- 1College of Physical Education and Health Sciences, Zhejiang Normal University, Jinhua, China
- 2Scientific Research Center and Laboratory of Aquatic Sports Science of General Administration of Sports China, Zhejiang College of Sports, Hangzhou, China
- 3Shanghai University of Finance and Economics Zhejiang College, Jinhua, China
Objective: This study aimed to determine if patients with chronic ankle instability (CAI) exhibit biomechanical changes associated with the increased risk of anterior cruciate ligament (ACL) injury during landing tasks.
Study Design: This study was conducted through systematic review and meta-analysis.
Data Sources: Searches were conducted in May 2024 across five electronic databases, including Web of Science, Scopus, PubMed, SPORTDiscus, and Cochrane Library.
Eligibility Criteria: Studies were included if they (1) involved subjects with CAI and healthy controls and (2) assessed biomechanical variables such as ground reaction forces, joint angles, and joint torques.
Results: Of the 675 identified studies, 171 were included in the review, and 13 were eligible for meta-analysis. The reviewed studies clearly defined research objectives, study populations, consistent participant recruitment, and exposures, and they used valid and reliable measures for outcomes. However, areas such as sample size calculation, study sample justification, blinding in assessments, and addressing confounders were not robust. This meta-analysis involved 542 participants (healthy group: n = 251; CAI group: n = 291). Compared with healthy individuals, patients with CAI exhibited a greater peak vertical ground reaction force (peak VGRF; SMD = 0.30, 95% CI: 0.07–0.53, p = 0.009), reduced hip flexion angles (SMD = −0.30, 95% CI: −0.51 to −0.17, p < 0.0001), increased trunk lateral flexion (SMD = 0.47, 95% CI: 0.05 to 0.9, p = 0.03), greater hip extension moments (SMD = 0.47, 95% CI: 0.09–0.84, p = 0.02), and increased knee extension moments (SMD = 0.39, 95% CI: 0.02–0.77, p = 0.04).
Conclusion: During landing tasks, patients with CAI demonstrate increased hip extension moments and knee extension moments, decreased hip flexion angles, increased peak VGRF, and increased trunk lateral flexion angles. These biomechanical variables are associated with an elevated risk of ACL injuries.
Systematic Review Registration: Identifier CRD42024529349.
1 Introduction
Anterior cruciate ligament (ACL) injuries are among the most severe injuries in sports, which remarkably affect athletic performance (Davey et al., 2019). The incidence and cost of recovery from ACL injuries are high, with approximately 100,000 to 2,50,000 cases occurring annually in the United States alone (Hewett et al., 1999; Mancino et al., 2023). The estimated cost per injury is about $17,000, with an annual cost of approximately $646 million for surgeries and rehabilitation among females with ACL injuries (Hewett et al., 1999). Moreover, pain, functional limitations, and radiographic signs of osteoarthritis in the knee are evident 12–20 years post-injury (Lohmander et al., 2004; Myklebust and Bahr, 2005). The current paradigms for ACL injury mechanisms during landing include four main theories: Ligament dominance, Quadriceps dominance, Trunk dominance, and Leg dominance (Hewett et al., 2010). Based on these theories, previous scholars have identified eight biomechanical variables associated with ACL injury risk during landing (dynamics and kinematics in the horizontal, frontal, and sagittal planes, impact loads, and trunk movements) (Chou et al., 2023; Hewett et al., 2010). For instance, small knee and hip flexion angles in sagittal plane kinematics have been previously reported to correlate with a great risk of ACL injuries (Devita and Skelly, 1992; Lin et al., 2012; Padua et al., 2009; Trigsted et al., 2017) (Table 1). Identifying the biomechanical changes and influencing factors of individual ACL injury patterns is crucial for assessing functional capabilities and predicting subsequent injury risks post-return to sports.
Existing research suggests that chronic ankle instability (CAI) may be important predisposing factor for ACL injuries. Epidemiological surveys indicate that 52%–60% of patients with ACL injuries have a history of ankle sprains (Kramer et al., 2007; Söderman et al., 2001), and most ankle sprains develop into CAI (Theisen and Day, 2019). Studies by Theisen and Day also suggest that the altered lower limb biomechanics associated with CAI may increase the susceptibility to non-contact ACL injuries (Theisen and Day, 2019). The etiology of CAI is often attributed to injuries of the lateral ankle ligaments, primarily the anterior talofibular ligament, resulting from sprains, with or without damage to the calcaneofibular ligament (van Putte-Katier et al., 2015). In addition, CAI is a common sequela of recurrent ankle sprains (Gribble et al., 2016). About 73% of individuals with ankle sprains develop CAI (Theisen and Day, 2019). Patients with CAI often experience persistent symptoms of pain or weakness in the ankle (Gribble et al., 2013), which affects their quality of life and participation in physical activities (Arnold et al., 2011; Hubbard-Turner and Turner, 2015), as well as increases the risk of recurrent sprains and post-traumatic ankle osteoarthritis (Gribble et al., 2016; Moisan et al., 2017).
In recent years, CAI has received attention as a risk factor for ACL injuries during landing motions (Kikumoto et al., 2024). Current literature proposes that the mechanism of ACL injuries in patients with CAI is due to distal responses in the kinematic chain triggered by the ankle during landing tasks, which result in alterations in the proximal movement chain (knee joint, hip joint, and trunk) (Hertel and Corbett, 2019; Sueki et al., 2013). Munn et al. have shown that several somatosensory domains are impaired in patients with CAI, possibly due to ligament and joint proprioceptor damage during injury and potential neural damage post-ligament injury (Munn et al., 2010). Impairments in proprioceptors can lead to altered feedforward motor control mediated by spinal or supraspinal mechanisms, affecting centrally mediated motor control strategies (Brown et al., 2004; Caulfield and Garrett, 2002; Hass et al., 2010). These outcomes may lead to changes in the proximal movement chain to compensate for ankle instability and functional impairment (Bullock-Saxton, 1994; Koshino et al., 2013). Such compensatory phenomena in proximal joints could cause ACL injuries (Jeon et al., 2021; Xu et al., 2022). For example, Terada et al. found reduced knee flexion at the peak of the anterior tibial shear force in patients with CAI (Terada et al., 2014), and Jeon et al.’s meta-analysis identified greater vertical ground reaction forces (VGRF) upon landing in patients with CAI (Jeon et al., 2021), both results were associated with ACL injury risk. However, the aforementioned studies identified only a single biomechanical variable difference associated with ACL injuries, probably due to the limited outcome indicators set in previous research, which failed to report more biomechanical differences related to ACL injuries. Consequently, many studies based on similar measurements have produced inconsistent results, and the adoption of a single variable alone is insufficient to objectively describe the ACL injury mechanisms in CAI patients. The lack of combined analyses of variables related to ACL injuries, which limits the effective examination of how proximal adaptations caused by CAI lead to ACL injuries.
A 13-year epidemiological study revealed that landing maneuvers are a common cause of ACL injuries in sports with frequent jumping activities, such as basketball and volleyball (Agel et al., 2005). Krosshaug et al. (2006) analyzed videos of 39 ACL injuries during basketball games and found that most injuries occurred during non-contact landing maneuvers. The landing action, given its high impact and frequent occurrence, warrants particular attention (Kim et al., 2017; Konradsen and Voigt, 2002). Moreover, landing provides a controlled and easily standardizable experimental condition, facilitating accurate assessment and analysis of biomechanical parameters. Considering the commonality, representativeness, high impact, and robustness of landing measurements in ACL injury studies, this research focuses on the landing maneuvers of patients with CAI.
Therefore, a systematic review and meta-analysis were conducted to investigate whether biomechanical changes related to ACL injury risk are present in patients with CAI during landing tasks. Previous studies have found that patients with CAI often fail to effectively utilize the lower limb buffering mechanisms during landing owing to ankle instability (Jeon et al., 2021). Additionally, changes have been observed in the proximal movement chain of the lower limbs in CAI patients (Bullock-Saxton, 1994; Koshino et al., 2013). We hypothesized that compared with healthy individuals, those with CAI will exhibit a greater peak VGRF and an upright body posture (i.e., reduced knee and hip flexion), leading to an increased risk of ACL injury.
2 Methods
This review was conducted in accordance with the PRISMA guidelines for systematic reviews and meta-analyses. It was prospectively registered in the international database for systematic reviews. (PROSPERO registration number: CRD42024529349).
2.1 Identification of ACL injury-related variables
Four theories based on the mechanism of landing ACL injury (Ligament dominance; Quadriceps dominance; Trunk dominance; leg dominance) (Hewett et al., 2010), and previous prospective and case-control studies identified eight biomechanical variables associated with ACL injury risk (Chou et al., 2023; Hewett et al., 2010). These variables have been adopted in several high-quality studies (Chou et al., 2023), thereby proving their reliability. Table 1 illustrates the detrimental directionality of biomechanical variables in each construct (high injury risk). Following data extraction, clarifying which outcome indicators are used for correlation analysis is crucial, serving as the bridge to determine whether CAI is associated with ACL injuries.
2.2 Data sources and search strategy
In May 2024, a systematic search was conducted across five electronic databases: Web of Science, Scopus, PubMed, SPORTDiscus, and Cochrane Library. Keywords related to CAI and biomechanical variables were used to identify relevant articles. Keywords for each category were combined using the Boolean operator “OR” and then combined across categories using “AND” for each database search. There was no restriction on publication date. Boolean logic was utilized for all database searches: (chronic ankle instability OR ankle instability OR functional ankle instability OR mechanical ankle instability OR CAI OR FAI OR MAI) AND (lower limb OR lower extremity OR hip OR knee OR ankle OR trunk) AND (kinematic OR kinetics OR biomechanics OR Moment OR Torque OR dynamic) AND [1) stop jump: (stop jump OR stop-jump OR stop jumping OR stop-jumping), 2) landing: (land OR landing OR jump land OR jump landing OR jump-land OR jump-landing OR drop-vertical jump OR single-leg landing OR single-leg land OR jump OR jumping)]. The detailed search strategy is presented in Table 2.
2.3 Inclusion criteria
The search results were independently screened by two reviewers (Z.H., B.Y.) on the basis of the predetermined inclusion and exclusion criteria, with disputes resolved by the corresponding author (H.Z.) if necessary. Studies were included if they met the following criteria: In accordance with the PICOS guidelines, studies meeting the following criteria were included: P: The study population consists of individuals with CAI and healthy control subjects. I: Participants perform landing tasks as an intervention measure. C: CAI patients are compared with a healthy control group. O: The reported outcomes are biomechanical variables, such as ground reaction forces, joint angles, and joint torques. S: Cross-sectional studies are included. Exclusion criteria included the following: 1) variables unrelated to ACL injury outcome indicators (Table 1); 2) task location is not on a flat surface (differences in biomechanics of landing on flat and sloped surfaces may affect the robustness of study results. Additionally, sloped landings are uncommon in real-life activities.); 3) studies employing one-dimensional statistical parametric mapping analysis (Data cannot be pooled for meta-analysis); 4) review articles, editorials, speeches, commentaries, abstracts, case studies, surgical procedures, and non-peer-reviewed articles.
2.4 Study selection
Titles and abstracts were screened by two independent reviewers, with any discrepancies resolved through consultation with a third author. If eligibility could not be determined from the title and abstract, then full texts were obtained and reviewed. Cohen’s kappa coefficient (κ) and percentage agreement were used to assess inter-reviewer consistency. κ was interpreted on the basis of Landis and Koch’s standards: Values less than 0 indicated no agreement, 0–0.20 indicated slight agreement; 0.21–0.40 indicated fair agreement; 0.41–0.60 indicated moderate agreement; 0.61–0.80 indicated substantial agreement; and 0.81–1 indicated almost perfect agreement (Landis and Koch, 1977). Full texts of all eligible studies were retrieved, and data such as demographics (e.g., gender and age), sample size, and biomechanical variable results (e.g., standardized ground reaction forces, joint angles, and standardized joint torques) were extracted.
2.5 Quality assessment and analysis
The quality of included studies was assessed using the NIH quality assessment tool for observational cohort and cross-sectional studies. Two researchers (Z.H. and B.Y.) independently evaluated the quality of each study, with any disagreements resolved through discussion until consensus was reached.
2.6 Publication bias
Publication bias was assessed using funnel plots and Egger’s regression test. Visual inspection of funnel plot symmetry indicated an unbiased range of effect sizes among the included studies. Asymmetry in the funnel plot suggested bias toward specific effect sizes or sample sizes. Egger’s regression test quantified bias using the effect size of each included study, with a p-value less than 0.05 indicating significant publication bias (Borenstein et al., 2021).
2.7 Sensitivity analysis
Sensitivity analyses were conducted on studies included in the meta-analysis to determine if the exclusion of influential studies affected the overall effect results. Thus, the most influential studies were excluded from the meta-analysis and analysis was repeated.
2.8 Data extraction and statistical analysis
Data were extracted by two authors (Z.H., B.Y.) using standardized forms from each included study. The extracted data were as follows: 1) sample description, encompassing the sample size, age, and gender; 2) information related to CAI, including the definition of CAI and scores from the Cumberland Ankle Instability Tool; 3) biomechanical variables related to outcome indicators (Table 1); 4) standardized results, including means, SDs, p-values, and standardized difference in effect sizes; 5) task conditions, specifically limited to landing tasks performed on flat surfaces. The data extracted from all included studies were then synthesized for comprehensive analysis. Statistical analysis compared biomechanical variables between patients with CAI and healthy individuals as the effect sizes for meta-analysis. To avoid errors caused by multiple biomechanical variables within each structure influencing the final outcome, we conducted separate meta-analyses for biomechanical outcome indicators within each structure (e.g., separate meta-analyses for Trunk flexion and trunk lateral flexion within the trunk mechanism structure). Additionally, to prevent reliance on effect sizes from the same study, we averaged the effect sizes based on specific task conditions (e.g., averaging the parameters of a biomechanical variable at different stages during a landing task) (Borenstein et al., 2021).
The overall effect size for each variable was calculated using sample size, means, standard deviations, mean differences, and p-values. Meta-analyses were performed using Review Manager V.5.3 (Copenhagen, Denmark: The Nordic Cochrane Centre, The Cochrane Collaboration, 2014). Owing to variations in drop platform heights and the differences between single and double foot landings among the included studies, ensuring complete consistency across multicenter and multiprotocol studies is challenging. Therefore, this study calculated the effect size of each biomechanical variable using the standardized mean difference (SMD) with a 95% confidence interval (CI). A random effect model was employed. Heterogeneity among the included studies was assessed using the I2 statistic.
3 Results
3.1 Study selection and characteristics
An initial search across five databases yielded 1,615 articles. After duplicates were removed, 675 studies remained. Title and abstract screening by two reviewers eliminated 504 studies (consistency rate = 97.62%, κ = 0.49), and 171 studies were assessed for eligibility through full-text review. After this review, 157 studies were excluded, leaving 14 studies included in the meta-analysis (consistency rate = 100%, κ = 1). The detailed exclusion process at each stage is depicted in Figure 1. A total of 564 participants were involved in this study (healthy group: n = 262; CAI group: n = 302), with detailed characteristics of the included studies provided in Table 3. For variables related to ACL injury, seven studies were included on peak VGRF (Caulfield and Garrett, 2004; De Ridder et al., 2015; Jeon and Park, 2021; Lee et al., 2017; Watabe et al., 2022; Watanabe et al., 2021; Zhang et al., 2012), three studies on loading rate (De Ridder et al., 2015; Jeon and Park, 2021; Lee et al., 2017); nine and ten studies on hip flexion angle (Brown et al., 2011; Caulfield and Garrett, 2004; Gribble and Robinson, 2009; Han et al., 2023; Jeon and Park, 2021; Sagawa et al., 2024; Terada et al., 2015; Terada et al., 2014; Watabe et al., 2022; Watanabe et al., 2021) and knee flexion angle (Brown et al., 2011; Caulfield and Garrett, 2004; Gribble and Robinson, 2009; Han et al., 2023; Jeon and Park, 2021; Sagawa et al., 2024; Terada et al., 2015; Terada et al., 2014; Watabe et al., 2022; Watanabe et al., 2021), respectively. Ankle flexion angle is covered in 10 studies (Gribble and Robinson, 2009; Han et al., 2023; Jeon and Park, 2021; Kipp and Palmieri-Smith, 2012; Lee et al., 2017; Sagawa et al., 2024; Terada et al., 2015; Watabe et al., 2022; Watanabe et al., 2021; Zhang et al., 2012); and two studies each on hip extension moment (Jeon and Park, 2021; Watanabe et al., 2021) and knee extension moment (Jeon and Park, 2021; Watanabe et al., 2021). Four studies on hip abduction angle (Brown et al., 2011; Jeon and Park, 2021; Sagawa et al., 2024; Terada et al., 2015) and three studies on knee abduction angle (De Ridder et al., 2015; Jeon and Park, 2021; Lee et al., 2017); two studies on trunk flexion (Brown et al., 2011; Watabe et al., 2022) and two studies on trunk lateral flexion (Brown et al., 2011; Watabe et al., 2022). No other biomechanical variables related to ACL injuries were reviewed in this study (Table 4).
3.2 Quality assessment
The results of the quality assessment tool developed by NIH for observational cohorts and cross-sectional studies ranged from five to 9 (Table 5). The evaluation results highlight some apparent strengths and limitations of the included literature. The strengths lie in the studies having clear objectives, defined study populations, and consistent participant recruitment, and using valid and reliable methods for exposure definition and outcome measurement. These aspects are important indicators of high research quality, suggesting that the design and execution of the studies were successful in these respects. However, some shortcomings remain. Only two studies calculated the sample size and determined an appropriate study sample size, which could affect the statistical power of the results and the reliability of the conclusions. Furthermore, the lack of assessment of blinding implementation and insufficient justification for confounding variables could lead to bias and errors in the study outcomes.
3.3 Publication bias
Publication bias was detected in the trunk flexion angle (p = 0.007). No publication bias was found for the remaining variables (Supplementary Appendix S1).
3.4 Heterogeneity
Heterogeneity was present in the ankle dorsiflexion angle (Figure 2C), loading rate (Figure 3A), knee flexion angle (Figure 2B), and trunk flexion (Figure 4B).
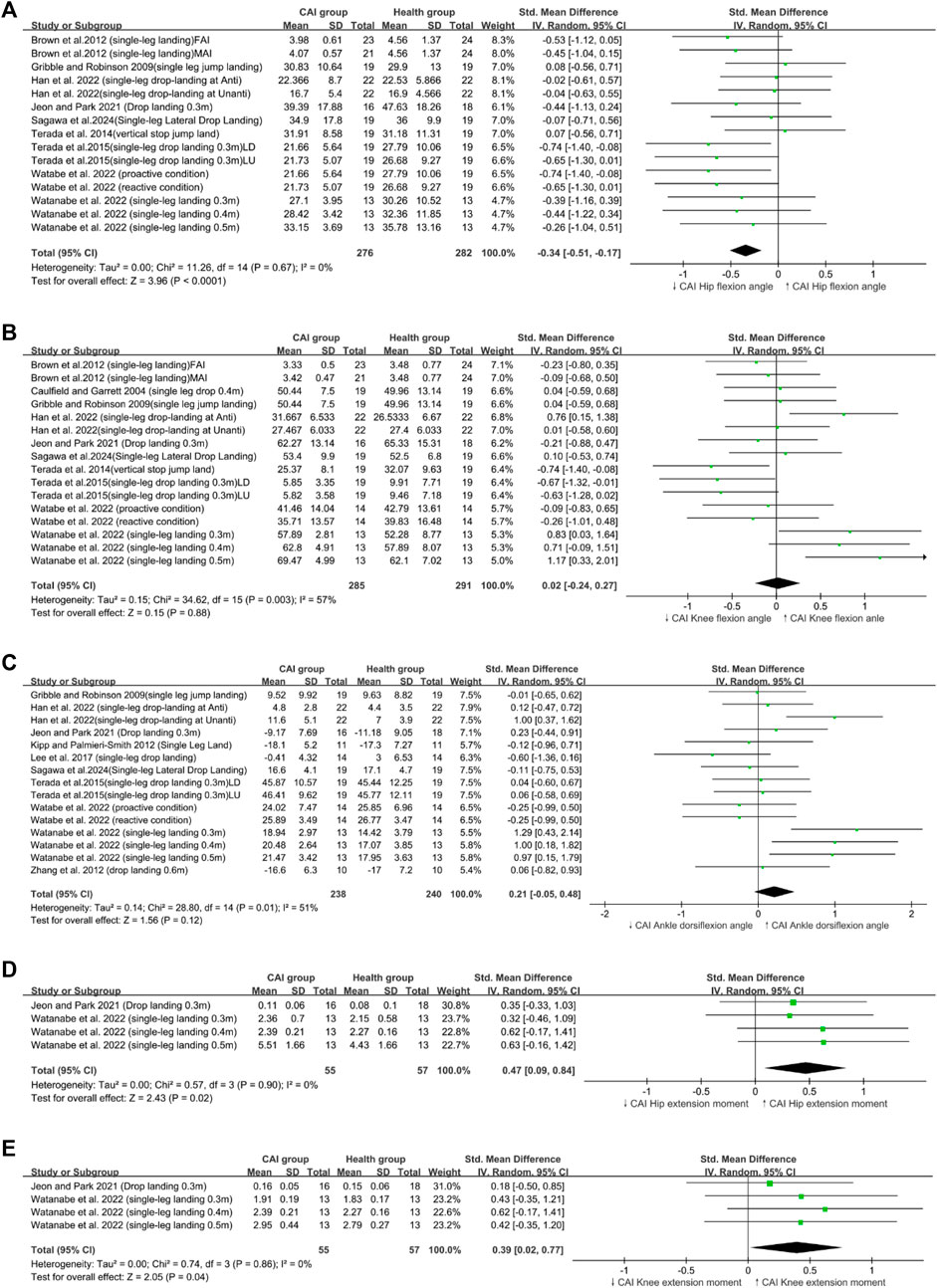
Figure 2. Sagittal Plane biomechanical parameters; (A) Hip flexion angle, (B) Knee flexion angle, (C) Ankle dorsiflexion angle (D) Hip extension moment, (E) Knee extension moment.
3.5 Study findings
Our research findings indicated that patients with CAI exhibited a greater maximum (peak VGRF; SMD = 0.30, 95% CI: 0.07–0.53, p = 0.009), a reduced hip flexion angle (SMD = −0.34,95% CI: −0.53 to −0.19, p < 0.0001), (Figure 4A) and an increased trunk lateral flexion angle (SMD = 0.47,95% CI: 0.05–0.9, p = 0.03) during landing tasks. In Addition, these patients showed increased hip extension moments (SMD = 0.47,95% CI: 0.09–0.84, p = 0.02) and greater knee extension moments (SMD = 0.39,95% CI: 0.02–0.77, p = 0.04) (Figures 2C, D) compared with healthy controls. By contrast, no significant differences in the loading rate, knee flexion angle, trunk flexion angle, knee abduction angle (Figure 3B), ankle dorsiflexion angle (Figure 2E) and hip abduction angle were found between patients with CAI and healthy individuals. The sensitivity analysis results reveal that the outcomes for Knee extension moment and Loading rate are significantly influenced by individual studies (Supplementary Appendix S4). Specifically, removing the study by Jeon and Park (2021) led to a significant change in the p-value for Knee extension moment (p < 0.05), and removing Lee et al. (2017) similarly affected the p-value for Loading rate (p < 0.05). Due to the limited number of studies included, further subgroup analysis to explore these differences was not feasible. This limitation highlights the need for cautious interpretation of our conclusions. Additionally, the results for other variables remained consistent and unchanged in the sensitivity analysis.
4 Discussion
This systematic review aimed to determine whether changes in lower limb biomechanics during landing tasks in the CAI population increase the risk of ACL injury compared with that in a healthy population. This meta-analysis included data from 14 studies (Brown et al., 2011; Caulfield and Garrett, 2004; De Ridder et al., 2015; Gribble and Robinson, 2009; Han et al., 2023; Jeon and Park, 2021; Kipp and Palmieri-Smith, 2012; Lee et al., 2017; Sagawa et al., 2024; Terada et al., 2015; Terada et al., 2014; Watabe et al., 2022; Watanabe et al., 2021; Zhang et al., 2012), which compared the landing biomechanics between patients with CAI and healthy individuals. Our findings indicated that patients with CAI exhibited a greater peak VGRF during landing compared with healthy individuals, which was consistent with our initial hypothesis. A decreased hip flexion angle was also observed, partially supporting our hypothesis that the body was upright, although no significant difference was found in knee flexion angles. Additionally, an increased trunk lateral flexion, an increased hip extension moment, and an increased knee extension moment were observed in patients with CAI.
4.1 Sagittal plane kinematics and kinetics of the lower limb
Our study revealed that individuals with CAI landed with decreased hip flexion angles and increased knee and hip extension moments. However, contrary to our expectations, patients with CAI did not exhibit reduced knee flexion angles or dorsiflexion angles. This finding is also in contrast to the findings of Chan et al. (2022) and Aaron and James (Theisen and Day, 2019), who reported reduced knee flexion and ankle dorsiflexion in similar contexts (Chan et al., 2022). This inconsistency may stem from our meta-analysis incorporating anticipated and unanticipated landing conditions (Han et al., 2023; Watabe et al., 2022) and changes in visual focus during landing (Terada et al., 2015). Analyzing multiple states together led to high heterogeneity, but this result was considered acceptable because it reflected the different dynamic states encountered during athletic activities. Moreover, our findings suggested that patients with CAI developed neuromuscular feedforward mechanisms that resulted in upright postures and increased hip extension moments during landing, thereby supporting our study outcomes (Figure 2) (Beckman and Buchanan, 1995; Kim et al., 2017).
High knee extension moments during landing are associated with increased risk of ACL injuries (Alentorn-Geli et al., 2009). This injury mechanism is prevalent among female athletes (Karita et al., 2017). The primary reason is that women typically have wider hips, which leads to a larger Q-angle (the angle between the lateral femoral axis and the tibial axis) compared with men (Mizuno et al., 2001). An increased Q-angle enhances the lateral pulling force of the quadriceps, resulting in greater knee extension torque (Heiderscheit et al., 2000). Additionally, women’s weaker muscle strength and neuromuscular control may contribute to increased knee extension torque during landing (Hewett et al., 2005). Cadaver studies have shown that intense quadriceps contractions can cause ACL ruptures (DeMorat et al., 2004). Furthermore, during high-speed eccentric contractions and quadriceps activation during landing, the ACL is subjected to greater forces compared with those observed during maximum isometric contractions of the quadriceps (Griffin et al., 2000). Previous research also supported our findings, indicating that patients with CAI exhibited a higher quadriceps/hamstring co-activation ratio during inclined plane landings compared with healthy individuals (Li et al., 2017). During landing, the hamstring’s force is diminished, and the quadriceps play a dominant role. Previous studies have shown that greater hamstring activation can reduce the ACL load exerted by the quadriceps and provide dynamic knee stability by resisting anterior tibial translation, lateral tibial translation, and transverse tibial rotation (Renström et al., 1986; Withrow et al., 2008). Therefore, the increased knee extension moment observed during landing in patients with CAI may be a potential mechanism leading to ACL injuries in this population.
Previous research has revealed that the contraction of the knee and hip extensors does not decrease VGRF during the landing contact phase; by contrast, the associated energy is transferred to the bones and ligaments, thereby increasing joint contact stress and the risk of ACL and meniscal injuries (Mills et al., 2009). In addition, this phenomenon has been observed in the biomechanical factors related to ACL injury and knee joint energy absorption. At the terminal phase of landing, knee joint energy absorption is inversely related to VGRF and hip extension moments and directly related to peak hip flexion angles (Norcross et al., 2010). These findings indicated that high hip extension moments and reduced hip flexion angles could lead to insufficient knee joint energy dissipation capacity, producing high ACL loads and increasing ACL injury risks (Alentorn-Geli et al., 2009; Norcross et al., 2010).
Overall, the biomechanical pattern of sagittal plane landings in the CAI group, characterized by increased knee and hip extension torques and reduced hip flexion angles, may provide a potential mechanism for the heightened risk of individual ACL injuries following CAI.
4.2 Frontal plane kinematics of the lower limb
Contrary to our expectations, no significant differences in hip abduction angles and knee abduction angles were observed between patients with CAI and the healthy group. Our review included four studies on hip abduction angles (Brown et al., 2011; Jeon and Park, 2021; Sagawa et al., 2024; Terada et al., 2015) and three on knee abduction angles (De Ridder et al., 2015; Jeon and Park, 2021; Lee et al., 2017). The collected data generally showed similar knee and hip abduction angles between patients with CAI and healthy individuals during jump-landing tasks, with only one study reporting a reduced knee abduction angle (Jeon and Park, 2021) (Figure 3). Therefore, our meta-analysis found no significant differences between the two groups. During landing tasks, patients with CAI and healthy individuals experienced similar frontal plane kinematics.
Excessive knee abduction angles and knee abduction torques during jump landing are known critical factors influencing ACL injuries (Hewett et al., 2005; Olsen et al., 2004; Quatman and Hewett, 2009). A prospective cohort study of 205 female athletes found that those who suffered ACL injuries had previously exhibited larger knee abduction angles, smaller hip abduction angles, and greater knee abduction torques than their counterparts, These variables were considered predictive of ACL injuries (Hewett et al., 2005). Therefore, avoiding large knee abduction angles and torques and small hip abduction angles during landing may help reduce ACL injury risks in patients with CAI. Given the limited research on related frontal plane dynamics, our study did not include metrics of knee abduction/adduction torques linked to ACL injuries. Future research should incorporate outcomes related to frontal plane biomechanics to elucidate the relationship between the landing frontal plane biomechanics of patents with CAI and ACL injury risks.
4.3 Impact loading
Our review included seven studies on peak VGRF (Caulfield and Garrett, 2004; De Ridder et al., 2015; Jeon and Park, 2021; Lee et al., 2017; Watabe et al., 2022; Watanabe et al., 2021; Zhang et al., 2012) and three on vertical loading rates (De Ridder et al., 2015; Jeon and Park, 2021; Lee et al., 2017) as measures of VGRF and loading rates. The results indicated that patients with CAI exhibited a comparable loading rate with healthy individuals, with an increased peak VGRF (Figure 4).
Previous reviews were consistent with our findings that is, patients with CAI have a high peak VGRF during landing (Jeon et al., 2021; Simpson et al., 2018), which has been identified as a non-contact ACL injury risk (Lin et al., 2012). A prospective study found that female athletes with knee injuries who later experienced ACL ruptures had a 20% higher peak VGRF than those without knee injuries and ACL damage (Hewett et al., 2005; Paterno et al., 2007). The maximum ACL load during landing tasks occurs at the moment of impact peak VGRF (Paterno et al., 2007; Watabe et al., 2022), with an increase in peak VGRF in patients with CAI leading to high ACL loading and great ACL injury risks. Therefore, minimizing the peak VGRF during landing may reduce ACL injury risks in patients with CAI.
The loading rate, as a factor contributing to ACL injuries during landing, has been noted in previous studies to be elevated in patients with CAI (Paterno et al., 2007; Simpson et al., 2018), Although this finding contradicted our results, no significant differences in loading rates were observed. This discrepancy may stem from our dataset, which included only a few studies on loading rates, highlighting the need for further research on this phenomenon.
Other impact loading–related metrics could be used to distinguish between abnormal lower limb impact loads in patients with CAI and healthy individuals. Frontal plane ground reaction forces (Hewett et al., 2009), sagittal plane ground reaction forces (Lin et al., 2012), and symmetry of landing forces are all associated with increased risks of ACL injuries (Paterno et al., 2007; Paterno et al., 2010; Paterno et al., 2011). However, these metrics were not included in this study. In the future, we will to incorporate more indicators of impact loading to clarify the relationship between impact loading during landing in patients with CAI and ACL injury risks.
4.4 Trunk mechanics
Low trunk flexion is associated with ACL injuries (Griffin et al., 2000). A cross-sectional cohort study found that female subjects with ACL injuries exhibited less trunk flexion compared with a control group of women, aligning their trunk with their legs in a way that increases valgus and axial forces (Hewett et al., 2009). High trunk flexion angles can prolong the time to reach peak VGRF, reduce landing impulse, and effectively absorb VGRF, decreasing the load on the ACL (Paterno et al., 2007; Watabe et al., 2022). However, this phenomenon not observed in patients with CAI. This review included two studies on this topic (Brown et al., 2011; Watabe et al., 2022). Watabe et al. (2022) studied the differences in lower limb and trunk biomechanics during active and passive single-leg landings between patients with CAI and healthy individuals, demonstrating that patients with CAI exhibit high trunk flexion angles in active and passive settings. Brown et al. (2011) examined the variability in movements during single-leg landing tasks among FAI, MAI, and healthy groups, and they found no significant differences in trunk flexion angles between patients with FAI/MAI and healthy individuals. Our review included these two studies (Brown et al., 2011; Watabe et al., 2022), and publication bias was statistically tested using Egger’s test (p = 0.007), indicating that the likelihood of publishing significant results may be higher than that for nonsignificant results. The presence of this bias necessitates a cautious approach in understanding and interpreting the relationship between trunk flexion and ACL injuries. Future research should utilize a broader range of data sources to ensure the comprehensiveness and balance of the findings. Hence, the clinical significance of the current findings remains uncertain.
Furthermore, our review revealed that patients with CAI exhibited greater trunk lateral flexion than healthy controls (Figure 5). A prospective study of over 900 athletes supported this finding, showing that female athletes with ankle injuries (such as CAI and lateral ankle sprains) demonstrate greater trunk sway compared with uninjured athletes (Beynnon et al., 2006). Insufficient neuromuscular control of the body’s core and decreased dynamic postural stability may contribute to increased trunk lateral flexion angles during landing (Kibler et al., 2006). The limitations associated with CAI have been shown to impair dynamic postural stability and neuromuscular control during unilateral jump landings, leading to sensorimotor function impairment (Caulfield et al., 2004; Caulfield and Garrett, 2002; Gribble and Robinson, 2009). Impaired neuromuscular control, especially in the hip muscles, is often observed in patients with CAI, who typically exhibit symptoms of deficient neuromuscular control (Bullock-Saxton et al., 1994; McCann et al., 2017; Terada et al., 2016). Therefore, the increased trunk lateral flexion observed in patients with CAI was due to decreased dynamic postural stability and inadequate neuromuscular control of the hip. Zazulak et al. (2007) suggested that trunk lateral displacement is a strong predictor of knee ligament injury, including ACL injuries. Their study of athletes over 3 years found that those with knee, ligament, or ACL injuries exhibit greater trunk displacement in all planes compared with uninjured athletes, identifying lateral displacement as the strongest predictor of ligament injuries. Trunk lateral flexion causes the lateral ground reaction force vector to shift sideways and, having a longer lever arm relative to the knee joint center, directly increases the likelihood of knee abduction motion and torque, thereby elevating the risk of ACL injuries (Hewett et al., 2009). Therefore, the landing mechanics of patients with CAI characterized by pronounced trunk lateral flexion could be factor contributing to the increased risk of ACL injuries in this population.
4.5 Limitation
Our study has the following limitations and weaknesses. First, the current research includes young athletes (under 27 years old) from various sports and regularly exercising individuals. Therefore, our results may not be applicable to other populations with different ages and levels of physical activity. Second, given limited previous research on horizontal biomechanical variables related to ACL injuries and knee coronal plane torque as outcome measures, we were unable to perform meta-analyses on these aspects. Third, many risky movements,such as cutting and sudden stopping, can lead to ACL injuries; considering the heterogeneity and representativeness of injury movements, we selected only landing actions for analysis. Fourth, our results only indicate the differences in landing biomechanics between individuals with CAI and healthy controls during landing tasks. We cannot ascertain whether these differences would vary with different experimental protocols. Even for the same type of jump-landing tasks, the implementation protocols were not uniform. We also observed variations in platform height and single versus double foot landings, which led to high heterogeneity in some outcome indicator analyses. Lastly, each meta-analysis included only a small number of studies; Thus, the results of this systematic review should be interpreted with caution.
5 Conclusion
This study confirmed the association between CAI and increased sagittal plane kinetics, reduced hip flexion angles, increased peak VGRF, and increased trunk lateral flexion, all of which are related to a heightened risk of ACL injuries during landing tasks. These findings provide a basis for improving the understanding of ACL injury risks during landing tasks in individuals with CAI. This knowledge can guide future preventative measures and rehabilitation strategies to mitigate ACL injury risks in this population.
Data availability statement
The original contributions presented in the study are included in the article/Supplementary Material further inquiries can be directed to the corresponding author.
Author contributions
ZH: Formal Analysis, Investigation, Methodology, Visualization, Writing–original draft, Writing–review and editing. HZ: Funding acquisition, Project administration, Supervision, Validation, Visualization, Writing–review and editing. BY: Investigation, Methodology, Writing–original draft, Writing–review and editing. ZZ: Data curation, Validation, Writing–review and editing. GL: Data curation, Validation, Writing–review and editing. HP: Supervision, Writing–review and editing. RL: Supervision, Validation, Visualization, Writing–review and editing.
Funding
The author(s) declare that financial support was received for the research, authorship, and/or publication of this article. The present study was funded by the Ministry of Education Humanities and Social Science Research Youth Fund Project (No. 22YJC890056), the Department of Science and Technology of Zhejiang Province (No. 2023C03197) and the National Undergraduate Training Program on Innovation and Entrepreneurship (No.202410345009).
Conflict of interest
The authors declare that the research was conducted in the absence of any commercial or financial relationships that could be construed as a potential conflict of interest.
Publisher’s note
All claims expressed in this article are solely those of the authors and do not necessarily represent those of their affiliated organizations, or those of the publisher, the editors and the reviewers. Any product that may be evaluated in this article, or claim that may be made by its manufacturer, is not guaranteed or endorsed by the publisher.
Supplementary material
The Supplementary Material for this article can be found online at: https://www.frontiersin.org/articles/10.3389/fphys.2024.1428879/full#supplementary-material
References
Agel J., Arendt E. A., Bershadsky B. (2005). Anterior cruciate ligament injury in national collegiate athletic association basketball and soccer: a 13-year review. Am. J. Sports Med. 33, 524–530. doi:10.1177/0363546504269937
Alentorn-Geli E., Myer G. D., Silvers H. J., Samitier G., Romero D., Lázaro-Haro C., et al. (2009). Prevention of non-contact anterior cruciate ligament injuries in soccer players. Part 1: mechanisms of injury and underlying risk factors. Knee Surg. Sports Traumatol. Arthrosc. 17, 705–729. doi:10.1007/s00167-009-0813-1
Arnold B. L., Wright C. J., Ross S. E. (2011). Functional ankle instability and health-related quality of life. J. Athl. Train. 46, 634–641. doi:10.4085/1062-6050-46.6.634
Beckman S. M., Buchanan T. S. (1995). Ankle inversion injury and hypermobility: effect on hip and ankle muscle electromyography onset latency. Arch. Phys. Med. Rehab 76, 1138–1143. doi:10.1016/s0003-9993(95)80123-5
Beynnon B., Vacek P. M., Abate III J. A., Murphy D., Paller D. (2006). A prospective study of risk factors for first time ankle inversion ankle ligament trauma.
Boden B. P., Torg J. S., Knowles S. B., Hewett T. E. (2009). Video analysis of anterior cruciate ligament injury: abnormalities in hip and ankle kinematics. Am. J. Sports Med. 37, 252–259. doi:10.1177/0363546508328107
Borenstein M., Hedges L. V., Higgins J. P., Rothstein H. R. (2021). Introduction to meta-analysis. John Wiley and Sons.
Brown C., Bowser B., Simpson K. J. (2011). Movement variability during single leg jump landings in individuals with and without chronic ankle instability. Clin. Biomech. 27, 52–63. doi:10.1016/j.clinbiomech.2011.07.012
Brown C., Ross S., Mynark R., Guskiewicz K. (2004). Assessing functional ankle instability with joint position sense, time to stabilization, and electromyography. J. Sport Rehabil. 13, 122–134. doi:10.1123/jsr.13.2.122
Bullock-Saxton J. E. (1994). Local sensation changes and altered hip muscle function following severe ankle sprain. Phys. Ther. 74, 17–28. doi:10.1093/ptj/74.1.17
Bullock-Saxton J. E., Janda V., Bullock M. I. (1994). The influence of ankle sprain injury on muscle activation during hip extension. Int. J. Sports Med. 15, 330–334. doi:10.1055/s-2007-1021069
Caulfield B., Crammond T., O Sullivan A., Reynolds S., Ward T. (2004). Altered ankle-muscle activation during jump landing in participants with functional instability of the ankle joint. J. Sport Rehabil. 13, 189–200. doi:10.1123/jsr.13.3.189
Caulfield B., Garrett M. (2004). Changes in ground reaction force during jump landing in subjects with functional instability of the ankle joint. Clin. Biomech. 19, 617–621. doi:10.1016/j.clinbiomech.2004.03.001
Caulfield B. M., Garrett M. (2002). Functional instability of the ankle: differences in patterns of ankle and knee movement prior to and post landing in a single leg jump. Int. J. Sports Med. 23, 64–68. doi:10.1055/s-2002-19272
Chan L. Y. T., Sim Y. T. N., Gan F. K., Bin Abd Razak H. R. (2022). Effect of chronic ankle instability on lower extremity kinematics, dynamic postural stability, and muscle activity during unilateral jump-landing tasks: a systematic review and meta-analysis. Phys. Ther. Sport 55, 176–188. doi:10.1016/j.ptsp.2022.04.005
Chou T., Huang Y., Leung W., Brown C. N., Kaminski T. W., Norcross M. F. (2023). Does prior concussion lead to biomechanical alterations associated with lateral ankle sprain and anterior cruciate ligament injury? A systematic review and meta-analysis. Brit J. Sport Med. 57, 1509–1515. doi:10.1136/bjsports-2023-106980
Davey A. P., Vacek P. M., Caldwell R. A., Slauterbeck J. R., Gardner-Morse M. G., Tourville T. W., et al. (2019). Risk factors associated with a noncontact anterior cruciate ligament injury to the contralateral knee after unilateral anterior cruciate ligament injury in high school and college female athletes: a prospective study. Am. J. Sports Med. 47, 3347–3355. doi:10.1177/0363546519886260
DeMorat G., Weinhold P., Blackburn T., Chudik S., Garrett W. (2004). Aggressive quadriceps loading can induce noncontact anterior cruciate ligament injury. Am. J. Sports Med. 32, 477–483. doi:10.1177/0363546503258928
De Ridder R., Willems T., Vanrenterghem J., Robinson M. A., Palmans T., Roosen P. (2015). Multi-segment foot landing kinematics in subjects with chronic ankle instability. Clin. Biomech. 30, 585–592. doi:10.1016/j.clinbiomech.2015.04.001
Devita P., Skelly W. A. (1992). Effect of landing stiffness on joint kinetics and energetics in the lower extremity. Med. Sci. Sports Exerc 24, 108–115. doi:10.1249/00005768-199201000-00018
Gribble P., Robinson R. (2009). Differences in spatiotemporal landing variables during a dynamic stability task in subjects with cai. Scand. J. Med. Sci. Spor 20, e63–e71. doi:10.1111/j.1600-0838.2009.00899.x
Gribble P. A., Bleakley C. M., Caulfield B. M., Docherty C. L., Fourchet F., Fong D. T., et al. (2016). Evidence review for the 2016 international ankle consortium consensus statement on the prevalence, impact and long-term consequences of lateral ankle sprains. Brit J. Sport Med. 50, 1496–1505. doi:10.1136/bjsports-2016-096189
Gribble P. A., Delahunt E., Bleakley C., Caulfield B., Docherty C., Fourchet F., et al. (2013). Selection criteria for patients with chronic ankle instability in controlled research: a position statement of the International Ankle Consortium. Brit J. Sport Med. 48, 1014–1018. doi:10.1136/bjsports-2013-093175
Gribble P. A., Robinson R. H. (2009). Alterations in knee kinematics and dynamic stability associated with chronic ankle instability. J. Athl. Train. 44, 350–355. doi:10.4085/1062-6050-44.4.350
Griffin L. Y., Agel J., Albohm M. J., Arendt E. A., Dick R. W., Garrett W. E., et al. (2000). Noncontact anterior cruciate ligament injuries: risk factors and prevention strategies. J. Am. Acad. Orthop. Sur 8, 141–150. doi:10.5435/00124635-200005000-00001
Han S., Lee H., Hopkins J. T. (2023). Effects of anticipation on joint kinematics during inversion perturbation in individuals with chronic ankle instability. Scand. J. Med. Sci. Spor 33, 1116–1124. doi:10.1111/sms.14342
Hass C. J., Bishop M. D., Doidge D., Wikstrom E. A. (2010). Chronic ankle instability alters central organization of movement. Am. J. Sports Med. 38, 829–834. doi:10.1177/0363546509351562
Heiderscheit B. C., Hamill J., Caldwell G. E. (2000). Influence of q-angle on lower-extremity running kinematics. J. Orthop. Sport Phys. 30, 271–278. doi:10.2519/jospt.2000.30.5.271
Hertel J., Corbett R. O. (2019). An updated model of chronic ankle instability. J. Athl. Train. 54, 572–588. doi:10.4085/1062-6050-344-18
Hewett T. E., Ford K. R., Hoogenboom B. J., Myer G. D. (2010). Understanding and preventing acl injuries: current biomechanical and epidemiologic considerations - update 2010. North Am. J. Sports Phys. Ther. Najspt 5, 234–251.
Hewett T. E., Lindenfeld T. N., Riccobene J. V., Noyes F. R. (1999). The effect of neuromuscular training on the incidence of knee injury in female athletes. A prospective study. Am. J. Sports Med. 27, 699–706. doi:10.1177/03635465990270060301
Hewett T. E., Myer G. D., Ford K. R., Heidt R. S., Colosimo A. J., McLean S. G., et al. (2005). Biomechanical measures of neuromuscular control and valgus loading of the knee predict anterior cruciate ligament injury risk in female athletes: a prospective study. Am. J. Sports Med. 33, 492–501. doi:10.1177/0363546504269591
Hewett T. E., Torg J. S., Boden B. P. (2009). Video analysis of trunk and knee motion during non-contact anterior cruciate ligament injury in female athletes: lateral trunk and knee abduction motion are combined components of the injury mechanism. Brit J. Sport Med. 43, 417–422. doi:10.1136/bjsm.2009.059162
Hubbard-Turner T., Turner M. J. (2015). Physical activity levels in college students with chronic ankle instability. J. Athl. Train. 50, 742–747. doi:10.4085/1062-6050-50.3.05
Jeon H. G., Lee S. Y., Park S. E., Ha S. (2021). Ankle instability patients exhibit altered muscle activation of lower extremity and ground reaction force during landing: a systematic review and meta-analysis. J. Sport Sci. Med. 20, 373–390. doi:10.52082/jssm.2021.373
Jeon K., Park J. (2021). Biomechanical characteristic on lower extremity with or without chronic ankle instability during double leg drop landing. Korean J. Sport Biomechanics 31, 113–118. doi:10.5103/KJSB.2021.31.2.113
Karita Y., Kimura Y., Yamamoto Y., Naraoka T., Sasaki S., Miura K., et al. (2017). Mechanisms of anterior cruciate ligament injuries in volleyball. Brit J. Sport Med. 51, 338–339. doi:10.1136/bjsports-2016-097372.140
Kibler W. B., Press J., Sciascia A. (2006). The role of core stability in athletic function. Sports Med. 36, 189–198. doi:10.2165/00007256-200636030-00001
Kikumoto T., Kobayashi M., Omori G., Kubo M. (2024). Single-legged landing behavior of high school basketball players with chronic ankle instability. J. Bodyw. Mov. Ther. 39, 454–462. doi:10.1016/j.jbmt.2024.03.054
Kim H., Son S. J., Seeley M. K., Hopkins J. T. (2017). Kinetic compensations due to chronic ankle instability during landing and jumping. Med. and Sci. Sports and Exerc. 50, 308–317. doi:10.1249/mss.0000000000001442
Kipp K., Palmieri-Smith R. M. (2012). Principal component based analysis of biomechanical inter-trial variability in individuals with chronic ankle instability. Clin. Biomech. 27, 706–710. doi:10.1016/j.clinbiomech.2012.02.005
Konradsen L., Voigt M. (2002). Inversion injury biomechanics in functional ankle instability: a cadaver study of simulated gait. Scand. J. Med. Sci. Spor 12, 329–336. doi:10.1034/j.1600-0838.2002.00108.x
Koshino Y., Yamanaka M., Ezawa Y., Ishida T., Kobayashi T., Samukawa M., et al. (2013). Lower limb joint motion during a cross cutting movement differs in individuals with and without chronic ankle instability. Phys. Ther. Sport 15, 242–248. doi:10.1016/j.ptsp.2013.12.001
Kramer L. C., Denegar C. R., Buckley W. E., Hertel J. (2007). Factors associated with anterior cruciate ligament injury: history in female athletes. J. Sports Med. Phys. Fit. 47, 446–454.
Kristianslund E., Faul O., Bahr R., Myklebust G., Krosshaug T. (2012). Sidestep cutting technique and knee abduction loading: implications for acl prevention exercises. Brit J. Sport Med. 48, 779–783. doi:10.1136/bjsports-2012-091370
Krosshaug T., Nakamae A., Boden B. P., Engebretsen L., Smith G., Slauterbeck J. R., et al. (2006). Mechanisms of anterior cruciate ligament injury in basketball: video analysis of 39 cases. Am. J. Sports Med. 35, 359–367. doi:10.1177/0363546506293899
Landis J. R., Koch G. G. (1977). The measurement of observer agreement for categorical data. Biometrics 33, 159–174. doi:10.2307/2529310
Lee M., Youm C., Son M., Kim J., Kim Y. (2017). Effects of chronic ankle instability and induced mediolateral muscular fatigue of the ankle on competitive taekwondo athletes. J. Phys. Ther. Sci. 29, 1329–1335. doi:10.1589/jpts.29.1329
Li Y., Ko J., Walker M. A., Brown C. N., Schmidt J. D., Kim S., et al. (2017). Does chronic ankle instability influence lower extremity muscle activation of females during landing? J. Electromyogr. Kines 38, 81–87. doi:10.1016/j.jelekin.2017.11.009
Lin C., Liu H., Gros M. T., Weinhold P., Garrett W. E., Yu B. (2012). Biomechanical risk factors of non-contact acl injuries: a stochastic biomechanical modeling study. J. Sport Health Sci. 1, 36–42. doi:10.1016/j.jshs.2012.01.001
Lohmander L. S., Ostenberg A., Englund M., Roos H. (2004). High prevalence of knee osteoarthritis, pain, and functional limitations in female soccer players twelve years after anterior cruciate ligament injury. Arthritis and Rheumatism 50, 3145–3152. doi:10.1002/art.20589
Mancino F., Gabr A., Plastow R., Haddad F. S. (2023). Anterior cruciate ligament injuries in female athletes. Bone and Jt. J. 105-B, 1033–1037. doi:10.1302/0301-620x.105b10.bjj-2023-0881.r1
McCann R. S., Crossett I. D., Terada M., Kosik K. B., Bolding B. A., Gribble P. A. (2017). Hip strength and star excursion balance test deficits of patients with chronic ankle instability. J. Sci. Med. Sport 20, 992–996. doi:10.1016/j.jsams.2017.05.005
Mills C., Pain M. T. G., Yeadon M. R. (2009). Reducing ground reaction forces in gymnastics' landings may increase internal loading. J. Biomech. 42, 671–678. doi:10.1016/j.jbiomech.2009.01.019
Mizuno Y., Kumagai M., Mattessich S. M., Elias J. J., Ramrattan N., Cosgarea A. J., et al. (2001). Q-angle influences tibiofemoral and patellofemoral kinematics. J. Orthop. Res. 19, 834–840. doi:10.1016/s0736-0266(01)00008-0
Moisan G., Descarreaux M., Cantin V. (2017). Effects of chronic ankle instability on kinetics, kinematics and muscle activity during walking and running: a systematic review. Gait Posture 52, 381–399. doi:10.1016/j.gaitpost.2016.11.037
Munn J., Sullivan S. J., Schneiders A. G. (2010). Evidence of sensorimotor deficits in functional ankle instability: a systematic review with meta-analysis. J. Sci. Med. Sport 13, 2–12. doi:10.1016/j.jsams.2009.03.004
Myklebust G., Bahr R. (2005). Return to play guidelines after anterior cruciate ligament surgery. Brit J. Sport Med. 39, 127–131. doi:10.1136/bjsm.2004.010900
Norcross M. F., Blackburn J. T., Goerger B. M., Padua D. A. (2010). The association between lower extremity energy absorption and biomechanical factors related to anterior cruciate ligament injury. Clin. Biomech. 25, 1031–1036. doi:10.1016/j.clinbiomech.2010.07.013
Olsen O., Myklebust G., Engebretsen L., Bahr R. (2004). Injury mechanisms for anterior cruciate ligament injuries in team handball: a systematic video analysis. Am. J. Sports Med. 32, 1002–1012. doi:10.1177/0363546503261724
Padua D. A., Marshall S. W., Boling M. C., Thigpen C. A., Garrett Jr W. E., Beutler A. I. (2009). The landing error scoring system (less) is a valid and reliable clinical assessment tool of jump-landing biomechanics: the jump-acl study. Am. J. Sports Med. 37, 1996–2002. doi:10.1177/0363546509343200
Paterno M. V., Ford K. R., Myer G. D., Heyl R., Hewett T. E. (2007). Limb asymmetries in landing and jumping 2 years following anterior cruciate ligament reconstruction. Clin. J. Sport Med. 17, 258–262. doi:10.1097/jsm.0b013e31804c77ea
Paterno M. V., Schmitt L. C., Ford K. R., Rauh M. J., Myer G. D., Hewett T. E. (2011). Effects of sex on compensatory landing strategies upon return to sport after anterior cruciate ligament reconstruction. J. Orthop. Sport Phys. 41, 553–559. doi:10.2519/jospt.2011.3591
Paterno M. V., Schmitt L. C., Ford K. R., Rauh M. J., Myer G. D., Huang B., et al. (2010). Biomechanical measures during landing and postural stability predict second anterior cruciate ligament injury after anterior cruciate ligament reconstruction and return to sport. Am. J. Sports Med. 38, 1968–1978. doi:10.1177/0363546510376053
Quatman C. E., Hewett T. E. (2009). The anterior cruciate ligament injury controversy: is "valgus collapse" a sex-specific mechanism? Brit J. Sport Med. 43, 328–335. doi:10.1136/bjsm.2009.059139
Renström P., Arms S. W., Stanwyck T. S., Johnson R. J., Pope M. H. (1986). Strain within the anterior cruciate ligament during hamstring and quadriceps activity. Am. J. Sports Med. 14, 83–87. doi:10.1177/036354658601400114
Sagawa Y., Yamada T., Ohmi T., Moriyama Y., Kato J. (2024). Differences in lower extremity kinematics during single-leg lateral drop landing of healthy individuals, injured but asymptomatic patients, and patients with chronic ankle instability-a cross-sectional observational study. Plos One 19, e0297660. doi:10.1371/journal.pone.0297660
Simpson J. D., Stewart E. M., Macias D. M., Chander H., Knight A. C. (2018). Individuals with chronic ankle instability exhibit dynamic postural stability deficits and altered unilateral landing biomechanics: a systematic review. Phys. Ther. Sport 37, 210–219. doi:10.1016/j.ptsp.2018.06.003
Söderman K., Alfredson H., Pietilä T., Werner S. (2001). Risk factors for leg injuries in female soccer players: a prospective investigation during one out-door season. Knee Surg. Sports Traumatol. Arthrosc. 9, 313–321. doi:10.1007/s001670100228
Sueki D. G., Cleland J. A., Wainner R. S. (2013). A regional interdependence model of musculoskeletal dysfunction: research, mechanisms, and clinical implications. J. Man. and Manip. Ther. 21, 90–102. doi:10.1179/2042618612y.0000000027
Terada M., Ball L. M., Pietrosimone B. G., Gribble P. A. (2015). Altered visual focus on sensorimotor control in people with chronic ankle instability. J. Sport Sci. 34, 171–180. doi:10.1080/02640414.2015.1043324
Terada M., Kosik K. B., McCann R. S., Gribble P. A. (2016). Diaphragm contractility in individuals with chronic ankle instability. Med. and Sci. Sports and Exerc. 48, 2040–2045. doi:10.1249/mss.0000000000000994
Terada M., Pietrosimone B., Gribble P. A. (2014). Individuals with chronic ankle instability exhibit altered landing knee kinematics: potential link with the mechanism of loading for the anterior cruciate ligament. Clin. Biomech. 29, 1125–1130. doi:10.1016/j.clinbiomech.2014.09.014
Theisen A., Day J. (2019). Chronic ankle instability leads to lower extremity kinematic changes during landing tasks: a systematic review. Texas, United States: International Journal of Exercise Science.
Trigsted S. M., Post E. G., Bell D. R. (2017). Landing mechanics during single hop for distance in females following anterior cruciate ligament reconstruction compared to healthy controls. Knee Surg. Sports Traumatol. Arthrosc. 25, 1395–1402. doi:10.1007/s00167-015-3658-9
van Putte-Katier N., van Ochten J. M., van Middelkoop M., Bierma-Zeinstra S. M. A., Oei E. H. G. (2015). Magnetic resonance imaging abnormalities after lateral ankle trauma in injured and contralateral ankles. Eur. J. Radiol. 84, 2586–2592. doi:10.1016/j.ejrad.2015.09.028
Watabe T., Takabayashi T., Tokunaga Y., Watanabe T., Kubo M. (2022). Copers exhibit altered ankle and trunk kinematics compared to the individuals with chronic ankle instability during single-leg landing. Sport Biomech., 1–13. doi:10.1080/14763141.2022.2058989
Watanabe K., Koshino Y., Ishida T., Samukawa M., Tohyama H. (2021). Energy dissipation during single-leg landing from three heights in individuals with and without chronic ankle instability. Sport Biomech. 21, 408–427. doi:10.1080/14763141.2021.2009549
Withrow T. J., Huston L. J., Wojtys E. M., Ashton-Miller J. A. (2008). Effect of varying hamstring tension on anterior cruciate ligament strain during in vitro impulsive knee flexion and compression loading. J. Bone and Jt. Surg. 90, 815–823. doi:10.2106/jbjs.f.01352
Xu Y., Song B., Ming A., Zhang C., Ni G. (2022). Chronic ankle instability modifies proximal lower extremity biomechanics during sports maneuvers that may increase the risk of acl injury: a systematic review. Front. Physiol. 13, 1036267. doi:10.3389/fphys.2022.1036267
Zazulak B. T., Hewett T. E., Reeves N. P., Goldberg B., Cholewicki J. (2007). Deficits in neuromuscular control of the trunk predict knee injury risk: a prospective biomechanical-epidemiologic study. Am. J. Sports Med. 35, 1123–1130. doi:10.1177/0363546507301585
Keywords: chronic ankle instability, proximal, joint biomechanics, landing, anterior cruciate ligament
Citation: He Z, Zhu H, Ye B, Zheng Z, Liu G, Pan H and Liu R (2024) Does chronic ankle instability patients lead to changes in biomechanical parameters associated with anterior cruciate ligament injury during landing? A systematic review and meta-analysis. Front. Physiol. 15:1428879. doi: 10.3389/fphys.2024.1428879
Received: 07 May 2024; Accepted: 16 August 2024;
Published: 29 August 2024.
Edited by:
Qipeng Song, Shandong Sport University, ChinaReviewed by:
Luca Russo, University of eCampus, ItalyJia Han, Shanghai University of Medicine and Health Sciences, China
Copyright © 2024 He, Zhu, Ye, Zheng, Liu, Pan and Liu. This is an open-access article distributed under the terms of the Creative Commons Attribution License (CC BY). The use, distribution or reproduction in other forums is permitted, provided the original author(s) and the copyright owner(s) are credited and that the original publication in this journal is cited, in accordance with accepted academic practice. No use, distribution or reproduction is permitted which does not comply with these terms.
*Correspondence: Ronghua Liu, WjIwMDgyNjZAc2h1ZmUtemouZWR1LmNu
†These authors have contributed equally to this work