- 1Computational Physiology Department, Simula Research Laboratory, Oslo, Norway
- 2School of Economics Innovation and Technology, Kristiania University College, Oslo, Norway
- 3ProCardio Center for Innovation, Department of Cardiology, Oslo University Hospital, Oslo, Norway
- 4Institute of Clinical Medicine, Faculty of Medicine, University of Oslo, Oslo, Norway
Background: The electrophysiological mechanism connecting mitral valve prolapse (MVP), premature ventricular complexes and life-threatening ventricular arrhythmia is unknown. A common hypothesis is that stretch activated channels (SACs) play a significant role. SACs can trigger depolarizations or shorten repolarization times in response to myocardial stretch. Through these mechanisms, pathological traction of the papillary muscle (PM), as has been observed in patients with MVP, may induce irregular electrical activity and result in reentrant arrhythmia.
Methods: Based on a patient with MVP and mitral annulus disjunction, we modeled the effect of excessive PM traction in a detailed medical image-derived ventricular model by activating SACs in the PM insertion region. By systematically varying the onset of SAC activation following sinus pacing, we identified vulnerability windows for reentry with 1 ms resolution. We explored how reentry was affected by the SAC reversal potential
Results: In models with healthy tissue or fibrosis modeled solely as CV slowing, we observed two vulnerable periods of reentry: For
Conclusion: Stretch of the PM insertion region following sinus activation may initiate ventricular reentry in patients with MVP, with or without fibrosis. Depending on the SAC reversal potential and timing of stretch, reentry may be triggered by ectopy due to SAC-induced depolarizations or by early repolarization within the SAC region.
1 Introduction
Mitral valve prolapse (MVP), a displacement of a part of the mitral valve into the left atrium, is a common abnormality in the general population, with a reported prevalence of about 2.4% (Freed et al., 1999). Associated with MVP is mitral annulus disjunction (MAD), a separation between the ventricular myocardium and the mitral annulus (Moscatelli and Antonakaki, 2023). Although MVP with or without MAD generally has a good prognosis, a small subset of patients experience life-threatening ventricular arrhythmias (Nishimura et al., 1985; Miller et al., 2018). The electrophysiological mechanism of arrhythmia in these patients is still unclear, and various explanations have been proposed. A leading hypothesis is that arrhythmia may be initiated by premature ventricular complexes (PVCs) triggered by excessive stretch of the papillary muscles (PMs) and inferobasal wall by prolapsing mitral leaflets (Basso and Perazzolo Marra, 2018; Enriquez et al., 2019; Hei et al., 2019). Suggesting the presence of such stretch, studies on MVP patients have reported abnormal myocardial strain patterns (Huttin et al., 2017; Nagata et al., 2023), an abnormal shift of the PMs towards the mitral annulus (Sanfilippo et al., 1992; Hei et al., 2019) as well as fibrosis, inflammation or ischemia within the PMs (Dejgaard et al., 2018; Fulton et al., 2018; Miller et al., 2020; Figliozzi et al., 2023).
It is widely recognized that myocardial stretch can lead to local changes in the membrane potential via activation of stretch activated channels (SACs) (Reed et al., 2014; Quinn and Kohl, 2021). In a myocardial cell, depending on the reversal potential of SAC
While the above-mentioned studies have addressed different components to the relation between MVP/MAD, stretch and arrhythmia, the electrophysiological mechanism of how PM stretch may cause sustained arrhythmia is still an open question. Computational electrophysiological studies have investigated stretch-induced reentry in 2D (Garny and Kohl, 2004), in 3D simulations of commotio cordis following electrical pacing from the apex (Li et al., 2004) and within a region of acute ischemia in the rabbit ventricle (Jie et al., 2010). However, the conditions required to trigger ectopy and subsequent reentry from stretch of the PM region following sinus rhythm, potentially linked to MVP and MAD, remains unexplored.
In this study, we simulated sinus activity followed by SAC activation in the PM insertion region of the human cardiac ventricles. Simulations were conducted in an electrophysiological model based on cardiovascular magnetic resonance (CMR) images of a patient with MVP and concomitant MAD. We thus demonstrated that depending on
2 Materials and methods
2.1 Image data and geometrical mesh construction
To construct the ventricular geometry, we used short-axis late gadolinium-enhanced CMR images of a single patient with MVP and concomitant MAD, provided by the Oslo University Hospital (Aabel et al., 2021). The patient presented with PM fibrosis, PVCs and sustained ventricular tachycardia. The images consisted of 14 slices, with a resolution of 0.91 mm and slice thickness of 10 mm. Using the software Segment (Heiberg et al., 2010), we manually identified the epi- and endocardium of both ventricles. An openly available automatic workflow (Marciniak et al., 2016; Marciniak, 2017) was used to construct a 3D finite element model of the segmented ventricle. The pipeline involves initial slice alignment and the subsequent construction of surfaces using the Visualization Toolkit (Schroeder et al., 1998). From these surfaces, a finite element ventricular model was created using gmsh (Geuzaine and Remacle, 2009). The final model consisted of 6,173,699 elements with a mean resolution of 530
2.2 Spatial SAC region
We represented the effect of mechanical traction on the PM by activating SAC currents in a specific region of tissue, from here on referred to as the SAC region. Since PVCs originating from either of the PMs have been reported in multiple MVP patients (Wilde et al., 1997; Fulton et al., 2018; Conti et al., 2023), we selected a point representative of the inferior PM insertion point for our SAC region. Specifically, the SAC region (Figure 1A) was defined as a spherical region centered in a point in the inferior left ventricular wall, 1/3 of the apico-basal distance away from the apex (Carpentier et al., 2010).
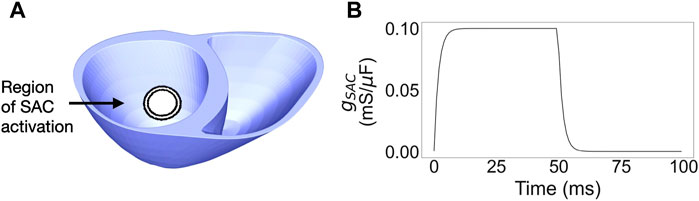
Figure 1. (A) Ventricular mesh with the layered SAC region, from 7 to 10 mm radius. (B) Time dependence of the SAC conductance,
Large individual variations exist regarding both PM shape and PM attachment to the ventricular wall (Rajiah et al., 2019). The latter may range from a single point of attachment to an extensive network of trabeculae connected to varying portions of the wall (Ranganathan and Burch, 1969; Axel, 2004). To investigate how the size of the stretched region affected reentry, we created models with varying radii of the SAC region. Starting from 10 mm, we reduced the radius in steps of 1 mm to investigate which size of the SAC region was needed to induce reentry. If we could no longer induce reentry after shrinking the SAC region, we did not reduce it further. Thus the smallest region used for simulations was of 7 mm radius. For an overview of all ventricular models and their SAC regions, see Table 1.
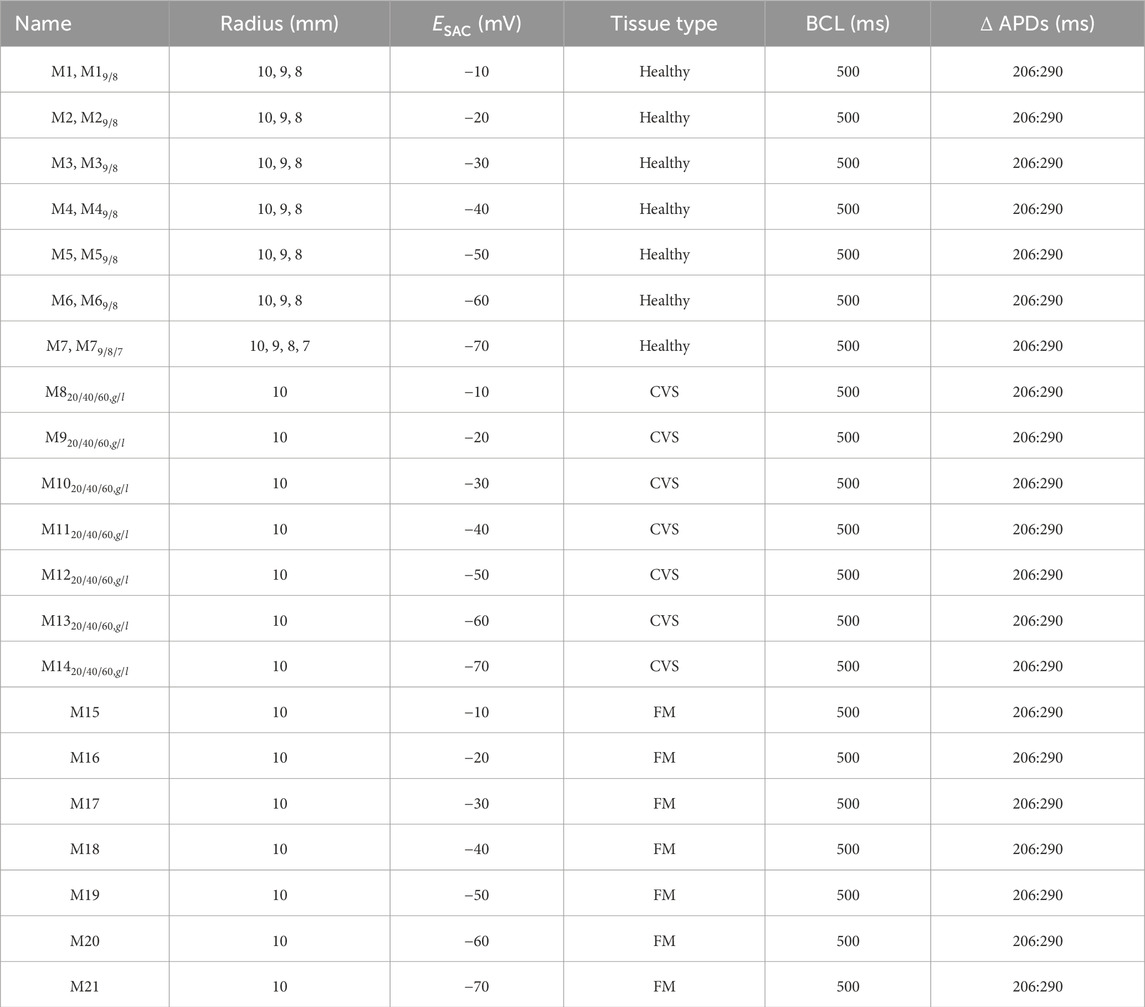
Table 1. Parameter combinations for models included in the main manuscript. For all listed combinations, we activated SAC for a duration of 50 ms, with a maximum conductance
2.3 Electrophysiology
2.3.1 Electrophysiological models
Tissue propagation was represented with the monodomain model as given by:
where
where
2.3.2 Action potential heterogeneity
Various types of heterogeneity in action potential duration (APD), including patient-specific variations, have been reported clinically (Opthof et al., 2017). In our model, in accordance with previous work by Sung et al. (2021) and Keller et al. (2011), we applied linear APD gradients along the apicobasal and transmural direction by scaling the slow delayed rectifier K+ current
We refer to the APD heterogeneity in this study by noting the minimum and maximum APDs when measured at 90
2.3.3 Conductivities
We considered three types of tissue conductivity properties in our models: one with only healthy tissue, one with diffuse fibrosis and one with fibrosis in the SAC region. In the healthy case we tuned the model tissue conductivities (given by
For modelling fibrosis in models
A wide range of CV values have been reported in fibrotic tissue, depending on fibrosis type and severity (Anderson et al., 1993; Kawara et al., 2001). Thus, for both types of fibrosis (diffuse or focal), we created three models with different levels of conduction slowing: 20%, 40% and 60% CV reduction. All CVs were tuned using a 10 cm rod of myocardial tissue with 530
2.3.4 Purkinje activation
We simulated Purkinje activation by stimulating from four root points on the endocardium based on previous work (Cranford et al., 2018), as illustrated in Figure 2. Each point, defined as a spherical volume of 1 mm radius, was stimulated with a transmembrane current of 100
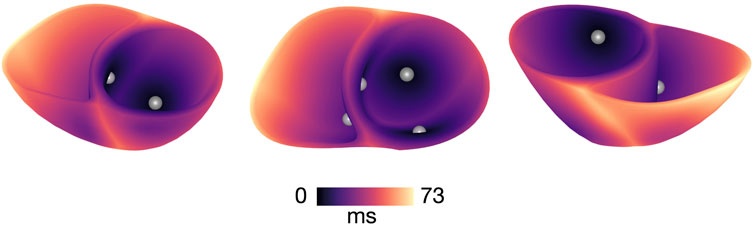
Figure 2. Activation map of sinus stimulation in a model with healthy conductivities and
2.3.5 SAC time course
In accordance with previous work (Hu et al., 2013), we used a simple representation of SAC where the current is given by:
where
The opening probability of SAC channels responds instantaneously to stretch (Reed et al., 2014) and increases with stretch amplitude (Pueyo et al., 2016; Gerach and Loewe, 2024). Thus, in the present study, we used a time dependent
where
The value of
2.4 Fibrotic microstructure
In addition to representing fibrosis using conduction slowing, we created an additional set of models to investigate the effect of fibrotic microstructure. Fibrotic microstructure was applied within the SAC region, based on clinical reports of PM-related fibrosis in MVP and/or MAD (Dejgaard et al., 2018; Nagata et al., 2023). The microstructure was modeled using a mesh splitting approach previously implemented by Balaban et al. (2020). The algorithm creates electrically insulating clefts between mesh elements by duplicating individual mesh facets based on a given probability. We defined this probability as:
where
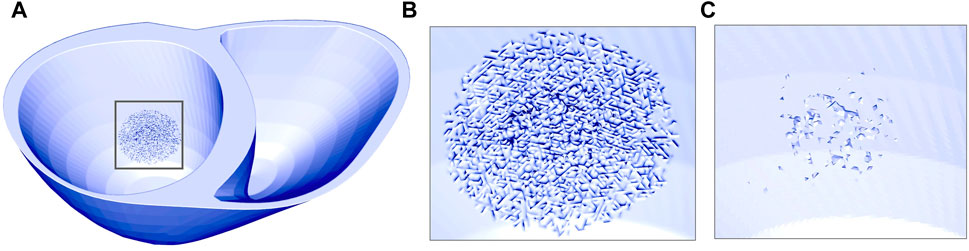
Figure 3. Ventricular mesh with fibrotic microstructure in the SAC region represented by mesh splitting. (A) and (B) illustrates the mesh surfaces. (C) Shows the elements which were completely isolated after mesh splitting and thus removed from the mesh.
2.5 Single AP recordings
To investigate the effect of SAC activation on a single AP, we conducted simulations on a single element mesh in openCARP (open Cardiac Arrhythmia Research Package) (Plank et al., 2021; openCARP consortium et al., 2023). Using the SAC pulse defined in Section 2.3.5, we measured the effect of SAC activation at different time points relative to the AP of an example cell. The simulations were initiated from a prepaced state equal to that used in our
2.6 Whole ventricular simulations
We conducted full ventricular model simulations in openCARP (Plank et al. (2021); openCARP consortium et al. (2023)) for all parameter combinations given in Table 1 and Supplementary Table S2 in Supplementary Material, equaling a total of 82 models. We first initialized our ventricular simulations from a single cell steady state, before further prepacing the entire model across three sinus beats. The single cell steady state was obtained by pacing a single cell 50 times, using the same basic cycle length (BCL) and ionic model as in the full ventricular simulation. Since the value of
We used a BCL of 500 ms as a baseline for our simulations (Table 1). However, to investigate how the simulation results could vary depending on the selected heart rate, we additionally included a set of simulations for BCL of 1,000 ms [see Supplementary Material (Supplementary Table S2)].
2.6.1 Extracellular potential recordings
Throughout our simulations, we recorded the extracellular potential in a point corresponding to the left shoulder. This point was determined by first orienting our ventricular mesh according to a previously generated cardiac mesh with surrounding torso (Uv et al., 2022). A point on the left shoulder (see Supplementary Figure S1) of the torso was then selected manually using ParaView (Ayachit, 2015). The extracellular potential was calculated using the
2.6.2 Definition of reentry
We aimed to investigate conditions which could result in a globalized arrhythmia, rather than a small local reactivation of tissue. To achieve this we recorded a reentry in our simulations as a global reactivation of the myocardium following external stimuli (sinus pacing) or ectopic activity (SAC-induced depolarization). Global reactivation was visually determined as a spike in extracellular potential (Figures 6, 7). In some cases, the activation wave could propagate back into an early repolarized SAC region without subsequent escape into the surrounding myocardium, and consequently, with no visible spike in the recorded extracellular potential. By our definition, these cases were not considered as cases of reentry, as global reactivation did not occur. We classified reentry as sustained if there was still reentrant activity 1,000 ms after the last sinus activation and unsustained otherwise.
2.6.3 Determination of vulnerability windows for reentry and ectopy
To identify potential vulnerability windows for reentry, we varied the delay between the last sinus pacing and the activation of the SAC region in 1 ms intervals. When exploring how the reentrant mechanism and vulnerability window varied as a function of
When investigating the effect of varying size of the SAC region, CV and APD heterogeneity, we used the vulnerability windows identified previously (M1-M7, Table 1) as starting points when searching for reentry. For this purpose, we again varied the SAC onset in steps of 1 ms until either 1) both the beginning and end of a vulnerability window was identified or 2) all time points within the period of 1–350 ms after sinus activation had been investigated. In case 1), we additionally searched within 5 ms on each side of the vulnerability window to check that reentry was not induced within these periods. Furthermore, in models where SAC triggered ectopy through local depolarization (
3 Results
3.1 Effect of SAC reversal potential in single cells
The effect of varying
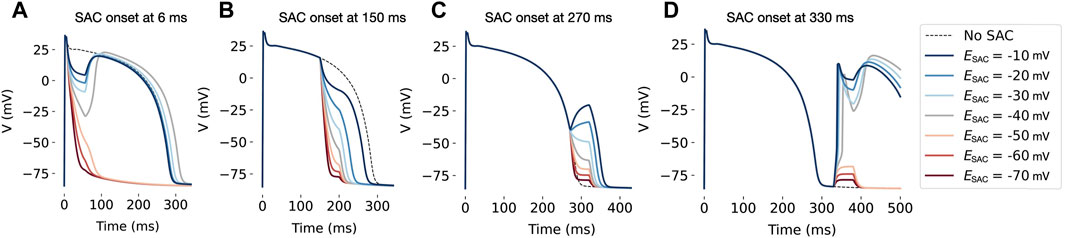
Figure 4. Effect of SAC activation in a single cell, with duration of 50 ms and onset at various time points during the AP. In all models, SAC has a repolarizing effect when applied at 6 ms (A) and 150 ms (B) after AP initiation. When onset is at 270 ms (C), models with
3.2 Effect of SAC reversal potential on reentry in ventricular models
We explored the effect of varying
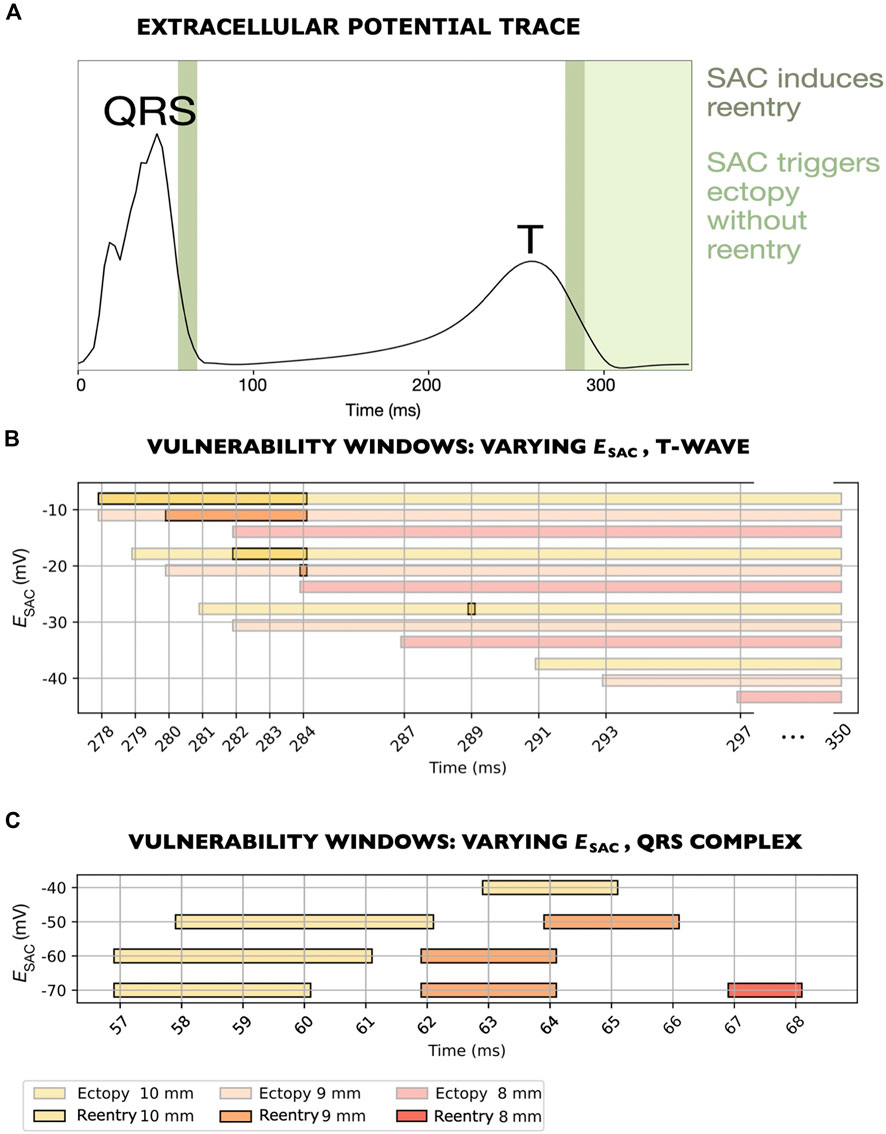
Figure 5. Vulnerability windows for reentry across various levels of
We observed one of the reentrant mechanism in models with
The second mechanism was observed in the remaining models, with
In one of the models where reentry was triggered by early repolarization (
3.3 Effect of size of SAC region
For all values of
Decreasing the SAC radius from 10 to 9 mm also slightly affected which times of SAC onset that triggered ectopy, although the effect was small: The earliest SAC onsets which triggered ectopy were shifted from 279 to 280 ms, from 281 to 282 ms and from 291 to 293 ms in models with
A further decrease in SAC radius from 9 to 8 mm rendered six of the seven models non-inducible to reentry. Only for
3.4 Reentrant mechanisms
3.4.1 SAC activation during the T-wave
The mechanism of reentry caused by local, SAC-induced depolarization in models M1-M3 (Table 1) is illustrated in Figure 6 (M1, Table 1). In sufficiently repolarized tissue and high enough
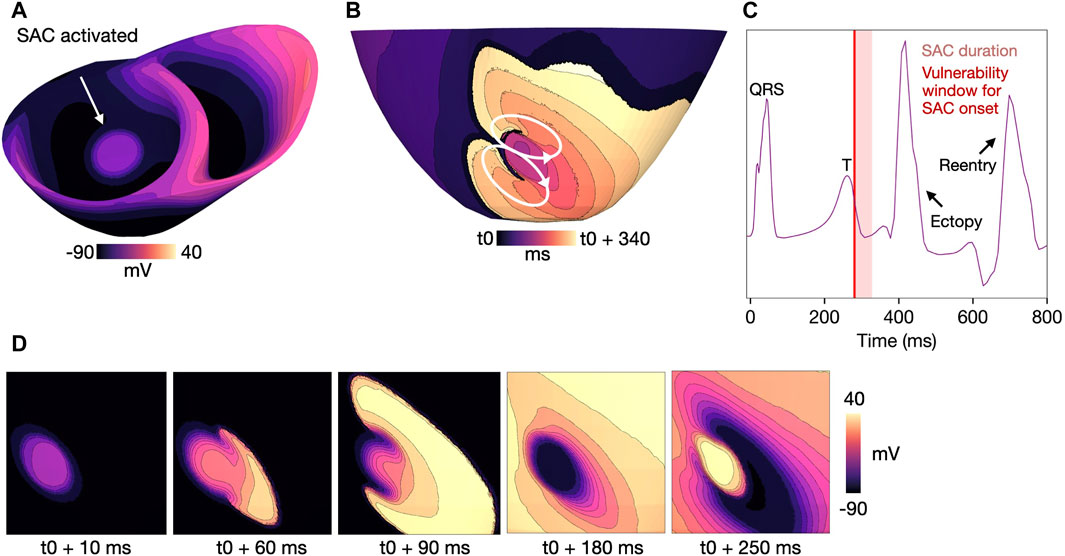
Figure 6. Example reentry in a model with
3.4.2 SAC activation during QRS complex
Reentry was initiated due to early repolarization in the SAC region in models M4-M7 (
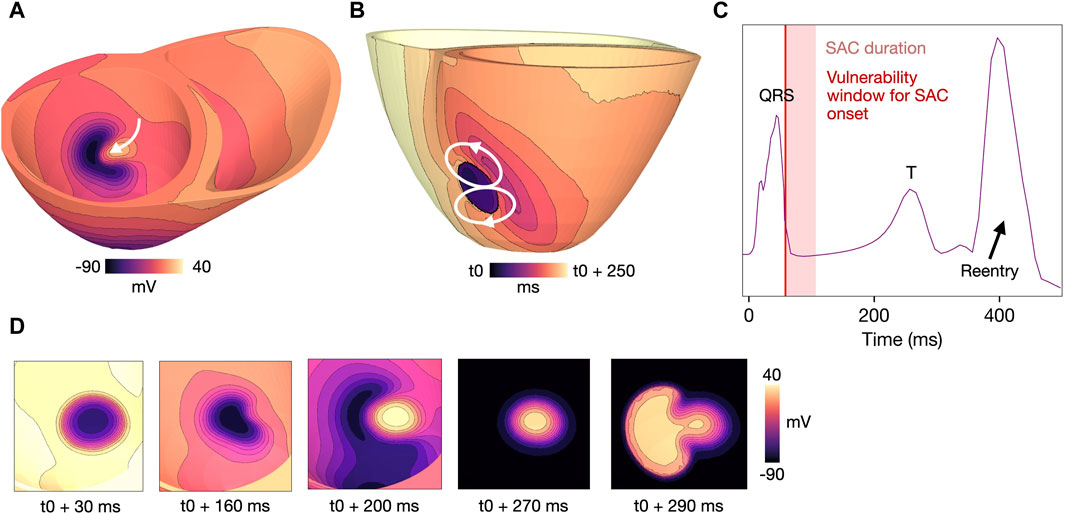
Figure 7. Example reentry for a model with
3.5 Effect of conduction slowing
We investigated how conduction slowing affected reentry due to both SAC-induced depolarization and early repolarization. We performed simulations for all
3.5.1 Global CV slowing
When reducing the CV globally (models
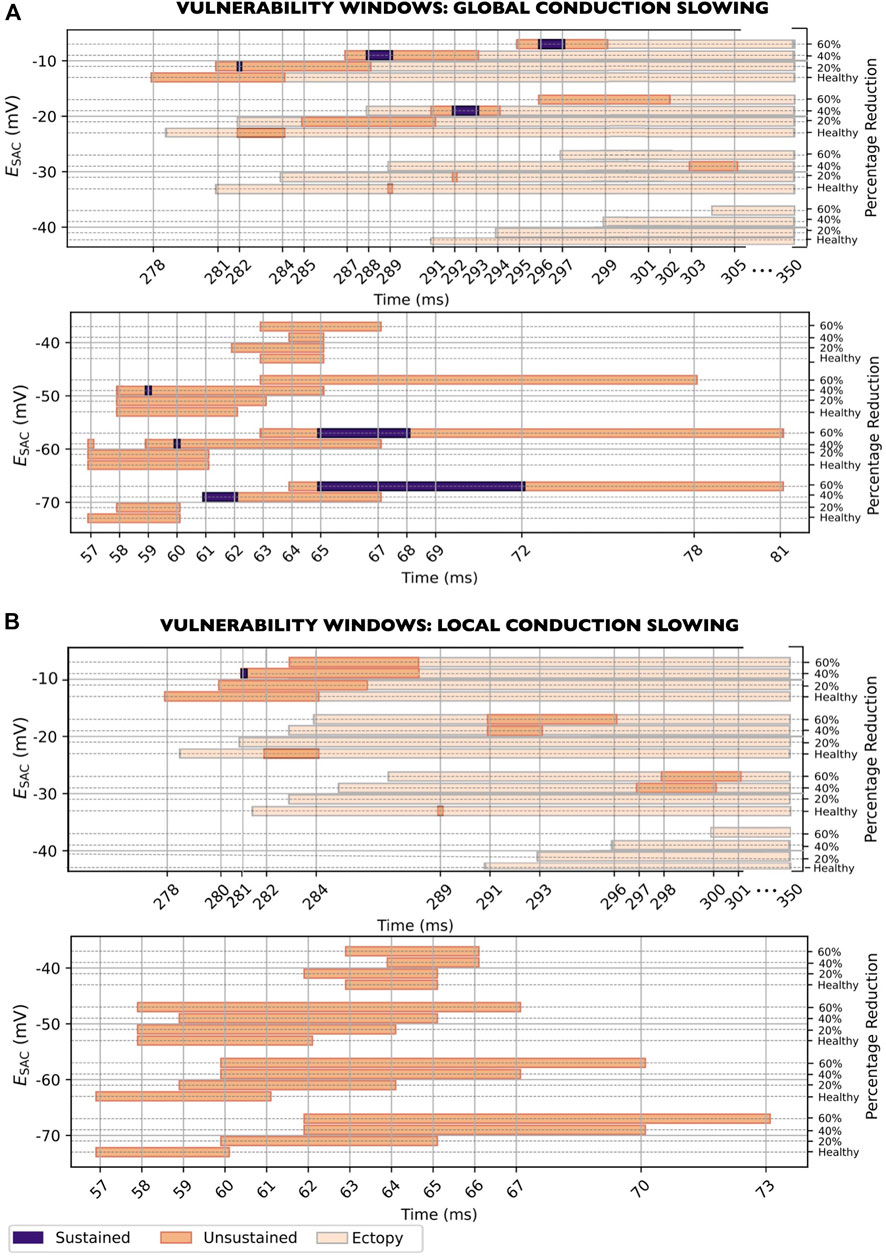
Figure 8. Vulnerability windows for reentry across all levels of
Regarding the width of the vulnerability windows, global CV slowing increased the duration of the vulnerability windows for most
3.5.2 CV slowing in the SAC region
When reducing the CV in the SAC region only (models
The width of the vulnerability windows differed only slightly when changing from healthy CV to maximum CV slowing: from 7 to 6 ms, from 3 to 6 ms, from 1 to 4 ms, from 3 to 4 ms, from 5 to 10 ms, from 5 to 11 ms and from 4 to 12 ms for
3.6 Effect of fibrotic microstructure
To investigate how fibrotic microstructure affected reentry vulnerability, we represented fibrosis in the SAC region using mesh splitting (see Methods and Materials Section 2.4). In these models, 10/96 (10%) reentries were sustained (Figure 9). Figure 9A presents the vulnerability windows for reentry triggered by SAC-induced depolarization. These reentries were observed for
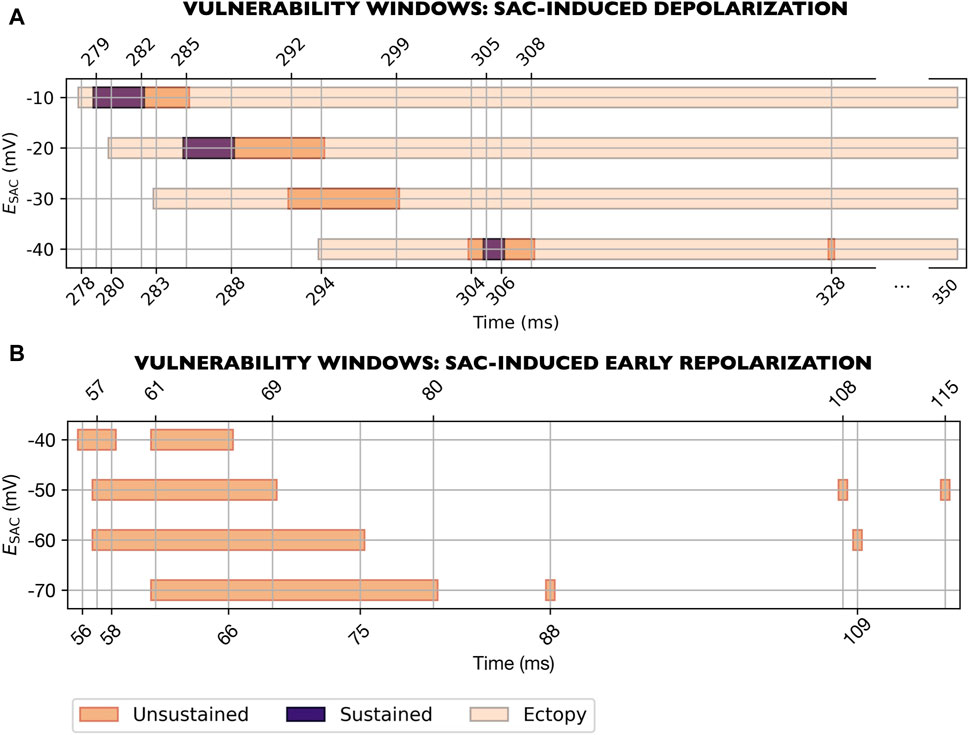
Figure 9. Vulnerability windows for reentry for models with fibrotic microstructure in the SAC region (M15-M21, Table 1). Results for all levels of
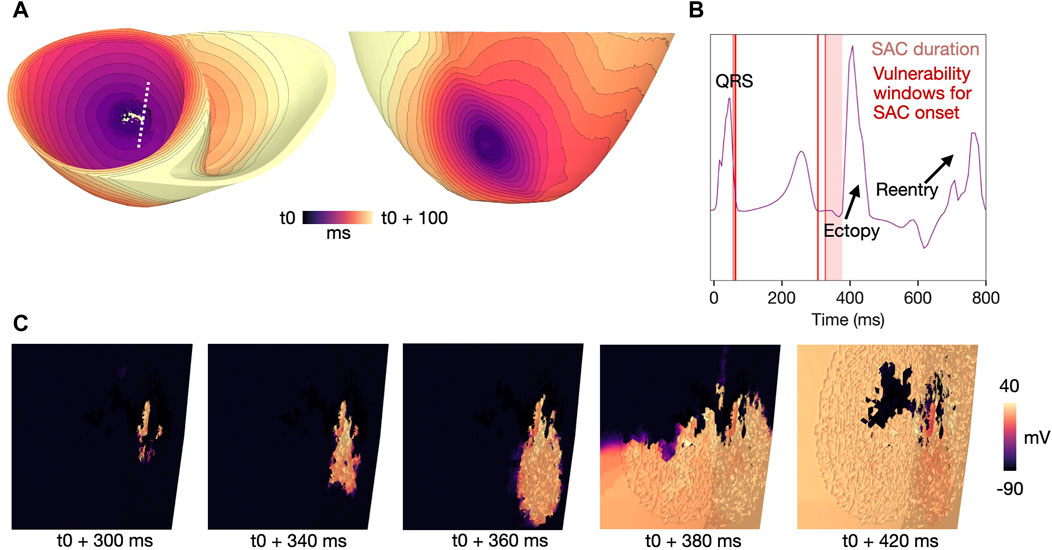
Figure 10. Example of a micro-reentry induced in a model with fibrotic microstructure for
Figure 9B shows the vulnerability windows for reentry triggered by SAC-induced early repolarization. These reentries were observed in models with
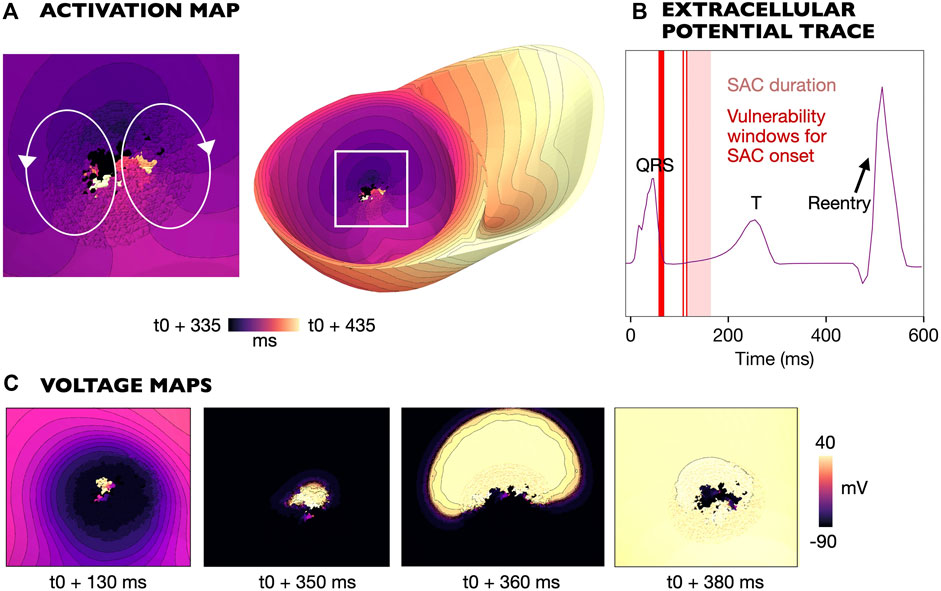
Figure 11. Example of a micro-reentry induced in a model with fibrotic microstructure and
4 Discussion
In the present study, we demonstrated that stretch through SAC activation of the PM insertion region could induce reentry in an image-based model of a patient with MVP and MAD. Two main mechanisms of reentry were identified: 1) SAC-induced depolarization and 2) SAC-induced early repolarization. For both mechanisms of reentry initiation, the activation of SAC could lead to a tissue state wherein the SAC-region was activated whilst the surrounding myocardium was partly repolarized, as illustrated in Figure 6A. From this state, the wavefront within the SAC region could escape and form a reentrant figure-of-eight circuit (Figures 6B, 7B). Additionally, in models with fibrotic microstructure, SAC-induced depolarization and SAC-induced early repolarization could both trigger the formation of micro-reentry originating from the SAC region (Figures 10, 11). To summarize, the key findings of this study are:
In models with
4.1 Timing of stretch and reentry vulnerability window
Timing of the stretch by SAC activation was a key component to whether reentry was induced. The time with respect to the previous sinus activation determines the degree of repolarization within and around the SAC region, and therefore whether a reentrant circuit is able to form. In the present study, the vulnerability windows were short (1–20 ms) and generally coinciding with either the end of the QRS complex (
In swine, ventricular fibrillation could be induced by mechanical impact delivered in the period of 40–1 ms before the peak of the T-wave [Link (2003; 2012)]. During this period, the authors reported a clear increase in impact-induced arrhythmia, even though impacts during the QRS or ST segment could occasionally cause nonsustained ventricular tachycardia. While the above studies point to the T-wave as the most vulnerable period for stretch-induced reentry, we identified vulnerability windows both during the T-wave and the QRS complex. In terms of patients with MVP, further investigation is required to determine the most vulnerable period for arrhythmia induction. Specifically, vulnerability for reentry would depend on potentially patient-specific SAC dynamics and periods of abnormal stretch throughout the cardiac cycle. Patient electrophysiological measurements or strain-profiles (Huttin et al., 2017) may be incorporated into ventricular models in order to move computational investigations on MVP patients from the general to the patient-specific mechanisms of reentry.
Since SAC activate immediately after stretch of the affected tissue (Reed et al., 2014; Quinn and Kohl, 2021), clinically-measured timings of stretch can offer insight as to when SAC are likely to be activated in MVP patients. With respect to the cardiac cycle, abnormal stretch of the PMs and adjacent myocardium have been clinically observed at different time points. Studies have measured a superior shift of the PM tip (Sanfilippo et al., 1992) and an abnormal increase in PM velocity (Han et al., 2010) in the period of mid-to end systole in patients with MVP. In patients presenting with late systolic MVP, reduced PM contraction from early to late systole has been measured (Hei et al., 2019). The findings of Nagata et al. (2023) suggested stretch of the basal myocardium around late-to end systole, an observation hypothesized to be caused by leaflet prolapse and excessive PM traction. Lastly, Huttin et al. (2017) showed that reduced myocardial strain, possibly related to abnormal PM traction, was present in early systole and late-to post systole. Late systole, consistently reported as a period of stretch or reduced contraction of the PM or adjacent myocardium in the above studies, corresponds with our vulnerability window during the T-wave. Sufficient stretch of the PM insertion region during late systole may give rise to the reentrant mechanism we observed (reentry caused by SAC-induced depolarization). Conversely, abnormal motion during early systole as described in the above studies could potentially trigger reentry through early repolarization, corresponding to the vulnerability window during the QRS complex observed in the present study. In our simulations, for both mechanisms of reentry initiation, the first ectopic beat occurred after the T-wave (Figures 6, 7), consistent with ECG recordings of PVCs originating from the PMs in MVP patients (Fulton et al., 2018).
4.2 SAC reversal potential determines reentrant mechanism
SAC are defined as a category of ion channels that open in response to stretch, usually divided into non-selective cation channels (
Ectopy triggered by late systolic or diastolic stretch, known to occur experimentally (Gornick et al., 1986; Zabel et al., 1996; Quinn et al., 2017), was only observed in our models with
In the present study, only models with
In summary, stretch-induced depolarization mechanisms are more concordant with experimental reports in terms of induced ectopic activity and observed vulnerability windows. However, clinical validation of the two mechanisms (both SAC-induced depolarization and SAC-induced early repolarization) as potential triggers of reentry in MVP patients is needed.
4.3 APD heterogeneity and reentry
Previous simulation studies have shown that in a region with partly repolarized tissue, ectopic activity may form a reentrant wave around the temporary conduction block (Garny and Kohl, 2004; Li et al., 2004). Similarly, we found that after SAC activation, the wavefront moved out of the SAC region via the side of the myocardium which repolarized first towards the side repolarizing later, forming a reentrant circuit (Figures 6, 7).
Spatial differences in repolarization times across the myocardium are determined by the local activation times and APDs. Although reports differ in the direction and strength of the measured gradients, the presence of spatial heterogeneity in repolarization has been well documented (Janse et al., 2005; Glukhov et al., 2010; Boukens et al., 2015; Srinivasan et al., 2016). Both individual differences among patients as well as local gradients in various regions of the heart have been observed (Opthof et al., 2017). Repolarization times may also be altered in patients with cardiac disease, including patients with MVP (Digeos-Hasnier et al., 2005; Chauhan et al., 2006). Due to the large variation in reported repolarization gradients, we tested two additional APD gradients (see Materials and Methods) in two example models (
4.4 Effect of size of the SAC region
Imaging and histological studies report a large variation in how the PM base is attached to the ventricular wall (Ranganathan and Burch, 1969; Axel, 2004; Rajiah et al., 2019). The PM itself may take a range of shapes, and both single and multiple PM bases are common (Rajiah et al., 2019). In the present study, reentry was only observed for relatively large SAC regions (
Furthermore, the myocardium surrounding PM insertion may be stretched either as a result of PM traction or related abnormal myocardial motion. Indeed, the region adjacent to the PMs have indeed been shown to experience increased stretch during late systole in patients with MVP, proposedly due to the prolapse of the mitral valve (Nagata et al., 2023). In future studies, clinically-measured strain profiles (Huttin et al., 2017) may be used to more accurately represent the distribution of stretch in MVP patients.
4.5 Effect of fibrosis on reentry
Focal fibrosis in the region of the PMs, here represented by either mesh splitting or local CV slowing in the SAC region, has been linked to PVCs and arrhythmic risk in patients with MVP (Dejgaard et al., 2018; Fulton et al., 2018; Kelley et al., 2022). Others have associated diffuse fibrosis, identified by T1-mapping via CMR imaging, with arrhythmic incidence in MVP patients (Chivulescu et al., 2022). Here, diffuse fibrosis was represented as global CV slowing.
We first investigated how both global and local CV slowing affected reentry inducibility and sustainability. Reducing the CV decreases the wavelength of the propagating wavefront, which is known to promote arrhythmic inducibility (Katz, 2010). On the other hand, decreasing the CV increases the activation delay between different parts of the myocardium, affecting the repolarization gradient, in turn also affecting reentry inducibility. Interestingly, reentry induction did not require the presence of fibrosis in our models, consistent with clinical findings that many MVP patients who have suffered arrhythmia or sudden cardiac death do not show evidence of fibrosis by histology or late gadolinium enhancement on CMR imaging (Muthukumar et al., 2020). Furthermore, while CV slowing generally increased the width of the vulnerability windows, a few models became non-inducible to reentry when CV was reduced (models
Introducing fibrotic microstructure into our models allowed micro-reentries with highly heterogeneous activation patterns to form. These reentries could be triggered by SAC activation outside the QRS complex and T-wave, the two only vulnerable periods identified in models without microstructure. Due to the fibrotic clefts, the activation wave could move slowly through small, partly isolated portions of tissue while the remaining myocardium repolarized. The resulting reentries had a foci-like appearance, originating from the SAC region. Similar propagation patterns have been described in previous studies which modeled fibrotic microstructure using the same mesh splitting approach and which induced reentry using an electric pacing protocol (Balaban et al., 2020; Myklebust et al., 2024).
4.6 Limitations and future work
In this study, we simulated reentry induced by stretch in the PM insertion region. Our model was created from CMR images of an MVP patient. Due to limited image resolution challenging the reconstruction of the PMs, we did not model the PMs explicitly. Rather, we chose to focus on mechanisms of reentry within the ventricular wall. However, future studies should consider inclusion of the PMs, either via image-based modeling or using a simplified geometry such as a Gaussian function.
Reentry in MVP may also be induced via alternative mechanisms to those investigated in this study. In particular, recent clinical work on MVP patients have suggested the presence of myocardial inflammation (Miller et al., 2023), which could increase vulnerability to arrhythmia through its effect on cell membrane dynamics (Bi et al., 2022). Future in silico studies may investigate how pathological changes to ionic membrane currents, including accounting for inflammatory effects, could be involved in arrhythmogenesis in MVP and MAD. In addition, exercise have also been shown to trigger PVCs and nonsustained ventricular tachycardia in MVP patients (Guenancia et al., 2022). Thus, potential effects of exercise on conduction properties and membrane dynamics, including SAC time dependence, could be explored in future studies.
Previous work has shown a relationship between reentry inducibility, myocardial repolarization gradient and PVC origin (Cluitmans et al., 2021). Thus, the effect of the exact location of the PM insertion point may be addressed in future computational studies. Furthermore, PVCs of other origins than the PMs, including the outflow tract and fascicular region, have been reported in MVP patients (Sriram et al., 2013). Stretch-induced depolarization caused by PM traction may also originate from within the PM themselves rather than in the myocardium. While the origin of PVCs have frequently been mapped to the PMs in patients with MVP (Syed et al., 2016; Enriquez et al., 2019; Conti et al., 2023), the precise location is difficult to determine. Traction of the PMs may trigger depolarization anywhere between the PM insertion point and the PM tip. Such activations may give rise to reentrant circuits similar to those we observed or even micro-reentry within the PMs. Since we did not model the PM geometry, reentry occurring within the PMs was not investigated. Whether micro-reentry within the PMs can occur in MVP patients is still an open question, and the potential relation between such reentries and global arrhythmia is an area for future investigation.
Another limitation of the present study is that we chose to only include the electrophysiological effects of stretch rather than to model mechanical deformation directly. The reason for this choice is the high computational demand of simultaneously simulating cardiac mechanics and electrophysiology. Nevertheless, we aimed to look at stretch due to PM traction specifically rather than at global stretch, motivating our choice of simply simulating SAC activation in the PM insertion region.
We exclusively considered reentry induced by a single period of SAC activation. However, abnormal depolarization or repolarization caused by SAC activation may have downstream effects or accumulate across multiple cardiac cycles. Thus, the effects of PM traction across multiple sinus beats remain an area for further research.
Here, we only at looked at a general, non-specific formulation for SAC currents. However, we plan to investigate the potential effects of a more detailed SAC implementation on reentry dynamics in future studies.
We used an LDRB algorithm to assign fiber orientation in our model, and did thus not incorporate patient-specific fiber orientations. Furthermore, vortices of fiber arrangement have been observed at the PM base in experimental studies of the right ventricle (Campanale et al., 2020). The effect of varying fiber orientation at the PM base, including the influence of such fiber vortices is an interesting direction for future computational studies.
Lastly, as our goal was to investigate potential mechanisms of reentry subsequent to SAC activation, the timing of stretch of the PM implemented in this study was systematically varied rather than patient-specific. Future studies may incorporate clinical strain measurements into models of MVP in order to investigate patient-specific mechanisms of stretch and arrhythmia. The effect of other patient-specific features, such as changes in wall thickness or the PM insertion location, could also be explored in silico.
4.7 Conclusion
Stretch of the PM insertion region simulated by SAC-activation could trigger reentrant arrhythmia in a ventricular model of a patient with MVP and MAD. Fibrosis was not required to induce reentry. In models without fibrotic microstructure, we observed one of two mechanisms of reentry initiation depending on
Data availability statement
The raw data supporting the conclusion of this article will be made available by the authors, without undue reservation.
Ethics statement
The studies involving humans were approved by Regionale komiteer for medisinsk og helsefaglig forskningsetikk (REK). The studies were conducted in accordance with the local legislation and institutional requirements. The participants provided their written informed consent to participate in this study.
Author contributions
LM: Conceptualization, Formal Analysis, Investigation, Methodology, Project administration, Software, Visualization, Writing–original draft, Writing–review and editing. GM: Conceptualization, Formal Analysis, Investigation, Visualization, Writing–original draft, Writing–review and editing. GB: Conceptualization, Writing–review and editing. EA: Writing–review and editing, Resources. MR: Writing–review and editing, Resources, Investigation. AC: Resources, Writing–review and editing. NH: Resources, Writing–review and editing. CB: Resources, Writing–review and editing. CF: Resources, Writing–review and editing. KH: Resources, Writing–review and editing. MM: Conceptualization, Funding acquisition, Project administration, Resources, Supervision, Writing–review and editing. HA: Conceptualization, Funding acquisition, Project administration, Supervision, Writing–review and editing.
Funding
The author(s) declare that financial support was received for the research, authorship, and/or publication of this article. LM and HA Research Council of Norway (project number: 303178). MM and HA Simula Research Laboratory, Ministry of Research and Education of Norway, NO. MM and GM ProCardio—Precision Health Center for optimized cardiac care, SFI IV 2020–2028 (project number: 309762).
Acknowledgments
Cardiac simulations for this research were performed on resources provided by Sigma2 - the National Infrastructure for High Performance Computing and Data Storage in Norway and on Fujitsu PRIMERGY CX400M1/CX2550M5 (Oakbridge-CX) provided by The University of Tokyo through Joint Usage/Research Center for Interdisciplinary Large-scale Information Infrastructures and High Performance Computing Infrastructure in Japan (Project ID: jh210021).
Conflict of interest
The authors declare that the research was conducted in the absence of any commercial or financial relationships that could be construed as a potential conflict of interest.
Publisher’s note
All claims expressed in this article are solely those of the authors and do not necessarily represent those of their affiliated organizations, or those of the publisher, the editors and the reviewers. Any product that may be evaluated in this article, or claim that may be made by its manufacturer, is not guaranteed or endorsed by the publisher.
Supplementary material
The Supplementary Material for this article can be found online at: https://www.frontiersin.org/articles/10.3389/fphys.2024.1447938/full#supplementary-material
References
Aabel E. W., Chivulescu M., Dejgaard L. A., Ribe M., Gjertsen E., Hopp E., et al. (2021). Tricuspid annulus disjunction: novel findings by cardiac magnetic resonance in patients with mitral annulus disjunction. Cardiovasc. Imaging 14, 1535–1543. doi:10.1016/j.jcmg.2021.01.028
Aabel E. W., Chivulescu M., Lie Ø. H., Hopp E., Gjertsen E., Ribe M., et al. (2023). Ventricular arrhythmias in arrhythmic mitral valve syndrome—a prospective continuous long-term cardiac monitoring study. Europace 25, 506–516. doi:10.1093/europace/euac182
Anderson K. P., Walker R., Urie P., Ershler P. R., Lux R. L., Karwandee S. V., et al. (1993). Myocardial electrical propagation in patients with idiopathic dilated cardiomyopathy. J. Clin. investigation 92, 122–140. doi:10.1172/JCI116540
Avula U. M. R., Abrams J., Katchman A., Zakharov S., Mironov S., Bayne J., et al. (2019). Heterogeneity of the action potential duration is required for sustained atrial fibrillation. JCI insight 4, e128765. doi:10.1172/jci.insight.128765
Axel L. (2004). Papillary muscles do not attach directly to the solid heart wall. Circulation 109, 3145–3148. doi:10.1161/01.CIR.0000134276.06719.F3
Ayachit U. (2015). The paraview guide: a parallel visualization application. New York, United States: Kitware, Inc.
Balaban G., Costa C. M., Porter B., Halliday B., Rinaldi C. A., Prasad S., et al. (2020). 3d electrophysiological modeling of interstitial fibrosis networks and their role in ventricular arrhythmias in non-ischemic cardiomyopathy. IEEE Trans. Biomed. Eng. 67, 3125–3133. doi:10.1109/TBME.2020.2976924
Basso C., Iliceto S., Thiene G., Perazzolo Marra M. (2019). Mitral valve prolapse, ventricular arrhythmias, and sudden death. Circulation 140, 952–964. doi:10.1161/CIRCULATIONAHA.118.034075
Basso C., Perazzolo Marra M. (2018). Mitral annulus disjunction: emerging role of myocardial mechanical stretch in arrhythmogenesis. J. Am. Coll. Cardiol. 72, 1610–1612. doi:10.1016/j.jacc.2018.07.069
Bayer J., Prassl A. J., Pashaei A., Gomez J. F., Frontera A., Neic A., et al. (2018). Universal ventricular coordinates: a generic framework for describing position within the heart and transferring data. Med. image Anal. 45, 83–93. doi:10.1016/j.media.2018.01.005
Bayer J. D., Blake R. C., Plank G., Trayanova N. A. (2012). A novel rule-based algorithm for assigning myocardial fiber orientation to computational heart models. Ann. Biomed. Eng. 40, 2243–2254. doi:10.1007/s10439-012-0593-5
Bi X., Zhang S., Jiang H., Ma W., Li Y., Lu W., et al. (2022). Mechanistic insights into inflammation-induced arrhythmias: a simulation study. Front. Physiology 13, 843292. doi:10.3389/fphys.2022.843292
Bishop M. J., Plank G. (2011). Bidomain ecg simulations using an augmented monodomain model for the cardiac source. IEEE Trans. Biomed. Eng. 58, 2297–2307. doi:10.1109/TBME.2011.2148718
Boukens B. J., Sulkin M. S., Gloschat C. R., Ng F. S., Vigmond E. J., Efimov I. R. (2015). Transmural apd gradient synchronizes repolarization in the human left ventricular wall. Cardiovasc. Res. 108, 188–196. doi:10.1093/cvr/cvv202
Bui A. H., Roujol S., Foppa M., Kissinger K. V., Goddu B., Hauser T. H., et al. (2017). Diffuse myocardial fibrosis in patients with mitral valve prolapse and ventricular arrhythmia. Heart 103, 204–209. doi:10.1136/heartjnl-2016-309303
Campanale C. M., Scherrer B., Afacan O., Majeed A., Warfield S. K., Sanders S. P. (2020). Myofiber organization in the failing systemic right ventricle. J. Cardiovasc. Magnetic Reson. 22, 49. doi:10.1186/s12968-020-00637-9
Carpentier A., Adams D. H., Filsoufi F. (2010). Carpentier’s reconstructive valve surgery. Elsevier Health Sciences.
Chauhan V. S., Downar E., Nanthakumar K., Parker J. D., Ross H. J., Chan W., et al. (2006). Increased ventricular repolarization heterogeneity in patients with ventricular arrhythmia vulnerability and cardiomyopathy: a human in vivo study. Am. J. Physiology-Heart Circulatory Physiology 290, H79–H86. doi:10.1152/ajpheart.00648.2005
Chivulescu M., Aabel E. W., Gjertsen E., Hopp E., Scheirlynck E., Cosyns B., et al. (2022). Electrical markers and arrhythmic risk associated with myocardial fibrosis in mitral valve prolapse. EP Eur. 24, 1156–1163. doi:10.1093/europace/euac017
Cluitmans M. J., Bear L. R., Nguyên U. C., van Rees B., Stoks J., Ter Bekke R. M., et al. (2021). Noninvasive detection of spatiotemporal activation-repolarization interactions that prime idiopathic ventricular fibrillation. Sci. Transl. Med. 13, eabi9317. doi:10.1126/scitranslmed.abi9317
Conti S., Sabatino F., Caserta C., Sgarito G. (2023). Papillary muscle ventricular ectopy ablation in a malignant mitral valve prolapse: ablation in malignant mitral valve prolapse: ablation in malignant mitral valve prolapse. Echocardiography 40, 271–275. doi:10.1111/echo.15529
Cranford J. P., O’Hara T. J., Villongco C. T., Hafez O. M., Blake R. C., Loscalzo J., et al. (2018). Efficient computational modeling of human ventricular activation and its electrocardiographic representation: a sensitivity study. Cardiovasc. Eng. Technol. 9, 447–467. doi:10.1007/s13239-018-0347-0
[Dataset] openCARP consortium Augustin C., Azzolin L., Bayer J., Bishop M., Boyle P. M., Caforio F., et al. (2023). openCARP. doi:10.35097/1027
Dejgaard L. A., Skjølsvik E. T., Lie Ø. H., Ribe M., Stokke M. K., Hegbom F., et al. (2018). The mitral annulus disjunction arrhythmic syndrome. J. Am. Coll. Cardiol. 72, 1600–1609. doi:10.1016/j.jacc.2018.07.070
Diaz Babio G., Vera Janavel G. L., Carrero C., Masson Juarez G., Mezzadra M., Constantin I., et al. (2020). Papillary muscles. dark side of the heart: a simple approach for a forgotten structure. Echocardiography 37, 993–998. doi:10.1111/echo.14785
Dick D. J., Lab M. J. (1998). Mechanical modulation of stretch-induced premature ventricular beats: induction of mechanoelectric adaptation period. Cardiovasc. Res. 38, 181–191. doi:10.1016/s0008-6363(97)00314-3
Digeos-Hasnier S., Copie X., Paziaud O., Abergel E., Guize L., Diebold B., et al. (2005). Abnormalities of ventricular repolarization in mitral valve prolapse. Ann. noninvasive Electrocardiol. 10, 297–304. doi:10.1111/j.1542-474X.2005.00630.x
Durrer D., Van Dam R. T., Freud G., Janse M., Meijler F., Arzbaecher R. (1970). Total excitation of the isolated human heart. Circulation 41, 899–912. doi:10.1161/01.cir.41.6.899
Enriquez A., Shirai Y., Huang J., Liang J., Briceño D., Hayashi T., et al. (2019). Papillary muscle ventricular arrhythmias in patients with arrhythmic mitral valve prolapse: electrophysiologic substrate and catheter ablation outcomes. J. Cardiovasc. Electrophysiol. 30, 827–835. doi:10.1111/jce.13900
Figliozzi S., Georgiopoulos G., Lopes P. M., Bauer K. B., Moura-Ferreira S., Tondi L., et al. (2023). Myocardial fibrosis at cardiac mri helps predict adverse clinical outcome in patients with mitral valve prolapse. Radiology 306, 112–121. doi:10.1148/radiol.220454
Finsberg H. (2022). Laplace-Dirichlet Rule-Based (LDRB) algorithm for assigning myocardial fiber orientations
Freed L. A., Levy D., Levine R. A., Larson M. G., Evans J. C., Fuller D. L., et al. (1999). Prevalence and clinical outcome of mitral-valve prolapse. N. Engl. J. Med. 341, 1–7. doi:10.1056/NEJM199907013410101
Fulton B. L., Liang J. J., Enriquez A., Garcia F. C., Supple G. E., Riley M. P., et al. (2018). Imaging characteristics of papillary muscle site of origin of ventricular arrhythmias in patients with mitral valve prolapse. J. Cardiovasc. Electrophysiol. 29, 146–153. doi:10.1111/jce.13374
Garny A., Kohl P. (2004). Mechanical induction of arrhythmias during ventricular repolarization: modeling cellular mechanisms and their interaction in two dimensions. Ann. N. Y. Acad. Sci. 1015, 133–143. doi:10.1196/annals.1302.011
Gerach T., Loewe A. (2024). Differential effects of mechano-electric feedback mechanisms on whole-heart activation, repolarization, and tension. J. Physiology. doi:10.1113/JP285022
Geuzaine C., Remacle J.-F. (2009). Gmsh: a 3-d finite element mesh generator with built-in pre-and post-processing facilities. Int. J. Numer. methods Eng. 79, 1309–1331. doi:10.1002/nme.2579
Gillette K., Gsell M. A., Nagel C., Bender J., Winkler B., Williams S. E., et al. (2023). Medalcare-xl: 16,900 healthy and pathological synthetic 12 lead ecgs from electrophysiological simulations. Sci. Data 10, 531. doi:10.1038/s41597-023-02416-4
Glukhov A. V., Fedorov V. V., Lou Q., Ravikumar V. K., Kalish P. W., Schuessler R. B., et al. (2010). Transmural dispersion of repolarization in failing and nonfailing human ventricle. Circulation Res. 106, 981–991. doi:10.1161/CIRCRESAHA.109.204891
Gornick C. C., Tobler H. G., Pritzker M., Tuna I., Almquist A., Benditt D. (1986). Electrophysiologic effects of papillary muscle traction in the intact heart. Circulation 73, 1013–1021. doi:10.1161/01.cir.73.5.1013
Guenancia C., Pace N., Hossu G., Selton-Suty C., Mandry D., Beaumont M., et al. (2022). Prevalence and determinants of pvcs originating from the mitral apparatus in patients with mvp. Clin. Electrophysiol. 8, 526–528. doi:10.1016/j.jacep.2021.12.005
Han Y., Peters D. C., Kissinger K. V., Goddu B., Yeon S. B., Manning W. J., et al. (2010). Evaluation of papillary muscle function using cardiovascular magnetic resonance imaging in mitral valve prolapse. Am. J. Cardiol. 106, 243–248. doi:10.1016/j.amjcard.2010.02.035
Hazim A., Belhamadia Y., Dubljevic S. (2021). A simulation study of the role of mechanical stretch in arrhythmogenesis during cardiac alternans. Biophysical J. 120, 109–121. doi:10.1016/j.bpj.2020.11.018
Hei S., Iwataki M., Jang J.-Y., Kuwaki H., Mahara K., Fukuda S., et al. (2019). Possible mechanism of late systolic mitral valve prolapse: systolic superior shift of leaflets secondary to annular dilatation that causes papillary muscle traction. Am. J. Physiology-Heart Circulatory Physiology 316, H629-H638–H638. doi:10.1152/ajpheart.00618.2018
Heiberg E., Sjögren J., Ugander M., Carlsson M., Engblom H., Arheden H. (2010). Design and validation of segment-freely available software for cardiovascular image analysis. BMC Med. imaging 10, 1–13. doi:10.1186/1471-2342-10-1
Hu H., Sachs F. (1997). Stretch-activated ion channels in the heart. J. Mol. Cell. Cardiol. 29, 1511–1523. doi:10.1006/jmcc.1997.0392
Hu Y., Gurev V., Constantino J., Bayer J. D., Trayanova N. A. (2013). Effects of mechano-electric feedback on scroll wave stability in human ventricular fibrillation. PloS one 8, e60287. doi:10.1371/journal.pone.0060287
Huttin O., Pierre S., Venner C., Voilliot D., Sellal J.-M., Aliot E., et al. (2017). Interactions between mitral valve and left ventricle analysed by 2d speckle tracking in patients with mitral valve prolapse: one more piece to the puzzle. Eur. Heart Journal-Cardiovascular Imaging 18, 323–331. doi:10.1093/ehjci/jew075
Janse M. J., Sosunov E. A., Coronel R., Opthof T., Anyukhovsky E. P., de Bakker J. M., et al. (2005). Repolarization gradients in the canine left ventricle before and after induction of short-term cardiac memory. Circulation 112, 1711–1718. doi:10.1161/CIRCULATIONAHA.104.516583
Jie X., Gurev V., Trayanova N. (2010). Mechanisms of mechanically induced spontaneous arrhythmias in acute regional ischemia. Circulation Res. 106, 185–192. doi:10.1161/CIRCRESAHA.109.210864
Kawara T., Derksen R., de Groot J. R., Coronel R., Tasseron S., Linnenbank A. C., et al. (2001). Activation delay after premature stimulation in chronically diseased human myocardium relates to the architecture of interstitial fibrosis. Circulation 104, 3069–3075. doi:10.1161/hc5001.100833
Keller D. U., Weiss D. L., Dossel O., Seemann G. (2011). Influence of I(Ks) heterogeneities on the genesis of the T-wave: a computational evaluation heterogeneities on the genesis of the t-wave: a computational evaluation. IEEE Trans. Biomed. Eng. 59, 311–322. doi:10.1109/TBME.2011.2168397
Kelley B. P., Chaudry A. M., Syed F. F. (2022). Developing a mechanistic approach to sudden death prevention in mitral valve prolapse. J. Clin. Med. 11, 1285. doi:10.3390/jcm11051285
Kléber A. G., Janse M. J., Fast V. G. (2011). Normal and abnormal conduction in the heart. Compr. Physiol., 455–530. doi:10.1002/cphy.cp020112
Kohl P., Hunter P., Noble D. (1999). Stretch-induced changes in heart rate and rhythm: clinical observations, experiments and mathematical models. Prog. biophysics Mol. Biol. 71, 91–138. doi:10.1016/s0079-6107(98)00038-8
Konofagou E. E., Provost J. (2012). Electromechanical wave imaging for noninvasive mapping of the 3d electrical activation sequence in canines and humans in vivo. J. biomechanics 45, 856–864. doi:10.1016/j.jbiomech.2011.11.027
Kuo C., Reddy C., Munakata K., Surawicz B. (1985). Mechanism of ventricular arrhythmias caused by increased dispersion of repolarization. Eur. Heart J. 6, 63–70. doi:10.1093/eurheartj/6.suppl_d.63
Li W., Kohl P., Trayanova N. (2004). Induction of ventricular arrhythmias following mechanical impact: a simulation study in 3d. J. Mol. histology 35, 679–686. doi:10.1007/s10735-004-2666-8
Link M. S. (2003). Mechanically induced sudden death in chest wall impact (commotio cordis). Prog. biophysics Mol. Biol. 82, 175–186. doi:10.1016/s0079-6107(03)00014-2
Link M. S. (2012). Commotio cordis: ventricular fibrillation triggered by chest impact–induced abnormalities in repolarization. Circulation Arrhythmia Electrophysiol. 5, 425–432. doi:10.1161/CIRCEP.111.962712
Marciniak M., Arevalo H., Tfelt-Hansen J., Jespersen T., Jabbari R., Glinge C., et al. (2016). “From cmr image to patient-specific simulation and population-based analysis: tutorial for an openly available image-processing pipeline,” in International workshop on statistical atlases and computational models of the heart (Springer), 106–117.
Marciniak M. P. (2017). Automatic classification of cardiac disease state from medical image data. Oslo, Norway: University of Oslo (Master’s thesis).
Miller M. A., Adams D. H., Pandis D., Robson P. M., Pawale A., Pyzik R., et al. (2020). Hybrid positron emission tomography/magnetic resonance imaging in arrhythmic mitral valve prolapse. JAMA Cardiol. 5, 1000–1005. doi:10.1001/jamacardio.2020.1555
Miller M. A., Devesa A., Robson P. M., Liao S. L., Pyzik R., El-Eshmawi A., et al. (2023). Arrhythmic mitral valve prolapse with only mild or moderate mitral regurgitation: characterization of myocardial substrate. Clin. Electrophysiol. 9, 1709–1716. doi:10.1016/j.jacep.2023.04.011
Miller M. A., Dukkipati S. R., Turagam M., Liao S. L., Adams D. H., Reddy V. Y. (2018). Arrhythmic mitral valve prolapse: acc review topic of the week. J. Am. Coll. Cardiol. 72, 2904–2914. doi:10.1016/j.jacc.2018.09.048
Moscatelli S., Antonakaki D. (2023). How to assess mitral valve prolapse and mitral annular disjunction, how to look for arrhythmogenic features. Cardiovasc. Genomics Insight.
Muthukumar L., Jahangir A., Jan M. F., Moreno A. C. P., Khandheria B. K., Tajik A. J. (2020). Association between malignant mitral valve prolapse and sudden cardiac death: a review. JAMA Cardiol. 5, 1053–1061. doi:10.1001/jamacardio.2020.1412
Myklebust L., Maleckar M. M., Arevalo H. (2024). Fibrosis modeling choice affects morphology of ventricular arrhythmia in non-ischemic cardiomyopathy. Front. Physiology 15, 1370795. doi:10.3389/fphys.2024.1370795
Nagata Y., Bertrand P. B., Baliyan V., Kochav J., Kagan R. D., Ujka K., et al. (2023). Abnormal mechanics relate to myocardial fibrosis and ventricular arrhythmias in patients with mitral valve prolapse. Circ. Cardiovasc. Imaging 16, e014963. doi:10.1161/CIRCIMAGING.122.014963
Nishimura R. A., McGoon M. D., Shub C., Miller Jr F. A., Ilstrup D. M., Tajik A. J. (1985). Echocardiographically documented mitral-valve prolapse: long-term follow-up of 237 patients. N. Engl. J. Med. 313, 1305–1309. doi:10.1056/NEJM198511213132101
Opthof T., Remme C. A., Jorge E., Noriega F., Wiegerinck R. F., Tasiam A., et al. (2017). Cardiac activation–repolarization patterns and ion channel expression mapping in intact isolated normal human hearts. Heart rhythm. 14, 265–272. doi:10.1016/j.hrthm.2016.10.010
Pak H.-N., Hong S. J., Hwang G. S., Lee H. S., Park S.-W., Ahn J. C., et al. (2004). Spatial dispersion of action potential duration restitution kinetics is associated with induction of ventricular tachycardia/fibrillation in humans. J. Cardiovasc. Electrophysiol. 15, 1357–1363. doi:10.1046/j.1540-8167.2004.03569.x
Plank G., Loewe A., Neic A., Augustin C., Huang Y.-L. C., Gsell M., et al. (2021). The openCARP simulation environment for cardiac electrophysiology. Comput. Methods Programs Biomed. 208, 106223. doi:10.1016/j.cmpb.2021.106223
Pueyo E., Orini M., Rodríguez J. F., Taggart P. (2016). Interactive effect of beta-adrenergic stimulation and mechanical stretch on low-frequency oscillations of ventricular action potential duration in humans. J. Mol. Cell. Cardiol. 97, 93–105. doi:10.1016/j.yjmcc.2016.05.003
Quinn T. A., Jin H., Lee P., Kohl P. (2017). Mechanically induced ectopy via stretch-activated cation-nonselective channels is caused by local tissue deformation and results in ventricular fibrillation if triggered on the repolarization wave edge (commotio cordis). Circulation Arrhythmia Electrophysiol. 10, e004777. doi:10.1161/CIRCEP.116.004777
Quinn T. A., Kohl P. (2011). Mechanical triggers and facilitators of ventricular tachy-arrhythmias. Cardiac mechano-electric coupling Arrhythm., 160–167. doi:10.1093/med/9780199570164.003.0022
Quinn T. A., Kohl P. (2021). Cardiac mechano-electric coupling: acute effects of mechanical stimulation on heart rate and rhythm. Physiol. Rev. 101, 37–92. doi:10.1152/physrev.00036.2019
Rajiah P., Fulton N. L., Bolen M. (2019). Magnetic resonance imaging of the papillary muscles of the left ventricle: normal anatomy, variants, and abnormalities. Insights into imaging 10, 83–17. doi:10.1186/s13244-019-0761-3
Ranganathan N., Burch G. (1969). Gross morphology and arterial supply of the papillary muscles of the left ventricle of man. Am. Heart J. 77, 506–516. doi:10.1016/0002-8703(69)90160-4
Reed A., Kohl P., Peyronnet R. (2014). Molecular candidates for cardiac stretch-activated ion channels. Glob. Cardiol. Sci. Pract. 2014, 19–25. doi:10.5339/gcsp.2014.19
Salvador M., Regazzoni F., Pagani S., Dede' L., Trayanova N., Quarteroni A. (2022). The role of mechano-electric feedbacks and hemodynamic coupling in scar-related ventricular tachycardia. Comput. Biol. Med. 142, 105203. doi:10.1016/j.compbiomed.2021.105203
Sanfilippo A. J., Harrigan P., Popovic A. D., Weyman A. E., Levine R. A. (1992). Papillary muscle traction in mitral valve prolapse: quantitation by two-dimensional echocardiography. J. Am. Coll. Cardiol. 19, 564–571. doi:10.1016/s0735-1097(10)80274-8
Schroeder W., Martin K. M., Lorensen W. E. (1998). The visualization toolkit an object-oriented approach to 3D graphics. Prentice-Hall, Inc.
Srinivasan N. T., Orini M., Simon R. B., Providência R., Khan F. Z., Segal O. R., et al. (2016). Ventricular stimulus site influences dynamic dispersion of repolarization in the intact human heart. Am. J. Physiology-Heart Circulatory Physiology 311, H545–H554. doi:10.1152/ajpheart.00159.2016
Sriram C. S., Syed F. F., Ferguson M. E., Johnson J. N., Enriquez-Sarano M., Cetta F., et al. (2013). Malignant bileaflet mitral valve prolapse syndrome in patients with otherwise idiopathic out-of-hospital cardiac arrest. J. Am. Coll. Cardiol. 62, 222–230. doi:10.1016/j.jacc.2013.02.060
Stacy Jr G. P., Jobe R., Taylor L. K., Hansen D. E. (1992). Stretch-induced depolarizations as a trigger of arrhythmias in isolated canine left ventricles. Am. J. Physiology-Heart Circulatory Physiology 263, H613–H621. doi:10.1152/ajpheart.1992.263.2.H613
Sung E., Prakosa A., Trayanova N. A. (2021). Analyzing the role of repolarization gradients in post-infarct ventricular tachycardia dynamics using patient-specific computational heart models. Front. Physiology 12, 740389. doi:10.3389/fphys.2021.740389
Syed F. F., Ackerman M. J., McLeod C. J., Kapa S., Mulpuru S. K., Sriram C. S., et al. (2016). Sites of successful ventricular fibrillation ablation in bileaflet mitral valve prolapse syndrome. Circulation Arrhythmia Electrophysiol. 9, e004005. doi:10.1161/CIRCEP.116.004005
Ten Tusscher K. H., Panfilov A. V. (2006). Alternans and spiral breakup in a human ventricular tissue model. Am. J. Physiology-Heart Circulatory Physiology 291, H1088–H1100. doi:10.1152/ajpheart.00109.2006
Trayanova N., Li W., Eason J., Kohl P. (2004). Effect of stretch-activated channels on defibrillation efficacy. Heart rhythm. 1, 67–77. doi:10.1016/j.hrthm.2004.01.002
Uv J. J., Myklebust L., Rudsari H. K., Welle H., Arevalo H. (2022). “3d simulations of fetal and maternal ventricular excitation for investigating the abdominal ecg,” in Computational physiology: Simula summer school 2021- student reports (Cham: Springer International Publishing), 13–24.
Wilde A. A., Düren D. R., Hauer R. N., de Bakker J. M., Bakker P. F., Becker A. E., et al. (1997). Mitral valve prolapse and ventricular arrhythmias: observations in a patient with a 20-year history. J. Cardiovasc. Electrophysiol. 8, 307–316. doi:10.1111/j.1540-8167.1997.tb00793.x
Keywords: mitral annulus disjunction, mitral valve prolapse, ventricular reentry, stretch activated channels, computational modeling, cardiac electrophysiology, stretch induced arrhythmia, mechanical stretch
Citation: Myklebust L, Monopoli G, Balaban G, Aabel EW, Ribe M, Castrini AI, Hasselberg NE, Bugge C, Five C, Haugaa K, Maleckar MM and Arevalo H (2024) Stretch of the papillary insertion triggers reentrant arrhythmia: an in silico patient study. Front. Physiol. 15:1447938. doi: 10.3389/fphys.2024.1447938
Received: 12 June 2024; Accepted: 01 August 2024;
Published: 19 August 2024.
Edited by:
T. Alexander Quinn, Dalhousie University, CanadaReviewed by:
Viviane Timmermann, University Heart Center Freiburg, GermanyJason D. Bayer, Université de Bordeaux, France
Frank B. Sachse, The University of Utah, United States
Copyright © 2024 Myklebust, Monopoli, Balaban, Aabel, Ribe, Castrini, Hasselberg, Bugge, Five, Haugaa, Maleckar and Arevalo. This is an open-access article distributed under the terms of the Creative Commons Attribution License (CC BY). The use, distribution or reproduction in other forums is permitted, provided the original author(s) and the copyright owner(s) are credited and that the original publication in this journal is cited, in accordance with accepted academic practice. No use, distribution or reproduction is permitted which does not comply with these terms.
*Correspondence: Hermenegild Arevalo, aGVybWVuZWdpbGRAc2ltdWxhLm5v