- 1Division of Molecular and Regenerative Prosthodontics, Tohoku University Graduate School of Dentistry, Sendai, Japan
- 2Stem Cell Institute, University of Science, Viet Nam National University Ho Chi Minh City, Ho Chi Minh, Vietnam
- 3Veterinary Clinical Stem Cell and Bioengineering Research Unit, Faculty of Veterinary Science, Chulalongkorn University, Bangkok, Thailand
- 4Veterinary Stem Cell and Bioengineering Innovation Center, Faculty of Veterinary Science, Chulalongkorn University, Bangkok, Thailand
- 5Department of Pharmacology, Faculty of Veterinary Science, Chulalongkorn University, Bangkok, Thailand
Introduction: Type 2 diabetes (T2D) is the predominant form of diabetes mellitus and is among the leading causes of death with an increasing prevalence worldwide. However, the pathological mechanism underlying T2D remains complex and unclear. An increasing number of studies have suggested an association between circadian clock disruption and high T2D prevalence.
Method: This review explores the physiological and genetic evidence underlying T2D symptoms associated with circadian clock disturbances, including insulin secretion and glucose metabolism.
Results and Discussion: Notably, circadian clock disruption reduces insulin secretion and insulin sensitivity and negatively affects glucose homeostasis. The circadian clock regulates the hypothalamic–pituitary–adrenal axis, an important factor that regulates glucose metabolism and influences T2D progression. Therefore, circadian clock regulation is an attractive, novel therapeutic approach for T2D, and various circadian clock stabilizers play therapeutic roles in T2D. Lastly, this review suggests novel therapeutic and preventive approaches using circadian clock regulators for T2D.
1 Introduction
Diabetes mellitus is a chronic disease characterized by hyperglycemia and is classified into two major types: types 1 and 2. Type 2 diabetes (T2D) represents approximately 95% of all diagnosed diabetes cases, and its prevalence is increasing worldwide (Ong et al., 2023). The characteristic symptoms of T2D are thirst, frequent infections, and weight loss. In addition, T2D can lead to poor peripheral circulation, cardiovascular disease, kidney failure, and even death if left untreated (Chatterjee et al., 2017). However, a considerable number of cases remain undiagnosed due to the slow progression of hyperglycemia to T2D (Zheng et al., 2018). Furthermore, the pathological mechanisms underlying T2D remain complex and unclear.
Diverse environmental factors, including circadian rhythms, drive T2D pathogenesis and progression. An increasing number of studies suggest an association between circadian rhythm disruptions, such as rotating work shifts and jet lag, and a high prevalence of T2D (Pan et al., 2011; Onaolapo and Onaolapo, 2018; Gao et al., 2020). The circadian rhythm is fundamental in all living organisms, synchronizing their physiological functions with the daily environmental cycle (Reppert and Weaver, 2002). The central pacemaker of circadian rhythms is the hypothalamic suprachiasmatic nucleus (SCN) in the central nervous system. The SCN is primarily controlled by light via the retinohypothalamic tract and transmits rhythmic signals to peripheral organs (Ono et al., 2020; Jones et al., 2021). The circadian clock regulates the circadian rhythms of peripheral organs, which in turn affects metabolism, glucose intake, and insulin secretion (Stenvers et al., 2019; Parameswaran and Ray, 2022). However, the molecular mechanisms underlying the correlation between the circadian clock and T2D remain poorly understood.
The hypothalamic–pituitary–adrenal (HPA) axis is responsible for maintaining homeostasis by controlling blood glucose levels via glucocorticoid secretion (Lightman and Conway-Campbell, 2010). HPA axis dysfunction can lead to dysregulation of glucocorticoid levels, resulting in T2D progression (Li and Cummins, 2022). The HPA axis is regulated by the circadian clock; therefore, its hormone secretion exhibits circadian rhythmicity and can be modulated by day/light rhythmicity (Lightman and Conway-Campbell, 2010; Kalsbeek et al., 2012). In addition, the circadian clock controls glucocorticoid levels via the HPA axis (den Boon and Sarabdjitsingh, 2017). Thus, the circadian clock appears to be associated with T2D progression through the HPA axis.
This review summarizes the molecular mechanisms by which the circadian clock regulates blood glucose levels. Moreover, we discuss the relationship between the HPA axis and circadian clock and suggest a potential role of the circadian clock in T2D pathogenesis and progression through the HPA axis. This provides a basis for novel approaches based on regulation of the circadian clock for the prevention and treatment of T2D.
2 Pathophysiology of T2D
Under normal physiological conditions, increased plasma glucose levels promote insulin production and secretion from pancreatic β-cells, which stimulates blood glucose uptake into peripheral tissues and inhibits hepatic gluconeogenesis. Insulin binds to receptors on various tissues, including muscles, liver, and adipose tissue, and enhances glucose uptake into these tissues to maintain blood glucose homeostasis (Norton et al., 2022). Following uptake, glucose is used to generate energy or is stored as glycogen in the muscle and liver or as triglycerides in adipose tissue, acting as a reservoir for low blood glucose conditions (James et al., 2021).
T2D is caused by two pathophysiological conditions: pancreatic β-cell dysfunction and insulin resistance in insulin-targeting tissues. Continuous hyperglycemia promotes insulin resistance in peripheral tissues, leading to decreased sensitivity of cells to insulin. In the prediabetic stage, pancreatic β-cells increase their insulin secretion to offset insulin resistance and regulate blood glucose levels. However, chronic increased insulin secretion leads to pancreatic β-cell dysfunction and insulin deficiency, which eventually leading to the redevelopment of hyperglycemia, and ultimately T2D (DeFronzo, 2004; Muoio and Newgard, 2008).
3 Disruption of circadian rhythm induces T2D
3.1 Circadian clock regulates circadian rhythm
The circadian clock orchestrates the 24-h cycle through the transcription-translation feedback loop (TTFL) (Zhuang et al., 2023) (Figure 1A). In the TTFL, circadian clock molecules brain and muscle Arnt-like protein 1 (BMAL1) and circadian locomotor output cycle kaput (CLOCK) provide positive feedback. The BMAL1 and CLOCK heterodimer binds to a cis-regulatory enhancer sequence known as E-box to induce the transcription of period (PER) and cryptochrome (CRY). Complexes formed by PER and CRY translocate into the nucleus to suppress the transcriptional activation of BMAL1 and CLOCK. When PER and CRY protein levels decrease, the BMAL1 and CLOCK complexes rebind to E-box and reactivate circadian clock gene transcription, initiating a new cycle. This feedback loop serves as a core regulator of the 24-h cycle (Ono, 2022).
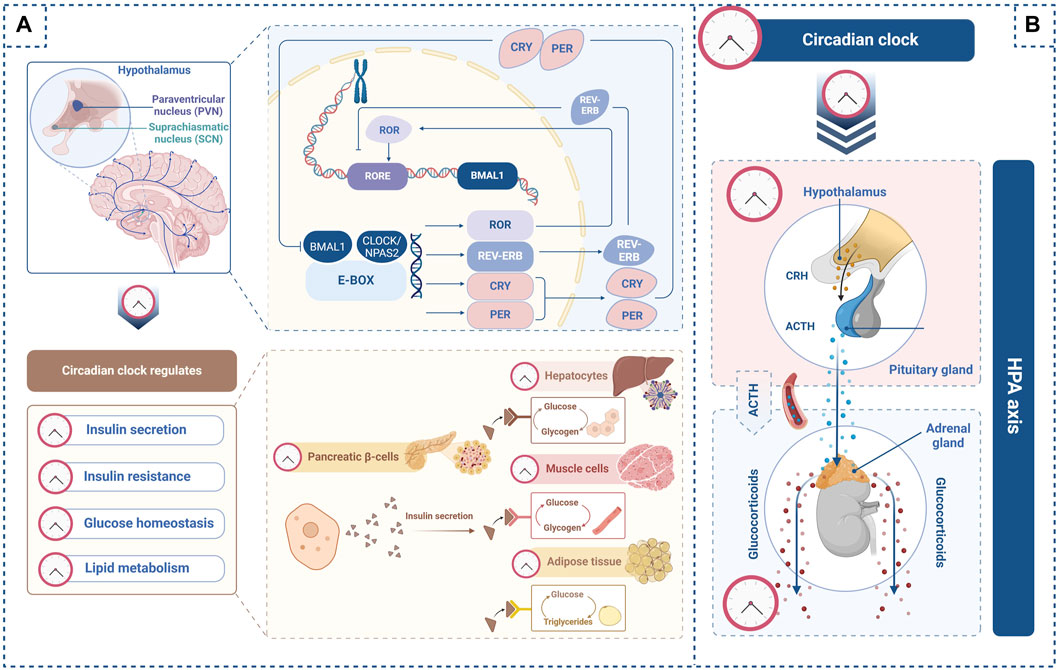
Figure 1. Circadian clock regulates glucose homeostasis through the hypothalamic–pituitary–adrenal (HPA) axis. (A) In the transcription-translation feedback loop, BMAL1 and CLOCK bind to E-box to activate PER, CRY, ROR, and REV-ERB. Npas2, a potential ortholog of CLOCK, can form a heterodimer with BMAL1. PER and CRY complexes suppress the transcriptional activation of the BMAL1 and CLOCK complex. ROR binds to the BMAL1 promoter to enhance its transcription, whereas REV-ERB inhibits BMAL1 gene transcription. The circadian clock regulates insulin secretion from pancreatic β-cells as well as insulin sensitivity in the liver, muscle, and adipose tissue. Furthermore, the circadian clock regulates glycogen synthesis in the liver and muscle and lipid metabolism in adipose tissues. (B) The circadian clock regulates the secretion of corticotropin-releasing hormone (CRH) from the paraventricular nucleus (PVN) of the hypothalamus and the secretion of adrenocorticotropic hormone (ACTH) from the pituitary gland within the HPA axis. The circadian clock also regulates glucocorticoid secretion from the adrenal glands along the HPA axis. These images were created using BioRender.com.
Neuronal PAS domain protein-2 (Npas2), another circadian clock molecule, is thought to be an ortholog of CLOCK and can form a heterodimer with BMAL1 (Okawa et al., 2020). The BMAL1 and Npas2 complex may work in a compensatory manner with the BMAL1 and CLOCK complex to maintain the rhythmic cycle (Fagiani et al., 2022). Recently, Npas2 has received attention in the medical field owing to its various roles in disease progression (Morinaga et al., 2019; Yang et al., 2019).
Retinoid-related orphan receptor (ROR) and reverse orientation c-erb (REV-ERB) are other circadian rhythm regulators. ROR binds to the orphan receptor response element (RORE) in the BMAL1 promoter to enhance its transcription, whereas REV-ERB inhibits BMAL1 transcription (Patke et al., 2020). These subloops contribute to the 24-h rhythm by regulating molecular oscillations.
3.2 Circadian clock dysfunction induces T2D
Disruption of the circadian rhythm can negatively affect glucose metabolism, resulting in the promotion of diabetogenic states. Shift workers exposed to circadian rhythm misalignment show reduced insulin sensitivity and higher expression of inflammatory markers (Leproult et al., 2014). Furthermore, shift workers with circadian rhythm misalignment exhibited reduced glucose tolerance (Morris et al., 2015). Moreover, both populations with short and long sleep periods are at an increased risk of T2D progression compared with those with an optimal sleep period of 7–8 h per night (Lu et al., 2021).
The molecular mechanism of these phenomena might be explained by circadian clock function. Bmal1 or Clock mutant mice exhibit T2D symptoms, including hyperglycemia and lower insulin levels. In addition, Clock mutant mice exhibit decreased expression of genes involved in insulin signaling, glucose sensing, and islet development (Marcheva et al., 2010). Human studies support the association between the circadian clock-associated gene variants and T2D incidence. Genome-wide association studies have demonstrated a significant link between BMAL2 gene variants and T2D population in Japan (Yamaguchi et al., 2015). In addition, specific BMAL1 haplotypes are reportedly strongly associated with an elevated T2D risk in the British population (Woon et al., 2007). Moreover, the CLOCK variant increases T2D incidence (Corella et al., 2016). Thus, circadian clock dysfunction contributes to T2D progression and is a key factor underlying the biological mechanisms involved in T2D progression.
4 Effects of the circadian clock on insulin secretion and resistance
4.1 Circadian clock regulates insulin secretion
Circadian rhythms regulate glucose metabolism, and a 24-h cycle can be detected in blood glucose and insulin levels. The insulin secretion rate peaks at noon and 6:00 p.m. and dips between midnight and 6:00 a.m. (Boden et al., 1996). The circadian clock has been demonstrated to regulate insulin secretion in pancreatic islets (Figure 1A). Rat pancreatic cells show circadian oscillations of circadian clock genes, such as Bmal1, Clock, Per1, Per2, and CRY1, and the output gene Rev-erb (Mühlbauer et al., 2004). Clock knockout mice show decreased proliferation and insulin secretion in pancreatic islets and hyperglycemia (Marcheva et al., 2010). In addition, in mice, pancreas-specific mutations in Bmal1 impair normal insulin secretion (Marcheva et al., 2010; Rakshit et al., 2016), while Cry1/2 knockout results in unregulated insulin secretion (Barclay et al., 2013).
4.2 Circadian clock regulates insulin resistance
Insulin resistance is regulated by the circadian clock (Figure 1A), and several studies have investigated this association. Inappropriate sleep duration, late chronotype, social jetlag, and shift work are associated with the progression of insulin resistance (Stenvers et al., 2019). Individuals with the CRY1 variant exhibit increased insulin resistance and diabetes risk compared with those without the CRY1 variant (Dashti et al., 2014). CLOCK gene polymorphisms have also been closely associated with insulin resistance (Garcia-Rios et al., 2014). Additionally, Bmal1 knockout mice display higher rates of insulin resistance compared to wild-type mice, and transgenic Bmal1 overexpression in knockout mice ameliorates the elevated insulin resistance (Shi et al., 2013). Moreover, relative to wild-type mice, Clock or Bmal1 knockdown mice exhibit insulin resistance in muscle cells (Liu et al., 2016).
5 Effects of circadian clock on glucose metabolism
5.1 Circadian clock regulates glycogen synthesis and lipid metabolism
Glycogen synthesis in the muscle and liver displays circadian rhythms and is regulated by the circadian clock of glucose homeostasis (Reinke and Asher, 2019). The circadian clock regulates glycogen synthesis by regulating the expression of glycogen synthesis-related genes, such as glycogen synthase 2 (GYS2) and phosphoenolpyruvate carboxykinase (PEPCK). CLOCK can bind to the E-box located in the intron of Gys2, inducing Gys2 expression (Doi et al., 2010). On the other hand, Cry1 and Cry2 suppress Pepck expression to maintain the rhythmic cycle of glycogen storage and utilization (Zhang et al., 2010; Lamia et al., 2011).
Long-term blood glucose insufficiency prompts the enzymatic hydrolysis of triglycerides stored in white adipose tissues to produce free fatty acids and glycerol, which are released into the blood as an energy source (Unger et al., 2010). However, the redundant deposition of triglycerides by continuously increasing glucose levels leads to obesity and ultimately the development of T2D. Adipose tissues show rhythmic expression of circadian clock genes that control lipid metabolism (Ando et al., 2005). In addition, disruption of the circadian clock induces uncontrolled lipid metabolism. Clock mutant mice exhibit lower and non-rhythmic blood free fatty acid and glycerol levels with a decreased rate of lipolysis compared with that in wild-type mice (Shostak et al., 2013). Furthermore, adipose tissue-specific Bmal1 deficient mice exhibit impaired triglyceride hydrolysis compared with that in wild-type mice (Paschos et al., 2012). Rev-erb knockout mice display higher circulating triglyceride levels and lower free fatty acid levels than wild-type mice, suggesting that Rev-erb deficiency results in dysregulation of lipid metabolism (Cho et al., 2012). Hence, circadian clock disruption impairs glycogen synthesis and lipid metabolism (Figure 1A), increasing the risk of T2D.
5.2 Insulin regulates glucose metabolism through the circadian clock
The relationship between insulin and the circadian clock is bidirectional. Insulin signaling affects the circadian clock and various downstream functions in peripheral tissues. For instance, insulin administration induces a circadian rhythm phase shift in rat liver (Yamajuku et al., 2012). Individuals with insulin resistance exhibit disrupted rhythmic patterns in the expression of muscle circadian clock-associated genes, suggesting that insulin signaling plays an important role in the circadian clock of muscles (Wefers et al., 2020). Insulin directly regulates the expression of circadian clock-associated genes in both human and mouse adipose tissues (Tuvia et al., 2021). Moreover, insulin signaling induces Cry1 expression and regulates glucose and lipid metabolism (Jang et al., 2016). Chronic high serum insulin concentrations induced by a high-fat diet were reported to disrupt circadian rhythms, including Bmal1 and Clock expression, and inhibit the expression of lipogenesis-related genes in the mouse liver when compared with mice fed a normal diet (Honma et al., 2016). Thus, insulin signaling regulates glucose metabolism by regulating the expression of circadian clock molecules.
6 Relationship between HPA axis and T2D
Upon activation of the HPA axis, the paraventricular nucleus (PVN) in the hypothalamus releases corticotropin-releasing hormone (CRH), which stimulates the pituitary gland to secrete adrenocorticotropin (ACTH). ACTH then travels through the systemic circulation to the adrenal cortex where it induces glucocorticoid secretion (Herman et al., 2016; Lightman et al., 2020).
Glucocorticoids are essential for living organisms under stress conditions (Sapolsky et al., 2000). However, excessive glucocorticoid levels can lead to the development of T2D through induction of hyperglycemia, insulin resistance, and dyslipidemia (van Raalte and Diamant, 2014). Glucocorticoids directly increase hepatic insulin resistance and gluconeogenesis-related enzymes in the liver, leading to hyperglycemia (Vegiopoulos and Herzig, 2007). In the adipose tissue, glucocorticoids upregulate lipid uptake, triglyceride synthesis, and lipolysis, contributing to the local free fatty acid pool (Wang et al., 2012). In addition, glucocorticoids can decrease insulin sensitivity and glucose uptake in the skeletal muscles (Ruzzin et al., 2005). Furthermore, long-term glucocorticoid exposure leads to pancreatic β-cell apoptosis and disrupts insulin secretion (Li and Cummins, 2022).
Additionally, disruption of the HPA axis results in dysregulation of glucocorticoid production, contributing to T2D progression. HPA axis dysfunction can lead to increased daily glucocorticoid levels, hyperglycemia, and insulin resistance (Gan et al., 2023). The metabolic stress in T2D can further disrupt the HPA axis, exacerbating hyperglycemia through unregulated glucocorticoid levels (Joseph and Golden, 2017).
7 Effect of the circadian clock on the HPA axis
HPA axis-dependent glucocorticoid release is rhythmic given that it is regulated by the light-activated master circadian clock, which is the central pacemaker in the SCN (Ishida et al., 2005). Glucocorticoid levels increase in the morning and decrease after 2–3 h of sleep (Shimba and Ikuta, 2020). The master circadian clock is thought to regulate CRH release from the PVN in the hypothalamus and ACTH secretion from the pituitary gland within the HPA axis (Kalsbeek et al., 2012). In addition, the master circadian clock controls glucocorticoid secretion from the adrenal gland in the HPA axis by regulating adrenal cortex sensitivity to ACTH (Oster et al., 2006).
Various studies have shown that the circadian clock regulates the HPA axis and glucocorticoid secretion from adrenal glands. BMAL1 is reportedly expressed in CRH neurons in the PVN (Hung et al., 2021). Furthermore, the expression of circadian clock molecules, including Bmal1, CRY1, Per1, and Rev-erb, has been observed in the adrenal glands (Dumbell et al., 2016). Per1 mutant mice exhibit elevated glucocorticoid levels without a circadian rhythm, while Per2 mutant mice display higher circulating glucocorticoid levels than wild-type mice (Dallmann et al., 2006).
The loss of Bmal1 in CRH neurons causes arrhythmic activity and reduces glucocorticoid secretion (Jones et al., 2021). Additionally, adrenal gland-specific Per2 and Cry1 double-knockout mice exhibit impaired glucocorticoid production (Oster et al., 2006). These results suggest that the peripheral circadian clock in the HPA axis regulates glucocorticoid secretion.
Taken together, the light input-dependent master circadian clock regulates glucocorticoid secretion through activation of the HPA axis, whereas the peripheral circadian clock controls the rhythmic release of glucocorticoids from the adrenal glands (Figure 1B).
8 Circadian clock regulation as a therapeutic target for T2D
The circadian clock is closely associated with T2D progression. We understand that too much data comes from animal experiments at present, and the timing and composition of meals still need to be considered in humans. However, the reported results prompted interesting pharmacological investigations. In recent years, regulation of the circadian clock to control blood glucose levels has gained attention as a novel therapeutic approach for T2D (Hudec et al., 2020) (Table 1). For example, cryptochrome stabilizers would be therapeutic reagents for T2D. TW68 suppresses the gene expression of Pepck1 and glucose-6-phosphatase, which play important roles in glucogenesis, by stabilizing CRY1/2, resulting in lower blood glucose levels in high-fat diet-fed mice (Surme et al., 2023). On the other hand, KL001 prevents CRY1 and CRY2 degradation, extending the circadian period, and KL001-mediated CRY stabilization suppresses hepatocyte glucogenesis (Hirota et al., 2012). Metformin, a biguanide anti-hyperglycemic agent, activates adenosine monophosphate-activated protein kinase, a cellular metabolic regulator, by upregulating Bmal1 expression in T2D mice (Alex et al., 2020). Nobiletin, a phytochemical compound, significantly suppresses blood glucose levels and improves insulin sensitivity by upregulating the expression of various circadian clock-associated genes, including Bmal1, Per2, Cry1, Cry2, Ror, and Rev-erba (He et al., 2016). Furthermore, PF-5006739, a casein kinase 1δ and ε inhibitor, normalizes the dysregulation of various circadian clock-associated genes, including Bmal1 and Rev-erba, improving glucose tolerance (Cunningham et al., 2016). Similarly, thiazolidinedione resolves the disruption of the expression of circadian clock-associated genes(Bmal1, Per2, Cry1), improving insulin resistance (Yang et al., 2013). Circadian clock regulators, such as antidepressants, antipsychotics, and mood stabilizers, which can regulate the expression of Bmal1, Clock, and Per1, decrease the incidence of HPA axis dysregulation in patients with T2D, improving their blood glucose and glycosylated hemoglobin levels (Min et al., 2023).
High-throughput screening (HTS) is a promising tool for identifying novel circadian clock stabilizers for T2D treatment. Although HTS was initially used to identify new pharmacological agents (Kennedy et al., 2008), it can uncover new aspects of well-characterized chemical compounds (Entzeroth et al., 2009). Using HTS, a compound that can regulate Npas2 expression has been identified; this compound has been demonstrated to be useful for skin wound treatment by regulating the circadian clock (Clements et al., 2022; Shibuya et al., 2022). Therefore, HTS might help identify novel compounds that may regulate target circadian molecules for T2D treatment.
9 Conclusion
In this review, we discuss the potential role of the circadian clock in T2D progression. Circadian clock disruption decreases insulin secretion, increases insulin resistance, and disrupts glucose homeostasis, contributing to T2D progression. Additionally, this review suggests that the circadian clock regulates the HPA axis, which is vital for glucose homeostasis and T2D progression. While current evidence suggests a link between circadian rhythm disruption, the HPA axis, and T2D, further research is necessary to fully understand the mechanisms underlying this complex link in T2D pathogenesis and progression. The use of circadian clock stabilizers is a therapeutic approach for T2D, and HTS is a useful tool for identifying novel chemical compounds for regulation of the circadian clock.
Author contributions
HT: Conceptualization, Writing–original draft, Writing–review and editing. TK: Conceptualization, Writing–original draft, Writing–review and editing, AA: Writing–original draft, Writing–review and editing, YF: Writing–original draft, Writing–review and editing. HO: Writing–original draft, Writing–review and editing. CS: Writing–review and editing. HE: Conceptualization, Supervision, Writing–original draft, Writing–review and editing.
Funding
The author(s) declare that financial support was received for the research, authorship, and/or publication of this article. This study was supported by a Grant-in-Aid from the Japan Society for the Promotion of Science Research Fellowship for Young Scientists (grant number: 23K16081 (TK), 22K17104 (HO)).
Conflict of interest
The authors declare that the research was conducted in the absence of any commercial or financial relationships that could be construed as a potential conflict of interest.
Publisher’s note
All claims expressed in this article are solely those of the authors and do not necessarily represent those of their affiliated organizations, or those of the publisher, the editors and the reviewers. Any product that may be evaluated in this article, or claim that may be made by its manufacturer, is not guaranteed or endorsed by the publisher.
References
Alex A., Luo Q., Mathew D., Di R., Bhatwadekar A. D. (2020). Metformin corrects abnormal circadian rhythm and Kir4.1 channels in diabetes. Invest. Ophthalmol. Vis. Sci. 61, 46. [Epub 2020/06/24]. doi:10.1167/iovs.61.6.46
Ando H., Yanagihara H., Hayashi Y., Obi Y., Tsuruoka S., Takamura T., et al. (2005). Rhythmic messenger ribonucleic acid expression of clock genes and adipocytokines in mouse visceral adipose tissue. Endocrinology 146, 5631–5636. [Epub 2005/09/17]. doi:10.1210/en.2005-0771
Barclay J. L., Shostak A., Leliavski A., Tsang A. H., Jöhren O., Müller-Fielitz H., et al. (2013). High-fat diet-induced hyperinsulinemia and tissue-specific insulin resistance in Cry-deficient mice. Am. J. Physiol. Endocrinol. Metab. 304, E1053–E1063. [Epub 2013/03/28]. doi:10.1152/ajpendo.00512.2012
Boden G., Ruiz J., Urbain J. L., Chen X. (1996). Evidence for a circadian rhythm of insulin secretion. Am. J. Physiol. 271, E246–E252. [Epub 1996/08/01]. doi:10.1152/ajpendo.1996.271.2.E246
Chatterjee S., Khunti K., Davies M. J. (2017). Type 2 diabetes. Lancet 389, 2239–2251. doi:10.1016/S0140-6736(17)30058-2
Cho H., Zhao X., Hatori M., Yu R. T., Barish G. D., Lam M. T., et al. (2012). Regulation of circadian behaviour and metabolism by REV-ERB-α and REV-ERB-β. Nature 485, 123–127. doi:10.1038/nature11048
Clements A., Shibuya Y., Hokugo A., Brooks Z., Roca Y., Kondo T., et al. (2022). In vitro assessment of Neuronal PAS domain 2 mitigating compounds for scarless wound healing. Front. Med. (Lausanne). 9, 1014763. [Epub 2023/02/24]. doi:10.3389/fmed.2022.1014763
Corella D., Asensio E. M., Coltell O., Sorlí J. V., Estruch R., Martínez-González M. Á., et al. (2016). CLOCK gene variation is associated with incidence of type-2 diabetes and cardiovascular diseases in type-2 diabetic subjects: dietary modulation in the PREDIMED randomized trial. Cardiovasc. Diabetol. 15, 4. [Epub 2016/01/08]. doi:10.1186/s12933-015-0327-8
Cunningham P. S., Ahern S. A., Smith L. C., da Silva Santos C. S., Wager T. T., Bechtold D. A. (2016). Targeting of the circadian clock via CK1δ/ε to improve glucose homeostasis in obesity. Sci. Rep. 6, 29983. [Epub 2016/07/22]. doi:10.1038/srep29983
Dallmann R., Touma C., Palme R., Albrecht U., Steinlechner S. (2006). Impaired daily glucocorticoid rhythm in Per1 (Brd) mice. J. Comp. Physiol. A Neuroethol. Sens. Neural Behav. Physiol. 192, 769–775. [Epub 2006/03/01]. doi:10.1007/s00359-006-0114-9
Dashti H. S., Smith C. E., Lee Y. C., Parnell L. D., Lai C. Q., Arnett D. K., et al. (2014). CRY1 circadian gene variant interacts with carbohydrate intake for insulin resistance in two independent populations: mediterranean and North American. Chronobiol. Int. 31, 660–667. [Epub 2014/02/20]. doi:10.3109/07420528.2014.886587
DeFronzo R. A. (2004). Pathogenesis of type 2 diabetes mellitus. Med. Clin. North Am. 88, 787–835. ix. [Epub 2004/08/17]. doi:10.1016/j.mcna.2004.04.013
den Boon F. S., Sarabdjitsingh R. A. (2017). Circadian and ultradian patterns of HPA-axis activity in rodents: significance for brain functionality. Best. Pract. Res. Clin. Endocrinol. Metab. 31, 445–457. doi:10.1016/j.beem.2017.09.001
Doi R., Oishi K., Ishida N. (2010). CLOCK regulates circadian rhythms of hepatic glycogen synthesis through transcriptional activation of Gys2. J. Biol. Chem. 285, 22114–22121. doi:10.1074/jbc.M110.110361
Dumbell R., Matveeva O., Oster H. (2016). Circadian clocks, stress, and immunity. Front. Endocrinol. (Lausanne). 7, 37. [Epub 2016/05/21]. doi:10.3389/fendo.2016.00037
Entzeroth M., Flotow H., Condron P. (2009). Overview of high-throughput screening. Curr. Protoc. Pharmacol. 9, 4. [Epub 2009/03/01]. doi:10.1002/0471141755.ph0904s44
Fagiani F., Di Marino D., Romagnoli A., Travelli C., Voltan D., Di Cesare Mannelli L., et al. (2022). Molecular regulations of circadian rhythm and implications for physiology and diseases. Signal Transduct. Target. Ther. 7, 41. [Epub 2022/02/10]. doi:10.1038/s41392-022-00899-y
Gan L., Li N., Heizhati M., Li M., Yao L., Hong J., et al. (2023). Diurnal cortisol features and type 2 diabetes risk in patients with hypertension and obstructive sleep apnea: a cohort Study. J. Clin. Endocrinol. Metab. 108, e679–e686. [Epub 2023/04/07]. doi:10.1210/clinem/dgad184
Gao Y., Gan T., Jiang L., Yu L., Tang D., Wang Y., et al. (2020). Association between shift work and risk of type 2 diabetes mellitus: a systematic review and dose-response meta-analysis of observational studies. Chronobiol. Int. 37, 29–46. [Epub 2019/11/07]. doi:10.1080/07420528.2019.1683570
Garcia-Rios A., Gomez-Delgado F. J., Garaulet M., Alcala-Diaz J. F., Delgado-Lista F. J., Marin C., et al. (2014). Beneficial effect of CLOCK gene polymorphism rs1801260 in combination with low-fat diet on insulin metabolism in the patients with metabolic syndrome. Chronobiol. Int. 31, 401–408. [Epub 2013/12/18]. doi:10.3109/07420528.2013.864300
He B. K., Nohara K., Park N., Park Y. S., Guillory B., Zhao Z. Y., et al. (2016). The small molecule nobiletin targets the molecular oscillator to enhance circadian rhythms and protect against metabolic syndrome. Cell Metab. 23, 610–621. doi:10.1016/j.cmet.2016.03.007
Herman J. P., McKlveen J. M., Ghosal S., Kopp B., Wulsin A., Makinson R., et al. (2016). Regulation of the hypothalamic-pituitary-adrenocortical stress response. Compr. Physiol. 6, 603–621. [Epub 2016/04/12]. doi:10.1002/cphy.c150015
Hirota T., Lee J. W., St John P. C., Sawa M., Iwaisako K., Noguchi T., et al. (2012). Identification of small molecule activators of cryptochrome. Science 337, 1094–1097. [Epub 2012/07/17]. doi:10.1126/science.1223710
Honma K., Hikosaka M., Mochizuki K., Goda T. (2016). Loss of circadian rhythm of circulating insulin concentration induced by high-fat diet intake is associated with disrupted rhythmic expression of circadian clock genes in the liver. Metabolism 65, 482–491. doi:10.1016/j.metabol.2015.12.003
Hudec M., Dankova P., Solc R., Bettazova N., Cerna M. (2020). Epigenetic regulation of circadian rhythm and its possible role in diabetes mellitus. Int. J. Mol. Sci. 24, 3005. [Epub 2020/04/30]. doi:10.3390/ijms21083005
Hung C. J., Yamanaka A., Ono D. (2021). Conditional knockout of Bmal1 in corticotropin-releasing factor neurons does not alter sleep-wake rhythm in mice. Front. Neurosci. 15, 808754. [Epub 2022/03/08]. doi:10.3389/fnins.2021.808754
Ishida A., Mutoh T., Ueyama T., Bando H., Masubuchi S., Nakahara D., et al. (2005). Light activates the adrenal gland: timing of gene expression and glucocorticoid release. Cell Metab. 2, 297–307. [Epub 2005/11/08]. doi:10.1016/j.cmet.2005.09.009
James D. E., Stöckli J., Birnbaum M. J. (2021). The aetiology and molecular landscape of insulin resistance. Nat. Rev. Mol. Cell Biol. 22, 751–771. doi:10.1038/s41580-021-00390-6
Jang H., Lee G. Y., Selby C. P., Lee G., Jeon Y. G., Lee J. H., et al. (2016). SREBP1c-CRY1 signalling represses hepatic glucose production by promoting FOXO1 degradation during refeeding. Nat. Commun. 7, 12180. [Epub 2016/07/15]. doi:10.1038/ncomms12180
Jones J. R., Chaturvedi S., Granados-Fuentes D., Herzog E. D. (2021). Circadian neurons in the paraventricular nucleus entrain and sustain daily rhythms in glucocorticoids. Nat. Commun. 12, 5763. [Epub 2021/10/03]. doi:10.1038/s41467-021-25959-9
Joseph J. J., Golden S. H. (2017). Cortisol dysregulation: the bidirectional link between stress, depression, and type 2 diabetes mellitus. Ann. N. Y. Acad. Sci. Mar. 1391, 20–34. [Epub 2016/10/18]. doi:10.1111/nyas.13217
Kalsbeek A., van der Spek R., Lei J., Endert E., Buijs R. M., Fliers E. (2012). Circadian rhythms in the hypothalamo-pituitary-adrenal (HPA) axis. Mol. Cell. Endocrinol. 349, 20–29. [Epub 2011/07/26]. doi:10.1016/j.mce.2011.06.042
Kennedy J. P., Williams L., Bridges T. M., Daniels R. N., Weaver D., Lindsley C. W. (2008). Application of combinatorial chemistry science on modern drug discovery. J. Comb. Chem. 10, 345–354. [Epub 2008/01/29]. doi:10.1021/cc700187t
Lamia K. A., Papp S. J., Yu R. T., Barish G. D., Uhlenhaut N. H., Jonker J. W., et al. (2011). Cryptochromes mediate rhythmic repression of the glucocorticoid receptor. Nature 480, 552–556. doi:10.1038/nature10700
Leproult R., Holmbäck U., Van Cauter E. (2014). Circadian misalignment augments markers of insulin resistance and inflammation, independently of sleep loss. Diabetes 63, 1860–1869. [Epub 2014/01/25]. doi:10.2337/db13-1546
Li J. X., Cummins C. L. (2022). Fresh insights into glucocorticoid-induced diabetes mellitus and new therapeutic directions. Nat. Rev. Endocrinol. 18, 540–557. [Epub 2022/05/19]. doi:10.1038/s41574-022-00683-6
Lightman S. L., Birnie M. T., Conway-Campbell B. L. (2020). Dynamics of ACTH and cortisol secretion and implications for disease. Endocr. Rev. 1, bnaa002. [Epub 2020/02/16]. doi:10.1210/endrev/bnaa002
Lightman S. L., Conway-Campbell B. L. (2010). The crucial role of pulsatile activity of the HPA axis for continuous dynamic equilibration. Nat. Rev. Neurosci. 11, 710–718. [Epub 2010/09/16]. doi:10.1038/nrn2914
Liu J., Zhou B., Yan M., Huang R., Wang Y., He Z., et al. (2016). CLOCK and BMAL1 regulate muscle insulin sensitivity via SIRT1 in Male Mice. Endocrinology 157, 2259–2269. [Epub 2016/04/02]. doi:10.1210/en.2015-2027
Lu H., Yang Q., Tian F., Lyu Y., He H., Xin X., et al. (2021). A meta-analysis of a cohort Study on the association between sleep duration and type 2 diabetes mellitus. J. Diabetes Res. 2021, 8861038. [Epub 2021/04/10]. doi:10.1155/2021/8861038
Marcheva B., Ramsey K. M., Buhr E. D., Kobayashi Y., Su H., Ko C. H., et al. (2010). Disruption of the clock components CLOCK and BMAL1 leads to hypoinsulinaemia and diabetes. Nature 466, 627–631. [Epub 2010/06/22]. doi:10.1038/nature09253
Min W., Sun X., Tang N., Zhang Y., Luo F., Zhu M., et al. (2023). A new model for the treatment of type 2 diabetes mellitus based on rhythm regulations under the framework of psychosomatic medicine: a real-world study. Sci. Rep. 13, 1047. [Epub 2023/01/20]. doi:10.1038/s41598-023-28278-9
Morinaga K., Sasaki H., Park S., Hokugo A., Okawa H., Tahara Y., et al. (2019). Neuronal PAS domain 2 (Npas2) facilitated osseointegration of titanium implant with rough surface through a neuroskeletal mechanism. Biomaterials 192, 62–74. [Epub 2018/11/15]. doi:10.1016/j.biomaterials.2018.11.003
Morris C. J., Yang J. N., Garcia J. I., Myers S., Bozzi I., Wang W., et al. (2015). Endogenous circadian system and circadian misalignment impact glucose tolerance via separate mechanisms in humans. Proc. Natl. Acad. Sci. U. S. A. 112, E2225–E2234. [Epub 2015/04/15]. doi:10.1073/pnas.1418955112
Mühlbauer E., Wolgast S., Finckh U., Peschke D., Peschke E. (2004). Indication of circadian oscillations in the rat pancreas. FEBS Lett. 564, 91–96. [Epub 2004/04/20]. doi:10.1016/S0014-5793(04)00322-9
Muoio D. M., Newgard C. B. (2008). Mechanisms of disease:molecular and metabolic mechanisms of insulin resistance and beta-cell failure in type 2 diabetes. Nat. Rev. Mol. Cell Biol. 9, 193–205. [Epub 2008/01/18]. doi:10.1038/nrm2327
Norton L., Shannon C., Gastaldelli A., DeFronzo R. A. (2022). Insulin: the master regulator of glucose metabolism. Metabolism 129, 155142. [Epub 2022/01/24]. doi:10.1016/j.metabol.2022.155142
Okawa H., Egusa H., Nishimura I. (2020). Implications of the circadian clock in implant dentistry. Dent. Mat. J. 39, 173–180. [Epub 2020/03/03]. doi:10.4012/dmj.2019-291
Onaolapo A. Y., Onaolapo O. J. (2018). Circadian dysrhythmia-linked diabetes mellitus: examining melatonin’s roles in prophylaxis and management. World J. Diabetes 9, 99–114. [Epub 2018/08/07]. doi:10.4239/wjd.v9.i7.99
Ong K. L., Stafford L. K., McLaughlin S. A., Boyko E. J., Vollset S. E., Smith A. E. (2023). Global, regional, and national burden of diabetes from 1990 to 2021, with projections of prevalence to 2050: a systematic analysis for the Global Burden of Disease Study 2021. Lancet 402, 203–234. doi:10.1016/S0140-6736(23)01301-6
Ono D. (2022). Neural circuits in the central circadian clock and their regulation of sleep and wakefulness in mammals. Neurosci. Res. 182, 1–6. [Epub 2022/05/22]. doi:10.1016/j.neures.2022.05.005
Ono D., Mukai Y., Hung C. J., Chowdhury S., Sugiyama T., Yamanaka A. (2020). The mammalian circadian pacemaker regulates wakefulness via CRF neurons in the paraventricular nucleus of the hypothalamus. Sci. Adv. 6, eabd0384. [Epub 2020/11/08]. doi:10.1126/sciadv.abd0384
Oster H., Damerow S., Kiessling S., Jakubcakova V., Abraham D., Tian J., et al. (2006). The circadian rhythm of glucocorticoids is regulated by a gating mechanism residing in the adrenal cortical clock. Cell Metab. 4, 163–173. [Epub 2006/08/08]. doi:10.1016/j.cmet.2006.07.002
Pan A., Schernhammer E. S., Sun Q., Hu F. B. (2011). Rotating night shift work and risk of type 2 diabetes: two prospective cohort studies in women. PLOS Med. 8, e1001141. [Epub 2011/12/14]. doi:10.1371/journal.pmed.1001141
Parameswaran G., Ray D. W. (2022). Sleep, circadian rhythms, and type 2 diabetes mellitus. Clin. Endocrinol. (Oxf). 96, 12–20. [Epub 2021/10/13]. doi:10.1111/cen.14607
Paschos G. K., Ibrahim S., Song W. L., Kunieda T., Grant G., Reyes T. M., et al. (2012). Obesity in mice with adipocyte-specific deletion of clock component Arntl. Nat. Med. 18, 1768–1777. [Epub 2012/11/13]. doi:10.1038/nm.2979
Patke A., Young M. W., Axelrod S. (2020). Molecular mechanisms and physiological importance of circadian rhythms. Nat. Rev. Mol. Cell Biol. 21, 67–84. [Epub 2019/11/27]. doi:10.1038/s41580-019-0179-2
Rakshit K., Hsu T. W., Matveyenko A. V. (2016). Bmal1 is required for beta cell compensatory expansion, survival and metabolic adaptation to diet-induced obesity in mice. Diabetologia 59, 734–743. doi:10.1007/s00125-015-3859-2
Reinke H., Asher G. (2019). Crosstalk between metabolism and circadian clocks. Nat. Rev. Mol. Cell Biol. 20, 227–241. doi:10.1038/s41580-018-0096-9
Reppert S. M., Weaver D. R. (2002). Coordination of circadian timing in mammals. Nature 418, 935–941. [Epub 2002/08/29]. doi:10.1038/nature00965
Ruzzin J., Wagman A. S., Jensen J. (2005). Glucocorticoid-induced insulin resistance in skeletal muscles: defects in insulin signalling and the effects of a selective glycogen synthase kinase-3 inhibitor. Diabetologia. 48, 2119–2130. [Epub 2005/08/04]. doi:10.1007/s00125-005-1886-0
Sapolsky R. M., Romero L. M., Munck A. U. (2000). How do glucocorticoids influence stress responses? Integrating permissive, suppressive, stimulatory, and preparative actions. Endocr. Rev. 21, 55–89. [Epub 2000/03/04]. doi:10.1210/edrv.21.1.0389
Shi S. Q., Ansari T. S., McGuinness O. P., Wasserman D. H., Johnson C. H. (2013). Circadian disruption leads to insulin resistance and obesity. Curr. Biol. Cb. 23, 372–381. [Epub 2013/02/26]. doi:10.1016/j.cub.2013.01.048
Shibuya Y., Hokugo A., Okawa H., Kondo T., Khalil D., Wang L., et al. (2022). Therapeutic downregulation of neuronal PAS domain 2 (Npas2) promotes surgical skin wound healing. Elife 18, e71074. [Epub 2022/01/19]. doi:10.7554/eLife.71074
Shimba A., Ikuta K. (2020). Glucocorticoids regulate circadian rhythm of innate and adaptive immunity. Front. Immunol. 11, 2143. [Epub 2020/10/20]. doi:10.3389/fimmu.2020.02143
Shostak A., Meyer-Kovac J., Oster H. (2013). Circadian regulation of lipid mobilization in white adipose tissues. Diabetes 62, 2195–2203. doi:10.2337/db12-1449
Stenvers D. J., Scheer F. A. J. L., Schrauwen P., la Fleur S. E., Kalsbeek A. (2019). Circadian clocks and insulin resistance. Nat. Rev. Endocrinol. 15, 75–89. [Epub 2018/12/12]. doi:10.1038/s41574-018-0122-1
Surme S., Ergun C., Gul S., Akyel Y. K., Gul Z. M., Ozcan O., et al. (2023). TW68, cryptochromes stabilizer, regulates fasting blood glucose levels in diabetic ob/ob and high fat-diet-induced obese mice. Biochem. Pharmacol. 218, 115896. [Epub 2023/10/29]. doi:10.1016/j.bcp.2023.115896
Tuvia N., Pivovarova-Ramich O., Murahovschi V., Lück S., Grudziecki A., Ost A. C., et al. (2021). Insulin directly regulates the circadian clock in adipose tissue. Diabetes 70, 1985–1999. [Epub 2021/07/07]. doi:10.2337/db20-0910
Unger R. H., Clark G. O., Scherer P. E., Orci L. (2010). Lipid homeostasis, lipotoxicity and the metabolic syndrome. Biochim. Biophys. Acta. 1801, 209–214. [Epub 2009/12/02]. doi:10.1016/j.bbalip.2009.10.006
van Raalte D. H., Diamant M. (2014). Steroid diabetes: from mechanism to treatment? Neth. J. Med. 72, 62–72. [Epub 2014/03/25].
Vegiopoulos A., Herzig S. (2007). Glucocorticoids, metabolism and metabolic diseases. Mol. Cell. Endocrinol. 275, 43–61. [Epub 2007/07/13]. doi:10.1016/j.mce.2007.05.015
Wang J. C., Gray N. E., Kuo T., Harris C. A. (2012). Regulation of triglyceride metabolism by glucocorticoid receptor. Cell Biosci. 2, 19. [Epub 2012/05/30]. doi:10.1186/2045-3701-2-19
Wefers J., Connell N. J., Fealy C. E., Andriessen C., de Wit V., van Moorsel D., et al. (2020). Day-night rhythm of skeletal muscle metabolism is disturbed in older, metabolically compromised individuals. Mol. Metab. 41, 101050. [Epub 2020/07/14]. doi:10.1016/j.molmet.2020.101050
Woon P. Y., Kaisaki P. J., Bragança J., Bihoreau M. T., Levy J. C., Farrall M., et al. (2007). Aryl hydrocarbon receptor nuclear translocator-like (BMAL1) is associated with susceptibility to hypertension and type 2 diabetes. Proc. Natl. Acad. Sci. U. S. A. 104, 14412–14417. [Epub 2007/08/31]. doi:10.1073/pnas.0703247104
Yamaguchi M., Uemura H., Arisawa K., Katsuura-Kamano S., Hamajima N., Hishida A., et al. (2015). Association between brain-muscle-ARNT-like protein-2 (BMAL2) gene polymorphism and type 2 diabetes mellitus in obese Japanese individuals: a cross-sectional analysis of the Japan Multi-institutional Collaborative Cohort Study. Diabetes Res. Clin. Pract. 110, 301–308. [Epub 2015/10/27]. doi:10.1016/j.diabres.2015.10.009
Yamajuku D., Inagaki T., Haruma T., Okubo S., Kataoka Y., Kobayashi S., et al. (2012). Real-time monitoring in three-dimensional hepatocytes reveals that insulin acts as a synchronizer for liver clock. Sci. Rep. 2, 439. doi:10.1038/srep00439
Yang S. C., Tseng H. L., Shieh K. R. (2013). Circadian-clock system in mouse liver affected by insulin resistance. Chronobiol. Int. 30, 796–810. [Epub 2013/06/07]. doi:10.3109/07420528.2013.766204
Yang T., Yuan P., Yang Y., Liang N., Wang Q., Li J., et al. (2019). NPAS2 contributes to liver fibrosis by direct transcriptional activation of Hes1 in hepatic stellate cells. Mol. Ther. Nucleic Acids 18, 1009–1022. [Epub 2019/11/30]. doi:10.1016/j.omtn.2019.10.025
Zhang E. E., Liu Y., Dentin R., Pongsawakul P. Y., Liu A. C., Hirota T., et al. (2010). Cryptochrome mediates circadian regulation of cAMP signaling and hepatic gluconeogenesis. Nat. Med. 16, 1152–1156. [Epub 2010/09/21]. doi:10.1038/nm.2214
Zheng Y., Ley S. H., Hu F. B. (2018). Global aetiology and epidemiology of type 2 diabetes mellitus and its complications. Nat. Rev. Endocrinol. 14, 88–98. [Epub 2017/12/09]. doi:10.1038/nrendo.2017.151
Keywords: type 2 diabetes, circadian clock, insulin secretion, insulin resistance, glucose metabolism, HPA axis
Citation: Tran HT, Kondo T, Ashry A, Fu Y, Okawa H, Sawangmake C and Egusa H (2024) Effect of circadian clock disruption on type 2 diabetes. Front. Physiol. 15:1435848. doi: 10.3389/fphys.2024.1435848
Received: 21 May 2024; Accepted: 08 July 2024;
Published: 06 August 2024.
Edited by:
Tracey L. Sletten, Monash University, AustraliaReviewed by:
Felice Strollo, IRCCS San Raffaele Pisana, Rome, ItalyCopyright © 2024 Tran, Kondo, Ashry, Fu, Okawa, Sawangmake and Egusa. This is an open-access article distributed under the terms of the Creative Commons Attribution License (CC BY). The use, distribution or reproduction in other forums is permitted, provided the original author(s) and the copyright owner(s) are credited and that the original publication in this journal is cited, in accordance with accepted academic practice. No use, distribution or reproduction is permitted which does not comply with these terms.
*Correspondence: Takeru Kondo, dGFrZXJ1LmtvbmRvLmE3QHRvaG9rdS5hYy5qcA==; Hiroshi Egusa, ZWd1QHRvaG9rdS5hYy5qcA==