- 1Department of Ultrasound, The General Hospital of Western Theater Command, Chengdu, Sichuan, China
- 2Department of Cardiology, The General Hospital of Western Theater Command, Chengdu, Sichuan, China
Introduction: The trend of human migration to terrestrial high altitudes (HA) has been increasing over the years. However, no published prospective studies exist with follow-up periods exceeding 1 month to investigate the cardiac change. This prospective study aimed to investigate the changes in cardiac structure and function in healthy young male lowlanders following long-term migration to HA.
Methods: A total of 122 Chinese healthy young males were divided into 2 groups: those migrating to altitudes between 3600 m and 4000 m (low HA group, n = 65) and those migrating to altitudes between 4000 m and 4700 m (high HA group, n = 57). Traditional echocardiographic parameters were measured at sea level, 1 month and 1 year after migration to HA.
Results: All 4 cardiac chamber dimensions, areas, and volumes decreased after both 1 month and 1 year of HA exposure. This reduction was more pronounced in the high HA group than in the low HA group. Bi-ventricular diastolic function decreased after 1 month of HA exposure, while systolic function decreased after 1 year. Notably, these functional changes were not significantly influenced by altitude differences. Dilation of the pulmonary artery and a progressive increase in pulmonary artery systolic pressure were observed with both increasing exposure time and altitude. Additionally, a decreased diameter of the inferior vena cava and reduced bicuspid and tricuspid blood flow velocity indicated reduced blood flow following migration to the HA.
Discussion: 1 year of migration to HA is associated with decreased blood volume and enhanced hypoxic pulmonary vasoconstriction. These factors contribute to reduced cardiac chamber size and slight declines in bi-ventricular function.
1 Introduction
Transportation network advancements and improved medical care have facilitated an upsurge in human presence at terrestrial high altitudes (HA) (Luks and Hackett, 2022). These regions are characterized by distinct features, including low temperatures, low humidity, high ultraviolet radiation exposure, and most notably, hypobaric hypoxia. A lack of oxygen significantly impacts cellular function. Notably, the cardiovascular system, which is responsible for oxygen transport, plays a crucial role in enabling human adaptation to the challenging HA environments, as evidenced by research findings (Naeije, 2010; Richalet et al., 2024).
Changes in cardiac structure and function are an integral part of the cardiovascular system’s response to the challenges of high-altitude environments (Williams et al., 2022). These challenges include activation of the sympathetic nervous system, hypoxic pulmonary vasoconstriction, and myocardial oxygen deprivation, all of which contribute to increased afterload on the right ventricle (RV) and impaired left ventricular (LV) filling (Vogel and Harris, 1967). Acute responses in the first week at HA are well-documented, with reported increases in heart rate (HR) and decreases in stroke volume (SV) (Maufrais et al., 2017; Boussuges et al., 2000; Holloway et al., 2011). While LV diastolic function declines, LV systolic function remains largely preserved under acute hypoxia. However, changes in RV structural and functional parameters remain debatable (Maufrais et al., 2017; Yuan et al., 2021). Lifelong adaptation to HA has also been extensively studied. Long-term hypoxic exposure leads to remodeling of the pulmonary artery and RV, resulting in sustained pulmonary hypertension that cannot be readily reversed (Penaloza and Arias-Stella, 2007; Hultgren and Miller, 1967). Additionally, chronic mountain sickness patients, characterized by excessive erythropoiesis and debilitating symptoms, reportedly exhibit an adaptive decrease in RV systolic function (Pratali et al., 2013).
Individuals who consider migrating to high-altitude plateaus are concerned about the long-term progression of cardiac changes at different altitudes. This information can aid them in planning their itineraries safely and effectively. However, limited cross-sectional studies provide the only existing data, and several cardiac parameters in these studies exhibit inconsistencies (Liu et al., 2022; Chen et al., 2022). To date, no published prospective studies exist with follow-up periods exceeding 1 month, attributed to the logistical challenges associated with tracking subjects over extended periods in the harsh HA environments. We hypothesized that the LV and RV function would be preserved after 1 month of migration to HA but impaired after 1 year of migration to HA. Therefore, the present study aimed to conduct a prospective investigation of cardiac changes in healthy male lowlanders after 1 month and 1 year of migration to HA at different altitudes.
2 Materials and methods
2.1 Study participants
Lowlanders who were preparing to work on the Qinghai‒Tibet Plateau (altitude >3,600 m) for a long time were consecutively recruited in Chongqing (altitude <500 m) in May 2022. The study population consisted of civilians who were engaged in construction. They underwent a comprehensive medical examination before recruitment, and those with conditions such as patent foramen ovale or other heart diseases detected by conventional echocardiography have been excluded from working at high altitudes. The inclusion criteria were as follows: (Luks and Hackett, 2022): Adult males ages 18–65 years, and (Naeije, 2010) born in a low-altitude place (altitude <1,000 m), with (Richalet et al., 2024) no history of cardiovascular disease or (Williams et al., 2022) chronic conditions affecting heart function. The exclusion criteria included (Luks and Hackett, 2022) a long-term history of high-altitude residence; (Naeije, 2010); poor acoustic windows; (Richalet et al., 2024); loss to follow-up. The recruitment pathway is shown in Figure 1. Ultimately, 122 males with successful 1-month and 1-year follow-ups were prospectively enrolled in this study. Following an initial acclimatization period of several days at high altitude, they engaged in a standardized low-intensity and mid-intensity routine infrastructure work regimen for 6 h daily, 5 days per week. Notably, the participants resided continuously at their high-altitude workplaces for a full year without returning to lower elevations. The study protocol was approved by the Ethics Committee of The General Hospital of Western Theater Command of PLA (approval number: 2022EC3-ky062) and was performed according to the Declaration of Helsinki. Written informed consent was obtained from all participants.
2.2 Research design
All participants undertook a 30-h train journey to the Qinghai‒Tibet Plateau and then dispersed to work at different altitudes. To investigate the influence of altitude, participants were divided into 2 groups: the low-altitude group (LHA, n = 65), comprising individuals residing at altitudes between 3,600 m and 4,000 m, and the high-altitude group (HHA, n = 57), comprising individuals residing at altitudes between 4,000 m and 4,700 m. Baseline measurements were conducted in Chongqing 2 weeks before departure. Subsequent investigations were conducted in Tibet at 1 month (HA-1M) and 1 year (HA-1Y) after arrival at the corresponding altitudes. The data collection duration for each phase was controlled within 1 week.
2.3 Clinical measurements
Systolic blood pressure, diastolic blood pressure, pulsed oxygen saturation, and HR were measured after a minimum of 5 min of rest using a sphygmomanometer (HEM-6200, OMRON) and a pulse oximeter (PO2, Lepu Medical), respectively.
2.4 Echocardiography
After at least 10 min of rest, transthoracic echocardiograms were acquired in the left lateral decubitus position using portable ultrasound machines (M9 and M10; Mindray) equipped with low-frequency (1–5 MHz) phased array transducers by 2 well-trained ultrasound doctors.
Cardiac dimensions and functions were evaluated following the American Society of Echocardiography standards (Lang et al., 2015; Rudski et al., 2010). Detailed protocols of echocardiographic measurements are present in Supplementary Material. The LV ejection fraction was manually measured using Simpson’s biplane method. Cardiac output was calculated by multiplying SV by HR. LV mass was calculated using the linear method, and the LV mass index was determined by dividing LV mass by the body surface area. The left atrial volume was calculated using the biplane method, and the left atrial volume index was calculated by dividing the left atrial volume by the body surface area. Fraction area change and tricuspid annular plane systolic excursion (TAPSE) were measured to assess RV systolic function. The peak velocities of the mitral and tricuspid early (E) and late (A) waves were measured, and the E/A ratio was calculated to assess LV and RV diastolic function. The right atrial (RA) pressure was estimated based on the diameter of the inferior vena cava (IVC) and its inspiratory variation. The tricuspid regurgitation pressure gradient (TRPG) was calculated from the velocity of tricuspid regurgitation (TRV) using the simplified Bernoulli equation. Pulmonary artery systolic pressure (PASP) was estimated by adding TRPG to the RA pressure.
2.5 Statistical analysis
Statistical analyses were performed using SPSS 22.0 software (SPSS Inc., Chicago, IL), with significance set at p < 0.05. Descriptive statistics for categorical variables included frequencies and percentages, and for continuous variables, means ± SD. The normality of continuous variables was assessed using the Kolmogorov-Smirnov test.
Baseline group comparisons employed chi-square tests for categorical data and independent t-tests for continuous data, given compliance with normal distribution. Longitudinal differences in clinical and echocardiographic measures across time points (baseline, 1-month, and 1-year) and between altitude groups (low-altitude vs high-altitude) were analyzed using 2-way repeated measures ANOVA, focusing on interaction and main effects.
Significant findings from the ANOVA were further explored using post hoc Bonferroni correction to adjust for multiple comparisons if a significant main effect was detected with F > 3.86 and p < 0.05. Results were reported as exact p-values, and 95% confidence intervals (CIs) were provided where relevant.
By incorporating 95% CIs, this approach ensures a comprehensive and transparent analysis of the statistical effects of high-altitude migration on cardiac functions over time while maintaining rigorous statistical standards.
3 Results
3.1 Clinical characteristics
A total of 122 males were prospectively enrolled in this study, of whom 65 were in the LHA group and 57 were in the HHA group. The mean altitudes of the LHA and HHA groups were 3,714.8 ± 122.7 m and 4,290.8 ± 175.7 m, respectively. The locations and altitudes are detailed in Table 1. No significant differences were found between the 2 groups in terms of age, height, body mass index, body surface area, or smoking history (Table 2).
Changes in clinical characteristics are shown in Table 3. In HA-1M, the SpO₂ dropped significantly in the LHA group and further decreased in the HHA group. This decrease was maintained in HA-1Y. HR and blood pressure increased significantly in both groups in HA-1M, with no further significant increase in HA-1Y. However, diastolic blood pressure decreased in the HHA group in HA-1Y compared to that in HA-1M. In HA-1M, the HR was higher in the HHA group compared to the LHA group.
3.2 Left heart echocardiographic parameters
The left heart echocardiographic parameters are presented in Table 4; Figure 2, no significant difference was observed between the LHA and HHA groups at any time point. The end-diastolic dimension and volume of the LV, the LA volume, and the SV significantly decreased in HA-1M in both groups. In the LHA group, there was a further decrease in these parameters in HA-1Y, while the HHA group showed no further change. Both groups experienced a significant decrease in ejection fraction in HA-1Y, with no change in HA-1M. Notably, cardiac output decreased only in the LHA group during HA-1Y. The LA dimension and volume indices, LV end-systolic volume, LV mass, LV mass index, bicuspid E velocity, bicuspid A velocity, and bicuspid E/A ratio all decreased in HA-1M but remained unchanged in HA-1Y in both groups. Both interventricular septal thickness and LV posterior wall thickness remained unchanged throughout the year in both groups.
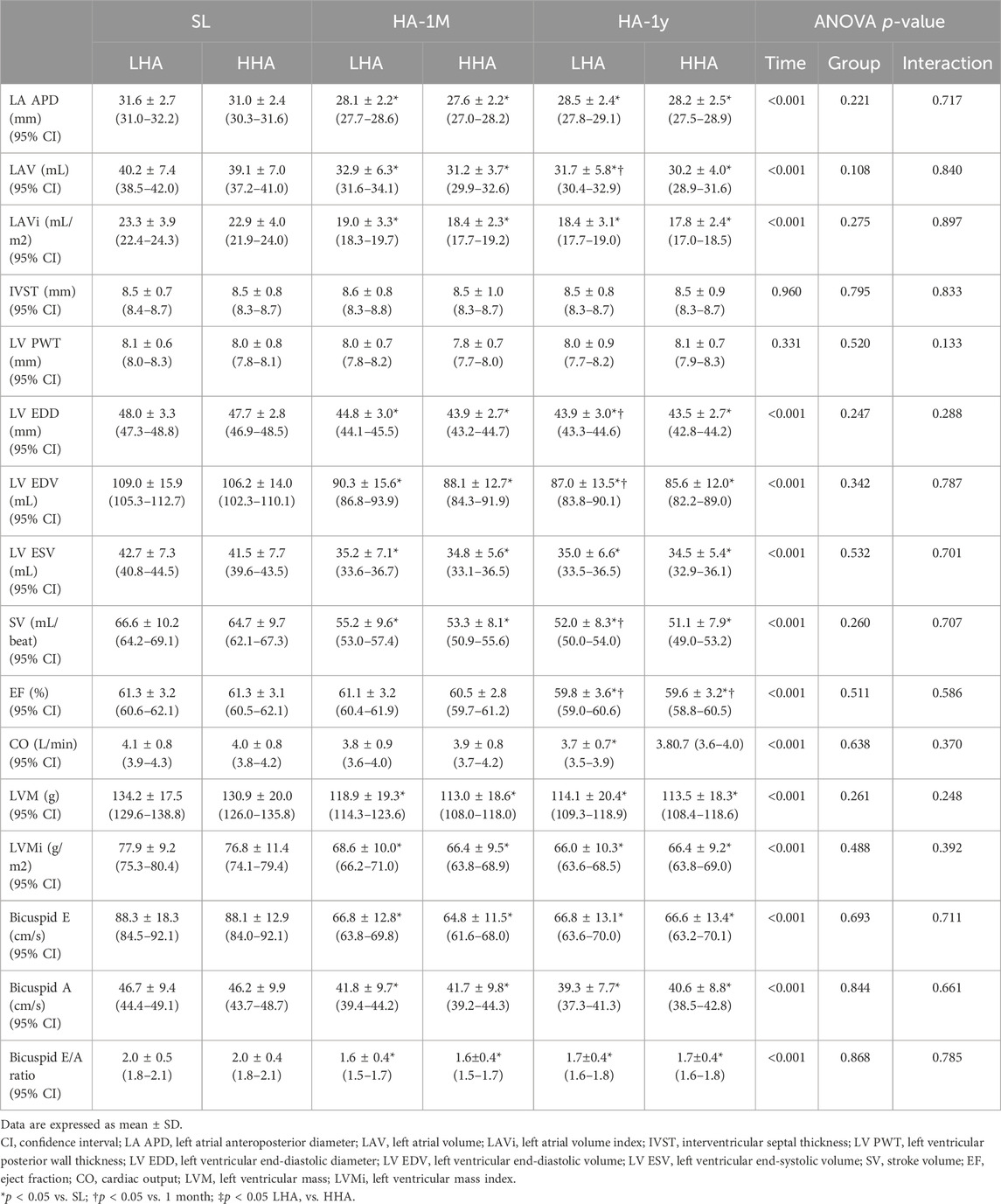
Table 4. Echocardiographic parameters of the left heart at sea level and after 1 month and 1 year of migration to HA.
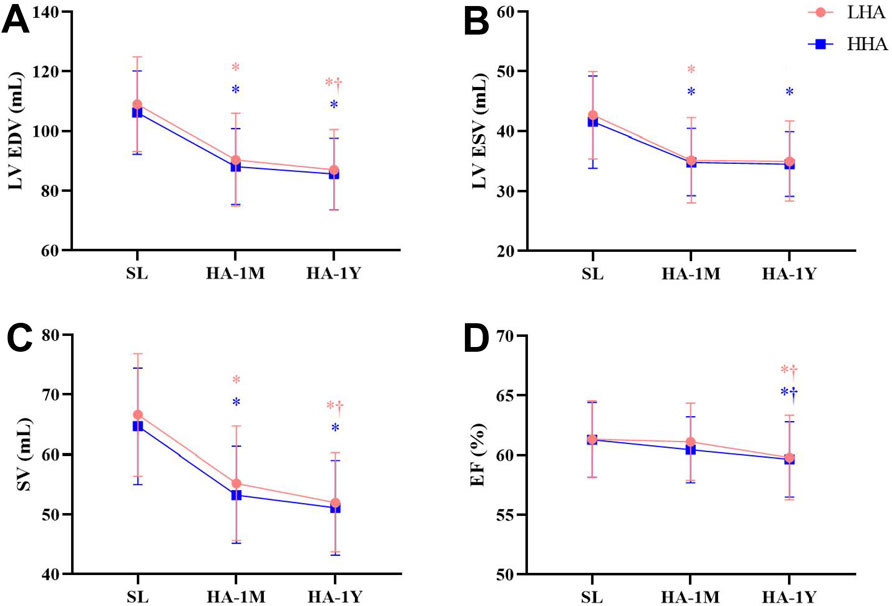
Figure 2. Echocardiographic parameters of the left heart at sea level and after 1 month and 1 year of migration to HA: (A) Left ventricular end-diastolic volume (LV EDV). (B) Left ventricular end-systolic volume (LV ESV). (C) Stroke volume (SV). (D) Ejection fraction (EF) *p < 0.05 vs. SL; †p < 0.05 vs. 1 month.
3.3 Right heart echocardiographic parameters
The right heart echocardiographic parameters are presented in Table 5; Figure 3. In both groups, the RA dimension and area significantly decreased in HA-1M and further decreased in HA-1Y for the LHA group, but not the HHA group. The mid-cavity and outflow tract dimension of the right ventricle, RV end-diastolic area, and tricuspid E/A ratio decreased in HA-1M and remained unchanged in both groups at HA-1Y. The RV free wall thickness remained unchanged across both groups and time points. While fractional area change decreased in HA-1Y, TAPSE only decreased in HA-1M, remaining unchanged in both groups at HA-1Y. Both groups experienced a decrease in tricuspid E velocity in HA-1M, with a further decrease in the HHA group but not the LHA group in HA-1Y. In contrast, the tricuspid A velocity decreased only in HA-1Y, not in HA-1M, for both groups. Notably, there were no significant differences in the dimensional or functional parameters of the RA or RV between the LHA and HHA groups.
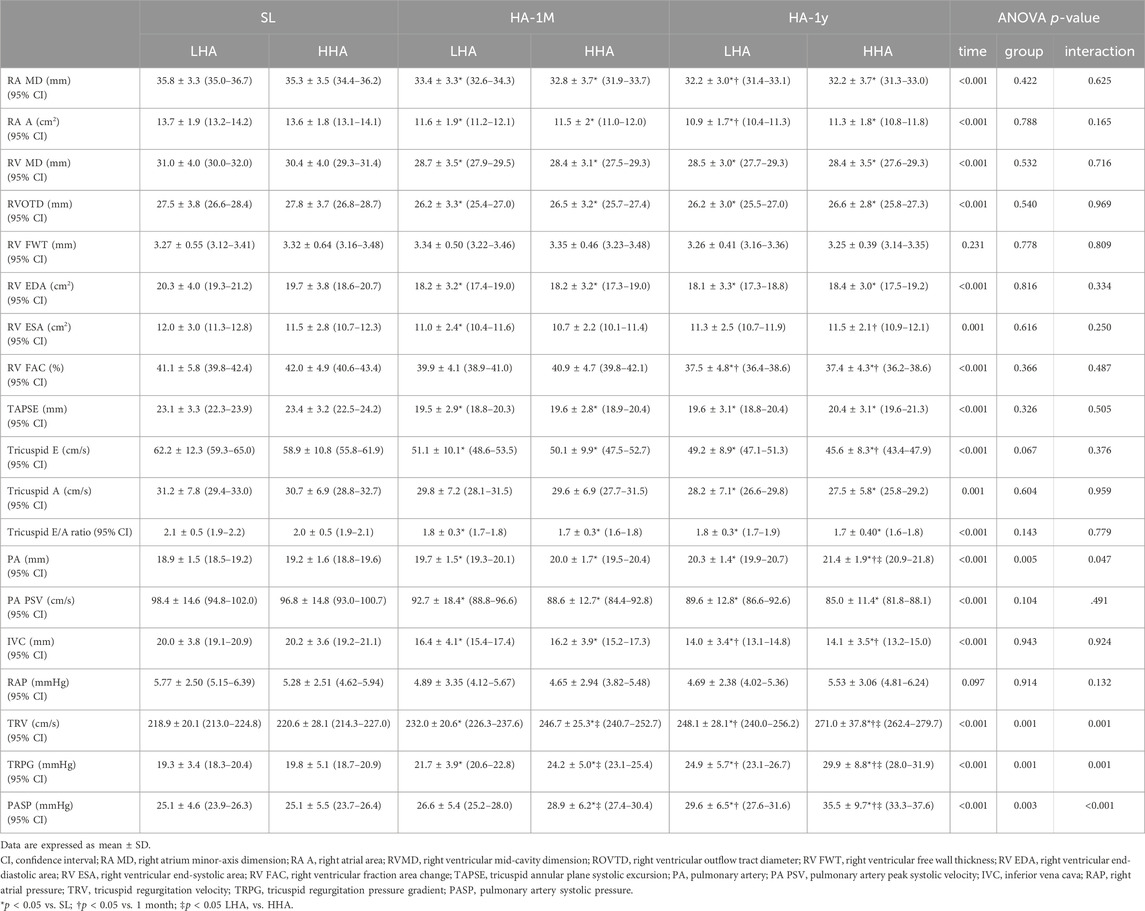
Table 5. Echocardiographic parameters of the right heart at sea level and after 1 month and 1 year of migration to HA.
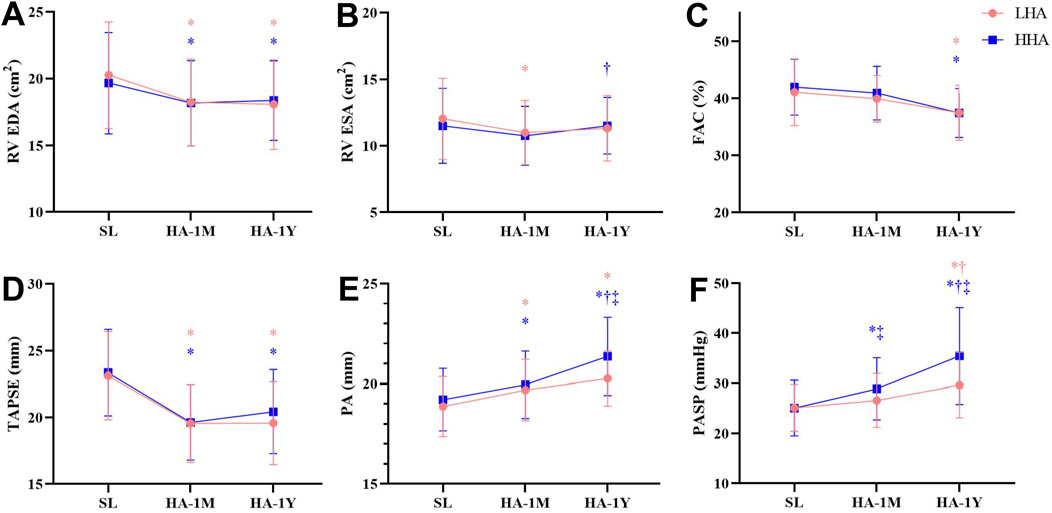
Figure 3. Echocardiographic parameters of the right heart at sea level and after 1 month and 1 year of migration to HA. (A) Right ventricular end-diastolic area (RV EDA). (B) Right ventricular end-systolic area (RV ESA). (C) Fraction area change (FAC). (D) Tricuspid annular plane systolic excursion (TAPSE). (E) Pulmonary artery (PA). (F) Pulmonary artery systolic pressure (PASP). *P < 0.05 vs. SL; †P < 0.05 vs. 1 month; ‡P < 0.05 LHA vs. HHA.
Pulmonary artery diameter widened markedly in HA-1M in both groups. The HHA group experienced further widening in HA-1Y, while the LHA group did not. Notably, the pulmonary artery diameters in the HHA group were significantly wider than those in the LHA group in HA-1Y. Both groups exhibited a decrease in pulmonary artery peak systolic velocity in HA-1M, remaining unchanged in HA-1Y with no significant difference between groups. The diameter of the IVC significantly decreased in HA-1M, with a further decrease in HA-1Y for both groups. However, the right atrial pressure (RAP) estimated by the diameter and variation of the IVC remained unchanged with altitude and time. Both groups showed a progressive increase in TRV, TRPG, and PASP over time. In both HA-1M and HA-1Y, the HHA group displayed significantly higher TRV, TRPG, and PASP compared to the LHA group. Abnormalities of HR, LV eject fraction, RV fraction area change, PASP are presented in Table 6.
4 Discussion
This investigation prospectively examined the impact of 1 month and 1 year of high-altitude exposure on cardiac structure and function in healthy young males across varying altitudes. Our key findings include: (i) Both the left and right atria and ventricle exhibited reductions in size, while the PA dilated, likely due to a combination of decreased blood volume and intensified hypoxic pulmonary vasoconstriction. Interestingly, chamber size reductions occurred at a faster rate with increasing altitude. (ii) Compromised bi-ventricular diastolic function emerged within 1 month of HA exposure, followed by deteriorated bi-ventricular systolic function after 1 year. Notably, these functional changes were independent of altitude. (iii) PASP progressively increased with both the duration and altitude of HA exposure. (iv) Echocardiographic markers of blood volume, including diminished IVC diameter and reduced bi-ventricular inflow velocities, indicated that blood volume decline over time, while no significant altitude-related differences were observed.
4.1 Cardiac remodeling
Over the years, studies examining the RV area in healthy lowlanders upon initial HA exposure have yielded inconsistent results, reporting slight increases (De Boeck et al., 2018), no changes (Maufrais et al., 2017; Stembridge et al., 1985a), or decreases after prolonged acclimatization (after more than 5 years) (Chen et al., 2022). Additionally, RV dimensions vary across populations at different altitudes: lower in Himalayan Sherpa (5,050 m) (Stembridge et al., 1985a), higher in Peruvian Andeans at La Rinconada (5,100 m) (Doutreleau et al., 2022), and similar to lowlanders in Tibetans (4,300 m) (Chen et al., 2022) and Peruvian Andeans at Puno (3,800 m) (Doutreleau et al., 2022). These discrepancies potentially arise from differences in age, ethnicity, and exposure time among cross-sectional study participants. Our longitudinal study revealed decreases in RV dimensional parameters 1 month and 1 year after HA exposure in healthy lowlanders, mirroring changes in the other three chambers. While LV and LA responses aligned with previous reports, the RA response differed unexpectedly, exhibiting a decrease unlike observations in earlier studies (Chen et al., 2022; Doutreleau et al., 2022).
Previous studies (Alexander and Grover, 1983; Stembridge et al., 1985b; Stembridge et al., 2019) have attributed the reduction in left heart size observed in lowlanders upon HA exposure to 2 mechanisms: decreased blood volume and increased hypoxic pulmonary vasoconstriction. However, Richalet et al. (2024) challenged the hypovolemia hypothesis, arguing that increased red blood cell volume maintains blood volume despite decreased plasma volume. While this is true, it is important to consider the temporal difference: red blood cell increases occur several weeks after HA exposure (Siebenmann et al., 1985a; Siebenmann et al., 1985b; Sawka et al., 2000), leaving a period where the plasma volume decrease remains uncompensated. Several studies have documented a reduction in plasma volume in lowlanders to varying degrees during the first 3 weeks of high-altitude exposure (Alexander et al., 1967; Grover et al., 1998; Robach et al., 1985). This decrease serves as a physiological mechanism to elevate the hemoglobin concentration and arterial oxygen content (Siebenmann et al., 1985a). Additionally, there is extensive evidence demonstrating a concomitant reduction in the total blood volume of lowlanders as plasma volume declines, at least during the initial 2 weeks of HA exposure (Jain et al., 1980; Poulsen et al., 1998; Robach et al., 2002). Our study supports this notion, as echocardiographic indicators of blood volume (inferior vena cava diameter, tricuspid and mitral E- and A-wave velocities significantly decreased after 1 month and 1 year of HA exposure, suggesting a decline during the first year of acclimatization. Furthermore, the observed right heart reduction cannot be solely explained by hypoxic pulmonary vasoconstriction, which typically leads to RV dilation due to elevated pulmonary pressure (Motley et al., 1947; Netzer et al., 2017). Although our study revealed increased PASP and PA dilation, suggesting that this mechanism in play, it cannot fully explain the right heart size decrease. It is highly conceivable that the secondary decrease in RV output, caused by increased PASP, resulted in a reduced left ventricular volume and output through serial interaction (Belenkie et al., 2001). Therefore, we propose that both decreased blood volume and hypoxic pulmonary vasoconstriction contribute to the reduction in size observed in all 4 heart chambers. These physiological mechanisms generally serve as adaptive responses to high-altitude exposure, helping to optimize arterial oxygen content and gas exchange (Williams et al., 2022). However, excessive or prolonged decreases in blood volume and hypoxic pulmonary vasoconstriction can lead to increased blood viscosity, pulmonary hypertension, and heart failure. In such cases, maintaining proper hydration, providing supplemental oxygen, and in some instances, administering medication are necessary (Gatterer et al., 2024).
The observed reductions in LV mass and LV mass index in our study are primarily attributed to decreased LV volume, as evidenced by unchanged wall thickness. Similarly, the lack of change in RV free wall thickness suggests potentially minimal myocardial remodeling following 1 year of hypoxic exposure at high altitude.
4.2 Cardiac function
Left and right ventricular systolic function have been shown to be preserved during short-term high-altitude exposure, regardless of altitude. Similarly, native highlanders exhibit this pattern of preserved LV systolic function (Maufrais et al., 2017; Holloway et al., 2011; Stembridge et al., 1985a; Huez et al., 2005). However, reports on RV systolic function in long-term HA residents are mixed, with findings of both decreased and preserved function compared to those in lowlanders (Stembridge et al., 1985a; Doutreleau et al., 2022; Huez et al., 2009; Dedobbeleer et al., 2015). Likewise, chronic mountain sickness patients reportedly have enlarged RVs and impaired RV systolic function (measured by echocardiography), but exercise testing revealed comparable RV contractile reserves to those of healthy highlanders (Pratali et al., 2013). This suggests that echocardiographic parameters may not always accurately reflect true RV systolic impairment. In our study, both the LV ejection fraction and RV fractional area change decreased, with an earlier decline in the TAPSE. While cardiac output was maintained by increased heart rate despite a decrease in SV, SV further dropped in the lower altitude group after 1 year. The early decrease in TAPSE could be secondary to the aforementioned reductions in RV diameter and volume, likely driven by decreased blood volume. Similarly, the decline in the bicuspid and tricuspid E/A ratio may result from diminished blood volume affecting both E-wave velocities. Hence, the presented parameters might not have accurately assessed LV diastolic function in our study. Future investigations should employ blood volume-independent parameters to provide a more reliable assessment of LV diastolic function.
4.3 Impact of different altitudes
Consequently, the lower air and arterial oxygen content at higher altitudes likely induces more severe hypoxic pulmonary vasoconstriction, possibly explaining a key finding of our study: a higher degree of PA dilation and PASP increase with increasing altitude. This finding aligns with previous reports (Doutreleau et al., 2022; Netzer et al., 2017; Sylvester et al., 2012; Sime et al., 1963). Besides, while the dimensional parameters of the LV, LA, and RA remained comparable between altitude groups, these parameters decreased more rapidly in higher altitude groups. However, the timing and degree of bi-ventricular function decline did not differ across altitudes.
4.4 Limitations
This investigation is subject to several limitations. First, the study population was restricted to healthy young males of Chinese ethnicity. This limits the generalizability of our findings to other populations, particularly females. Future research should investigate potential differences in cardiac remodeling and functional changes in females. Second, we were restricted from using traditional echocardiographic parameters due to equipment limitations. As we were unable to use Tissue Doppler Imaging, we could not make a complete assessment of LV diastolic function and RV systolic function. Utilizing more advanced equipment in future studies would allow for longer-term tracking and potentially reveal additional insights. Third, we were failed to include a control cohort staying at low altitude. So, the impact of potential confounding factors such as physical activity, diet, and genetic predispositions cannot be rule out. Incorporating a sea-level control group in future research could provide a more solid basis for comparing and interpreting the observed changes.
5 Conclusion
Our findings demonstrated a reduction in the size of cardiac chambers and a mild decline in bi-ventricular function following high-altitude exposure. These changes are likely attributable to a combination of decreased blood volume and increased hypoxic pulmonary vasoconstriction. Notably, higher altitudes were associated with increased PASP and a faster rate of chamber size reduction. However, all observed parameters of cardiac structure and function remained within the normal range, suggesting no clinically significant impairment during a year-long HA stay.
Data availability statement
The raw data supporting the conclusions of this article will be made available by the authors, without undue reservation.
Ethics statement
The studies involving humans were approved by the Ethics Committee of The General Hospital of Western Theater Command of PLA. The studies were conducted in accordance with the local legislation and institutional requirements. The participants provided their written informed consent to participate in this study.
Author contributions
M-DD: Conceptualization, Investigation, Methodology, Writing–original draft, Writing–review and editing. X-JZ: Data curation, Formal Analysis, Visualization, Writing–review and editing. QF: Resources, Validation, Writing–review and editing. RW: Formal Analysis, Software, Writing–review and editing. FH: Investigation, Writing–review and editing. F-WY: Investigation, Writing–review and editing. X-ML: Investigation, Writing–review and editing. F-FS: Investigation, Writing–review and editing. JT: Resources, Writing–review and editing. SL: Project administration, Supervision, Writing–review and editing. ZC: Funding acquisition, Supervision, Writing–review and editing.
Funding
The author(s) declare that financial support was received for the research, authorship, and/or publication of this article. This work was supported by the People’s Liberation Army The General Hospital of Western Theater Command: (No. 2021XZYG-A09), Chengdu, Sichuan, People’s Republic of China.
Conflict of interest
The authors declare that the research was conducted in the absence of any commercial or financial relationships that could be construed as a potential conflict of interest.
Publisher’s note
All claims expressed in this article are solely those of the authors and do not necessarily represent those of their affiliated organizations, or those of the publisher, the editors and the reviewers. Any product that may be evaluated in this article, or claim that may be made by its manufacturer, is not guaranteed or endorsed by the publisher.
Supplementary material
The Supplementary Material for this article can be found online at: https://www.frontiersin.org/articles/10.3389/fphys.2024.1459031/full#supplementary-material
References
Alexander J. K., Grover R. F. (1983). Mechanism of reduced cardiac stroke volume at high altitude. Clin. Cardiol. 6 (6), 301–303. doi:10.1002/clc.4960060612
Alexander J. K., Hartley L. H., Modelski M., Grover R. F. (1967). Reduction of stroke volume during exercise in man following ascent to 3,100 m altitude. J. Appl. Physiol. 23 (6), 849–858. doi:10.1152/jappl.1967.23.6.849
Belenkie I., Smith E. R., Tyberg J. V. (2001). Ventricular interaction: from bench to bedside. Ann. Med. 33 (4), 236–241. doi:10.3109/07853890108998751
Boussuges A., Molenat F., Burnet H., Cauchy E., Gardette B., Sainty J. M., et al. (2000). Operation Everest III (Comex '97): modifications of cardiac function secondary to altitude-induced hypoxia. An echocardiographic and Doppler study. Am. J. Respir. Crit. Care Med. 161 (1), 264–270. doi:10.1164/ajrccm.161.1.9902096
Chen X., Liu B., Deng Y., Yang F., Wang W., Lin X., et al. (2022). Cardiac adaptation to prolonged high altitude migration assessed by speckle tracking echocardiography. Front. Cardiovasc Med. 9, 856749. doi:10.3389/fcvm.2022.856749
De Boeck B. W., Toma A., Kiencke S., Dehnert C., Zügel S., Siebenmann C., et al. (2018). Altered left ventricular geometry and torsional mechanics in high altitude-induced pulmonary hypertension: a three-dimensional echocardiographic study. J. Am. Soc. Echocardiogr. 31 (3), 314–322. doi:10.1016/j.echo.2017.12.001
Dedobbeleer C., Hadefi A., Pichon A., Villafuerte F., Naeije R., Unger P. (2015). Left ventricular adaptation to high altitude: speckle tracking echocardiography in lowlanders, healthy highlanders and highlanders with chronic mountain sickness. Int. J. Cardiovasc Imaging 31 (4), 743–752. doi:10.1007/s10554-015-0614-1
Doutreleau S., Ulliel-Roche M., Hancco I., Bailly S., Oberholzer L., Robach P., et al. (2022). Cardiac remodelling in the highest city in the world: effects of altitude and chronic mountain sickness. Eur. J. Prev. Cardiol. 29 (17), 2154–2162. doi:10.1093/eurjpc/zwac166
Gatterer H., Villafuerte F. C., Ulrich S., Bhandari S. S., Keyes L. E., Burtscher M. (2024). Altitude illnesses. Nat. Rev. Dis. Prim. 10 (1), 43. doi:10.1038/s41572-024-00526-w
Grover R. F., Selland M. A., McCullough R. G., Dahms T. E., Wolfel E. E., Butterfield G. E., et al. (1998). Beta-adrenergic blockade does not prevent polycythemia or decrease in plasma volume in men at 4300 m altitude. Eur. J. Appl. Physiol. Occup. Physiol. 77 (3), 264–270. doi:10.1007/s004210050331
Holloway C. J., Montgomery H. E., Murray A. J., Cochlin L. E., Codreanu I., Hopwood N., et al. (2011). Cardiac response to hypobaric hypoxia: persistent changes in cardiac mass, function, and energy metabolism after a trek to Mt. Everest Base Camp. Faseb J. 25 (2), 792–796. doi:10.1096/fj.10-172999
Huez S., Faoro V., Guénard H., Martinot J. B., Naeije R. (2009). Echocardiographic and tissue Doppler imaging of cardiac adaptation to high altitude in native highlanders versus acclimatized lowlanders. Am. J. Cardiol. 103 (11), 1605–1609. doi:10.1016/j.amjcard.2009.02.006
Huez S., Retailleau K., Unger P., Pavelescu A., Vachiéry J. L., Derumeaux G., et al. (2005). Right and left ventricular adaptation to hypoxia: a tissue Doppler imaging study. Am. J. Physiol. Heart Circ. Physiol. 289 (4), H1391–H1398. doi:10.1152/ajpheart.00332.2005
Hultgren H. N., Miller H. (1967). Human heart weight at high altitude. Circulation 35 (1), 207–218. doi:10.1161/01.cir.35.1.207
Jain S. C., Bardhan J., Swamy Y. V., Krishna B., Nayar H. S. (1980). Body fluid compartments in humans during acute high-altitude exposure. Aviat. Space Environ. Med. 51 (3), 234–236.
Lang R. M., Badano L. P., Mor-Avi V., Afilalo J., Armstrong A., Ernande L., et al. (2015). Recommendations for cardiac chamber quantification by echocardiography in adults: an update from the American Society of Echocardiography and the European Association of Cardiovascular Imaging. Eur. Heart J. Cardiovasc Imaging 16 (3), 233–270. doi:10.1093/ehjci/jev014
Liu G., Zhao L., Xu Q., Lang M., Xiao R. (2022). Cardiac adaptation to high altitudes after short- and long-term exposure among Chinese Han lowlanders. Echocardiography 39 (3), 465–472. doi:10.1111/echo.15317
Luks A. M., Hackett P. H. (2022). Medical conditions and high-altitude travel. N. Engl. J. Med. 386 (4), 364–373. doi:10.1056/NEJMra2104829
Maufrais C., Rupp T., Bouzat P., Doucende G., Verges S., Nottin S., et al. (2017). Heart mechanics at high altitude: 6 days on the top of Europe. Eur. Heart J. Cardiovasc Imaging 18 (12), 1369–1377. doi:10.1093/ehjci/jew286
Motley H. L., Cournand A., et al. (1947). The influence of short periods of induced acute anoxia upon pulmonary artery pressures in man. Am. J. Physiol. 150 (2), 315–320. doi:10.1152/ajplegacy.1947.150.2.315
Naeije R. (2010). Physiological adaptation of the cardiovascular system to high altitude. Prog. Cardiovasc Dis. 52 (6), 456–466. doi:10.1016/j.pcad.2010.03.004
Netzer N. C., Strohl K. P., Högel J., Gatterer H., Schilz R. (2017). Right ventricle dimensions and function in response to acute hypoxia in healthy human subjects. Acta Physiol. (Oxf) 219 (2), 478–485. doi:10.1111/apha.12740
Penaloza D., Arias-Stella J. (2007). The heart and pulmonary circulation at high altitudes: healthy highlanders and chronic mountain sickness. Circulation 115 (9), 1132–1146. doi:10.1161/circulationaha.106.624544
Poulsen T. D., Klausen T., Richalet J. P., Kanstrup I. L., Fogh-Andersen N., Olsen N. V. (1998). Plasma volume in acute hypoxia: comparison of a carbon monoxide rebreathing method and dye dilution with Evans' blue. Eur. J. Appl. Physiol. Occup. Physiol. 77 (5), 457–461. doi:10.1007/s004210050360
Pratali L., Allemann Y., Rimoldi S. F., Faita F., Hutter D., Rexhaj E., et al. (2013). RV contractility and exercise-induced pulmonary hypertension in chronic mountain sickness: a stress echocardiographic and tissue Doppler imaging study. JACC Cardiovasc Imaging 6 (12), 1287–1297. doi:10.1016/j.jcmg.2013.08.007
Richalet J. P., Hermand E., Lhuissier F. J. (2024). Cardiovascular physiology and pathophysiology at high altitude. Nat. Rev. Cardiol. 21 (2), 75–88. doi:10.1038/s41569-023-00924-9
Robach P., Déchaux M., Jarrot S., Vaysse J., Schneider J. C., Mason N. P., et al. (1985)2000). Operation Everest III: role of plasma volume expansion on VO(2)(max) during prolonged high-altitude exposure. J. Appl. Physiol. 89 (1), 29–37. doi:10.1152/jappl.2000.89.1.29
Robach P., Lafforgue E., Olsen N. V., Déchaux M., Fouqueray B., Westerterp-Plantenga M., et al. (2002). Recovery of plasma volume after 1 week of exposure at 4,350 m. Pflugers Arch. 444 (6), 821–828. doi:10.1007/s00424-002-0894-x
Rudski L. G., Lai W. W., Afilalo J., Hua L., Handschumacher M. D., Chandrasekaran K., et al. (2010). Guidelines for the echocardiographic assessment of the right heart in adults: a report from the American Society of Echocardiography endorsed by the European Association of Echocardiography, a registered branch of the European Society of Cardiology, and the Canadian Society of Echocardiography. J. Am. Soc. Echocardiogr. 23 (7), 685–713. quiz 86-8. doi:10.1016/j.echo.2010.05.010
Sawka M. N., Convertino V. A., Eichner E. R., Schnieder S. M., Young A. J. (2000). Blood volume: importance and adaptations to exercise training, environmental stresses, and trauma/sickness. Med. Sci. Sports Exerc 32 (2), 332–348. doi:10.1097/00005768-200002000-00012
Siebenmann C., Cathomen A., Hug M., Keiser S., Lundby A. K., Hilty M. P., et al. (1985b). Hemoglobin mass and intravascular volume kinetics during and after exposure to 3,454-m altitude. J. Appl. Physiol. 119 (10), 1194–1201. doi:10.1152/japplphysiol.01121.2014
Siebenmann C., Robach P., Lundby C. (1985a). Regulation of blood volume in lowlanders exposed to high altitude. J. Appl. Physiol. 123 (4), 957–966. doi:10.1152/japplphysiol.00118.2017
Sime F., Banchero N., Penaloza D., Gamboa R., Cruz J., Marticorena E. (1963). Pulmonary hypertension in children born and living at high altitudes. Am. J. Cardiol. 11, 143–149. doi:10.1016/0002-9149(63)90054-7
Stembridge M., Ainslie P. N., Boulet L. M., Anholm J., Subedi P., Tymko M. M., et al. (2019). The independent effects of hypovolaemia and pulmonary vasoconstriction on ventricular function and exercise capacity during acclimatisation to 3800 m. J. Physiol. 597 (4), 1059–1072. doi:10.1113/jp275278
Stembridge M., Ainslie P. N., Hughes M. G., Stöhr E. J., Cotter J. D., Nio A. Q., et al. (1985a). Ventricular structure, function, and mechanics at high altitude: chronic remodeling in Sherpa vs. short-term lowlander adaptation. J. Appl. Physiol. 117 (3), 334–343. doi:10.1152/japplphysiol.00233.2014
Stembridge M., Ainslie P. N., Hughes M. G., Stöhr E. J., Cotter J. D., Tymko M. M., et al. (1985b). Impaired myocardial function does not explain reduced left ventricular filling and stroke volume at rest or during exercise at high altitude. J. Appl. Physiol. 119 (10), 1219–1227. doi:10.1152/japplphysiol.00995.2014
Sylvester J. T., Shimoda L. A., Aaronson P. I., Ward J. P. (2012). Hypoxic pulmonary vasoconstriction. Physiol. Rev. 92 (1), 367–520. doi:10.1152/physrev.00041.2010
Vogel J. A., Harris C. W. (1967). Cardiopulmonary responses of resting man during early exposure to high altitude. J. Appl. Physiol. 22 (6), 1124–1128. doi:10.1152/jappl.1967.22.6.1124
Williams A. M., Levine B. D., Stembridge M. (2022). A change of heart: mechanisms of cardiac adaptation to acute and chronic hypoxia. J. Physiol. 600 (18), 4089–4104. doi:10.1113/jp281724
Yuan F., Liu C., Yu S., Bian S., Yang J., Ding X., et al. (2021). The association between notching of the right ventricular outflow tract flow velocity Doppler envelope and impaired right ventricular function after acute high-altitude exposure. Front. Physiol. 12, 639761. doi:10.3389/fphys.2021.639761
Keywords: high altitude, hypoxia, echocardiography, cardiac remodeling, cardiac function
Citation: Deng M-D, Zhang X-J, Feng Q, Wang R, He F, Yang F-W, Liu X-M, Sun F-F, Tao J, Li S and Chen Z (2024) The impact of high-altitude migration on cardiac structure and function: a 1-year prospective study. Front. Physiol. 15:1459031. doi: 10.3389/fphys.2024.1459031
Received: 03 July 2024; Accepted: 20 August 2024;
Published: 30 August 2024.
Edited by:
Ginés Viscor, University of Barcelona, SpainReviewed by:
Camilo Povea, National University of Colombia, ColombiaJan Stepanek, Mayo Clinic Arizona, United States
Copyright © 2024 Deng, Zhang, Feng, Wang, He, Yang, Liu, Sun, Tao, Li and Chen. This is an open-access article distributed under the terms of the Creative Commons Attribution License (CC BY). The use, distribution or reproduction in other forums is permitted, provided the original author(s) and the copyright owner(s) are credited and that the original publication in this journal is cited, in accordance with accepted academic practice. No use, distribution or reproduction is permitted which does not comply with these terms.
*Correspondence: Shuang Li, bG91aXNfMTAxNEAxNjMuY29t; Zhong Chen, dWx0cmFzb3VuZGN6QDE2My5jb20=
†These authors have contributed equally to this work and share first authorship