tsuruga et al., 2009). It is believed that oxytalan fibers is not altered by age changes in the PDL under standard physiological conditions (Moxham and Evans, 1995). However, previous studies found oxytalan fibers in aged rats exhibiting different reactions under orthodontic force from those in young rats. More oxytalan fibers prevailed around blood vessels, oxytalan meshwork were more complex and irregular, indicating a loss of elasticity under orthodontic force in rats (Chantawiboonchai et al., 1999).
The characteristics of blood vessels in the PDL also undergo age-related changes. As important constituents of the PDL, blood vessels provide oxygen, nutrients, immune cells for the tissue reconstitution during the orthodontic process (Li et al., 2021). Under mechanical pressure, blood vessels are compressed and deformed, creating a hypoxic environment along with circulating immune cell migration, starting a sterile inflammatory response while starting the osteoclastic process. Vascular endothelial cells also stimulate osteoblast differentiation through Runx2 expression (Kohno et al., 2003). Ren et al. (2008b) measured the mean surface area of the periodontal blood vessels in mice. Adult mice presented higher blood vessel surface area than young mice without stress and during the initial phase (1–2 weeks) of orthodontic treatment. However, when moving on to the late phase (2–8 weeks), the blood vessel surface area exhibited a significant decrease, reaching a level similar to that of young mice. Meantime, the numbers of PDL blood vessels also increased during the late phase of OTM in all mice. Large (surface area > 500 µm2) and small (surface area < 500 µm2) blood vessels increased in young mice, while only small blood vessels increased in old mice. This discrepancy has not been clarified and still need more comprehensive research.
4 Delayed bone remodeling response in adults during orthodontic treatment
The ability of bone remodeling is crucial in the orthodontic procedure as movement of tooth depends on the resorption of alveolar bone on the pressure side and deposition on the tension side. In order to initiate bone remodeling, osteoblast and osteoclast activation and differentiation is fundamental. This relies on a cell-to-cell communications network which consists of PDLCs, osteocytes, osteoblasts, osteoclasts, immune cells as well as various cytokines (Li et al., 2021). Foremost, after orthodontic force application, with signals transduced through the membrane receptors and ion channels, a swift response of mechanosensitive PDLCs come into action (Jin et al., 2015; Chang et al., 2020; Jin et al., 2020). Subsequently, secreting cytokines are released into the extra cellular matrix. The expression of TNF-related ligand RANKL as well as the ratio with its antagonist OPG are increased, indicating the beginning of catabolic procedures in the alveolar bone (Kawasaki et al., 2006; Shen et al., 2020). Meanwhile, the activation of osteoclasts is also mediated by other inflammatory cytokines. The expression of the interleukin family (IL-1β, Il-2, Il-6, Il-8 etc.), TNF-α, IFN-γ were all upregulated as a result of force application, therefore regulating the signaling of several osteoclastogenic pathways (Grant et al., 2013; Kapoor et al., 2014). Meanwhile, activation of osteoblasts are also found with the elevation of its specific marker Osteocalcin, symbolizing the anabolic process of the alveolar bone (Teixeira et al., 2017).
4.1 Slower osteoclast activation and regulated cytokine expression in aged orthodontic process
Compared with adolescents, the alveolar bone density in adults is often higher (Bridges et al., 1988), especially during mid-age, both in human and animals (Boskey and Coleman, 2010). However, the number of osteoclasts in adult rats is lower than young rats during the initial phase of tooth movement (0–2 weeks), which corresponds to the faster movement speed in young rats during early phase (Ren et al., 2003). During the linear phase, the number decreases in young rats and increases in adult rats, gradually resulting in higher osteoclast numbers in adult rats (Ren et al., 2005; Li et al., 2016). Compared to adults, adolescents have a more rapid activation and recruitment of osteoclasts and may initiate the bone resorption process earlier (Figure 3). Similarly, after removal of orthodontic forces, the regression of osteoclast number is much faster in young rats than adult rats (Li et al., 2016).
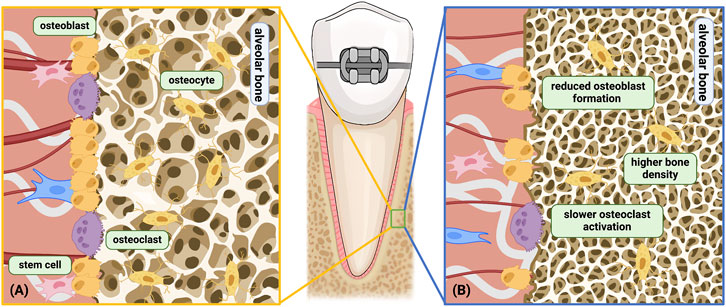
Figure 3. Age-related changes related to the alveolar bone under orthodontic force. (A) Young alveolar bone remodeling under orthodontic force; (B) aged alveolar bone remodeling under orthodontic force. Differences shown above: a) Higher bone density; b) Slower osteoclast activation and decreased cell sensitivity; c) Reduced osteoblast differentiation and formation; d) Hindered bone turnover. Among the above differences, b only occurs on the compression side, while a, c, d occurs on both the compression side and the tension side. Created with BioRender.com.
The activation and recruitment of osteoclasts relies on cytokines, especially RANKL and M-CSF, which activates the NF-κB pathway and the MAPK phosphorylation cascade (Brooks et al., 2011; Nakashima et al., 2011; Kitaura et al., 2020). RANKL can be expressed by osteoblasts, osteocytes, PDLCs, and some immune cells including T cells, B cells. When RANKL is ablated from PDLCs and bone lining cells, the formation of osteoclasts was blocked and tooth movement speed decreased (Yang et al., 2018). Currently, the changes in RANKL expression with age are still unclear and vary with patients. During the initial and linage phases, the RANKL expression in PDLCs increased in both young and adult patients. However, no significant differences was found between the two, with the expression being slightly higher in young patients (George et al., 2020). Similar results were found in animals, with a non-significant RANKL expression between young and adult rats on both the tension and the pressure side during OTM (Li et al., 2016). The changes in RANKL levels in gingival crevicular fluid are more unstable. Kawasaki et al. (2006) have found increased RANKL level after tooth movement in adolescent gingival crevicular fluid significantly higher than adults in human, while Alikhani et al. (2018) believe that the increase in RANKL levels in adult human is higher. The inconsistencies in experimental results may derive from research heterogenicity and difference in methodology. In addition, cytokine levels in gingival crevicular fluid can be influenced by multiple factors, including probing depth, oral hygiene, degree of gingival inflammation, periodontal treatment history, sampling area etc. (Hatipoğlu et al., 2007; Wassall and Preshaw, 2016; López Roldán et al., 2020).
OPG inhibits the activation of osteoclasts and the process of bone resorption by competitively binding to RANKL, preventing the interaction between RANKL and its receptor RANK (Kanzaki et al., 2001; Eriksen, 2010). During OTM, OPG expression level maintained a low expression pattern in adolescent hPDLCs, while its levels elevated in adult hPDLCs (George et al., 2020). Meantime, OPG level in the human GCF was downregulated during OTM, with a smaller reduction in adult GCF than adolescents (Kawasaki et al., 2006). Although the regulation tendency is different between the two studies, the OPG level is always higher in adults, promoting bone formation while limiting the osteoclastogenic rate of RANKL. The RANKL⁄OPG ratio is also an indicator of bone remodeling capability (Yamaguchi, 2009). The adult RANKL⁄OPG ratio in hPDLC expression as well as in the human GCF was relatively inferior to that in adolescents during OTM, especially during the initial phase (Kawasaki et al., 2006; George et al., 2020).
The concentration of TNF-α and CCL2 peaked in the GCF after 1 day of OTM in human, and soon returned to baseline at day 7 in adolescents, while maintaining a high level in adults till day 14 (Alikhani et al., 2018). Similarly, MMP9 concentration in the human GCF peaked at day 1 and returned to baseline at day 28 in both adults and adolescents, with a significantly higher concentration in adults during OTM, though no difference were found at baseline (Alikhani et al., 2018). TNF-α is a proinflammatory cytokines and can promote osteoclast formation through activating the NF-κB pathway (Marahleh et al., 2019; Noguchi et al., 2020). CCL2 belongs to the chemokine family, and promotes chemotaxis, recruitment and activation of osteoclasts (Taddei et al., 2012). Meanwhile, MMP9 is a kind of matrix metalloproteinase secreted by osteoclasts, and participates in mediation of proteolysis (Henriksen et al., 2011). It is also a critical participant in the OTM process, depletion of MMP9 inhibits tooth movement (Holliday et al., 2003). Conversely, adult rats have less osteoclasts than young rats during the initial phase of OTM(Ren et al., 2005; Li et al., 2016).
4.2 Reduced osteoblast differentiation and formation in aged orthodontic process
Besides bone resorption, bone deposition as an anabolic process is also an important part of bone remodeling. ALP and Osteocalcin are classic markers for osteogenic activity (Wei and Karsenty, 2015; Makris et al., 2023). ALP expression in human was significantly upregulated in adolescents during OTM, while remaining essentially stable in adults, leading to a significant difference between the two on day 28 (George et al., 2020). Osteocalcin, encoded by the BGLAP gene and solely produced by osteoblasts, plays a significant role in osteogenesis and bone mineralization. BGLAP expression experienced a delayed increase in adults during OTM. Its expression was upregulated in adolescent hPDLCs throughout the initial and late phase, while only increased from day 14 to day 28 in adult hPDLCs (George et al., 2020).
5 Root resorption, pulpal reaction, local pain sensation and gingival reactions experience age-related changes in orthodontic movement
Orthodontic treatment is often accompanied by various side effects, including root resorption, pulp reactions, and local pain. These can affect the patient’s treatment experience and the teeth integrity. Moreover, the occurrence and severity of these side effects can be influenced by maturation and aging.
5.1 Increased root resorption in adults during tooth movement
Root resorption is a common and inevitable complication in orthodontic treatment. Mechanical loading on the periodontal tissue leads to degradation and hyalinization of the PDLCs and blood vessels, creating a sterile inflammatory environment (Lin et al., 2023), which subsequently activates odontoclasts and resorption of the superficial root cementum or even the underlying dentin (Jepsen et al., 2023). Ren et al. found a significant increase in average root resorption severity and max root resorption severity on the pressure side between the early phase (0–2 weeks) and late phase (4–12 weeks) of OTM in adult rats, while no difference was seen in young rats (Ren et al., 2008a). The root resorption severity was positively correlated with tooth movement duration and amount, while negatively correlated with tooth movement speed in rats (Ren et al., 2007). However, no difference was spotted respecting the incident of root resorption, average root resorption severity and max root resorption severity between adult and young rats during the 12 weeks of tooth movement (Ren et al., 2008a). Adults may experience more severe root resorption than adolescents as treatment time extends. The elevated expression of inflammatory cytokines plays a crucial role in root resorption, activating odontoclasts (Yamaguchi and Fukasawa, 2021; Lin et al., 2023).
5.2 Reduced pulpal flow in adults during tooth movement
Age-related changes also occur in the dental pulp. Due to the production of secondary dentin with aging, the pulp chamber narrows, followed by increased calcification within the dentinal tubules. Meanwhile, pulpal fibrosis, decreased cellular components, nerves and blood vessels, as well as declined proliferation and differentiation of mesenchymal stem cells occurs with pulpal aging (Maeda, 2020; de Farias and Rezende, 2023). The orthodontic process further causes age-related changes in the dental pulp. Adult rats experience a significantly aggravated ablation of dental cusps during OTM than young rats (Von Böhl et al., 2016). A thicker layer of predentin at the pulpal horn and pulp chamber roof is exhibited, though no significant difference of the thickness was observed compared to the control group. Reaction of pulpal blood flow to orthodontic treatment was different in adults and adolescents as well. Throughout the orthodontic process, the pulp blood flow in adults was consistently lower than in adolescents in human (Ersahan and Sabuncuoglu, 2018). The pulpal blood flow in both groups decreased within the first week of tooth movement. However, the pulpal blood flow returned to baseline by week three in adolescents, whereas in adults, it had not recovered at 1 month. The delayed pulpal blood flow recovery in adults may be related to hindered angiogenesis response. Angiogenesis response takes place in the dental pulp after initiation of OTM, especially in the crown third and root third of the pulp (Derringer et al., 1996). Amid this process, the release of VEGF, FGF-2, PDGF, TGF-β and EGF in response to orthodontic forces promotes the regeneration of micro-vessels, therefore leading to reconstruction of the local vascular network and the rebound of pulpal blood flow (Derringer and Linden, 2004; von Böhl et al., 2012).
5.3 Enhanced pain response in adults during tooth movement
Pain is often seen after orthodontic force application. Adult patients experience more pain and discomfort during tooth movement (Alikhani et al., 2018). This is related to the enhanced periodontal inflammatory responses in adults after force application, including vascular, cellular and chemical events (Long et al., 2016). Under orthodontic forces, the blood vessels are compressed and undergo hypoxia, with subsequently increased permeability, immune cells are locally recruited and infiltrates the PDL. Meanwhile, these PDLCs produce abundant inflammatory cytokines such as IL-1β, IL-6, CCL2, TNF-α, PGE2, generating orthodontic pain, which are more expressed in adult PDLCs (Yang et al., 2014; Gameiro et al., 2015; Long et al., 2016; Toyama et al., 2023).
5.4 Compromised gingival reactions among adult orthodontic patients
The gingiva is believed to experience remodeling during orthodontic treatment. Changes on the keratinized gingiva height, sulcus depth, papilla height and gingival invaginations may appear at the space closure sites in premolar extraction patients, there are also risks of gingival recession in human (Ramos de Faria et al., 2023; Alsulaimani and Qali, 2024; Arsić et al., 2024; Han et al., 2024; Seidel et al. 2022). As age increase, the soft tissue reaction towards orthodontic treatment varies. A more severe gingival recession is found in adult orthodontic patients, compared with adolescents (Alhaija et al., 2018). The causative agent may be the difference in periodontal hygiene, as dental calculus and stains are more prevalent in adult orthodontic patients, however, there is significantly more dental biofilms in children than adults (Mei et al., 2017; Alhaija et al., 2018). On the other hand, other periodontal indices showed no significant difference between adults and adolescents in human, including gingival index, plaque index, probing depth (Alhaija et al., 2018). Furthermore, gingival invaginations are more severe in adult patents with a larger invaginate volume, though the prevalence were identical between adults and adolescents (Stappert et al., 2018). Gingival invaginations are believed to promote relapse and impair periodontal health, thus this difference must be recognized (Gölz et al., 2011).
6 Interventions for acceleration of adult orthodontic treatment
The primary factor deterring adults from seeking orthodontic treatment is the extended treatment duration. Thus, interventions for acceleration of tooth movement are of high demand. Interventions for tooth movement acceleration can be classified into three types, including surgical approaches, physical stimuli and biological approaches.
The regional acceleratory phenomenon (RAP) is believed to be the mechanism of surgical mediated acceleration of OTM, which is the enhancement of local bone turnover (Frost, 1983; Wilcko et al., 2009; Hu and Li, 2022). When the cortical bone is surgically injured, osteoblasts and osteoclasts gather, boosting local bone turnover (Zhou et al., 2019; Erdenebat et al., 2022). Immune cells such as macrophages also participates during this process, while there are increased expression inflammatory cytokines such as TNF-α (Kinjo et al., 2022; Wang et al., 2022). The effect of RAP often last for 4 months and peaks at 1–2 months after surgery (Yaffe et al., 1994). Common surgical approaches include corticotomy, piezocision, micro-osteoperforations and periodontally accelerated osteogenic orthodontics (PAOO), which are all effective in inducing tooth movement acceleration (Keser and Naini, 2022). Compared with corticotomy, piezocision and micro-osteoperforations is less traumatic, while PAOO may maintain or improve the periodontal environment after treatment, and increase the range of tooth movement (Gao et al., 2021).
Though surgical approaches provide committed acceleratory effects, trauma cannot be avoided. Therefore, alternative physical or biological methods are still being sought. Physical stimuli are believed to achieve accelerated tooth movement. Light vibrational forces (LVF) and photobiomodulation (PBM) therapy are common techniques aiming to increase tooth movement speed. LVF is believed to promote osteogenesis, while stimulate expression of inflammatory cytokines and number of osteoclasts, thus expected to improve OTM speed (Nishimura et al., 2008; Slatkovska et al., 2011; Phusuntornsakul et al., 2018). However, the acceleratory effect of LVF is still controversial, meta-analysis uncovered that neither did LVF reduce total orthodontic treatment duration, nor did it increase tooth movement speed at the alignment stage or the space closure stage (El-Angbawi et al., 2023). Yet, other studies detect a raise in tooth movement speed (Leethanakul et al., 2016; Tangtanawat et al., 2023). PBM therapy, also known as low- level laser therapy (LLLT), is believed to activate osteoclasts, elevate inflammatory cytokine level and modulate bone metabolism through local laser irradiation (Yamaguchi et al., 2010; Altan et al., 2012; Hsu et al., 2018). There is sufficient proof that PBM can stimulate tooth movement and reduce treatment duration, especially during the initial alignment stage (Cruz et al., 2004; Kau et al., 2013; El-Angbawi et al., 2023).
Other biological approaches have been tested for promoting tooth movement, one of the clinically applied methods is the use platelet-rich fibrin (PRF). It is believed that PRF may increase the concentration of bone metabolic related proteins including RANKL, MMP-8, TNF-α, and Il-1β, while able to speed up the retraction of anterior teeth (Erdur et al., 2021; Karakasli and Erdur, 2021; Barhate et al., 2022; Ammar et al., 2024).
7 Conclusion
Development in age decelerates the OTM speed, and impacts the orthodontic side effects including root resorption and orthodontic pain, which is a result of altered PDL remodeling and bone turnover abilities. The cellular status, cytokine expression and tissue structural changes all contributes to the age-related changes during the orthodontic process. Current researches on age-related changes in orthodontics proves to be highly heterogeneous and are mostly focus on phenotypic changes, while the intrinsic mechanisms are not yet thoroughly understood. In order to provide guidance for accelerating tooth movement in adult orthodontics and reducing treatment side effects, more high-quality, comprehensive researches are essential.
Author contributions
JW: Data curation, Investigation, Writing–original draft, Methodology. YH: Conceptualization, Writing–review and editing, Funding acquisition, Investigation, Methodology. FC: Writing–review and editing, Methodology. WL: Funding acquisition, Supervision, Writing–review and editing, Conceptualization, Resources.
Funding
The author(s) declare that financial support was received for the research, authorship, and/or publication of this article. This work was supported by the China Dental Care Foundation (A2023-015).
Conflict of interest
The authors declare that the research was conducted in the absence of any commercial or financial relationships that could be construed as a potential conflict of interest.
The author(s) declared that they were an editorial board member of Frontiers, at the time of submission. This had no impact on the peer review process and the final decision.
Publisher’s note
All claims expressed in this article are solely those of the authors and do not necessarily represent those of their affiliated organizations, or those of the publisher, the editors and the reviewers. Any product that may be evaluated in this article, or claim that may be made by its manufacturer, is not guaranteed or endorsed by the publisher.
References
Alhaija E. S. A., Al-Saif E. M., Taani D. Q. (2018). Periodontal health knowledge and awareness among subjects with fixed orthodontic appliance. Dent. Press J. Orthod. 23 (5), e1–e40. doi:10.1590/2177-6709.23.5.40.e1-9.onl
Alhashimi N., Frithiof L., Brudvik P., Bakhiet M. (2001). Orthodontic tooth movement and de novo synthesis of proinflammatory cytokines. Am. J. Orthod. Dentofac. Orthop. 119 (3), 307–312. doi:10.1067/mod.2001.110809
Alikhani M., Chou M. Y., Khoo E., Alansari S., Kwal R., Elfersi T., et al. (2018). Age-dependent biologic response to orthodontic forces. Am. J. Orthod. Dentofac. Orthop. 153 (5), 632–644. doi:10.1016/j.ajodo.2017.09.016
Alsulaimani L., Qali M. (2024). Relationship between cone-beam ct evaluation and clinical evaluation before and after orthodontic treatment and the rate of gingival recession: a systematic review. Cureus 16 (6), e62536. doi:10.7759/cureus.62536
Altan B. A., Sokucu O., Ozkut M. M., Inan S. (2012). Metrical and histological investigation of the effects of low-level laser therapy on orthodontic tooth movement. Lasers Med. Sci. 27 (1), 131–140. doi:10.1007/s10103-010-0853-2
Ammar A. M., Al-Sabbagh R., Hajeer M. Y. (2024). Evaluation of the effectiveness of the platelet-rich plasma compared to the injectable platelet-rich fibrin on the rate of maxillary canine retraction: a three-arm randomized controlled trial. Eur. J. Orthod. 46 (1), cjad056. doi:10.1093/ejo/cjad056
Arsić I., Marinković N., Pajević T., Marković J., Dragović M., Stamenković Z., et al. (2024). The impact of orthodontic extrusion on keratinized gingiva. Med. Kaunas. 60 (7), 1157. doi:10.3390/medicina60071157
Asiri S. N., Tadlock L. P., Buschang P. H. (2019). The prevalence of clinically meaningful malocclusion among US adults. Orthod. Craniofac Res. 22 (4), 321–328. doi:10.1111/ocr.12328
Bakker A. D., Kulkarni R. N., Klein-Nulend J., Lems W. F. (2014). IL-6 alters osteocyte signaling toward osteoblasts but not osteoclasts. J. Dent. Res. 93 (4), 394–399. doi:10.1177/0022034514522485
Barhate U. H., Duggal I., Mangaraj M., Sharan J., Duggal R., Jena A. K. (2022). Effects of autologous leukocyte-platelet rich fibrin (L-PRF) on the rate of maxillary canine retraction and various biomarkers in gingival crevicular fluid (GCF): a split mouth randomized controlled trial. Int. Orthod. 20 (4), 100681. doi:10.1016/j.ortho.2022.100681
Boskey A. L., Coleman R. (2010). Aging and bone. J. Dent. Res. 89 (12), 1333–1348. doi:10.1177/0022034510377791
Bridges T., King G., Mohammed A. (1988). The effect of age on tooth movement and mineral density in the alveolar tissues of the rat. Am. J. Orthod. Dentofac. Orthop. 93 (3), 245–250. doi:10.1016/s0889-5406(88)80010-6
Brooks P. J., Heckler A. F., Wei K., Gong S. G. (2011). M-CSF accelerates orthodontic tooth movement by targeting preosteoclasts in mice. Angle Orthod. 81 (2), 277–283. doi:10.2319/051210-258.1
Calcinotto A., Kohli J., Zagato E., Pellegrini L., Demaria M., Alimonti A. (2019). Cellular senescence: aging, cancer, and injury. Physiol. Rev. 99 (2), 1047–1078. doi:10.1152/physrev.00020.2018
Chang M., Lin H., Fu H., Wang J., Yang Y., Wan Z., et al. (2020). CREB activation affects mesenchymal stem cell migration and differentiation in periodontal tissues due to orthodontic force. Int. J. Biochem. Cell Biol. 129, 105862. doi:10.1016/j.biocel.2020.105862
Chantawiboonchai P., Iida J., Soma K. (1999). Effects of aging on oxytalan fibre in mouse periodontal ligament. J. Med. Dent. Sci. 46 (2), 75–82. doi:10.11480/JMDS.460202
Chen Y., Xu X., Tan Z., Ye C., Zhao Q., Chen Y. (2012). Age-related BMAL1 change affects mouse bone marrow stromal cell proliferation and osteo-differentiation potential. Arch. Med. Sci. 8 (1), 30–38. doi:10.5114/aoms.2012.27277
Chibebe P. C., Starobinas N., Pallos D. (2010). Juveniles versus adults: differences in PGE2 levels in the gingival crevicular fluid during orthodontic tooth movement. Braz Oral Res. 24 (1), 108–113. doi:10.1590/s1806-83242010000100018
Claudino D., Traebert J. (2013). Malocclusion, dental aesthetic self-perception and quality of life in a 18 to 21 year-old population: a cross section study. BMC Oral Health 13, 3. doi:10.1186/1472-6831-13-3
Cruz D. R., Kohara E. K., Ribeiro M. S., Wetter N. U. (2004). Effects of low-intensity laser therapy on the orthodontic movement velocity of human teeth: a preliminary study. Lasers Surg. Med. 35 (2), 117–120. doi:10.1002/lsm.20076
de Carlos F., Cobo J., Díaz-Esnal B., Arguelles J., Vijande M., Costales M. (2006). Orthodontic tooth movement after inhibition of cyclooxygenase-2. Am. J. Orthod. Dentofac. Orthop. 129 (3), 402–406. doi:10.1016/j.ajodo.2005.11.020
de Farias J. O., Rezende T. M. B. (2023). Dental pulp and apical papilla cells senescence: causes, consequences, and prevention. Biogerontology 24 (4), 533–539. doi:10.1007/s10522-023-10029-y
Derringer K. A., Jaggers D. C., Linden R. W. (1996). Angiogenesis in human dental pulp following orthodontic tooth movement. J. Dent. Res. 75 (10), 1761–1766. doi:10.1177/00220345960750100901
Derringer K. A., Linden R. W. (2004). Vascular endothelial growth factor, fibroblast growth factor 2, platelet derived growth factor and transforming growth factor beta released in human dental pulp following orthodontic force. Arch. Oral Biol. 49 (8), 631–641. doi:10.1016/j.archoralbio.2004.02.011
El-Angbawi A., McIntyre G., Fleming P. S., Bearn D. (2023). Non-surgical adjunctive interventions for accelerating tooth movement in patients undergoing fixed orthodontic treatment. Cochrane Database Syst. Rev. 6 (6), Cd010887. doi:10.1002/14651858.CD010887.pub2
Erdenebat T., Lee D. J., Kim S. J., Choi Y. J., Kim E. J., Choi E. H., et al. (2022). Effect of the number of micro-osteoperforations on the rate of tooth movement and periodontal response in mice. Front. Physiol. 13, 837094. doi:10.3389/fphys.2022.837094
Erdur E. A., Karakaslı K., Oncu E., Ozturk B., Hakkı S. (2021). Effect of injectable platelet-rich fibrin (i-PRF) on the rate of tooth movement. Angle Orthod. 91 (3), 285–292. doi:10.2319/060320-508.1
Eriksen E. F. (2010). Cellular mechanisms of bone remodeling. Rev. Endocr. Metab. Disord. 11 (4), 219–227. doi:10.1007/s11154-010-9153-1
Ersahan S., Sabuncuoglu F. A. (2018). Effect of age on pulpal blood flow in human teeth during orthodontic movement. J. Oral Sci. 60 (3), 446–452. doi:10.2334/josnusd.17-0316
Feng W., Yang P., Liu H., Zhang F., Li M. (2022). IL-6 promotes low concentration of RANKL-induced osteoclastic differentiation by mouse BMMs through trans-signaling pathway. J. Mol. Histol. 53 (3), 599–610. doi:10.1007/s10735-022-10077-7
Frost H. M. (1983). The regional acceleratory phenomenon: a review. Henry Ford. Hosp. Med. J. 31 (1), 3–9.
Gameiro G. H., Schultz C., Trein M. P., Mundstock K. S., Weidlich P., Goularte J. F. (2015). Association among pain, masticatory performance, and proinflammatory cytokines in crevicular fluid during orthodontic treatment. Am. J. Orthod. Dentofac. Orthop. 148 (6), 967–973. doi:10.1016/j.ajodo.2015.05.029
Gao J., Nguyen T., Oberoi S., Oh H., Kapila S., Kao R. T., et al. (2021). The significance of utilizing A corticotomy on periodontal and orthodontic outcomes: a systematic review and meta-analysis. Biol. (Basel) 10 (8), 803. doi:10.3390/biology10080803
George P., George J. K., Krishnan V., Vijayaraghavan N., Rajendran S. R., Chandran B. M., et al. (2020). Periodontal ligament cells in adolescents and adults: genetic level responses to orthodontic forces. Am. J. Orthod. Dentofac. Orthop. 158 (6), 816–823. doi:10.1016/j.ajodo.2019.10.024
Gölz L., Reichert C., Jäger A. (2011). Gingival invagination--a systematic review. J. Orofac. Orthop. 72 (6), 409–420. doi:10.1007/s00056-011-0046-z
Grant M., Wilson J., Rock P., Chapple I. (2013). Induction of cytokines, MMP9, TIMPs, RANKL and OPG during orthodontic tooth movement. Eur. J. Orthod. 35 (5), 644–651. doi:10.1093/ejo/cjs057
Han M., Li S. H., Yao Y., Zhao Y., You L. P., Zheng Q., et al. (2024). Risk factors for gingival invagination: a retrospective study. J. Clin. Periodontol. 51, 1199–1209. doi:10.1111/jcpe.14005
Hatipoğlu H., Yamalik N., Berberoğlu A., Eratalay K. (2007). Impact of the distinct sampling area on volumetric features of gingival crevicular fluid. J. Periodontol. 78 (4), 705–715. doi:10.1902/jop.2007.060331
Henriksen K., Bollerslev J., Everts V., Karsdal M. A. (2011). Osteoclast activity and subtypes as a function of physiology and pathology—implications for future treatments of osteoporosis. Endocr. Rev. 32 (1), 31–63. doi:10.1210/er.2010-0006
Holliday L. S., Vakani A., Archer L., Dolce C. (2003). Effects of matrix metalloproteinase inhibitors on bone resorption and orthodontic tooth movement. J. Dent. Res. 82 (9), 687–691. doi:10.1177/154405910308200906
Hsu L. F., Tsai M. H., Shih A. H., Chen Y. C., Chang B. E., Chen Y. J., et al. (2018). 970 nm low-level laser affects bone metabolism in orthodontic tooth movement. J. Photochem Photobiol. B 186, 41–50. doi:10.1016/j.jphotobiol.2018.05.011
Hu Y., Li H. (2022). Biological mechanism of surgery-mediated acceleration of orthodontic tooth movement: a narrative review. J. Int. Med. Res. 50 (9), 3000605221123904. doi:10.1177/03000605221123904
Jepsen K., Sculean A., Jepsen S. (2023). Complications and treatment errors involving periodontal tissues related to orthodontic therapy. Periodontol 92 (1), 135–158. doi:10.1111/prd.12484
Jin Y., Ding L., Ding Z., Fu Y., Song Y., Jing Y., et al. (2020). Tensile force-induced PDGF-BB/PDGFRβ signals in periodontal ligament fibroblasts activate JAK2/STAT3 for orthodontic tooth movement. Sci. Rep. 10 (1), 11269. doi:10.1038/s41598-020-68068-1
Jin Y., Li J., Wang Y., Ye R., Feng X., Jing Z., et al. (2015). Functional role of mechanosensitive ion channel Piezo1 in human periodontal ligament cells. Angle Orthod. 85 (1), 87–94. doi:10.2319/123113-955.1
Jonsson T., Arnlaugsson S., Karlsson K. O., Ragnarsson B., Arnarson E. O., Magnusson T. E. (2007). Orthodontic treatment experience and prevalence of malocclusion traits in an Icelandic adult population. Am. J. Orthod. Dentofac. Orthop. 131 (1), 8.e11–e18. doi:10.1016/j.ajodo.2006.05.030
Kang Y. G., Nam J. H., Kim K. H., Lee K. S. (2010). FAK pathway regulates PGE₂ production in compressed periodontal ligament cells. J. Dent. Res. 89 (12), 1444–1449. doi:10.1177/0022034510378521
Kanzaki H., Chiba M., Shimizu Y., Mitani H. (2001). Dual regulation of osteoclast differentiation by periodontal ligament cells through RANKL stimulation and OPG inhibition. J. Dent. Res. 80 (3), 887–891. doi:10.1177/00220345010800030801
Kapoor P., Kharbanda O. P., Monga N., Miglani R., Kapila S. (2014). Effect of orthodontic forces on cytokine and receptor levels in gingival crevicular fluid: a systematic review. Prog. Orthod. 15 (1), 65. doi:10.1186/s40510-014-0065-6
Karakasli K., Erdur E. A. (2021). The effect of platelet-rich fibrin (PRF) on maxillary incisor retraction rate. Angle Orthod. 91 (2), 213–219. doi:10.2319/050820-412.1
Kau C. H., Kantarci A., Shaughnessy T., Vachiramon A., Santiwong P., de la Fuente A., et al. (2013). Photobiomodulation accelerates orthodontic alignment in the early phase of treatment. Prog. Orthod. 14, 30. doi:10.1186/2196-1042-14-30
Kawasaki K., Takahashi T., Yamaguchi M., Kasai K. (2006). Effects of aging on RANKL and OPG levels in gingival crevicular fluid during orthodontic tooth movement. Orthod. Craniofac Res. 9 (3), 137–142. doi:10.1111/j.1601-6343.2006.00368.x
Keser E., Naini F. B. (2022). Accelerated orthodontic tooth movement: surgical techniques and the regional acceleratory phenomenon. Maxillofac. Plast. Reconstr. Surg. 44 (1), 1. doi:10.1186/s40902-021-00331-5
Kinjo R., Kitaura H., Ogawa S., Ohori F., Noguchi T., Marahleh A., et al. (2022). Micro-osteoperforations induce TNF-α expression and accelerate orthodontic tooth movement via TNF-α-responsive stromal cells. Int. J. Mol. Sci. 23 (6), 2968. doi:10.3390/ijms23062968
Kitaura H., Marahleh A., Ohori F., Noguchi T., Shen W. R., Qi J., et al. (2020). Osteocyte-related cytokines regulate osteoclast formation and bone resorption. Int. J. Mol. Sci. 21 (14), 5169. doi:10.3390/ijms21145169
Kohno S., Kaku M., Tsutsui K., Motokawa M., Ohtani J., Tenjo K., et al. (2003). Expression of vascular endothelial growth factor and the effects on bone remodeling during experimental tooth movement. J. Dent. Res. 82 (3), 177–182. doi:10.1177/154405910308200306
Kyomen S., Tanne K. (1997). Influences of aging changes in proliferative rate of PDL cells during experimental tooth movement in rats. Angle Orthod. 67 (1), 67–72. doi:10.1043/0003-3219(1997)067<0067:Ioacip>2.3.Co;2
Leethanakul C., Suamphan S., Jitpukdeebodintra S., Thongudomporn U., Charoemratrote C. (2016). Vibratory stimulation increases interleukin-1 beta secretion during orthodontic tooth movement. Angle Orthod. 86 (1), 74–80. doi:10.2319/111914-830.1
Li X., Li M., Lu J., Hu Y., Cui L., Zhang D., et al. (2016). Age-related effects on osteoclastic activities after orthodontic tooth movement. Bone Jt. Res. 5 (10), 492–499. doi:10.1302/2046-3758.510.Bjr-2016-0004.R2
Li Y., Zhan Q., Bao M., Yi J., Li Y. (2021). Biomechanical and biological responses of periodontium in orthodontic tooth movement: up-date in a new decade. Int. J. Oral Sci. 13 (1), 20. doi:10.1038/s41368-021-00125-5
Lim W. H., Liu B., Mah S. J., Chen S., Helms J. A. (2014). The molecular and cellular effects of ageing on the periodontal ligament. J. Clin. Periodontol. 41 (10), 935–942. doi:10.1111/jcpe.12277
Lin S., Marvidou A. M., Novak R., Moreinos D., Abbott P. V., Rotstein I. (2023). Pathogenesis of non-infection related inflammatory root resorption in permanent teeth: a narrative review. Int. Endod. J. 56 (12), 1432–1445. doi:10.1111/iej.13976
Lombardo G., Vena F., Negri P., Pagano S., Barilotti C., Paglia L., et al. (2020). Worldwide prevalence of malocclusion in the different stages of dentition: a systematic review and meta-analysis. Eur. J. Paediatr. Dent. 21 (2), 115–122. doi:10.23804/ejpd.2020.21.02.05
Long H., Wang Y., Jian F., Liao L. N., Yang X., Lai W. L. (2016). Current advances in orthodontic pain. Int. J. Oral Sci. 8 (2), 67–75. doi:10.1038/ijos.2016.24
López Roldán A., García Giménez J. L., Alpiste Illueca F. (2020). Impact of periodontal treatment on the RANKL/OPG ratio in crevicular fluid. PLoS One 15 (1), e0227757. doi:10.1371/journal.pone.0227757
Maeda H. (2020). Aging and senescence of dental pulp and hard tissues of the tooth. Front. Cell Dev. Biol. 8, 605996. doi:10.3389/fcell.2020.605996
Makris K., Mousa C., Cavalier E. (2023). Alkaline phosphatases: biochemistry, functions, and measurement. Calcif. Tissue Int. 112 (2), 233–242. doi:10.1007/s00223-022-01048-x
Marahleh A., Kitaura H., Ohori F., Kishikawa A., Ogawa S., Shen W. R., et al. (2019). TNF-Α directly enhances osteocyte RANKL expression and promotes osteoclast formation. Front. Immunol. 10, 2925. doi:10.3389/fimmu.2019.02925
Mayahara K., Kobayashi Y., Takimoto K., Suzuki N., Mitsui N., Shimizu N. (2007). Aging stimulates cyclooxygenase-2 expression and prostaglandin E2 production in human periodontal ligament cells after the application of compressive force. J. Periodontal Res. 42 (1), 8–14. doi:10.1111/j.1600-0765.2006.00885.x
Mei L., Chieng J., Wong C., Benic G., Farella M. (2017). Factors affecting dental biofilm in patients wearing fixed orthodontic appliances. Prog. Orthod. 18 (1), 4. doi:10.1186/s40510-016-0158-5
Mitsui N., Suzuki N., Maeno M., Mayahara K., Yanagisawa M., Otsuka K., et al. (2005). Optimal compressive force induces bone formation via increasing bone sialoprotein and prostaglandin E(2) production appropriately. Life Sci. 77 (25), 3168–3182. doi:10.1016/j.lfs.2005.03.037
Mohanakumar A., Vijay G. L., Vijayaraghavan N., Rajendran R. S., Chandran M. B., Thulasidharan M. U., et al. (2021). Morphological alterations, activity, mRNA fold changes, and aging changes before and after orthodontic force application in young and adult human-derived periodontal ligament cells. Eur. J. Orthod. 43 (6), 690–696. doi:10.1093/ejo/cjab025
Moxham B. J., Evans I. L. (1995). The effects of aging upon the connective tissues of the periodontal ligament. Connect. Tissue Res. 33 (1-3), 31–35. doi:10.3109/03008209509016978
Nakashima T., Hayashi M., Fukunaga T., Kurata K., Oh-Hora M., Feng J. Q., et al. (2011). Evidence for osteocyte regulation of bone homeostasis through RANKL expression. Nat. Med. 17 (10), 1231–1234. doi:10.1038/nm.2452
Nanci A., Bosshardt D. D. (2006). Structure of periodontal tissues in health and disease. Periodontol 40, 11–28. doi:10.1111/j.1600-0757.2005.00141.x
Nishimura M., Chiba M., Ohashi T., Sato M., Shimizu Y., Igarashi K., et al. (2008). Periodontal tissue activation by vibration: intermittent stimulation by resonance vibration accelerates experimental tooth movement in rats. Am. J. Orthod. Dentofac. Orthop. 133 (4), 572–583. doi:10.1016/j.ajodo.2006.01.046
Noguchi T., Kitaura H., Ogawa S., Qi J., Shen W. R., Ohori F., et al. (2020). TNF-α stimulates the expression of RANK during orthodontic tooth movement. Arch. Oral Biol. 117, 104796. doi:10.1016/j.archoralbio.2020.104796
Nunes L., Quintanilha L., Perinetti G., Capelli J. J. (2017). Effect of orthodontic force on expression levels of ten cytokines in gingival crevicular fluid. Arch. Oral Biol. 76, 70–75. doi:10.1016/j.archoralbio.2017.01.016
Phusuntornsakul P., Jitpukdeebodintra S., Pavasant P., Leethanakul C. (2018). Vibration enhances PGE(2), IL-6, and IL-8 expression in compressed hPDL cells via cyclooxygenase pathway. J. Periodontol. 89 (9), 1131–1141. doi:10.1002/jper.17-0653
Pilon J. J., Kuijpers-Jagtman A. M., Maltha J. C. (1996). Magnitude of orthodontic forces and rate of bodily tooth movement. An experimental study. Am. J. Orthod. Dentofac. Orthop. 110 (1), 16–23. doi:10.1016/s0889-5406(96)70082-3
Ramos de Faria F., de Sá Werneck C., Kuchenbecker Rösing C., Willer Farinazzo Vitral R., José da Silva Campos M. (2023). Lower incisor inclination and thickness of the alveolar process and mandibular symphysis in the development of gingival recession: a retrospective cohort study. Saudi Dent. J. 35 (6), 651–656. doi:10.1016/j.sdentj.2023.05.016
Ren Y., Kuijpers-Jagtman A. M., Maltha J. C. (2005). Immunohistochemical evaluation of osteoclast recruitment during experimental tooth movement in young and adult rats. Arch. Oral Biol. 50 (12), 1032–1039. doi:10.1016/j.archoralbio.2005.04.005
Ren Y., Maltha J. C., Kuijpers-Jagtman A. M. (2007). Tooth movement characteristics in relation to root resorption in young and adult rats. Eur. J. Oral Sci. 115 (6), 449–453. doi:10.1111/j.1600-0722.2007.00485.x
Ren Y., Maltha J. C., Liem R. S., Stokroos I., Kuijpers-Jagtman A. M. (2008a). Age-dependent external root resorption during tooth movement in rats. Acta Odontol. Scand. 66 (2), 93–98. doi:10.1080/00016350801982522
Ren Y., Maltha J. C., Stokroos I., Liem R. S., Kuijpers-Jagtman A. M. (2008b). Effect of duration of force application on blood vessels in young and adult rats. Am. J. Orthod. Dentofac. Orthop. 133 (5), 752–757. doi:10.1016/j.ajodo.2007.10.030
Ren Y., Maltha J. C., Stokroos L., Liem R. S., Kuijpers-Jagtman A. M. (2008c). Age-related changes of periodontal ligament surface areas during force application. Angle Orthod. 78 (6), 1000–1005. doi:10.2319/080107-357.1
Ren Y., Maltha J. C., Van 't Hof M. A., Kuijpers-Jagtman A. M. (2003). Age effect on orthodontic tooth movement in rats. J. Dent. Res. 82 (1), 38–42. doi:10.1177/154405910308200109
Ren Y., Maltha J. C., Van't Hof M. A., Von Den Hoff J. W., Kuijpers-Jagtman A. M., Zhang D. (2002). Cytokine levels in crevicular fluid are less responsive to orthodontic force in adults than in juveniles. J. Clin. Periodontol. 29 (8), 757–762. doi:10.1034/j.1600-051x.2002.290813.x
Seidel C. L., Gerlach R. G., Weider M., Wölfel T., Schwarz V., Ströbel A., et al. (2022). Influence of probiotics on the periodontium, the oral microbiota and the immune response during orthodontic treatment in adolescent and adult patients (ProMB Trial): study protocol for a prospective, double-blind, controlled, randomized clinical trial. BMC Oral Health 22 (1), 148. doi:10.1186/s12903-022-02180-8
Shen Y., Pan Y., Guo S., Sun L., Zhang C., Wang L. (2020). The roles of mechanosensitive ion channels and associated downstream MAPK signaling pathways in PDLC mechanotransduction. Mol. Med. Rep. 21 (5), 2113–2122. doi:10.3892/mmr.2020.11006
Shimpo S., Horiguchi Y., Nakamura Y., Lee M., Oikawa T., Noda K., et al. (2003). Compensatory bone formation in young and old rats during tooth movement. Eur. J. Orthod. 25 (1), 1–7. doi:10.1093/ejo/25.1.1
Sims M. R., Leppard P. I., Sampson W. J., Dreyer C. W. (1996). Microvascular luminal volume changes in aged mouse periodontal ligament. J. Dent. Res. 75 (7), 1503–1511. doi:10.1177/00220345960750071101
Slatkovska L., Alibhai S. M., Beyene J., Hu H., Demaras A., Cheung A. M. (2011). Effect of 12 months of whole-body vibration therapy on bone density and structure in postmenopausal women: a randomized trial. Ann. Intern Med. 155 (10), 668–679. doi:10.7326/0003-4819-155-10-201111150-00005
Stappert D., Geiman R., Zadi Z. H., Reynolds M. A. (2018). Gingival clefts revisited: evaluation of the characteristics that make one more susceptible to gingival clefts. Am. J. Orthod. Dentofac. Orthop. 154 (5), 677–682. doi:10.1016/j.ajodo.2018.01.018
Strydom H., Maltha J. C., Kuijpers-Jagtman A. M., Von den Hoff J. W. (2012). The oxytalan fibre network in the periodontium and its possible mechanical function. Arch. Oral Biol. 57 (8), 1003–1011. doi:10.1016/j.archoralbio.2012.06.003
Taddei S. R., Andrade I., Queiroz-Junior C. M., Garlet T. P., Garlet G. P., Cunha Fde Q., et al. (2012). Role of CCR2 in orthodontic tooth movement. Am. J. Orthod. Dentofac. Orthop. 141 (2), 153–160. doi:10.1016/j.ajodo.2011.07.019
Tangtanawat P., Thammanichanon P., Suttapreyasri S., Leethanakul C. (2023). Light orthodontic force with high-frequency vibration accelerates tooth movement with minimal root resorption in rats. Clin. Oral Investig. 27 (4), 1757–1766. doi:10.1007/s00784-022-04804-3
Teixeira C. C., Alansari S., Sangsuwon C., Nervina J., Alikhani M. (2017). “Biphasic theory and the biology of tooth movement,” in Clinical guide to accelerated orthodontics: with a focus on micro-osteoperforations. Editor M. Alikhani (Cham: Springer International Publishing), 1–18.
Toyama N., Ono T., Ono T., Nakashima T. (2023). The interleukin-6 signal regulates orthodontic tooth movement and pain. Biochem. Biophys. Res. Commun. 684, 149068. doi:10.1016/j.bbrc.2023.09.096
Tsuruga E., Nakashima K., Ishikawa H., Yajima T., Sawa Y. (2009). Stretching modulates oxytalan fibers in human periodontal ligament cells. J. Periodontal Res. 44 (2), 170–174. doi:10.1111/j.1600-0765.2008.01099.x
Ullrich N., Schröder A., Jantsch J., Spanier G., Proff P., Kirschneck C. (2019). The role of mechanotransduction versus hypoxia during simulated orthodontic compressive strain-an in vitro study of human periodontal ligament fibroblasts. Int. J. Oral Sci. 11 (4), 33. doi:10.1038/s41368-019-0066-x
von Böhl M., Ren Y., Fudalej P. S., Kuijpers-Jagtman A. M. (2012). Pulpal reactions to orthodontic force application in humans: a systematic review. J. Endod. 38 (11), 1463–1469. doi:10.1016/j.joen.2012.07.001
Von Böhl M., Ren Y., Kuijpers-Jagtman A. M., Fudalej P. S., Maltha J. C. (2016). Age-related changes of dental pulp tissue after experimental tooth movement in rats. PeerJ 4, e1625. doi:10.7717/peerj.1625
Wang Q., Xie J., Zhou C., Lai W. (2022). Substrate stiffness regulates the differentiation profile and functions of osteoclasts via cytoskeletal arrangement. Cell Prolif. 55 (1), e13172. doi:10.1111/cpr.13172
Wassall R. R., Preshaw P. M. (2016). Clinical and technical considerations in the analysis of gingival crevicular fluid. Periodontol 70 (1), 65–79. doi:10.1111/prd.12109
Wei J., Karsenty G. (2015). An overview of the metabolic functions of osteocalcin. Rev. Endocr. Metab. Disord. 16 (2), 93–98. doi:10.1007/s11154-014-9307-7
Wilcko M. T., Wilcko W. M., Pulver J. J., Bissada N. F., Bouquot J. E. (2009). Accelerated osteogenic orthodontics technique: a 1-stage surgically facilitated rapid orthodontic technique with alveolar augmentation. J. Oral Maxillofac. Surg. 67 (10), 2149–2159. doi:10.1016/j.joms.2009.04.095
Wu R. X., Bi C. S., Yu Y., Zhang L. L., Chen F. M. (2015). Age-related decline in the matrix contents and functional properties of human periodontal ligament stem cell sheets. Acta Biomater. 22, 70–82. doi:10.1016/j.actbio.2015.04.024
Yaffe A., Fine N., Binderman I. (1994). Regional accelerated phenomenon in the mandible following mucoperiosteal flap surgery. J. Periodontol. 65 (1), 79–83. doi:10.1902/jop.1994.65.1.79
Yamaguchi M. (2009). RANK/RANKL/OPG during orthodontic tooth movement. Orthod. Craniofac Res. 12 (2), 113–119. doi:10.1111/j.1601-6343.2009.01444.x
Yamaguchi M., Fukasawa S. (2021). Is inflammation a friend or foe for orthodontic treatment? inflammation in orthodontically induced inflammatory root resorption and accelerating tooth movement. Int. J. Mol. Sci. 22 (5), 2388. doi:10.3390/ijms22052388
Yamaguchi M., Hayashi M., Fujita S., Yoshida T., Utsunomiya T., Yamamoto H., et al. (2010). Low-energy laser irradiation facilitates the velocity of tooth movement and the expressions of matrix metalloproteinase-9, cathepsin K, and alpha(v) beta(3) integrin in rats. Eur. J. Orthod. 32 (2), 131–139. doi:10.1093/ejo/cjp078
Yang C. Y., Jeon H. H., Alshabab A., Lee Y. J., Chung C. H., Graves D. T. (2018). RANKL deletion in periodontal ligament and bone lining cells blocks orthodontic tooth movement. Int. J. Oral Sci. 10 (1), 3. doi:10.1038/s41368-017-0004-8
Yang Z., Luo W., Wang J., Tan Y., Fu R., Fang B. (2014). Chemokine ligand 2 in the trigeminal ganglion regulates pain induced by experimental tooth movement. Angle Orthod. 84 (4), 730–736. doi:10.2319/090213-643.1
Yin J., Zhang H., Zeng X., Yu J., Wang H., Jiang Y., et al. (2023). Prevalence and influencing factors of malocclusion in adolescents in Shanghai, China. BMC Oral Health 23 (1), 590. doi:10.1186/s12903-023-03187-5
Keywords: orthodontic tooth movement, aging, age-related changes, periodontal ligament, alveolar bone, tooth movement speed
Citation: Wang J, Huang Y, Chen F and Li W (2024) The age-related effects on orthodontic tooth movement and the surrounding periodontal environment. Front. Physiol. 15:1460168. doi: 10.3389/fphys.2024.1460168
Received: 05 July 2024; Accepted: 28 August 2024;
Published: 06 September 2024.
Edited by:
Masaru Kaku, Niigata University, JapanCopyright © 2024 Wang, Huang, Chen and Li. This is an open-access article distributed under the terms of the Creative Commons Attribution License (CC BY). The use, distribution or reproduction in other forums is permitted, provided the original author(s) and the copyright owner(s) are credited and that the original publication in this journal is cited, in accordance with accepted academic practice. No use, distribution or reproduction is permitted which does not comply with these terms.
*Correspondence: Yiping Huang, eWlwaW5naHVhbmdAYmptdS5lZHUuY24=; Weiran Li, d2VpcmFubGlAYmptdS5lZHUuY24=