- 1Sports Coaching College, Beijing Sport University, Beijing, China
- 2College of Basic Military and Political Education, National University of Defense Technology, Changsha, China
- 3School of Sport Medicine and Rehabilitation, Beijing Sport University, Beijing, China
- 4College of Acupuncture and Tuina and Rehabilitation, Hunan University of Chinese Medicine, Changsha, China
- 5China Institute of Sport and Health Science, Beijing Sport University, Beijing, China
- 6School of Human Sciences (Exercise and Sport Science), University of Western Australia, Perth, WA, Australia
Introduction: We assessed metabolic and hormonal responses to high-load resistance exercise under varying normobaric hypoxia conditions with a saturation clamp.
Methods: Employing a counterbalanced, crossover test design, ten well-trained men participated in three exercise trials with normoxic or hypoxic gas mixtures to maintain arterial oxygen saturation at −90% and 80% [moderate (MH) and severe (SH) hypoxia, respectively]. The resistance exercise regimen comprised five sets of 10 repetitions of barbell back squats at 70% of one repetition maximum, with 1-min rest between sets. Metabolic and hormonal responses were measured before normoxia or hypoxia exposures (Pre 1), 15 min after the exposures (Pre 2), and at 0-, 15-, and 30-min post-exercises (T0, T15, and T30, respectively).
Results: Compared to Pre 2, blood lactate concentrations and growth hormone values were elevated at T0, T15, and T30 (p ≤ 0.001), while testosterone values increased at T0 in all conditions (p ≤ 0.009). Epinephrine values increased significantly from Pre 2 to T0 in SH only (p < 0.001). SH had significantly higher blood lactate concentrations (p = 0.023), growth hormone (p = 0.050), and epinephrine (p = 0.020) values at T30 compared to NM. Cortisol values were elevated above Pre 2 at T15 in MH and SH, while lower testosterone values were noted at T0 and T15 for SH compared to NM and MH (all p ≤ 0.05).
Discussion: Severe simulated hypoxia, achieved through a saturation clamp during barbell back squats, may enhance metabolic and hormonal responses, particularly 30 min post-session. Nevertheless, the acute effects of hypoxia exposure seem to be overridden by the impact of high-load resistance exercise.
Introduction
Engaging in resistance exercise under systemic hypoxia (RTH), with a reduced inspired oxygen fraction (FiO2 0.120–0.160), is a popular intervention for improving muscular strength, hypertrophy, and overall health (Ramos-Campo et al., 2018). Pioneers like Nishimura et al. (2020) demonstrated greater improvements in muscle cross-sectional area and faster strength gains with 6-week moderate-intensity resistance training (70% of one repetition maximum) in hypoxia (FiO2 0.160) compared to normoxia. Subsequent studies (Manimmanakorn et al., 2013; Innes et al., 2016; Yan et al., 2016) support these benefits, although not all research consistently reports such advantages (Ho et al., 2014; Törpel et al., 2020). Limited oxygen availability, leading to metabolic accumulation and cell swelling, is believed to preferentially recruit type II muscle fibers, potentially influencing training adaptations (Scott et al., 2016). Moreover, acute hypoxia, as a stressor, can elevate the production of hormones crucial for regulating metabolic and anabolic processes (Athanasiou et al., 2023).
Research on endocrine responses to acute resistance exercise in hypoxia has produced mixed findings. Some studies report higher levels of lactate, growth hormone and/or cortisol during the 0–60 min post-exercise period in hypoxia compared to normoxia (Kon et al., 2010; Kon et al., 2012; Filopoulos et al., 2017), while others show no significant differences (Ho et al., 2014). These inconsistencies may stem from differences in resistance training protocols such as the load lifted and exercise structure, as well as the range of FiO2 levels applied (Timon et al., 2022). Exposure to systemic hypoxia introduces notable inter-individual variability (Chapman et al., 1998). For example, exposure to extreme hypoxia (FiO2 0.110) for 4 h led to a significant decrease in arterial oxygen saturation (SpO2) in all participants, with individual SpO2 drops ranging from 12% to 36% (D’Hulst et al., 2013). Consequently, relying on a fixed FiO2 as a marker of hypoxic dose may result in considerable inter-individual variability in metabolic and hormonal hypoxia responses (Soo et al., 2020).
A solution to this problem is the saturation clamp approach (Soo et al., 2020), which requires adjusting the FiO2 for each individual to maintain SpO2 at a target level. This method has been successfully employed in RTH literature. For instance, Manimmanakorn et al. (2013) implemented a 5-week training RTH program for netball athletes, employing low-loads (thrice weekly, three sets of knee extension and flexion to failure at 20% 1RM, FiO2 adjusted to maintain SpO2 at −80%). The RTH group exhibited a higher increase in repetitions at 20% 1RM compared to the normoxic training control. While most studies using a saturation clamp have focused on comparing physical performance, erythropoietin expression, and body composition between young and older adults using a single hypoxic dose of ∼80–85% (Törpel et al., 2019; Törpel et al., 2020), this approach has not been widely applied to investigate acute metabolic and hormonal responses to resistance exercise in varying levels of hypoxia.
The aim of this study was to evaluate the acute metabolic and hormonal responses to high-load resistance exercise under varying levels of normobaric hypoxia, employing a SpO2 clamp approach. We hypothesized that the most severe hypoxic condition would lead to increased blood lactate accumulation and anabolic hormone responses.
Methods
Participants
Ten well-trained men (mean age 21.2 ± 2.3 years; height 178.4 ± 2.7 cm; body weight 69.8 ± 6.3 kg), all experienced in resistance exercise, participated in the study. Participants were recruited from local sporting clubs and had a minimum of 2 years of resistance training experience. They were classified as “Trained/Development” (Tier 2) using established criteria (McKay et al., 2022). Throughout the testing phase, none of the participants were using substances (e.g., anabolic steroids, creatine, sympathoadrenal drugs) that could potentially impact the study results. During the study, participants resided at sea level in Beijing (China), and reported no exposure to altitudes above 1,500 m within 6 months before the experimental trials. The study was approved by Beijing Sport University Review Board for Human Participants (no. 2017004A), with written informed consent obtained from participants.
Study design
The study employed a single-blind, counterbalanced, crossover test design. Initially, participants underwent a preliminary session in normoxia, where they familiarized themselves with the correct technique for performing a barbell back squat and determined their one-repetition maximum mean (139.8 ± 16.3 kg) (Yan et al., 2016). Subsequently, participants completed three separate experimental sessions, during which they performed barbell back squats while inhaling either a normoxic (normoxia) or hypoxic gas mixture. In the hypoxic conditions, SpO2 was controlled at −90% and 80% for moderate (MH) and severe hypoxia (SH), respectively. Each session was separated by at least 2 weeks and conducted at the same time of day (8.30–11.30 a.m.) for each participant.
Experimental sessions
After an overnight fast, participants arrived at the laboratory and rested in a seated position for 30 min before the first blood collection (Pre 1) outside the environmental chamber while breathing room air (i.e., before exposure to normoxic or hypoxic gas mixtures). They then entered the environmental chamber, rested (i.e., sitting position) for an additional 15 min with exposure to either normoxic or hypoxic gas, and had the second capillary blood sample taken (Pre 2). Afterwards, participants commenced with a warm-up set of 10 repetitions of barbell back squats at 40% of 1RM, followed by the main exercise consisting of five sets of 10 repetitions of barbell back squats at 70% of 1RM (97.8 ± 11.4 kg), with a 1-min rest period between sets. During the squats, they were instructed to achieve a knee angle of 90° and were assisted if they experienced fatigue during the final repetitions. Three additional blood samples were collected immediately (T0), 15 (T15), and 30 min (T30) after completing the resistance exercise. After the initial blood sample (Pre 1), participants remained in the testing environment until the final blood sample was collected. The exposures continued until the experimental trial ended, at which points participants left the environmental chamber. Participants could consume water ad libitum throughout the session.
Saturation clamping
Participants were exposed to either normoxic or normobaric hypoxic air in an environmental chamber (L.O.S. LOWOXYGEN SYSTEMS GmbH, Germany). The “background” FiO2 inside the chamber was set at 0.209 for normoxic air (NM) and 0.166 for both MH and SH conditions. Participants also inhaled a gas mixture delivered by a hypoxic generator (Hypoxico, Inc. New York, United States), as adjusting FiO2 from this device has a faster response rate on physiological markers including SpO2 than an environmental chamber. Instead of fixing a specific simulated altitude or FiO2 for the hypoxic conditions, SpO2 was clamped at −90% and −80% in MH and SH, respectively. This was achieved by continuously adjusting the FiO2 individually during each trial, following a manual procedure that was piloted in advance. Continuous monitoring of SpO2 was conducted using a pulse oximeter (WristOx23150; Nonin Medical, Inc., United States) placed on the forefinger of each participant. For blinding purposes, participants consistently breathed through the same set-up, including during normoxia.
Blood sampling and analysis
Blood samples were drawn from an antecubital vein through an intravenous catheter at several time points: in normoxia (Pre 1), 15 min after exposure to either normoxia or hypoxia at rest (Pre 2), as well as immediately after, 15 and 30 min after exercise (T0, T15 and T30, respectively). Participants remained in the testing environment until the final blood sample was collected. After collection, the serum was separated by centrifugation at 5,000 rpm for 5 min and stored at −80°C until analysis. The storage time from collection to analysis was within 72 h.
Commercial test kits were used to measure the concentrations of growth hormone (Access Ultrasensitive hGH assay; Catalog No. 33580; Beckman Coulter, Inc., Brea, CA, United States), testosterone (Access Testosterone; Catalog No. 33560; Beckman Coulter, Inc., Brea, CA, United States) and cortisol (Access Cortisol; Catalog No. 33600; Beckman Coulter, Inc., Brea, CA, United States) using an automatic immunoassay system (Unicel DXI 800; Beckman Coulter, Inc., United States). The epinephrine concentration was determined using an enzyme immunoassay kit (CatCombi ELISA; Catalog No. RE59242; IBL International GmbH, Germany), while blood lactate concentration was measured using an automatic lactate analyzer (Biosen C-line; EKF diagnostic GmbH, Germany).
Statistical analysis
All statistical analyses were conducted using IBM SPSS Statistics for Windows, version 25.0 (IBM Corp., Armonk, NY, United States). Descriptive statistics are presented as mean ± SD. To evaluate the effects of high-load resistance exercise under varying normobaric hypoxia conditions on metabolic and hormonal responses, we employed generalized estimating equations (GEE) for each outcome variable. GEE was chosen for its robustness in handling repeated measures and correlated data inherent in our crossover study design. Each GEE model included a single metabolic or hormonal response variable as the dependent variable, with oxygen condition (NM, MH, and SH), time points (Pre 1, Pre 2, T0, T15, and T30), and their interaction as the main independent variables. An exchangeable correlation structure was used to account for repeated measures within participants, assuming equal correlation between all time points. The Pre 2 value was included as a covariate to control for its influence on the outcome variables. To assess the effectiveness of the SpO2 clamping procedure, we analyzed SpO2 data using a GEE model with SpO2 as the dependent variable, and the same independent variables (oxygen condition and time points) and their interaction. The Pre 1 value was included as a covariate. An exchangeable correlation structure was also applied. Post-hoc analyses were performed using the least significant difference (LSD) method to adjust for multiple comparisons following the GEE analysis. Statistical significance was set at p ≤ 0.05.
Results
Figure 1 demonstrates the effectiveness of the SpO2 clamping procedure. Compared to Pre 2, blood lactate concentrations and growth hormone values were elevated at T0, T15, and T30 (p ≤ 0.001; Figure 2), while testosterone values increased at T0 in all conditions (p ≤ 0.009; Figure 3). Epinephrine values increased significantly from Pre 2 to T0 in SH only (p < 0.001). Additionally, SH had significantly higher blood lactate concentrations (p = 0.023), growth hormone (p = 0.050), and epinephrine (p = 0.020) values at T30 compared to NM. Cortisol values were elevated above Pre 2 at T15 in MH (p = 0.007) and SH (p = 0.034). Lower testosterone values were noted at T0 and T15 for SH compared to NM and MH (p ≤ 0.023).
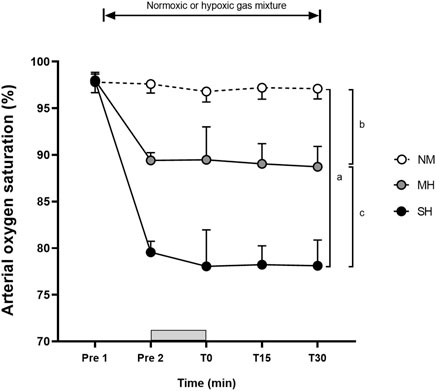
Figure 1. Arterial oxygen saturation levels before and following resistance exercise while inhaling either normoxic (NM) or hypoxic gas mixtures with controlled arterial oxygen saturation of −90% and 80% for moderate (MH) and severe hypoxia (SH), respectively. Mean ± SD (n = 10). Arterial oxygen saturation was obtained before exposure to normoxia or hypoxia (Pre 1), 15 min after exposure (Pre 2), as well as immediately after, 15 and 30 min after exercise (T0, T15, and T30, respectively), while participants remained in the testing environment. The Gy bar indicates the resistance exercise period. a, b, and c significant difference NM vs. SH, NM vs. MH, and MH vs. SH, respectively (p < 0.05).
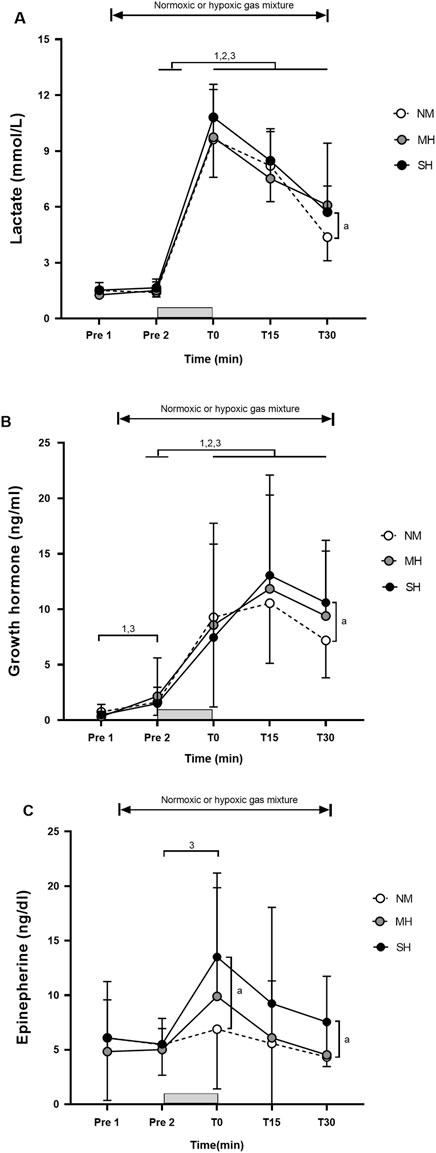
Figure 2. Blood lactate concentration (A), growth hormone (B), and epinephrine (C) before and following resistance exercise while inhaling either normoxic (NM) or hypoxic gas mixtures with controlled arterial oxygen saturation of −90% and 80% for moderate (MH) and severe hypoxia (SH), respectively. Mean±SD (n = 10). Arterial oxygen saturation was obtained before exposure to normoxia or hypoxia (Pre 1), 15 min after exposure (Pre 2), as well as immediately after, 15 and 30 min after exercise (T0, T15, and T30, respectively), while participants remained in the testing environment. The gray bar indicates the resistance exercise period. 1, 2, and 3 significantly different between time points for NM, MH and SH, respectively (p < .05). a significant difference NM vs. SH (p < 0.05).
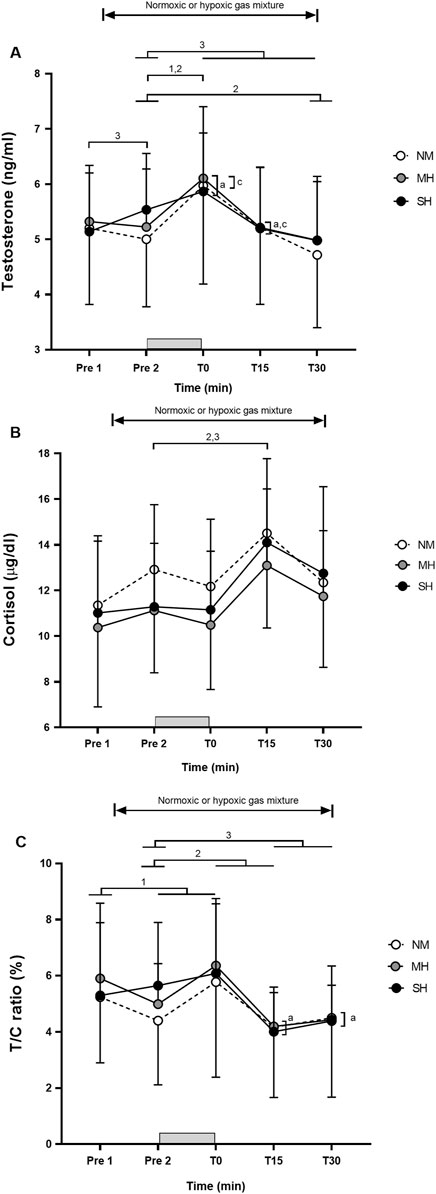
Figure 3. Testosterone (A), cortisol (B), and testosterone/cortisol ratio (C) before and following resistance exercise while inhaling either normoxic (NM) or hypoxic gas mixtures with controlled arterial oxygen saturation of −90% and 80% for moderate (MH) and severe hypoxia (SH), respectively. Mean ± SD (n = 10). Arterial oxygen saturation was obtained before exposure to normoxia or hypoxia (Pre 1), 15 min after exposure (Pre 2), as well as immediately after, 15 and 30 min after exercise (T0, T15, and T30, respectively), while participants remained in the testing environment. The gray bar indicates the resistance exercise period. 1, 2, and 3 significantly different between time points for NM, MH and SH, respectively (p < 0.05). a and c significant difference NM vs. SH and MH vs. SH, respectively (p < 0.05).
Discussion
High-load resistance exercise supersedes the acute effects of hypoxia exposure
In partial agreement with our hypothesis, it was observed that SH, but not MH, during high-load resistance exercise using a saturation clamp may slightly enhance metabolic and hormonal responses, particularly 30 min post-session, compared to normoxia. This finding occurred despite employing brief inter-set rest periods (1 min), high-load resistance exercise (70% of one repetition maximum) that recruited large muscle mass (barbell back squats), substantial exercise volume (five sets of 10 repetitions), and providing a significant hypoxic stimulus for all participants (Ramos-Campo et al., 2018). Notably, resting plasma growth hormone levels were previously found to increase only following hypoxic resistance training in severe hypoxia (FiO2 0.136), with no such increase noted in moderate hypoxia (FiO2 0.158) or normoxia (Namboonlue et al., 2020). Our findings align with the study by Ho et al. (2014), who reported that low-intensity squat exercise (30% of 1RM) performed under mild simulated hypoxia (FiO2 0.150; SpO2 −92%) did not induce significantly greater hormonal responses in growth hormone, total testosterone, and cortisol than normoxia. However, other studies have shown that performing bench press and leg press at 50%–85% of 1RM in severe normobaric hypoxic conditions (FiO2 0.130; SpO2 ∼82–85%) induced larger blood lactate accumulation as well as greater growth hormone and epinephrine responses than in the normoxic condition. Interestingly, other anabolic hormones such as testosterone or cortisol remained unchanged (Kon et al., 2010; Kon et al., 2012). Despite achieving consistent SpO2 values between conditions through our saturation clamp approach, high-load resistance exercise likely supersedes the acute effects of hypoxia exposure in well-trained men.
Concomitance between metabolic and hormonal responses
Overall, the magnitude and time course of changes in metabolic and hormonal responses during the 30-min post-exercise period were consistent with prior studies using fixed FiO2 levels ranging from 0.126 to 0.160 (Kon et al., 2010; Kon et al., 2012; Yan et al., 2016). The stimulation of growth hormone secretion during exercise is believed to be influenced by the accumulation of metabolic by-products, such as lactate or H+ (Gordon et al., 1994). The acidic muscle environment may stimulate sympathetic nerve activity through chemoreceptive reflexes (Victor and Seals, 1989), leading to increases in growth hormone, norepinephrine, and lactate levels (Buresh et al., 2009). However, in several studies (Kurobe et al., 2015; Yan et al., 2016; Namboonlue et al., 2020), changes in blood lactate concentrations did not fully explain the observed changes in growth hormone. Resting plasma growth hormone levels, for instance, were elevated only following hypoxic resistance training in severe hypoxia (FiO2 0.136), with no increase observed in moderate hypoxia (FiO2 0.158) or in normoxia (Namboonlue et al., 2020). In our study, blood lactate concentrations peaked at T0, while growth hormone values were highest at T15 in all conditions. Resistance training predominantly targets type II muscle fibers, associated with a higher release of lactic acid and growth hormone (Schoenfeld, 2010). However, the mechanisms underlying growth hormone production and release during and after hypoxic resistance training remain uncertain, and the precise role of growth hormone in this context is not fully understood. The significantly higher blood lactate concentrations, growth hormone, and epinephrine values at T30 in SH compared to NM in our study may suggest an alteration in muscle fiber recruitment (shifting from predominantly Type I to Type II) in the more severe hypoxic condition. This indirectly supports the notion of a slightly heightened anabolic response associated with RTH compared to equivalent training in normoxia due to a greater accumulation of metabolic by-products (Scott et al., 2015a).
Variability in metabolic and hormonal responses
Despite controlling SpO2 experimentally, participants showed significant variability in hormonal responses to acute resistance exercise, with or without hypoxia. This variability in post-exercise blood lactate concentration, growth hormone, and other hormonal responses across all time points and conditions is likely influenced by factors beyond hypoxia exposure. This is reinforced by the observation that growth hormone increased significantly from Pre 1 to Pre 2 in both SH and NM conditions, but not in MH; however, the changes with 15 min of passive hypoxic exposure are likely not clinically relevant. Testosterone increased only in SH. Higher, though not statistically significant, testosterone values were previously observed 15 min after exposure to both normoxic and hypoxic (FiO2 0.130) mixtures (Kon et al., 2012). Individual differences in hypoxic tolerance, genetics, and baseline fitness levels are probably contributors (Deldicque, 2022). Practitioners should recognize substantial between-individual differences in both the magnitude of adjustments to a resistance exercise protocol and responses to a given hypoxic stimulus. While the exact cause of this variability is beyond the scope of this study, our results underscore that the potential for hypoxia to enhance hormonal responses to resistance exercise is highly individualized, with some participants exhibiting stronger responses than others, even with a saturation clamp approach.
Arterial oxygen saturation
In our study, employing a saturation clamp, hypoxic exposure increased SpO2 response variability between participants compared to NM, particularly at T0, and to a lesser extent at both T15 and T30. Visual inspection of Figure 1 also suggests a comparable magnitude between MH and SH. Taken together, this suggests that exercise, in addition to hypoxia exposure, might have influenced SpO2 readings, especially when monitored from the finger where blood flow may have been limited during squatting. During back squat and deadlift exercises, prior observations noted SpO2 dropping from 95% in normoxia to −90% in moderate hypoxia (FiO2 0.160) and −75% in severe hypoxia (FiO2 0.130), with greater variability observed in the latter (Scott et al., 2015b). Similar to this study, the prevailing method for controlling SpO2 during hypoxic exposure involves manual adjustments to the individual’s FiO2 (Mira et al., 2021). One limitation is that the frequency and magnitude of manual adjustments in FiO2 depend on the discretion of investigators, introducing potential inter-rater reliability issues. To eliminate potential inter-rater differences from the manual approach, FiO2 adjustments were conducted by a single experimenter familiar with the equipment to minimize bias. With technological advancements, automatic FiO2 adjustments for SpO2 clamping are likely to be more reliable and accurate. Supporting this, automatic methods for heart rate clamping are more accurate than manual approaches (e.g., power output adjustments every 30 s by 0.5 or 10 W) during submaximal intensity continuous cycling (Li et al., 2024). Others have employed automatic saturation clamps by the hypoxicator using a biofeedback control system (Manimmanakorn et al., 2013; Törpel et al., 2019; Törpel et al., 2020), but data about the accuracy and reliability of this practice are lacking. Therefore, a more robust approach involving real-time and continuous adjustments to SpO2 is needed for achieving precise saturation clamps that can be practically applied.
Limitations and additional considerations
Several methodological considerations should be taken into account when interpreting our findings. Firstly, with the exception of one study in professional rugby players (Mayo et al., 2018) and one involving female netballers (Manimmanakorn et al., 2013), all RTH studies conducted so far focused on untrained participants or recreationally trained athletes (Deldicque, 2022). This limits the generalizability of our findings, particularly to individuals with different demographics, where gender differences in the endocrine and metabolic responses to hypoxic exercise have been noted (Raberin et al., 2023).
Secondly, substantial heterogeneity exists in the applied exercise stimulus across the RTH literature, encompassing differences in lifting velocity, load, and rest periods. For instance, benefits have been observed using loads ranging from 20% to 90% of 1RM (Deldicque, 2022; Benavente et al., 2023). Our study, like some others, utilized a moderate-load hypertrophy-based training prescription (e.g., 4 sets of 10 repetitions with 70% 1RM and 60 s rest; Nishimura et al., 2020). On the other hand, other studies incorporated heavy strength training sessions focusing on maximal strength (e.g., 2–4 sets of 3–6 repetitions with >75% 1RM and 180 s inter-set rest; Innes et al., 2016). There are also instances of light-load exercises seemingly targetting muscular endurance development (e.g., 3 sets to failure with 20% 1RM and 30 s rest; Manimmanakorn et al., 2013). Future studies employing different exercise structures should investigate whether more severe hypoxia could acutely enhance metabolic and hormonal responses compared to lower severities or normoxia. Another key methodological consideration is that our design, based on previous studies (Kon et al., 2012; Kon et al., 2021), required participants to remain in the chamber post-exercise, facilitating comparison with existing literature. Different hormonal responses might have occurred if participants had not stayed in the environment for 30 min after exercise until the final blood sample was collected. Caution is needed when generalizing our findings to conditions where the post-RTH recovery period is consistently performed in normoxia.
Thirdly, a crucial aspect in designing RTH programs is the metabolic stress created (Deldicque, 2022). Hypoxia fundamentally reduces oxygen availability, acutely altering metabolic processes essential for energy production. Due to the absence of near-infrared spectroscopy measurements in our study, it is challenging to ascertain whether severer hypoxia severities are more effective in causing a greater decline in muscle oxygenation during resistance exercise (Girard et al., 2022). Importantly, Benavente et al. (2023) found no significant effects of moderate (FiO2 0.143–0.160) versus severe (FiO2 = <0.142–0.110) hypoxia on RTH efficacy. Therefore, exercise programs should be tailored to leverage the metabolic challenge posed by hypoxia. While our study only modified SpO2 levels, practitioners should always consider the hypoxic dose along with the exercise stimulus demands when planning RTH programs. While beneficial effects of hypoxia over normoxia have been found with intensities ranging from 20% to 90% of 1-RM, moderate-load exercise (70% of 1-RM) with inter-set rest periods of 1 min and FiO2 inducing SpO2 levels ranging from 80% to 90% follows current recommendations for structuring RTH workouts (Deldicque, 2022). Considering exercise to failure as an additional variable, distinct from other exercise structure elements (e.g., inter-set recovery period, load lifted) or the severity of hypoxia, may amplify metabolic stress. It can not be ruled out that performing the final set or sets of the back squat exercise to failure could have generated additional metabolic stress (Deldicque, 2022), potentially resulting in more pronounced differences between conditions.
Finally, the implementation of high-load circuit-style resistance training in hypoxia emerges a promising RTH approach (Martinez-Guardado et al., 2019; Ramos-Campo et al., 2018). However, this intervention encounters challenges, especially when training large groups simultaneously in a climatic chamber or hypoxic tent. To date, the practice of surreptitiously adjusting FiO2 levels for a consistent reduction in SpO2 requires participants to wear a facemask connected to a hypoxicator. Therefore, the application of RTH using a saturation clamp is currently confined to individuals executing isolated localized or whole-body movements. Analogous to the automatic heart rate clamp approach during aerobic exercise (Li et al., 2023), technological development is essential to automatically control the internal load of resistance exercise (i.e., SpO2 levels or muscle oxygenation status using near-infrared spectroscopy) across various hypoxic conditions.
Conclusion
Achieving sever hypoxia through a saturation clamp during barbell back squats may enhance metabolic and hormonal responses, especially 30 min after the session. Nevertheless, the impact of high-load resistance exercise appears to surpass the acute effects of hypoxia exposure in well-trained men.
Data availability statement
The raw data supporting the conclusions of this article will be made available by the authors, without undue reservation.
Ethics statement
The studies involving humans were approved by Beijing Sport University Review Board for Human Participants (No. 2017004A). The studies were conducted in accordance with the local legislation and institutional requirements. The participants provided their written informed consent to participate in this study.
Author contributions
GJ: Data curation, Formal Analysis, Software, Writing–original draft, Writing–review and editing. SQ: Data curation, Formal Analysis, Software, Writing–original draft, Writing–review and editing. BY: Conceptualization, Investigation, Writing–review and editing. OG: Formal Analysis, Writing–review and editing.
Funding
The author(s) declare that no financial support was received for the research, authorship, and/or publication of this article.
Acknowledgments
The authors thank the participants for their dedication, commitment, and cooperation with this study.
Conflict of interest
The authors declare that the research was conducted in the absence of any commercial or financial relationships that could be construed as a potential conflict of interest.
Publisher’s note
All claims expressed in this article are solely those of the authors and do not necessarily represent those of their affiliated organizations, or those of the publisher, the editors and the reviewers. Any product that may be evaluated in this article, or claim that may be made by its manufacturer, is not guaranteed or endorsed by the publisher.
References
Athanasiou N., Bogdanis G. C., Mastorakos G. (2023). Endocrine responses of the stress system to different types of exercise. Rev. Endocr. Metab. Disord. 24, 251–266. doi:10.1007/s11154-022-09758-1
Benavente C., Schoenfeld B. J., Padial P., Feriche B. (2023). Efficacy of resistance training in hypoxia on muscle hypertrophy and strength development: a systematic review with meta-analysis. Sci. Rep. 13 (1), 3676. doi:10.1038/s41598-023-30808-4
Buresh R., Berg K., French J. (2009). The effect of resistive exercise rest interval on hormonal response, strength, and hypertrophy with training. J. Strength Cond. Res. 23 (1), 62–71. doi:10.1519/JSC.0b013e318185f14a
Chapman R. F., Stray-Gundersen J., Levine B. D. (1998). Individual variation in response to altitude training. J. Appl. Physiol. 85 (4), 1448–1456. doi:10.1152/jappl.1998.85.4.1448
Deldicque L. (2022). Does normobaric hypoxic resistance training confer benefit over normoxic training in athletes? A narrative review. J. Sci. Sport Exerc 4, 306–314. doi:10.1007/s42978-021-00159-5
D'Hulst G., Jamart C., Van Thienen R., Hespel P., Francaux M., Deldicque L. (2013). Effect of acute environmental hypoxia on protein metabolism in human skeletal muscle. Acta Physiol. 208 (3), 251–264. doi:10.1111/apha.12086
Filopoulos D., Cormack S. J., Whyte D. G. (2017). Normobaric hypoxia increases the growth hormone response to maximal resistance exercise in trained men. Eur. J. Sport Sci. 17 (7), 821–829. doi:10.1080/17461391.2017.1317834
Girard O., Mariotti-Nesurini L., Malatesta D. (2022). Acute performance and physiological responses to upper-limb multi-set exercise to failure: effects of external resistance and systemic hypoxia. Eur. J. Sport Sci. 22 (12), 1877–1888. doi:10.1080/17461391.2021.2002951
Gordon S. E., Kraemer W. J., Vos N. H., Lynch J. M., Knuttgen H. G. (1994). Effect of acid-base balance on the growth hormone response to acute high-intensity cycle exercise. J. Appl. Physiol. 76 (2), 821–829. doi:10.1152/jappl.1994.76.2.821
Ho J. H., Huang T. Y., Chien Y. C., Chen Y. C., Liu S. Y. (2014). Effects of acute exposure to mild simulated hypoxia on hormonal responses to low-intensity resistance exercise in untrained men. Res. Sports Med. 22 (3), 240–252. doi:10.1080/15438627.2014.915834
Inness M. W., Billaut F., Walker E. J., Petersen A. C., Sweeting A. J., Aughey R. J. (2016). Heavy resistance training in hypoxia enhances 1RM squat performance. Front. Physiol. 7, 502. doi:10.3389/fphys.2016.00502
Kon M., Ikeda T., Homma T., Akimoto T., Suzuki Y., Kawahara T. (2010). Effects of acute hypoxia on metabolic and hormonal responses to resistance exercise. Med. Sci. Sports Exerc 42 (7), 1279–1285. doi:10.1249/MSS.0b013e3181ce61a5
Kon M., Ikeda T., Homma T., Suzuki Y. (2012). Effects of low-intensity resistance exercise under acute systemic hypoxia on hormonal responses. J. Strength Cond. Res. 26 (3), 611–617. doi:10.1519/JSC.0b013e3182281c69
Kurobe K., Huang Z., Nishiwaki M., Yamamoto M., Kanehisa H., Ogita F. (2015). Effects of resistance training under hypoxic conditions on muscle hypertrophy and strength. Clin. Physiol. Funct. Imaging 35 (3), 197–202. doi:10.1111/cpf.12147
Kon M., Ikeda T., Homma T., Suzuki Y. (2021). Responses of angiogenic regulators to resistance exercise under systemic hypoxia. Journal of Strength and Conditioning Research. 35 (2), 436–441.
Li S. N., Peeling P., Scott B. R., Peiffer J. J., Shaykevich A., Girard O. (2023). Automatic heart rate clamp: a practical tool to control internal and external training loads during aerobic exercise. Front. Physiol. 14, 1170105. doi:10.3389/fphys.2023.1170105
Li S. N., Peeling P., Scott B. R., Peiffer J. J., Shaykevich A., Girard O. (2024). Automatic adjustment of cycle ergometer power output to accurately clamp heart rate. J. Sports Sci. 42 (9), 847–850. doi:10.1080/02640414.2024.2369353
Mira J., Floreani M., Savoldelli A., Amery K., Koral J., Oranchuk D. J., et al. (2020). Neuromuscular fatigue of cycling exercise in hypoxia. Medi. Sci. Spor. Exerci. 52 (9), 1888–1899.
Mayo B., Miles C., Sims S., Driller M. (2018). The effect of resistance training in a hypoxic chamber on physical performance in elite rugby athletes. High Alti. Medi. Bio. 19 (1), 28–34.
Manimmanakorn A., Hamlin M. J., Ross J. J., Taylor R., Manimmanakorn N. (2013). Effects of low-load resistance training combined with blood flow restriction or hypoxia on muscle function and performance in netball athletes. J. Sci. Med. Sport 16 (4), 337–342. doi:10.1016/j.jsams.2012.08.009
Martinez-Guardado I., Ramos-Campo D. J., Olcina G. J., Rubio-Arias J. A., Chung L. H., Marín-Cascales E., et al. (2019). Effects of high-intensity resistance circuit-based training in hypoxia on body composition and strength performance. Eur. J. Sport Sci. 19 (7), 941–951. doi:10.1080/17461391.2018.1564796
McKay A. K., Stellingwerff T., Smith E. S., Martin D. T., Mujika I., Goosey-Tolfrey V. L., et al. (2022). Defining training and performance caliber: a participant classification framework. Int. J. Sports Physiol. Perform. 17 (3), 317–331. doi:10.1123/ijspp.2021-0451
Namboonlue C., Hamlin M. J., Sirasaporn P., Manimmanakorn N., Wonnabussapawich P., Sumethanurakkhakun W., et al. (2020). Optimal degree of hypoxia combined with low-load resistance training for muscle strength and thickness in athletes. J. Phys. Educ. Sport 20 (2), 828–838. doi:10.7752/jpes.2020.02119
Nishimura A., Sugita M., Kato K., Fukuda A., Sudo A., Uchida A. (2020). Hypoxia increases muscle hypertrophy induced by resistance training. Int. J. Sports Physiol. Perform. 5 (4), 497–508. doi:10.1123/ijspp.5.4.497
Raberin A., Burtscher J., Citherlet T., Manferdelli G., Krumm B., Bourdillon N., et al. (2023). Women at altitude: sex-related physiological responses to exercise in hypoxia. Sports Med. 54, 271–287. doi:10.1007/s40279-023-01954-6
Ramos-Campo D. J., Martínez-Guardado I., Olcina G., Marín-Pagán C., Martínez-Noguera F. J., Carlos-Vivas J., et al. (2018). Effect of high-intensity resistance circuit-based training in hypoxia on aerobic performance and repeat sprint ability. Scand. J. Med. Sci. Sports 28 (10), 2135–2143. doi:10.1111/sms.13223
Ramos-Campo D. J., Scott B. R., Alcaraz P. E., Rubio-Arias J. A. (2018). The efficacy of resistance training in hypoxia to enhance strength and muscle growth: a systematic review and meta-analysis. Eur. J. Sport Sci. 18 (1), 92–103. doi:10.1080/17461391.2017.1388850
Schoenfeld B. J. (2010). The mechanisms of muscle hypertrophy and their application to resistance training. J. Strength Cond. Res. 24 (10), 2857–2872. doi:10.1519/JSC.0b013e3181e840f3
Scott B. R., Goods P. S. R., Slattery K. M. (2016). High-intensity exercise in hypoxia: is increased reliance on anaerobic metabolism important? Front. Physiol. 7, 637. doi:10.3389/fphys.2016.00637
Scott B. R., Slattery K. M., Dascombe B. J. (2015b). Intermittent hypoxic resistance training: is metabolic stress the key moderator? Med. Hypotheses 84, 145–149. doi:10.1016/j.mehy.2014.12.001
Scott B. R., Slattery K. M., Sculley D. V., Hodson J. A., Dascombe B. J. (2015a). Physical performance during high-intensity resistance exercise in normoxic and hypoxic conditions. J. Strength Cond. Res. 29 (3), 807–815. doi:10.1519/JSC.0000000000000680
Soo J., Girard O., Ihsan M., Fairchild T. (2020). The use of the SpO2 to FiO2 ratio to individualize the hypoxic dose in sport science, exercise, and health settings. Front. Physiol. 11, 570472. doi:10.3389/fphys.2020.570472
Timon R., Olcina G., Padial P., Bonitch-Góngora J., Martínez-Guardado I., Benavente C., et al. (2022). Effects of resistance training in hypobaric vs. normobaric hypoxia on circulating ions and hormones. Int. J. Environ. Res. Public Health 19, 3436. doi:10.3390/ijerph19063436
Törpel A., Peter B., Hamacher D., Schega L. (2019). Dose-response relationship of intermittent normobaric hypoxia to stimulate erythropoietin in the context of health promotion in young and old people. Eur. J. Appl. Physiol. 119, 1065–1074. doi:10.1007/s00421-019-04096-8
Törpel A., Peter B., Schega L. (2020). Effect of resistance training under normobaric hypoxia on physical performance, hematological parameters, and body composition in young and older people. Front. Physiol. 11, 335. doi:10.3389/fphys.2020.00335
Victor R. G., Seals D. R. (1989). Reflex stimulation of sympathetic outflow during rhythmic exercise in humans. Am. J. Physiol. Heart Circ. Physiol. 257 (6), H2017–H2024. doi:10.1152/ajpheart.1989.257.6.H2017
Keywords: systemic hypoxia, simulated altitude, strength, endocrine responses, anabolic hormones
Citation: Jiang G, Qin S, Yan B and Girard O (2024) Metabolic and hormonal responses to acute high-load resistance exercise in normobaric hypoxia using a saturation clamp. Front. Physiol. 15:1445229. doi: 10.3389/fphys.2024.1445229
Received: 07 June 2024; Accepted: 21 August 2024;
Published: 02 September 2024.
Edited by:
Ignacio Bartolomé Sánchez, Pontifical University of Salamanca, SpainReviewed by:
Jan Mieszkowski, Gdansk University of Physical Education and Sport, PolandAdam C McDonnell, Institut Jožef Stefan (IJS), Slovenia
Copyright © 2024 Jiang, Qin, Yan and Girard. This is an open-access article distributed under the terms of the Creative Commons Attribution License (CC BY). The use, distribution or reproduction in other forums is permitted, provided the original author(s) and the copyright owner(s) are credited and that the original publication in this journal is cited, in accordance with accepted academic practice. No use, distribution or reproduction is permitted which does not comply with these terms.
*Correspondence: Bing Yan, eWFuYmluZ0Bic3UuZWR1LmNu
†ORCID: Bing Yan, orcid.org/0000-0003-0951-9186
‡These authors have contributed equally to this work