- 1Laboratório de Bioquímica e Fisiologia de Insetos, Instituto Oswaldo Cruz (IOC/Fiocruz), Rio de Janeiro, Brazil
- 2Programa de Pós-Graduação em Ciências e Biotecnologia, Universidade Federal Fluminense, Niterói, Brazil
- 3Universidade Federal Fluminense, Instituto de Biologia, Departamento de Biologia Geral, Laboratório de Biologia de Insetos, Niterói, Brazil
- 4Instituto Nacional de Entomologia Molecular (INCT-EM), Rio de Janeiro, Brazil
- 5Department of Parasitology, Faculty of Science, Charles University, Praha, Czechia
Introduction: Rhodnius prolixus is a hematophagous insect and one of the main vectors for Trypanosoma cruzi and Trypanosoma rangeli parasites in Latin America. Gut microbiota and insect immune responses affect T. cruzi and T. rangeli infection within triatomines. Particularly the Toll and IMD signaling pathways activations and how they orchestrate the antimicrobial peptides (AMPs) expressions in R. prolixus, especially when infected by T. rangeli.
Objectives: Examine how T. rangeli infection modulates R. prolixus cellular and humoral immunity and its impacts on insect microbiota.
Methods: R. prolixus was fed on blood containing epimastigotes of T. rangeli, and infection was quantified in insect tissues. The gene expression of dorsal, cactus, relish, PGRP, and AMPs was examined in the midgut, fat body, and salivary glands by quantitative real-time PCR. Microbiota composition was analyzed using RT-q PCR targeting specific bacterial species. Hemocyte numbers and phenoloxidase activity were quantified to assess cellular immune responses.
Results: T. rangeli infection modulated triatomine immunity in midgut and hemocoel, activating the expression of the NF-kB gene dorsal, associated with the Toll pathway; increasing expression of the gene encoding PGRP receptor, a component involved in the IMD pathway, both in the intestine and fat body; repressing the expression of the relish transcription factor, mainly in salivary glands. Among the R. prolixus AMPs studied, T. rangeli infection repressed all AMP gene expression, other than defensin C which increased mRNA levels. The PO activity was enhanced in the hemolymph of infected insects. T. rangeli infection did not induce hemocyte number alterations compared to control insects. However, an increase in hemocyte microaggregation was detected in infected insects.
Discussion: R. prolixus recognizes T. rangeli infection and triggers humoral and cellular immune responses involving Toll pathway activation, defensin C synthesis, increased phenoloxidase activity, and enhanced hemocyte aggregation. On the other hand, T. rangeli infection suppressed some IMD pathway components, suggesting that, in R. prolixus, this pathway is involved in defensins A and B gene regulation. Importantly, these immune responses altered the bacterial microbiota composition, potentially favoring T. rangeli establishment in the insect vector.
Introduction
The hematophagous insect Rhodnius prolixus is an important triatomine vector for T. cruzi, Latin America’s causative agent of Chagas disease. This species has the propensity to inhabit synanthropic environments, presents a fast life cycle, high population density and is very susceptible to Trypanosoma cruzi infection (Guhl et al., 2007; Coura and Junqueira, 2015). The genus Rhodnius is also known to be the natural invertebrate host for another Trypanosomatidae, the T. rangeli (Eger-Mangrich et al., 2001; Guhl and Vallejo, 2003; Urrea et al., 2005). T. rangeli displays varying degrees of pathogenicity in its vector, inducing behavioral and physiological variations (Andrade et al., 2023; Duarte da Silva and Guarneri, 2023).
Triatomines and mammals, including chronic Chagas disease patients, can be naturally coinfected by T. rangeli and T. cruzi (de Sousa et al., 2008). When identifying vector infections, the co-infection may promote difficulty in distinguishing parasite strains and, therefore, lead to incorrect identification of the etiological agent of Chagas disease (Ramirez et al., 2002; Castillo-Castañeda et al., 2022; Herrera et al., 2022; Vergara-Meza et al., 2022).
T. cruzi and T. rangeli present different life cycles in the vector, where the latter develops into metacyclic trypomastigotes in insect salivary glands. These infective forms are inoculated in vertebrate hosts through insect bites (Eger-Mangrich et al., 2001; Guhl and Vallejo, 2003). T. rangeli life cycle within the invertebrate host begins on trypomastigotes ingestion from infected vertebrates’ bloodstream. Following a brief interval post-infection, within the insect midgut, parasites undergo differentiation into epimastigotes, in its replicative form. These epimastigotes could cross the intestinal epithelium via an intracellular pathway, subsequently accessing the hemocoel within a timeframe ranging from 24 h to several weeks, depending on the parasite strain (Vallejo et al., 2009). In the hemolymph, T. rangeli continues multiplying freely or inside hemocytes, being able to invade salivary glands later on, where it multiplies and transforms into metacyclic trypomastigotes (de Oliveira and de Souza, 2001; Guarneri and Lorenzo, 2017) (Supplementary Material 2).
T. rangeli must overcome the immune responses of midgut, hemocoel, and salivary glands to complete its life cycle in the invertebrate host (Garcia et al., 2009). In the midgut, the parasite faces digestive factors, intestinal microbiota, and immune responses as obstacles. On the other hand, while in the hemocoel, T. rangeli must survive not only the humoral but also the cellular immune responses promoted by hemocytes (Mello et al., 1995; Azambuja et al., 1999; Garcia et al., 2004).
During an infection process, the invading microorganism is recognized by pathogen recognition patterns, which bind to pathogen-associated molecular patterns (Gillespie et al., 1997; Gottar et al., 2006). This process triggers the activation of different immune signaling pathways that increase the vector’s defense responses, both cellular and humoral (Salcedo-Porras and Lowenberger, 2019). In R. prolixus, the main signaling pathways are Toll, which involves the Dorsal transcription factor (TF) and its inhibitor cactus, and the immunodeficiency pathway (IMD), including the Relish TF (Stöven et al., 2000; Zasloff, 2002; Vieira et al., 2018). Essential pathogen recognition patterns related to the IMD pathway are peptidoglycan recognition proteins (PGRPs) that recognize Gram - bacteria (Ferrandon et al., 2007). These pathways, when activated, lead to antimicrobial peptide (AMP) expressions (Bulet et al., 1999; Vieira et al., 2015) such as defensins (A, B, and C) (Lambert et al., 1989; Dimarcq et al., 1990; Vieira et al., 2015; 2016) and prolixicin (Ursic-Bedoya et al., 2011).
R. prolixus has a rich microbiota (Azambuja et al., 2005), composed of a wide variety of microorganisms, including commensal bacteria, such as Rhodococcus rhodnii, a symbiont that plays an essential role in vector physiology, helping in nutrition and vitamin production from complex B (Dillon and Dillon, 2004; Batista et al., 2021). Some strains of Serratia marcescens, a gut commensal bacteria of R. prolixus (Azambuja et al., 2004; da Mota et al., 2012; Da Mota et al., 2019), produce a hemolytic factor aiding blood digestion and exhibiting effective lytic activity against trypanosomatids (Castro et al., 2007a; Castro et al., 2007b; Da Mota et al., 2019). According to our previous work, T. rangeli Macias infection activates AMP gene expression in the insect midgut, impacting gut microbiota by reducing Enterococcaceae levels (Vieira et al., 2015). Hence, digestive tract commensal microbiota can be affected by vector immune system activation post-parasite exposure, therefore influencing the success of the parasite infection (Castro et al., 2012; Vieira et al., 2015; 2016; Batista et al., 2021).
Regarding the tripartite interaction, parasite-insect-microbiota, to complete its biological cycle, T. rangeli needs to overcome the vector humoral and cellular immunity in addition to dealing with the intestinal microbiota, including S. marcescens that could promote epimastigote lysis. Thus, understanding the modulation of insect immune responses during tripartite interaction will favor identifying new parasite-killing potential targets.
Materials and methods
Rhodnius prolixus colony maintenance and ethics statement
R. prolixus was reared at Laboratório de Bioquímica e Fisiologia de Insetos, Instituto Oswaldo Cruz (IOC/Fiocruz) under a relative humidity of 50%–60% at 27°C (Azambuja and Garcia, 1997). Four weeks starved fifth instar nymphs were randomly chosen and fed in an artificial feeding system with defibrinated rabbit blood (Azambuja and Garcia, 1997). The rabbit blood taken by cardiac puncture was provided by the Instituto de Ciência e Tecnologia em Biomodelos, following the Ethical Principles in Animal Experimentation and approved by the Comissão de Ética no Uso de Animais (CEUA/Fiocruz, under the protocol number LW019/17).
Trypanosoma rangeli culture
T. rangeli Macias strain epimastigotes were cultivated in Brain Heart Infusion media (Sigma-Aldrich, São Paulo, Brazil) supplemented with 20% heat-inactivated sterile-filtered bovine fetal serum at 28°C, under sub-cultivation twice a week (Vieira et al., 2015). As documented previously, T. rangeli Macias was selected based on its infectivity rate and capacity to invade R. prolixus hemocele (Machado et al., 2006).
R. prolixus infection with Trypanosoma rangeli
R. prolixus fifth instar nymphs were randomly selected and were infected with T. rangeli. Epimastigotes in the exponential growth phase were quantified in a Neubauer chamber under a phase contrast optical microscope. Defibrinated rabbit blood was previously centrifuged at 2,000 x g for 15 min at 4°C, and the supernatant (plasma) was collected and incubated at 55°C for 30 min to inactivate the plasma complement system (Vieira et al., 2015). Afterward, the plasma was mixed with the blood erythrocytes after adding epimastigotes in a final concentration adjusted to 1 × 106 epimastigotes/mL blood. The blood offered to control insects received the same volume of Brain Heart Infusion medium culture instead of epimastigotes. The blood containing or not T. rangeli was offered to R. prolixus through the artificial membrane-feeding apparatus at 37°C.
Trypanosoma rangeli detection and quantification in Rhodnius prolixus tissues
In three experiments, 52 insects infected with T. rangeli were dissected 7, 15, 21, and 29 days after feeding/infection (DAF). Insect’s hemolymph, midgut, and salivary glands were individually collected, placed in 1.5 mL microtubes, and homogenized in PBS to confirm and quantify T. rangeli infection. Parasites were counted using a Neubauer chamber under a light contrast microscope and expressed as parasites/mL. For parasite quantification in hemolymph samples, the insects had their first pair of legs cut off, and individual drops of hemolymph were immediately stored in 1.5 mL microtubes. For parasite detection in R. prolixus salivary glands, the glands were dissected by removing the pronotum using tweezers and scissors to expose the pair of salivary glands. The tissue was stored in 1.5 mL microtubes containing 50 µL PBS and macerated with pistils. To collect the midgut, the abdominal cuticle was removed, and midgut samples were collected separately, each placed in sterile 1.5 mL microtubes containing 90 µL PBS.
R. prolixus tissue collection for microbiota and molecular biology analysis
Midgut, fat body, and salivary glands were collected 1 and 7 days after T. rangeli infection in three pools of five insects (n = 15), as described above. However, R. prolixus salivary glands were only collected 7 days after feeding with T. rangeli, considering the time the parasite takes to invade the insect hemolymph as well as salivary glands, as described previously (Hecker et al., 1990; Garcia et al., 2012; Paim et al., 2013; Ferreira et al., 2015).
The collection of R. prolixus tissues for qPCR and microbiota analysis was carried out under sterile conditions. Microbiota was studied in midgut samples 7 DAF. Tissues were immediately placed in sterile, empty 1.5 mL tubes and then conditioned in dry ice, for rapid freezing and stored at −80°C.
Gene expression quantification
Gene expression analyses were carried out using cDNA from the fat body, midgut, and salivary glands of R. prolixus. Firstly, total RNA was extracted and quantified using the NucleoSpin ® RNA II kit (Macherey-Nagel) and the NanoDrop 2000 (Thermo Scientific) respectively. The cDNA was made using the First-Strand cDNA Synthesis kit (GE Healthcare), following the manufacturer’s protocol, from 2.5 µg total RNA. For RT-qPCR, the GoTaq® qPCR Master Mix kit (PROMEGA) used to analyze the expression of AMPs (RpdefA, RpdefB, RpdefC, Rpprol) and genes related to the Toll and IMD pathways (Rpdorsal, Rpcactus, and Rprelish), which were normalized based on constitutive R. prolixus genes expression (α-tubulin and GAPDH). The specific primers for the genes of interest, as well as the constitutive genes of R. prolixus, were designed and used as previously published: α-tubulin and GAPDH (Paim et al., 2012), RpdefA, RpdefB and RpdefC (Lopez et al., 2003; Vieira et al., 2016), Rpprol (Ursic-Bedoya et al., 2011; Vieira et al., 2016), Rpcactus (Ribeiro et al., 2014), Rprelish, Rpdorsal (Mesquita et al., 2015).
For microbiota analysis, the relative expression of S. marcescens, R. rhodnii, and Enterococcaceaee 16S-rRNA genes was analyzed in midgut samples collected at 7 DAF as previously described (Vieira et al., 2016). These bacteria species were chosen based on relevant evidence from previous publications (Vieira et al., 2015; da Mota et al., 2019). Bacterial 16S-rRNA and R. prolixus gene expressions were calculated using the ΔΔCT (relative quantification) method (Livak and Schmittgen, 2001). The RT-qPCR reaction was performed on the 7,500 equipment (Applied Biosystems). PCR details: initial denaturation at 95°C for 20 s, denaturation at 95°C for 3 s, annealing and extension for 30 s, repeated 40 times. The final extension occurred at 72°C following a melting curve analysis. Secondary analyses were carried out based on Vieira (2016), using the Expression Suite v1.0.3 software (Life Technologies), considering the amplification efficiency of each target (Vieira et al., 2016; 2018). Primers used in the present work are described in Supplementary Table.
Hemocyte and nodule quantification
R. prolixus hemolymph samples (10 µL) were individually collected, placed in 1.5 mL microtubes, and immediately mixed with 10 µL anticoagulant solution (0.01 M ethylenediamine tetraacetic acid, 0.1 M glucose, 0.062 M sodium chloride, 0.03 M trisodium citrate, 0.026 M citric acid, pH 4.6) (Garcia and Azambuja, 1991) at 2, 7, and 12 DAF. Hemocytes and nodule formations in 10 µL hemolymph/anticoagulant solution were quantified using a Neubauer chamber and a phase-contrast optical microscope. Only clusters from five or more hemocytes were considered as hemocyte microaggregation (Garcia et al., 2004).
Phenoloxidase assays
Phenoloxidase (PO) activities were assessed in freshly collected hemolymph from the fifth instar nymphs at 7 and 12 DAF. 10 μL hemolymph volume was collected from individual insects and diluted in 200 µL ultrapure water, followed by centrifugation at 10,000 x g for 10 min. The supernatant was further diluted tenfold for analysis. Each group contained five insects, and the experiments were performed in triplicate (n = 15). Hemolymph samples (25 μL) were mixed with 10 μL cacodylate-CaCl2 buffer (10 mM sodium cacodylate, 10 mM CaCl2, pH 7.4) in 96-well plates. Subsequently, 25 μL saturated solution of DOPA (4 mg/mL) was added to each well and then incubated at 28°C in a Spectra Max 190 Microplate Reader (Molecular Devices, California, United States) at 37°C for 120 min. The absorbance at 490 nm, indicative of dopachrome formation from DOPA, was continuously monitored in the hemolymph samples. PO activity was quantified and expressed as absorbance/min × 100, then calculated based on the dopachrome formation, as formerly described (Genta et al., 2010).
Trypanolytic activity
S. marcescens RPH1 was grown in Brain Heart Infusion Agar medium for 24 h. A colony was isolated and cultivated in 20 mL Brain Heart Infusion Broth for 18 h at 30°C and 90 rpm. The bacterial culture in the stationary growth phase was collected and adjusted to a concentration of 1 × 108 CFU/mL. T. rangeli Macias epimastigotes were grown to a concentration of 2.5 × 106 parasites/mL in the exponential growth phase and then incubated with S. marcescens RPH1 for 120 min at 30°C. After the incubation, motile parasites were counted using a Neubauer chamber and an optical microscope with phase contrast at ×40 magnification (Castro et al., 2007a).
Statistical analysis
Results were analyzed by GraphPad Prism 5.0 program using 1 Way ANOVA, normality test, t-test (unpaired), or Mann Whitney test (nonparametric test) depending on data distribution and number of treatments. Differences between groups are considered not statistically significant when p > 0.05.
Results
Trypanosoma rangeli infection
R. prolixus infected with T. rangeli were dissected at 7, 15, 21, and 29 days after feeding/infection (DAF). Parasites were detectable in midgut samples starting at 15 DAF, with an average of 1,5 × 106 parasites/mL in the digestive tract. At 29 DAF, we found 1 × 104 parasites/mL in the salivary glands of two insects. Then, 55.8% of the nymphs were infected.
Relative expression of humoral immunity genes in R. prolixus infected by Trypanosoma rangeli
T. rangeli infection modulated factors that regulate AMP transcription in different R. prolixus tissues. Rpdorsal TF expression, related to the Toll pathway, was significantly higher in the midgut (p < 0.05) and fat body (p < 0.01) of T. rangeli-infected insects than in control at 1 DAF, 2.5-fold higher in the latter (p < 0.01; Figure 1). On the other hand, Rpcactus, the Toll pathway inhibitor, was negatively modulated by T. rangeli infection in the insect midgut at 1 DAF (p < 0.05), but not in the fat body (Figure 1). The Rprelish gene expression, the IMD pathway transcription factor, was not significantly modulated by T. rangeli infection in both midgut or fat body at 1 DAF compared to non-infected insects (Figure 2). Although, we observed a high expression level of the RpPGRP gene receptor (a component of the IMD pathway) in the midgut (p < 0.01) and fat body (p < 0.01) on T. rangeli-infected insects 1 DAF (Figure 2).
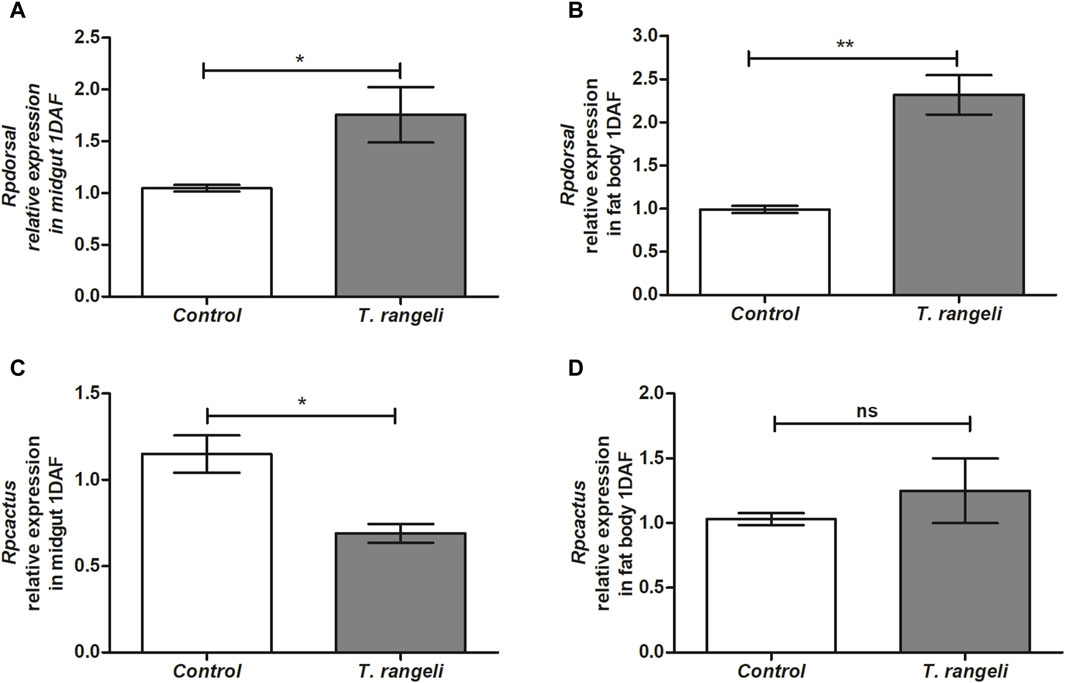
Figure 1. Relative gene expression of components from the Toll pathway in Rhodnius prolixus infected with Trypanosoma rangeli. Rpdorsal and Rpcactus gene expressions were analyzed using the midgut and fat body samples from R. prolixus fifth instar nymphs 1 day after feeding (DAF) with blood containing T. rangeli (106 epimastigotes/mL). Data were quantified using the gene expression of uninfected insects fed on blood as the calibrator (white columns) in comparison with infected insects (gray columns) and shown as the relative expression of (A) Rpdorsal in anterior midgut, (B) Rpdorsal in the fat body, (C) Rpcactus in anterior midgut, (D) Rpcactus in the fat body. Bars represent the mean ± SEM of three independent experiments with three pools of insects (n = 3). Means were compared using Student’s T-test; *p < 0.05, **p < 0.01, ns = non-significant difference.
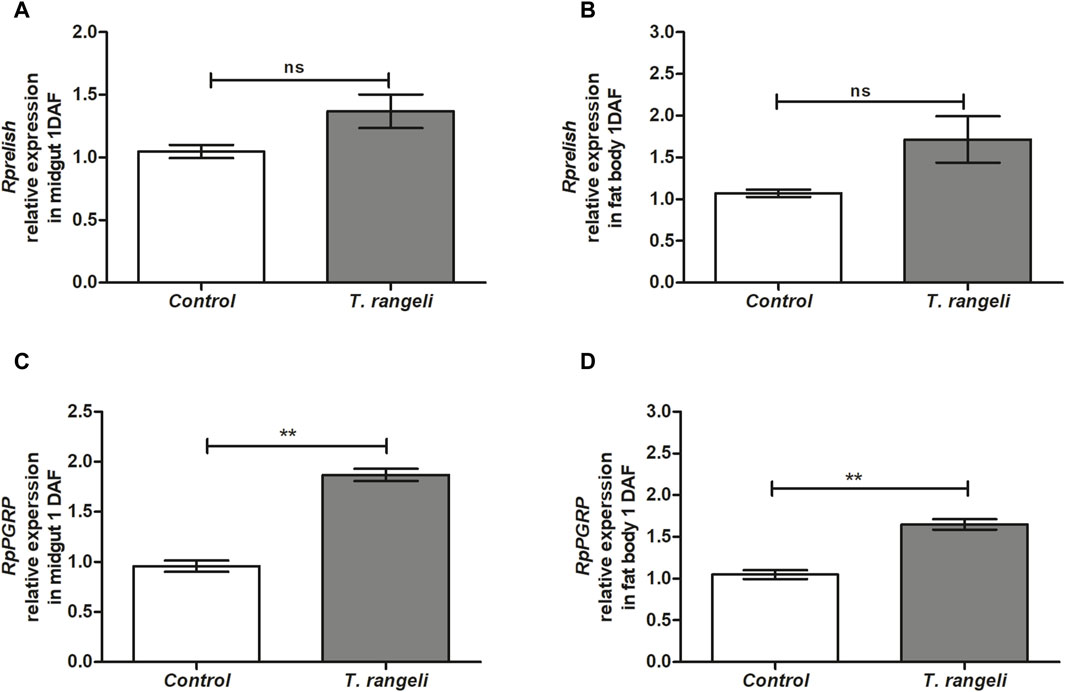
Figure 2. Relative gene expression of components from the IMD pathway in Rhodnius prolixus infected with Trypanosoma rangeli. Rprelish and RpPGRP gene expressions were analyzed using the midgut and fat body samples from R. prolixus fifth instar nymphs 1 day after feeding (DAF) with blood containing T. rangeli 106 epimastigotes/mL). Data were quantified using the gene expression of uninfected insects fed on blood as the calibrator (white columns) in comparison with infected insects (gray columns) and shown as the relative expression of (A) Rprelish in anterior midgut, (B) Rprelish in the fat body, (C) RpPGRP in anterior midgut, (D) RpPGRP in the fat body. Bars represent the mean ± SEM of three independent experiments with three pools of insects (n = 3). Means were compared using Student’s T-test; **p < 0.01, ns = non-significant difference.
AMPs expression in the fat body of R. prolixus infected by Trypanosoma rangeli
In the fat body, the expression of the AMPs genes, RpdefA and RpdefB, as well as Rpprol, was reduced at 1 DAF (Figure 3A) but not changed at 7 DAF (Figures 3A, B). In contrast, AMP RpdefC expression in T. rangeli infected insects was 70 times higher (p < 0.01; Figure 3A) than control insects at 1 DAF and remained high (2.5-fold) at 7 DAF (p < 0.01 Figure 3B).
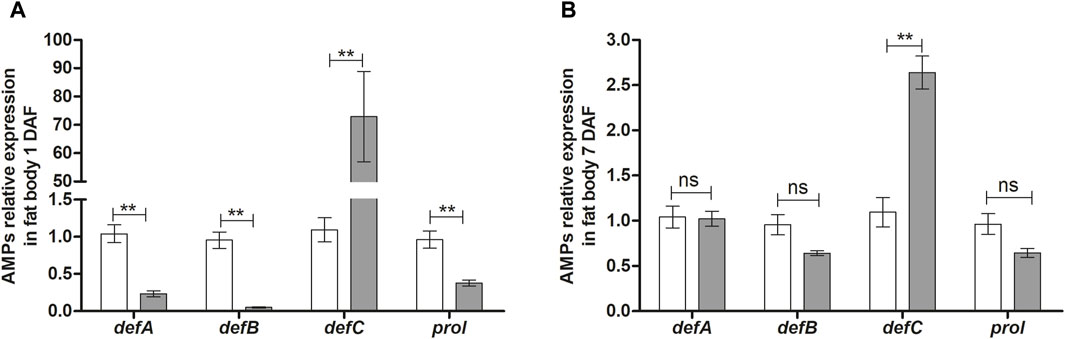
Figure 3. Antimicrobial peptides gene expression in the fat body of Rhodnius prolixus infected with Trypanosoma rangeli. R. prolixus fifth instar nymphs were fed on blood containing T. rangeli epimastigotes (106 parasites/mL of blood). Data were quantified using the gene expression of untreated control insects as the calibrator (white columns). The gray columns show the relative expression of the antimicrobial peptide genes on the (A) 1st and (B) 7th days after feeding insects on blood containing T. rangeli epimastigotes. Relative gene expression of RpdefA, RpdefB, RpdefC, Rpprol. Each bar represents three independent experiments performed in duplicate (n = 6). Means were compared using one-way ANOVA and Student’s t-test; **p < 0.01, ns = non-significant difference.
Expression of NF-kB transcription factors and AMPs in the salivary glands of R. prolixus infected by Trypanosoma rangeli
In T. rangeli-infected insect’s salivary glands, the TF Dorsal expression was not modulated at 7 DAF (Figure 4A). On the other hand, the Rprelish gene was downregulated in the same group (p < 0.01; Figure 4B). In the context of the AMP’s expression, T. rangeli infection induced suppression of RpdefA (p < 0.01) and RpdefB (p < 0.001) gene expression, while upregulated RpdefC (p < 0.01) expression in R. prolixus salivary glands at 7 DAF (Figure 4C). Rpprol was not modulated by parasite infection (Figure 4C).
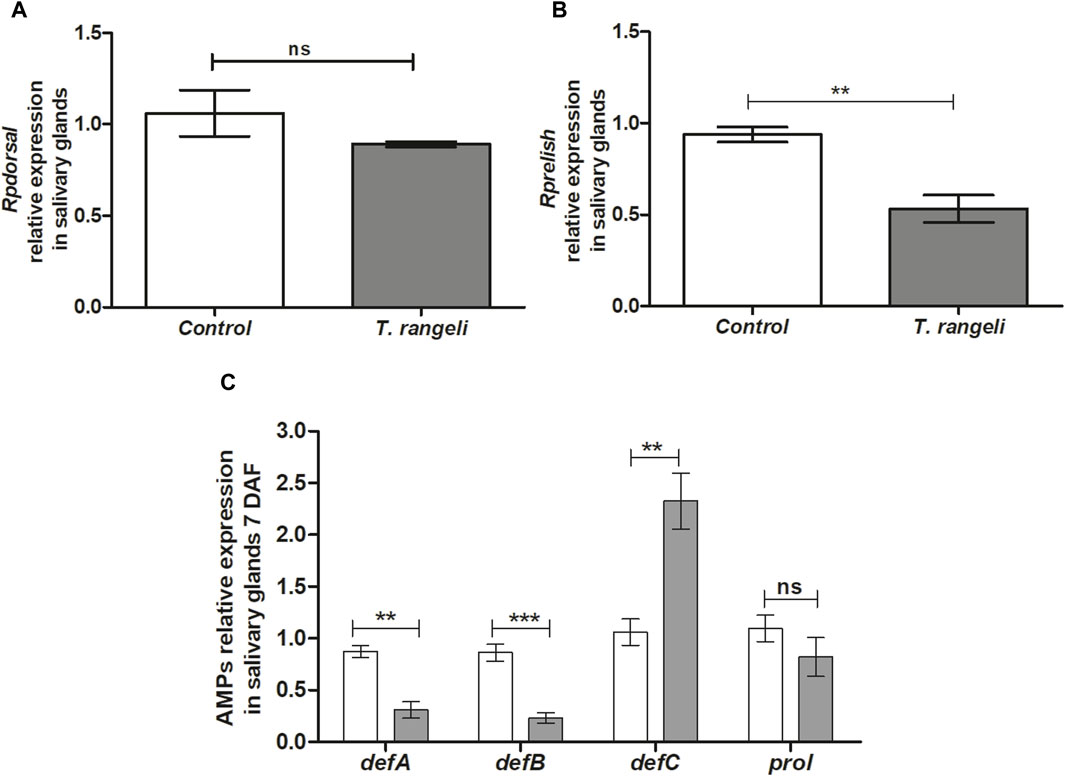
Figure 4. NF-kB transcription factors and antimicrobial peptides gene expression in the salivary glands of Rhodnius prolixus oral infected with Trypanosoma rangeli. Fifth instar nymphs of R. prolixus were fed on blood containing T. rangeli epimastigotes (106 parasites/mL of blood). Data were quantified using the gene expression of untreated control insects as the calibrator (white columns). The grey columns show the relative expression of the (A) Rpdorsal (B)- Rprelish and (C) antimicrobial peptides genes (RpdefA, RpdefB, RpdefC, Rpprol), analyzed at 7 days after feeding (DAF). Each bar represents three independent experiments performed in duplicate (n = 6). Means were compared using one-way ANOVA and Student’s t-test; ***p < 0.001, **p < 0.01, ns = non-significant difference.
Modulation of hemocyte number and microaggregation in R. prolixus after Trypanosoma rangeli infection
Hemocyte quantification and microaggregation in R. prolixus infected hemolymph were evaluated at 2, 7 and 12 DAF. No significant differences between control and infected insects were detected in hemocyte number at 2 and 7 DAF (Figure 5A). However, at 12 DAF, a significant reduction in this quantification was observed (p < 0.05) (Figure 5A). On the other hand, T. rangeli infection increased hemocyte microaggregation in R. prolixus hemolymph at 7 and 12 DAF (both p < 0.05) (Figure 5B), comparing with control uninfected insects.
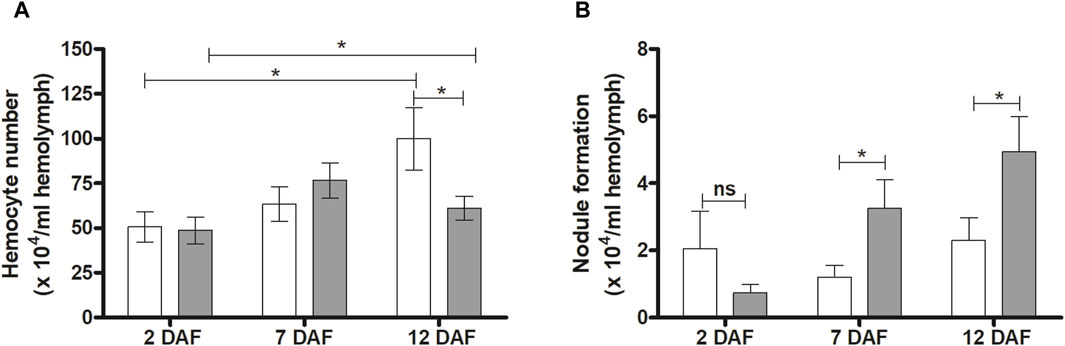
Figure 5. Effects of Trypanosoma rangeli infection on hemocytes and hemocyte microaggregation in Rhodnius prolixus hemolymph. The number of hemocytes (A) and hemocyte microaggregation (B) were counted in the hemolymph of R. prolixus fifth instar nymphs 2, 7, and 12 days after feeding (DAF) with blood containing T. rangeli epimastigotes (106 parasites/mL). White column - control, non-infected insects; grey column - insects infected with T. rangeli. Each data represents the mean of three independent experiments with five insects in each group per day analyzed (n = 15). The vertical bars indicate the standard error (±EP). Controls and treatments were compared each day with a t-test or Mann-Whitney test. *p < 0.05 and ns = non-significant difference.
Phenoloxidase activity in the hemolymph of R. prolixus infected with Trypanosoma rangeli
Phenoloxidase activity in the hemolymph of R. prolixus infected by T. rangeli was examined at 7 and 12 DAF. At 7 DAF, there was a significant increase in PO activity in T. rangeli infected insects (p < 0.001) compared to the control group and a tendency without statistical significance to decrease PO activity at 12 DAF (Figure 6).
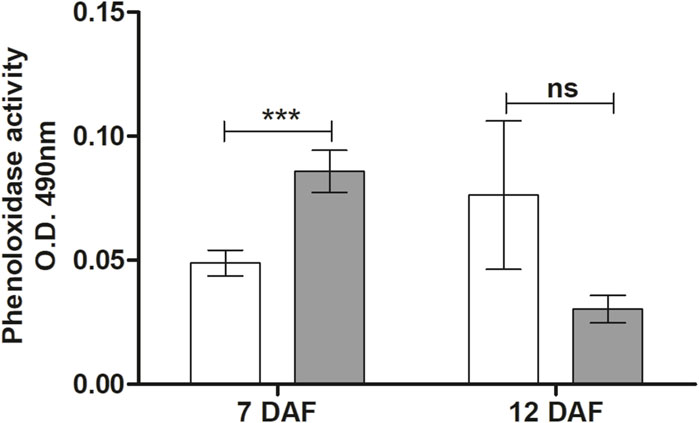
Figure 6. Phenoloxidase activity in the hemolymph of Rhodnius prolixus infected with Trypanosoma rangeli. Phenoloxidase activities were measured in the hemolymph collected from fifth instar nymphs of R. prolixus fed on blood containing T. rangeli epimastigotes (106 parasites/mL of blood) on different days after feeding (7 and 12 DAF). White column - control, not infected insects; grey column - insects infected with T. rangeli. Each data represents the mean of three independent experiments with five insects in each group (n = 15) per day analyzed. The vertical bars indicate the standard error (±SEM). Groups were compared for each day, using the t-test, n = 15 insects. ***p < 0.001 and ns = Non-significant difference.
Changes of R. prolixus midgut microbiota composition after Trypanosoma rangeli infection
The R. prolixus bacterial gut microbiota population was quantified 7 days after T. rangeli infection. According to qPCR the populations of S. marcescens (p < 0.001) and R. rhodnii (p < 0.001) were significantly reduced (Figure 7). On the other hand, bacteria from the Enterococcaceae family exhibited a remarkable population increase in the midgut of insects infected with T. rangeli (p < 0.001) (Figure 7).
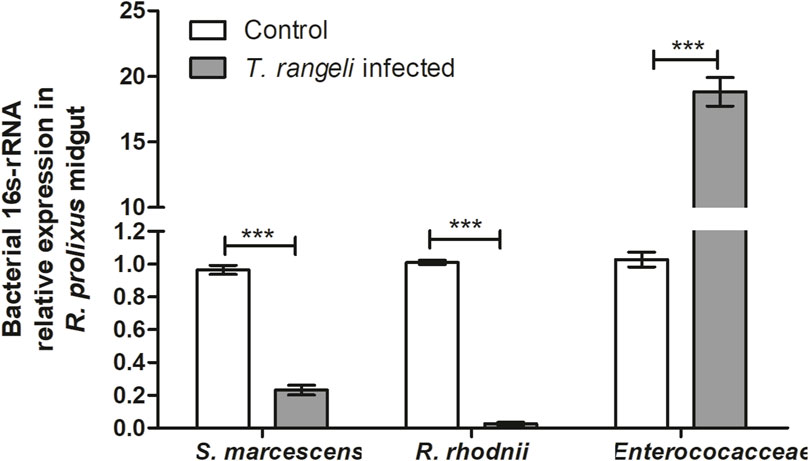
Figure 7. Trypanosoma rangeli infection modifies bacterial microbiota abundance in Rhodnius prolixus midgut. Determination of bacterial load in the midgut of R. prolixus fifth instar nymphs fed with blood containing T. rangeli epimastigotes (106 parasites/mL). The relative expression of 16S-rRNA of Serratia marcescens, Rhodococcus rhodnii, and Enterococcaceae was evaluated by RT-qPCR. Data were normalized to the R. prolixus 18S RNA gene and calculated using the gene expression of control insects fed on blood as the calibrator. White column - control insects fed on blood; gray column–insects fed on blood containing T. rangeli. Bars represent the mean ± SEM of three independent experiments with ten insects (n = 30). Means were compared using the Student’s T-test; ***p < 0.001.
The Serratia marcescens RPH1 trypanolytic activity against Trypanosoma rangeli
The lytic activity of S. marcescens RPH1 was tested in vitro against the T. rangeli Macias strain. Two hours after parasite incubation with S. marcescens, a significant trypanolytic effect against T. rangeli was observed, resulting in a decrease of 50% in the parasite population (p < 0.05) (Figure 8).
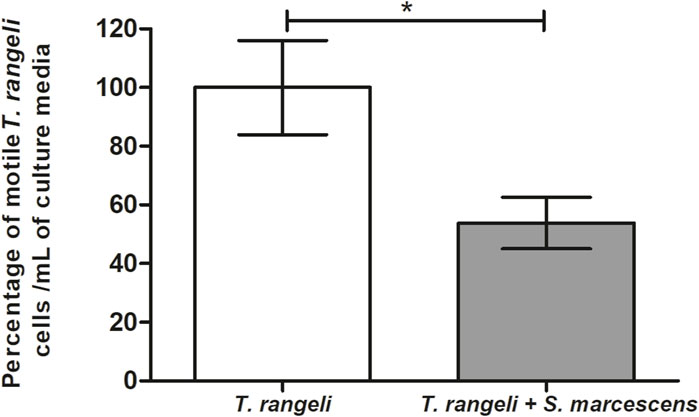
Figure 8. Trypanolytic activity of Serratia marcescens RPH1 on Trypanosoma rangeli. The trypanolytic activity of S. marcescens (S.m) RPH1 strain against T. rangeli (T.r) epimastigotes was tested by incubating both microorganisms for 2 hours at 30°C. The vertical axis represents the percentage of intact parasites/mL compared to the controls without the bacteria (0%). Bars represent the mean ± SEM of three independent experiments. The asterisks above the bars indicate statistical significance calculated using a t-test. *p < 0.05.
Discussion
Detecting non-self-components is crucial to ensure animal survival. Insect vectors have the difficult task of dealing with parasite infection, immune system activation, and microbiota maintenance, an uneasy balance that could cost insect fitness and life span. Primarily, the insects rely on phylogenetically germ-line encoded recognition molecules within their innate immunity to identify and kill microbes, thereby avoiding local and systemic infections (Janeway and Medzhitov, 2002; Ferrandon et al., 2007). In nature, R. prolixus could be infected by T. cruzi, T. rangeli, or both simultaneously (Ramirez et al., 2002; Castillo-Castañeda et al., 2022; Herrera et al., 2022; Vergara-Meza et al., 2022). The molecular mechanisms used by R. prolixus to recognize T. rangeli infection and how the parasite modulates specific insect immune effectors and gut microbiota have not been fully explored. However, T. cruzi is recognized by R. prolixus through NF-kB signaling pathways (Vieira et al., 2018), which ultimately leads to the activation of AMP, PO cascades, as well as RNS and ROS in insect gut (Castro et al., 2012; Vieira et al., 2016; Vieira et al., 2018; Batista et al., 2020). Notably, the Toll and IMD pathways have been the most studied immune signaling pathways in R. prolixus (Mesquita et al., 2015; Vieira et al., 2018; Vieira et al., 2021; Salcedo-Porras et al., 2019; Salcedo-Porras and Lowenberger, 2019). The present study sheds light on the modulation of Toll and IMD signaling pathways in R. prolixus infected with T. rangeli not only in the midgut (local immune response) but also in the fat body and salivary glands (systemic and local immune responses) and the effects on AMP expression, PO activity, hemocyte microaggregation, and microbiota homeostasis.
Even though R. prolixus possesses a well-orchestrated mechanism for eliminating invading pathogenic microorganisms (Azambuja et al., 2017; Salcedo-Porras and Lowenberger, 2019), T. rangeli establishes infection in insect midgut as epimastigotes and activate R. prolixus local immune responses (Vieira et al., 2015; Rolandelli et al., 2021) which in turn affects insect microbiota (Vieira et al., 2015). Before invading the insect hemocoel, the T. rangeli oral infection leads to immunosuppression of the cellular and humoral immune responses (Whitten et al., 2001; Gomes et al., 2003; Garcia et al., 2004; Figueiredo et al., 2008). This process of hemocoel’s immune response modulation before the parasite invasion is probably correlated to interorgan communication and participation of signaling molecules such as nitric oxide and eicosanoids, as observed in mosquitos (Das et al., 2018). Then, these epimastigotes traverse the midgut epithelium, invade the insect hemolymph, and evade R. prolixus immune responses through various molecular mechanisms (Mello et al., 1995; Garcia et al., 2012; Peterson and Graham, 2016; Ferreira et al., 2018). Finally, the parasite infiltrates salivary glands to complete its life cycle in the invertebrate host (Ellis et al., 1980; Azambuja and Garcia, 2005; Paim et al., 2013).
Herein, we could observe a successful infection of the T. rangeli Macias by encountering parasites in salivary glands of R. prolixus. Although we found few insects with successful infection in salivary glands, several studies demonstrate that crossing the hemocoel, which has potent immune responses, is a bottleneck for developing T. rangeli. Hemolymph infections can occur at frequencies from 2% to 50% in orally infected insects (Hecker et al., 1990). Furthermore, the detection of T. rangeli in salivary glands may vary concerning the days after infection, ranging from 5 to 40 days, depending on the strain of the parasite and the insect vector species (Hecker et al., 1990; Ferreira et al., 2010; Garcia et al., 2012; Paim et al., 2013). This variation in the success of parasite development is related to the capacity of the parasite to modulate the insect’s immune responses (Castro et al., 2012; Vieira et al., 2016).
Oral infection by T. rangeli in R. prolixus modulates cellular immune responses such as microaggregation and phagocytosis by inhibiting the enzyme phospholipase A2, which initiates the eicosanoids and platelet activation factor pathways (Garcia et al., 2004; Garcia et al., 2009; Machado et al., 2006; Figueiredo et al., 2008; Kim et al., 2018). However, no studies have demonstrated the recognition of T. rangeli by R. prolixus pattern recognition receptors, in general, related to Toll or IMD pathways.
In this work, we detected the Toll pathway activation by significantly increasing Rpdorsal TF expression in the midgut and fat body of infected insects with T. rangeli Macias. Furthermore, its inhibitor (Rpcactus) expression was reduced on nymphs’ midgut 1 DAF. Therefore, the recognition of T. rangeli activates the Toll pathway by reducing the TF inhibitor, allowing more efficient Rpdorsal TF translocation to the nucleus. This modulation causes an increase in effector molecule synthesis, such as antimicrobial peptides, for parasite control. The Toll pathway is well conserved in R. prolixus (Mesquita et al., 2015); in its genome, the presence of homologous death-domain and protein adaptor genes corresponding to NF-KappaB has been observed (Zumaya-Estrada et al., 2018; Nishide et al., 2019; Salcedo-Porras and Lowenberger, 2019).
When R. prolixus was orally treated with IMD-0354, a drug that blocks NF- KappaB translocation to the nucleus and challenged with E. coli or Serratia aureus, it was a rapid increase in Rp-Cactus expression (1 DAF) (Vieira et al., 2018). Lately, 7 days after bacterial challenge, the mRNA levels of Rpcactus decreased significantly in the vector’s midgut (Vieira et al., 2018). In this context, we note an autoregulation profile on inhibitor expression, where the parasite is initially present. T. rangeli triggers an effective expression of Rpcactus with joint action of the Rpdorsal TF. But, during the interaction with the pathogen, the gene transcript level is controlled. In D. melanogaster, the Cactus gene and its isoforms are induced in the face of pathogens, being rapidly degraded when released into the cytosol but reinduced when necessary, reinforcing the autoregulation scenario in invertebrates after exposure and humoral immunity action against invading pathogen (Nicolas et al., 1998).
When observing a reduction of Rp-cactus in the midgut, it is essential to regard that this is an important region for T. rangeli development since it is where the parasite migrates from midgut to hemolymph and that the Toll pathway activation triggers a cascade of signals inducing effector genes transcription and pathogen control (Zumaya-Estrada et al., 2018).
The parasite must reach the insect’s salivary glands to effectively complete the T. rangeli life cycle and subsequent transmission to a vertebrate host. Notably, activating immune responses in the midgut directly influences the parasite’s ability to establish a successful infection. Prolonged infections with T. rangeli strain CHOACHI revealed a notable observation: insects hosting the parasite in their gut, rather than in the hemolymph, exhibited a significant upregulation of Rpcactus inhibitor transcription in the midgut (Rolandelli et al., 2021). Therefore, diverse T. rangeli strains may distinctly impact specific aspects of humoral immunity in the vector. In the present work, we observed IMD pathway activation, an R. prolixus pathway not wholly identified in the genome (Mesquita et al., 2015). However, bioinformatic analyses later identified these elements in R. prolixus (Salcedo-Porras and Lowenberger, 2019) and other triatomine species (Zumaya-Estrada et al., 2018). Intent to explore a primary immune response by evaluating T. rangeli Macias infection, Rprelish TF expression was not modulated on both fat body and midgut. However, knowing that pathogen recognition patterns mediate the initial recognition of an infection, we also evaluate PGRP expression, which is a receptor associated with IMD pathway activation. Since RpPGRP expression in both midgut and fat body 1 DAF was increased, these results indicate that T. rangeli infection in R. prolixus is recognized by the expression of PGRPs. A reduction in AMP expression was shown after PGRPs silencing the Hemiptera Plautia stali and its infection by Gram + and Gram–bacteria (Nishide et al., 2019). R. prolixus Rprelish TF silencing controls humoral effectors, leading to Defensin C inhibition in insects challenged with Gram + and Gram - bacteria (Salcedo-Porras and Lowenberger, 2019), as well as reduced defensin A and increased lysozyme A in the midgut of insects challenged with T. cruzi (Mesquita et al., 2015).
The expression of AMPs directly depends on the activation of signaling pathways. R. prolixus challenged with T. rangeli Macias presents an increase in the expression of RpdefC in fat body 1 and 7 DAF, while in salivary glands the upregulation occurred at 7 DAF. When R. prolixus was challenged with T. cruzi Dm28c, the expression of these AMPs was also shown to be elevated in the midgut of insects 7 DAF (Vieira et al., 2018). In contrast, when these insects are challenged by Gram + and Gram - bacteria, RpdefC expression is increased in the midgut at 24 h post infection but reduces over days (Vieira et al., 2014). This dynamic pattern of AMP expression reflects the insect’s response to different types of pathogens over time.
Still, little is known about which signaling pathway is responsible for expressing such specific AMPs in R. prolixus to control the invading organism. In our results, T. rangeli stimulates Rp-dorsal expression and inhibits Rpcactus. Consequently, we observed a significant increase in RpdefC expression. Oppositely, the parasite negatively modulated RpdefA, RpdefB, and Rpprol. Together, these results indicate that T. rangeli activates the Toll pathway in R. prolixus, which regulates the expression of RpdefC, in agreement with the previous publication (Vieira et al., 2015). On the other hand, T. rangeli infection seems to inhibit the IMD pathway, which explains the suppression of RpdefA and RpdefB.
Regarding cellular immune responses modulated by T. rangeli Macias oral infection in R. prolixus, we observed increased PO activity and nodule formation. The prophenoloxidase system is a rapid response process that occurs independently of gene expression (Cerenius et al., 2010). It uses the enzyme PO to produce enzymatic groups polymerized into melanin. These events are triggered when an insect suffers an injury or infection by a pathogen, leading to the melanization of invading microorganisms (Söderhäll and Cerenius, 1998; Christensen et al., 2005; González-Santoyo and Córdoba-Aguilar, 2012). Triatomines respond differently depending on the challenge they receive. The presence of T. rangeli in R. prolixus hemolymph inhibits PO activity (Gregório and Ratcliffe, 1991). When R. prolixus was inoculated with short epimastigotes of T. rangeli strain H14, it caused an increase in PO activity. However, when oral feeding was carried out with different forms of T. rangeli H14 epimastigotes, it caused a suppression of the PO system (Gomes et al., 2003). T. rangeli Macias oral infection also reduced the PO activity in R. prolixus, referring to spontaneous and total PO. However, this effect is not immediately observed, occurring only 12 days after feeding (Mattos, 2014; Vieira et al., 2015). Herein, we observed increased PO activity in the hemolymph of R. prolixus infected with T. rangeli at 7 DAF and no differences at 12 DAF compared to non-infected insects. Although we did not detect a significant difference, 12 DAF, an apparent reduction in PO activity can be observed.
Also, in the hemocele, the hemocytes can aggregate, forming nodules to fight microorganisms (Ratcliffe and Gagen, 1977; Satyavathi et al., 2014). We investigated hemocyte recruitment at 2 and 7 DAF. Here we observed a decrease in total free hemocytes in insects infected with T. rangeli at 12 DAF, compared to control insects. The formation of nodules depends on the aggregation of hemocytes, which reduces the presence of circulating hemocytes in the hemolymph. At 7 and 12 DAF, insects infected with T. rangeli strain Macias showed a significant increase in the population of nodules present in the hemolymph of R. prolixus, which could explain the decrease in hemocytes number at 12 DAF in infected insects compared to control.
In vitro, infection by T. rangeli stimulates the formation of nodules in R. prolixus hemolymph. However, when the T. rangeli infection occurs in vivo, through parasite injection into the R. prolixus hemocoel, there is an increase in the hemocyte population, but without significant stimulation in the formation of nodules (Gomes et al., 1999). Here, T. rangeli infection was performed orally through blood offered to R. prolixus. The presence of the parasite at 12 DAF caused a reduction in the hemocyte population and significantly increased the formation of hemocyte microaggregates (nodule formation) in R. prolixus hemolymph. The isolation of a lectin present in the hemolymph of R. prolixus impacts the motility of T. rangeli strain H14 and increases the formation of T. rangeli clusters in the hemolymph (Mello et al., 1999).
Alterations in insect immune responses can significantly impact the intestinal microbiota, particularly the modulation of antimicrobial peptides (AMPs). Our study reveals notable changes in the bacterial microbiota composition of R. prolixus infected with T. rangeli Macias, substantially reducing the populations of R. rhodnii and S. marcescens. Our results agree with former publications where the authors observed T. rangeli infections impact the R. rhodnii population in R. prolixus anterior midgut (Watkins, 1971; Eichler and Schaub, 2002). Previous investigations employing pyrosequencing on R. prolixus infected with the T. rangeli Macias strain did not detect differences in the number of sequences from the Nocardiaceae and Enterobacteriaceae families, associated with R. rhodnii and S. marcescens (Vieira et al., 2015). However, a decrease in Enterococcaceae and an increase in Burkholderiaceae were documented in T. rangeli infected insects (Vieira et al., 2015). Interestingly, a reduction in S. marcescens and R. rhodnii populations was observed in the midgut of R. prolixus infected with the T. cruzi Dm28c strain (Vieira et al., 2016).
Once AMP expression is differentially regulated in the midgut depending on the species and strains of parasite-infected insects, it impacts the microbiota composition inside the intestinal tract, where certain species are negatively modulated, and others are positively modulated.
Defensin C is correlated with bacterial microbiota regulation of R. prolixus (Vieira et al., 2015; Vieira et al., 2016; Vieira et al., 2018; Vieira et al., 2021). Vieira et al. (2015) observed an increase in RpdefB and RpdefC levels and a decrease of cultivable bacteria in the anterior midgut in short-term T. rangeli Macias strain infected R. prolixus. Moreover, in T. rangeli, a long-term infection caused a massive upregulation of RpdefC in the posterior midgut and decreased the bacteria population (Vieira et al., 2015). In R. prolixus infected with T. cruzi Dm28c, there was an increase in RpdefC and Rpprol expression levels in the anterior midgut and a drastic reduction of S. marcescens and R. rhodnii 16S gene expression (Vieira et al., 2016). Immunodepression of R. prolixus by treating the insects with IMD-0354, a selective inhibitor of IkB kinases, downregulated the expression of defensins, caused a reduced antibacterial activity of the insect anterior midgut against S. marcescens and an intense proliferation of the bacteria detected by 16S-RNA relative expression of S. marcescens, R. rhodnii and bacteria of the Enterococacceae family (Vieira et al., 2018). Also, the immune depression of R. prolixus by treatment with azadirachtin, an ecdysone inhibitor, caused a reduction in the transcription level of RpdefC (almost 200-fold) and an increase in the load of S. marcescens 16SRNA expression level (Vieira et al., 2021).
Considering all these observations collectively, it can be inferred that T. rangeli infection triggers the activation of the Toll signaling pathway in R. prolixus, thereby inducing the synthesis of defensin C, which plays a pivotal role in modulating the intestinal microbiota, particularly targeting S. marcescens. The relationship between gut-microbiota and pathogens transmitted by insect vectors is complex and it can be observed in different types of vectors, where pathogens can modify the microbial load in the midgut and/or the composition of the bacterial population (Gabrieli et al., 2021).
This complex modulation of the insect’s immune system and microbiota through T. rangeli infection could be the reason for the impact observed on the insect’s physiology as a trade-off (Guarneri et al., 2017; Ferreira et al., 2018).
Conclusion
In the present investigation, it became clear that the activation of the vector humoral immunity plays a pivotal role in the success of T. rangeli infection. T. rangeli Macias seems to be recognized mainly by the Toll pathway, which regulates RpdefC expression. Immunity modulation by T. rangeli substantially influences the population dynamics of S. marcescens in the insect midgut, thereby helping parasite evasion of the toxic trypanolytic effects exerted by microbiota, favoring parasite establishment in the vector. Parallel, during its invasion into the insect hemocele, T. rangeli distinctly engages with R. prolixus cellular immunity. Notably, T. rangeli leads to a marked reduction in the population of circulating hemocytes, accompanied by a significant increase in nodule formation. Also, even inside insect salivary glands, T. rangeli faces the activation of AMPs. Despite the activation of insect immune responses and release of immune effectors, T. rangeli successfully crosses the triatomine’s midgut, colonizes the insect’s hemolymph, and invades salivary glands, completing its life cycle.
Data availability statement
The original contributions presented in the study are included in the article/Supplementary Material, further inquiries can be directed to the corresponding author.
Ethics statement
The animal study was approved by Instituto de Ciência e Tecnologia em Biomodelos (ICTB), following the Ethical Principles in Animal Experimentation approved by the Comissão de Ética no Uso de Animais 2 (CEUA/Fiocruz, under the protocol number LW019/17). The study was conducted in accordance with the local legislation and institutional requirements.
Author contributions
SP: Formal Analysis, Methodology, Writing–original draft, Writing–review and editing, Investigation, Validation. DM: Formal Analysis, Methodology, Writing–original draft, Writing–review and editing, Investigation. MG: Data curation, Formal Analysis, Supervision, Writing–original draft, Writing–review and editing. CM: Writing–original draft, Writing–review and editing, Data curation, Formal Analysis, Visualization. PA: Conceptualization, Methodology, Supervision, Writing–original draft, Writing–review and editing, Funding acquisition, Project administration. DC: Investigation, Methodology, Writing–original draft, Writing–review and editing, Conceptualization, Resources, Supervision, Validation. CV: Data curation, Formal Analysis, Visualization, Writing–original draft, Writing–review and editing, Conceptualization, Methodology, Project administration, Supervision.
Funding
The author(s) declare that financial support was received for the research, authorship, and/or publication of this article. This research was funded by Fundação Oswaldo Cruz (Fiocruz), Conselho Nacional de Desenvolvimento Científico e Tecnológico (CNPq), Fundação de Amparo a Pesquisa do Estado do Rio de Janeiro (FAPERJ), and Instituto Nacional de Ciência e Tecnologia em Entomologia Molecular (INCT-EM).
Conflict of interest
The authors declare that the research was conducted in the absence of any commercial or financial relationships that could be construed as a potential conflict of interest.
Publisher’s note
All claims expressed in this article are solely those of the authors and do not necessarily represent those of their affiliated organizations, or those of the publisher, the editors and the reviewers. Any product that may be evaluated in this article, or claim that may be made by its manufacturer, is not guaranteed or endorsed by the publisher.
Supplementary material
The Supplementary Material for this article can be found online at: https://www.frontiersin.org/articles/10.3389/fphys.2024.1435447/full#supplementary-material
References
Andrade L. C., Majerowicz D., Oliveira P. L., Guarneri A. A. (2023). Alterations in the energy metabolism of Rhodnius prolixus induced by Trypanosoma rangeli infection. Insect Biochem. Mol. Biol. 159, 103987. doi:10.1016/j.ibmb.2023.103987
Azambuja P., Garcia E. S. (1997). “Care and maintenance of triatomine colonies,” in The Molecular Biology of Insect Disease Vectors: a Methods Manual. Editors J. M. Crampton, C. B. Beard, and K. Louis (London: Chapman & Hall), 56–64.
Azambuja P., Feder D., Garcia E. S. (2004). Isolation of Serratia marcescens in the midgut of Rhodnius prolixus: impact on the establishment of the parasite Trypanosoma cruzi in the vector. Exp. Parasitol. 107, 89–96. doi:10.1016/j.exppara.2004.04.007
Azambuja P., Feder D., Mello C., Gomes S., Garcia E. S. (1999). Immunity in Rhodnius prolixus: trypanosomatid-vector interactions. Mem. Inst. Oswaldo Cruz. 94, 219–222. doi:10.1590/S0074-02761999000700035
Azambuja P., Garcia E. S. (2005). Trypanosoma rangeli interactions within the vector Rhodnius prolixus - a mini review. Mem. Inst. Oswaldo Cruz. 100, 567–572. doi:10.1590/S0074-02762005000500019
Azambuja P., Garcia E. S., Ratcliffe N. A. (2005). Gut microbiota and parasite transmission by insect vectors. Trends Parasitol. 21, 568–572. doi:10.1016/j.pt.2005.09.011
Azambuja P., Garcia E. S., Waniek P. J., Vieira C. S., Figueiredo M. B., Gonzalez M. S., et al. (2017). Rhodnius prolixus: from physiology by Wigglesworth to recent studies of immune system modulation by Trypanosoma cruzi and Trypanosoma rangeli. J. Insect Physiol. 97, 45–65. doi:10.1016/j.jinsphys.2016.11.006
Batista K. K. S., Vieira C. S., Figueiredo M. B., Costa-Latgé S. G., Azambuja P., Genta F. A., et al. (2021). Influence of Serratia marcescens and Rhodococcus rhodnii on the humoral immunity of Rhodnius prolixus. Int. J. Mol. Sci. 22, 10901. doi:10.3390/ijms222010901
Batista K. K. S., Vieira C. S., Florentino E. B., Caruso K. F. B., Teixeira P. T. P., Moraes C. S., et al. (2020). Nitric oxide effects on Rhodnius prolixus's immune responses, gut microbiota and Trypanosoma cruzi development. J. Insect Physiol. 126, 104100. doi:10.1016/j.jinsphys.2020.104100
Bulet P., Hetru C., Dimarcq J. L., Hoffmann D. (1999). Antimicrobial peptides in insects; structure and function. Dev. Comp. Immunol. 23, 329–344. doi:10.1016/S0145-305X(99)00015-4
Castillo-Castañeda A. C., Patiño L. H., Zuñiga M. F., Cantillo-Barraza O., Ayala M. S., Segura M., et al. (2022). An overview of the trypanosomatid (Kinetoplastida: Trypanosomatidae) parasites infecting several mammal species in Colombia. Parasit. Vect. 15, 471. doi:10.1186/s13071-022-05595-y
Castro D. P., Moraes C. S., Garcia E. S., Azambuja P. (2007a). Inhibitory effects of d-mannose on trypanosomatid lysis induced by Serratia marcescens. Exp. Parasitol. 115, 200–204. doi:10.1016/j.exppara.2006.08.001
Castro D. P., Moraes C. S., Gonzalez M. S., Ratcliffe N. A., Azambuja P., Garcia E. S. (2012). Trypanosoma cruzi immune response modulation decreases microbiota in Rhodnius prolixus gut and is crucial for parasite survival and development. PLoS One 7, e36591. doi:10.1371/journal.pone.0036591
Castro D. P., Seabra S. H., Garcia E. S., de Souza W., Azambuja P. (2007b). Trypanosoma cruzi: ultrastructural studies of adhesion, lysis and biofilm formation by Serratia marcescens. Exp. Parasitol. 117, 201–207. doi:10.1016/j.exppara.2007.04.014
Cerenius L., Kawabata S., Lee B. L., Nonaka M., Söderhäll K. (2010). Proteolytic cascades and their involvement in invertebrate immunity. Trends Biochem. Sci. 35, 575–583. doi:10.1016/j.tibs.2010.04.006
Christensen B. M., Li J., Chen C. C., Nappi A. J. (2005). Melanization immune responses in mosquito vectors. Trends Parasitol. 21, 192–199. doi:10.1016/j.pt.2005.02.007
Coura J. R., Junqueira A. C. (2015). Surveillance, health promotion and control of Chagas disease in the Amazon region - medical attention in the Brazilian Amazon region: a proposal. Mem. Inst. Oswaldo Cruz. 110, 825–830. doi:10.1590/0074-02760150153
da Mota F. F., Castro D. P., Vieira C. S., Gumiel M., De Albuquerque J. P., Carels N., et al. (2019). In vitro trypanocidal activity, genomic analysis of isolates, and in vivo transcription of Type VI secretion system of Serratia marcescens belonging to the microbiota of Rhodnius prolixus digestive tract. Front. Microbiol. 9, 3205. doi:10.3389/fmicb.2018.03205
da Mota F. F., Marinho L. P., de Moreira C. J. C., Lima M. M., Mello C. B., Garcia E. S., et al. (2012). Cultivation-independent methods reveal differences among bacterial gut microbiota in triatomine vectors of Chagas disease. PLoS Negl. Trop. Dis. 6, e1631. doi:10.1371/journal.pntd.0001631
Das De T., Sharma P., Thomas T., Singla D., Tevatiya S., Kumari S., et al. (2018). Interorgan molecular communication strategies of “local” and “systemic” innate immune responses in mosquito Anopheles stephensi. Front. Immunol. 9, 148. doi:10.3389/fimmu.2018.00148
de Oliveira M. A., de Souza W. (2001). An electron microscopic study of penetration by Trypanosoma rangeli into midgut cells of Rhodnius prolixus. J. Invertebr. Pathol. 77, 22–26. doi:10.1006/jipa.2000.4988
de Sousa M. A., da Silva-Fonseca T., dos Santos B. N., dos Santos-Pereira S. M., Carvalhal C., Hasslocher-Moreno A. M., et al. (2008). Trypanosoma rangeli tejera, 1920, in chronic Chagas’ disease patients under ambulatory care at the evandro Chagas clinical research institute (IPEC—Fiocruz, Brazil). Parasitol. Res. 103, 697–703. doi:10.1007/s00436-008-1033-1
Dillon R. J., Dillon V. M. (2004). The gut bacteria of insects: nonpathogenic interactions. Annu. Rev. Entomol. 49, 71–92. doi:10.1146/annurev.ento.49.061802.123416
Dimarcq J. L., Zachary D., Hoffmann J. A., Hoffmann D., Reichhart J. M. (1990). Insect immunity: expression of the two major inducible antibacterial peptides, defensin and diptericin, in Phormia terranovae. EMBO J. 9, 2507–2515. doi:10.1002/j.1460-2075.1990.tb07430.x
Duarte-da-Silva B., Guarneri A. A. (2023). Trypanosoma rangeli infection impairs reproductive success of Rhodnius prolixus. Parasitology 150, 1–7. doi:10.1017/S0031182022001470
Eger-Mangrich I., De Oliveira M. A., Grisard E. C., De Souza W., Steindel M. (2001). Interaction of Trypanosoma rangeli Tejera, 1920 with different cell lines in vitro. Parasitol. Res. 87, 505–509. doi:10.1007/s004360000356
Eichler S., Schaub G. A. (2002). Development of symbionts in triatomine bugs and the effects of infections with trypanosomatids. Exp. Parasitol. 100, 17–27. doi:10.1006/expr.2001.4653
Ellis D. S., Evans D. A., Stamford S. (1980). The penetration of the salivary glands of Rhodnius prolixus by Trypanosoma rangeli. Z. Parasitenkd. 62, 63–74. doi:10.1007/BF00925367
Ferrandon D., Imler J. L., Hetru C., Hoffmann J. A. (2007). The Drosophila systemic immune response: sensing and signalling during bacterial and fungal infections. Nat. Rev. Immunol. 7, 862–874. doi:10.1038/nri2194
Ferreira L. L., Lorenzo M. G., Elliot S. L., Guarneri A. A. (2010). A standardizable protocol for infection of Rhodnius prolixus with Trypanosoma rangeli, which mimics natural infections and reveals physiological effects of infection upon the insect. J. Invertebr. Pathol. 105, 91–97. doi:10.1016/j.jip.2010.05.013
Ferreira L. L., Pereira M. H., Guarneri A. A. (2015). Revisiting Trypanosoma rangeli transmission involving susceptible and non-susceptible hosts. PLoS One 10, e0140575. doi:10.1371/journal.pone.0140575
Ferreira R. C., Teixeira C. F., de Sousa V. F. A., Guarneri A. A. (2018). Effect of temperature and vector nutrition on the development and multiplication of Trypanosoma rangeli in Rhodnius prolixus. Parasitol. Res. 117, 1737–1744. doi:10.1007/s00436-018-5854-2
Figueiredo M. B., Genta F. A., Garcia E. S., Azambuja P. (2008). Lipid mediators and vector infection: Trypanosoma rangeli inhibits Rhodnius prolixus hemocyte phagocytosis by modulation of phospholipase A2 and PAF-acetylhydrolase activities. J. Insect Physiol. 54, 1528–1537. doi:10.1016/j.jinsphys.2008.08.013
Gabrieli P., Caccia S., Varotto-Boccazzi I., Arnoldi I., Barbieri G., Comandatore F., et al. (2021). Mosquito trilogy: microbiota, immunity and pathogens, and their implications for the control of disease transmission. Front. Microbiol. 12, 630438. doi:10.3389/fmicb.2021.630438
Garcia E. S., Azambuja P. (1991). Development and interactions of Trypanosoma cruzi within the insect vector. Parasitol. Today. 7, 240–244. doi:10.1016/0169-4758(91)90237-I
Garcia E. S., Castro D. P., Figueiredo M. B., Azambuja P. (2012). Parasite-mediated interactions within the insect vector: Trypanosoma rangeli strategies. Parasit. Vectors 5, 105. doi:10.1186/1756-3305-5-105
Garcia E. S., Castro D. P., Figueiredo M. B., Genta F. A., Azambuja P. (2009). Trypanosoma rangeli: a new perspective for studying the modulation of immune reactions of Rhodnius prolixus. Parasit. Vectors 2, 33. doi:10.1186/1756-3305-2-33
Garcia E. S., Machado E. M. M., Azambuja P. (2004). Inhibition of hemocyte microaggregation reactions in Rhodnius prolixus larvae orally infected with Trypanosoma rangeli. Exp. Parasitol. 107, 31–38. doi:10.1016/j.exppara.2004.03.015
Genta F. A., Souza R. S., Garcia E. S., Azambuja P. (2010). Phenoloxidases from Rhodnius prolixus: temporal and tissue expression pattern and regulation by ecdysone. J. Insect Physiol. 56, 1253–1259. doi:10.1016/j.jinsphys.2010.03.027
Gillespie J. P., Kanost M. R., Trenczek T. (1997). Biological mediators of insect immunity. Annu. Rev. Entomol. 42, 611–643. doi:10.1146/annurev.ento.42.1.611
Gomes S. A. O., Feder D., Garcia E. S., Azambuja P. (2003). Suppression of the prophenoloxidase system in Rhodnius prolixus orally infected with Trypanosoma rangeli. J. Insect Physiol. 49, 829–837. doi:10.1016/S0022-1910(03)00133-1
Gomes S. A. O., Feder D., Thomas N. E. S., Garcia E. S., Azambuja P. (1999). Rhodnius prolixus infected with Trypanosoma rangeli: in vivo and in vitro experiments. J. Invertebr. Pathol. 73, 289–293. doi:10.1006/jipa.1998.4836
González-Santoyo I., Córdoba-Aguilar A. (2012). Phenoloxidase: a key component of the insect immune system. Entomol. Exp. Appl. 142, 1–16. doi:10.1111/j.1570-7458.2011.01187.x
Gottar M., Gobert V., Matskevich A. A., Reichhart J. M., Wang C., Butt T. M., et al. (2006). Dual detection of fungal infections in Drosophila via recognition of glucans and sensing of virulence factors. Cell 127, 1425–1437. doi:10.1016/j.cell.2006.10.046
Gregório E. A., Ratcliffe N. A. (1991). The prophenoloxidase system and in vitro interaction of Trypanosoma rangeli with Rhodnius prolixus and Triatoma infestans haemolymph. Parasit. Immunol. 13, 551–564. doi:10.1111/j.1365-3024.1991.tb00551.x
Guarneri A. A., Lorenzo M. G. (2017). Triatomine physiology in the context of trypanosome infection. J. Insect Physiol. 97, 66–76. doi:10.1016/j.jinsphys.2016.07.005
Guhl F., Aguilera G., Pinto N., Vergara D. (2007). Updated geographical distribution and ecoepidemiology of the triatomine fauna (Reduviidae: Triatominae) in Colombia. Biomedica 1, 143–162.
Guhl F., Vallejo G. A. (2003). Trypanosoma (Herpetosoma) rangeli Tejera, 1920: an updated review. Mem. Inst. Oswaldo Cruz. 98, 435–442. doi:10.1590/S0074-02762003000400001
Hecker H., Schwarzenbach M., Rudin W. (1990). Development and interactions of Trypanosoma rangeli in and with the reduviid bug Rhodnius prolixus. Parasitol. Res. 76, 311–318. doi:10.1007/BF00928185
Herrera L., Morocoima A., Lozano-Arias D., García-Alzate R., Viettri M., Lares M., et al. (2022). Infections and coinfections by trypanosomatid parasites in a rural community of Venezuela. Acta Parasitol. 67, 1015–1023. doi:10.1007/s11686-021-00505-1
Janeway C. A., Medzhitov R. (2002). Innate immune recognition. Annu. Rev. Immunol. 20, 197–216. doi:10.1146/annurev.immunol.20.083001.084359
Kim Y., Ahmed S., Stanley D., An C. (2018). Eicosanoid-mediated immunity in insects. Dev. Comp. Immunol. 83, 130–143. doi:10.1016/j.dci.2017.12.005
Lambert J., Keppi E., Dimarcq J. L., Wicker C., Reichhart J. M., Dunbar B., et al. (1989). Insect immunity: isolation from immune blood of the dipteran Phormia terranovae of two insect antibacterial peptides with sequence homology to rabbit lung macrophage bactericidal peptides. Proc. Natl. Acad. Sci. U. S. A. 86, 262–266. doi:10.1073/pnas.86.1.262
Livak K. J., Schmittgen T. D. (2001). Analysis of relative gene expression data using realtime quantitative PCR and the 2(-Delta Delta C(T)) Method. Methods 25, 402–408. doi:10.1006/meth.2001.1262
Lopez L., Morales G., Ursic R., Wolff M., Lowenberger C. (2003). Isolation and characterization of a novel insect defensin from Rhodnius prolixus, a vector of Chagas disease. Insect Biochem. Mol. Biol. 33, 439–447. doi:10.1016/S0965-1748(03)00008-0
Machado E. M. M., Azambuja P., Garcia E. S. (2006). WEB 2086, a platelet-activating factor antagonist, inhibits prophenoloxidase-activating system and hemocyte microaggregation reactions induced by Trypanosoma rangeli infection in Rhodnius prolixus hemolymph. J. Insect Physiol. 52, 685–692. doi:10.1016/j.jinsphys.2006.03.008
Mattos D. P. (2014). Interação de Rhodnius prolixus com Trypanosoma rangeli, avaliação do sistema de defesa celular e humoral e microbiota intestinal. Master's thesis. Rio de Janeiro (RJ): Inst. Oswaldo Cruz.
Mello C. B., Garcia E. S., Ratcliffe N. A., Azambuja P. (1995). Trypanosoma cruzi and Trypanosoma rangeli: interplay with hemolymph components of Rhodnius prolixus. J. Invertebr. Pathol. 65, 261–268. doi:10.1006/jipa.1995.1040
Mello C. B., Nigam Y., Garcia E. S., Azambuja P., Newton R. P., Ratcliffe N. A. (1999). Studies on a haemolymph lectin isolated from Rhodnius prolixus and its interaction with Trypanosoma rangeli. Exp. Parasitol. 91, 289–296. doi:10.1006/expr.1998.4385
Mesquita R. D., Vionette-Amaral R. J., Lowenberger C., Rivera-Pomar R., Monteiro F. A., Minx P., et al. (2015). Genome of Rhodnius prolixus, an insect vector of Chagas disease, reveals unique adaptations to hematophagy and parasite infection. Proc. Natl. Acad. Sci. U. S. A. 112, 14936–14941. doi:10.1073/pnas.1506226112
Nicolas E., Reichhart J. M., Hoffmann J. A., Lemaitre B. (1998). In vivo regulation of the IκB homologue cactus during the immune response of Drosophila. J. Biol. Chem. 273, 10463–10469. doi:10.1074/jbc.273.17.10463
Nishide Y., Kageyama D., Yokoi K., Jouraku A., Tanaka H., Futahashi R., et al. (2019). Functional crosstalk across IMD and Toll pathways: insight into the evolution of incomplete immune cascades. Proc. Biol. Sci. 286, 20182207. doi:10.1098/rspb.2018.2207
Paim R. M., Pereira M. H., Di Ponzio R., Rodrigues J. O., Guarneri A. A., Gontijo N. F., et al. (2012). Validation of reference genes for expression analysis in the salivary gland and the intestine of Rhodnius prolixus (Hemiptera, Reduviidae) under different experimental conditions by quantitative real-time PCR. BMC Res. Notes. 5, 128. doi:10.1186/1756-0500-5-128
Paim R. M. M., Pereira M. H., Araújo R. N., Gontijo N. F., Guarneri A. A. (2013). The interaction between Trypanosoma rangeli and the nitrophorins in the salivary glands of the triatomine Rhodnius prolixus (Hemiptera; Reduviidae). Insect Biochem. Mol. Biol. 43, 229–236. doi:10.1016/j.ibmb.2012.12.011
Peterson J. K., Graham A. L. (2016). What is the ‘true’ effect of Trypanosoma rangeli on its triatomine bug vector? J. Vector Ecol. 41, 27–33. doi:10.1111/jvec.12190
Ramirez L. E., Lages-Silva E., Alvarenga-Franco F., Matos A., Vargas N., Fernandes O., et al. (2002). High prevalence of Trypanosoma rangeli and Trypanosoma cruzi in opossums and triatomids in a formerly-endemic area of Chagas disease in Southeast Brazil. Acta Trop. 84, 189–198. doi:10.1016/S0001-706X(02)00185-7
Ratcliffe N. A., Gagen S. J. (1977). Studies on the in vivo cellular reactions of insects: an ultrastructural analysis of nodule formation in Galleria mellonella. Tissue Cell 9, 73–85. doi:10.1016/0040-8166(77)90050-7
Ribeiro J. M. C., Genta F. A., Sorgine M. H. F., Logullo R., Mesquita R. D., Paiva-Silva G. O., et al. (2014). An insight into the transcriptome of the digestive tract of the blood sucking bug, Rhodnius prolixus. PLoS Negl. Trop. Dis. 8, e2594. doi:10.1371/journal.pntd.0002594
Rolandelli A., Nascimento A. E. C., Silva L. S., Rivera-Pomar R., Guarneri A. A. (2021). Modulation of IMD, Toll, and Jak/STAT immune pathways genes in the fat body of Rhodnius prolixus during Trypanosoma rangeli infection. Front. Cell. Infect. Microbiol. 10, 598526. doi:10.3389/fcimb.2020.598526
Salcedo-Porras N., Guarneri A., Oliveira P. L., Lowenberger C. (2019). Rhodnius prolixus: identification of missing components of the IMD immune signaling pathway and functional characterization of its role in eliminating bacteria. PLoS One 14, e0214794. doi:10.1371/journal.pone.0214794
Salcedo-Porras N., Lowenberger C. (2019). The innate immune system of kissing bugs, vectors of Chagas disease. Dev. Comp. Immunol. 98, 119–128. doi:10.1016/j.dci.2019.04.007
Satyavathi V. V., Minz A., Nagaraju J. (2014). Nodulation: an unexplored cellular defense mechanism in insects. Cell Signal 26, 1753–1763. doi:10.1016/j.cellsig.2014.02.024
Söderhäll K., Cerenius L. (1998). Role of the prophenoloxidase-activating system in invertebrate immunity. Curr. Opin. Immunol. 10, 23–28. doi:10.1016/S0952-7915(98)80026-5
Stöven S., Ando I., Kadalayil L., Engström Y., Hultmark D. (2000). Activation of the Drosophila NF-κB factor relish by rapid endoproteolytic cleavage. EMBO Rep. 1, 347–352. doi:10.1093/embo-reports/kvd072
Urrea D. A., Carranza J. C., Cuba C. A. C., Gurgel-Gonçalves R., Guhl F., Schofield C. J., et al. (2005). Molecular characterisation of Trypanosoma rangeli strains isolated from Rhodnius ecuadoriensis in Peru, R. colombiensis in Colombia and R. pallescens in Panama, supports a co-evolutionary association between parasites and vectors. Infect. Genet. Evol. 5, 123–129. doi:10.1016/j.meegid.2004.07.005
Ursic-Bedoya R., Buchhop J., Joy J. B., Durvasula R., Lowenberger C. (2011). Prolixicin: a novel antimicrobial peptide isolated from Rhodnius prolixus with differential activity against bacteria and Trypanosoma cruzi. Insect Mol. Biol. 20, 775–786. doi:10.1111/j.1365-2583.2011.01107.x
Vallejo G. A., Guhl F., Schaub G. A. (2009). Triatominae-Trypanosoma cruzi/T. rangeli: vector-parasite interactions. Acta Trop. 110, 137–147. doi:10.1016/j.actatropica.2008.10.001
Vergara-Meza J. G., Brilhante A. F., Valente V. C., Villalba-Alemán E., Ortiz P. A., Cosmiro-de-Oliveira S., et al. (2022). Trypanosoma cruzi and Trypanosoma rangeli in Acre, Brazilian Amazonia: coinfection and notable genetic diversity in an outbreak of orally acquired acute Chagas disease in a forest community, wild reservoirs, and vectors. Parasitology 2, 350–365. doi:10.3390/parasitologia2040029
Vieira C. S., Figueiredo M. B., Moraes C. S., Pereira S. B., Dyson P., Mello C. B., et al. (2021). Azadirachtin interferes with basal immunity and microbial homeostasis in the Rhodnius prolixus midgut. Dev. Comp. Immunol. 114, 103864. doi:10.1016/j.dci.2020.103864
Vieira C. S., Mattos D. P., Waniek P. J., Santangelo J. M., Figueiredo M. B., Gumiel M., et al. (2015). Rhodnius prolixus interaction with Trypanosoma rangeli: modulation of the immune system and microbiota population. Parasit. Vectors 8, 135. doi:10.1186/s13071-015-0736-2
Vieira C. S., Moreira O. C., Batista K. K. S., Ratcliffe N. A., Castro D. P., Azambuja P. (2018). The NF-κB inhibitor, IMD-0354, affects immune gene expression, bacterial microbiota and Trypanosoma cruzi infection in Rhodnius prolixus midgut. Front. Physiol. 9, 1189. doi:10.3389/fphys.2018.01189
Vieira C. S., Waniek P. J., Castro D. P., Mattos D. P., Moreira O. C., Azambuja P. (2016). Impact of Trypanosoma cruzi on antimicrobial peptide gene expression and activity in the fat body and midgut of Rhodnius prolixus. Parasit. Vectors 9, 119. doi:10.1186/s13071-016-1398-4
Vieira C. S., Waniek P. J., Mattos D. P., Castro D. P., Mello C. B., Ratcliffe N. A., et al. (2014). Humoral responses in Rhodnius prolixus: bacterial feeding induces differential patterns of antibacterial activity and enhances mRNA levels of antimicrobial peptides in the midgut. Parasit. Vectors 7, 232. doi:10.1186/1756-3305-7-232
Watkins R. (1971). Histology of Rhodnius prolixus infected with Trypanosoma rangeli. J. Invertebr. Pathol. 17, 59–66. doi:10.1016/0022-2011(71)90126-1
Whitten M. M. A., Mello C. B., Gomes S. A. O., Nigam Y., Azambuja P., Garcia E. S., et al. (2001). Role of superoxide and reactive nitrogen intermediates in Rhodnius prolixus (Reduviidae)/Trypanosoma rangeli interactions. Exp. Parasitol. 98, 44–57. doi:10.1006/expr.2001.4615
Zasloff M. (2002). Antimicrobial peptides of multicellular organisms. Nature 415, 389–395. doi:10.1038/415389a
Zumaya-Estrada F. A., Martínez-Barnetche J., Lavore A., Rivera-Pomar R., Rodríguez M. H. (2018). Comparative genomics analysis of triatomines reveals common first line and inducible immunity-related genes and the absence of IMD canonical components among hemimetabolous arthropods. Parasit. Vectors 11, 48. doi:10.1186/s13071-017-2561-2
Keywords: Trypanosoma rangeli, Rhodnius prolixus, microbiota, immunity, signaling pathways
Citation: Pereira SB, Mattos DPd, Gonzalez MS, Mello CB, Azambuja P, Castro DPd and Vieira CS (2024) Immune signaling pathways in Rhodnius prolixus in the context of Trypanosoma rangeli infection: cellular and humoral immune responses and microbiota modulation. Front. Physiol. 15:1435447. doi: 10.3389/fphys.2024.1435447
Received: 20 May 2024; Accepted: 16 July 2024;
Published: 15 August 2024.
Edited by:
Amr A. Mohamed, Cairo University, EgyptReviewed by:
Ioannis Eleftherianos, George Washington University, United StatesGuenter Artur Schaub, Ruhr University Bochum, Germany
Copyright © 2024 Pereira, Mattos, Gonzalez, Mello, Azambuja, Castro and Vieira. This is an open-access article distributed under the terms of the Creative Commons Attribution License (CC BY). The use, distribution or reproduction in other forums is permitted, provided the original author(s) and the copyright owner(s) are credited and that the original publication in this journal is cited, in accordance with accepted academic practice. No use, distribution or reproduction is permitted which does not comply with these terms.
*Correspondence: Cecília Stahl Vieira, Y2VjaWxpYXN0YWhsQGdtYWlsLmNvbQ==