- 1Department of Entomology, MOA Key Lab of Pest Monitoring and Green Management, College of Plant Protection, China Agricultural University, Beijing, China
- 2College of Food Science and Nutritional Engineering, China Agricultural University, Beijing, China
- 3College of Horticulture and Plant Protection, Henan University of Science and Technology, Luoyang, China
Introduction: Plumbagin is an important phytochemical and has been reported to exhibit potent larvicidal activity against several insect pests, However, the insecticidal mechanism of plumbagin against pests is still poorly understood. This study aimed to investigate the insecticidal activities of plumbagin and the underlying molecular mechanisms against a devastating agricultural pest, the fall armyworm Spodoptera frugiperda.
Methods: The effects of plumbagin on S. frugiperda larval development and the activities of two detoxification enzymes were initially examined. Next, transcriptomic changes in S. frugiperda after plumbagin treatment were investigated. Furthermore, RNA-seq results were validated by qPCR.
Results: Plumbagin exhibited a high larvicidal activity against the second and third instar larvae of S. frugiperda with 72 h LC50 of 0.573 and 2.676 mg/g, respectively. The activities of the two detoxification enzymes carboxylesterase and P450 were significantly increased after 1.5 mg/g plumbagin treatment. Furthermore, RNA-seq analysis provided a comprehensive overview of complex transcriptomic changes in S. frugiperda larvae in response to 1.5 mg/g plumbagin exposure, and revealed that plumbagin treatment led to aberrant expression of a large number of genes related to nutrient and energy metabolism, humoral immune response, insect cuticle protein, chitin-binding proteins, chitin synthesis and degradation, insect hormone, and xenobiotic detoxification. The qPCR results further validated the reproducibility and reliability of the transcriptomic data.
Discussion: Our findings provide a valuable insight into understanding the insecticidal mechanism of the phytochemical plumbagin.
1 Introduction
The fall armyworm, Spodoptera frugiperda (J.E. Smith), a migratory, highly polyphagous, and widely distributed destructive lepidopteran pest species, is native to tropical and subtropical areas of the Americas (Kenis et al., 2023). Since 2016, this pest has invaded West Africa, and then rapidly spread to sub-Saharan Africa nations, Asia, and parts of Oceania such as southern Australia (Kenis et al., 2023; Tay et al., 2023). In China, S. frugiperda was first observed in January 2019 from maize field in Jiangcheng County, Yunnan Province (Guo et al., 2023; Sun et al., 2021), and then quickly spread to 27 provinces (autonomous regions and municipalities) across China, and thus being considered to be a major invasive crop pest posing a significant threat to crop production and agricultural food security (Wang et al., 2023). Generally, most farmers and agricultural practices in many invasive regions have relied primarily on the application of synthetic pesticides to manage S. frugiperda. Unfortunately, their improper application and excessive utilization have brought various negative effects such as nontarget toxicity, pesticide residue accumulation in agricultural products and the environment, and resistance of this pest to spinosad, diamides and Bacillus thuringiensis (Zhao et al., 2022; Tay et al., 2023). Over the past few years, significant progress has been made in the development of effective and sustainable management strategies to control this devastating pest in many recently invaded countries, including China. For example, effective and eco-friendly botanical pesticides have received increasing attention in sustainable agriculture, and are recognized as one of the most promising alternatives to synthetic insecticides for the development and practical implementation of integrated pest management programmes (Kenis et al., 2023).
Plumbagin, 5-hydroxy-2-methyl-1,4-naphthoquinone (C11H8O3), is an important secondary metabolite and bioactive compound in plants originally isolated from the medicinal plant Plumbago zeylanica and has been reported to exhibit potent larvicidal activity against several insect pests, including S. litura (Tokunaga et al., 2004; Rajendra Prasad et al., 2012), Achaea Janata (Rajendra Prasad et al., 2012), Trichoplusia ni (Akhtar et al., 2012a), Musca domestica (Pavela, 2013), Helicoverpa armigera (Hu et al., 2018), Pieris rapae (Hu et al., 2018), Mythimna separate (Shang et al., 2019), Nilaparvata lugens (Shang et al., 2019), S. littoralis (Davila-Lara et al., 2021; Rahman-Soad et al., 2021), Aedes aegypti (de Oliveira et al., 2022), and three aphid species (Akhtar et al., 2012b), as well as acaricidal activity against the herbivorous mite Tetranychus urticae (Akhtar et al., 2012b). The results of these studies have indicated that plumbagin has great potential to be developed as a potent botanical insecticide and an alternative to synthetic insecticides. However, little is known about the acute toxicity of plumbagin on S. frugiperda and the underlying molecular mechanisms of larvae in response to plumbagin exposure.
Recently, rapid and remarkable developments in sequencing technology and increasing numbers of complete insect genomes have opened up new avenues for exploring global transcriptome changes reflecting the physiological state of insect pests to plant metabolites exposures, unveiling previously unknown mechanisms and mining functional genes (Lin et al., 2023; Li et al., 2023). Huang et al. (2018) used RNA-Seq and qPCR to characterize the expression levels of detoxification genes, especially P450 genes in Sitophilus zeamais responding to terpinen-4-ol, the main constituent of Melaleuca alternifolia essential oil. Li et al. (2023) analyzed transcriptome profiling of linalool-exposed Pagiophloeus tsushimanus larvae using RNA-seq and single-molecule real-time sequencing. Gene ontology enrichment of DEGs revealed that overall upregulation of DEGs encoding cytochrome P450s and cuticular proteins was the primary response characteristic in larvae upon exposure to linalool. The effects of several plant metabolites such as carvacrol (Liu et al., 2023), toosendanin (Lin et al., 2023), camptothecin (Shu et al., 2021a; Shu et al., 2021b), azadirachtin (Shu et al., 2021c), and chlorogenic acid (Lin et al., 2023) on gene expression profiles and enzyme activities in S. frugiperda larvae have recently been studied using RNA-seq and enzyme activity assays in order to elucidate the response mechanism. These findings indicated that these metabolites influenced the normal physiological activities of S. frugiperda at multiple levels.
In this study, the effects of plumbagin on S. frugiperda larval development and on the activities of two detoxification enzymes were examined to assess its bioactivity against this devastating agricultural pest. Next, transcriptomic changes of S. frugiperda after plumbagin treatment were investigated to elucidate the underlying molecular mechanism of its insecticidal activity. Furthermore, RNA-seq results were confirmed by qPCR. Our results will provide a better understanding of the toxicological mechanism of plumbagin used as a botanical pesticide against pests.
2 Material and methods
2.1 Insect
Eggs of S. frugiperda used in this study were supplied by the Institute of Plant Protection, Chinese Academy of Agricultural Sciences, which were collected from maize fields in Jiangcheng, Yunnan Province, China. The larvae were reared on artificial diet in a growth room at 26°C ± 1°C, 70% ± 5% relative humidity, with a photoperiod of 14:10 (L:D) as described previously (Su et al., 2023).
2.2 Bioassays and enzyme activity assays
Plumbagin (CAS number: 481-42-5) was purchased from Sigma-Aldrich. Dissolved in acetone, plumbagin was incorporated into the artificial diet at various concentrations of 3, 1.5, 0.75, 0.375, 0.1875, 0.09375 mg/g. Artificial diet incorporating acetone was used as control. A single second or third instar S. frugiperda larvae was placed in petri dish (35 mm in diameter × 2 cm in height) and reared on 1 g artificial diet. They were allowed to feed for 7 days, and mortality was recorded. Three biological replicates, each comprising a total of 20 larvae, were analyzed. The larval weight of surviving S. frugiperda was also recorded daily for 4 days.
Ten third instar larvae fed on diet containing 1.5 mg/g plumbagin were homogenized in 1 mL phosphate buffer solution. The homogenate was centrifuged for 20 min (13,000 rpm, 4°C), and the supernatants were collected for further enzyme activity assays. The enzyme activities of two detoxification enzymes carboxylesterase (CarE) and P450 were determined using the Carboxylesterase Test Kit (A133-1-1) and the Cytochrome P450 Assay Kit (H303-1-2) from Nanjing Jiancheng Bioengineering Institute (Jiangsu, China) following the manufacturer’s protocol.
2.3 RNA isolation and illumina sequencing
Third-instar S. frugiperda larvae were provided with an artificial diet incorporating 1.5 mg/g plumbagin as described above for bioassays. The larvae fed with an artificial diet containing acetone were used as controls. After 3 days, the survival larvae were collected and then subjected to RNA extraction and RNA sequencing analysis. Total RNA extraction was performed using TRIzol reagent (Invitrogen) following the manufacturer’s instructions. At least four biological replicates were analyzed for each treatment.
RNA purity was evaluated using the NanoPhotometer® spectrophotometer (IMPLEN, CA, United States). A total of 3 μg RNA per sample was utilized for cDNA library preparation with the NEBNext Ultra RNA Library Prep Kit according to the manufacturer’s instructions. Samples were sequenced on an Illumina NovaSeq6000 platform (Biomics Biotech Co., Ltd., Beijing, China). Clean reads were aligned to the chromosome-level assembled genome of S. frugiperda available at http://v2.insect-genome.com/Organism/715 using TopHat v2.0.12 software. Gene Ontology (GO) and KEGG pathway enrichment analyses for differentially expressed genes (DEGs) were conducted using the GOseq R package and KOBAS software, respectively. Genes with a log2|fold change| > 1 and FDR < 0.05 were considered as DEGs.
2.4 qPCR validation
The qPCR validation was carried out based on SuperReal PreMix Plus (SYBR Green) reagent kit (TianGen, Beijing, China), according to the manufacturer’s instructions (Huang et al., 2018) and using the same samples used for RNA-seq. The qPCR programme was 95°C for 10 min, followed by 40 cycles of 95°C for 15 s and 60°C for 30 s. The gene β-actin was used as a reference gene as described previously (Su et al., 2023). The primers for qPCR were listed in Supplementary Table S1.
2.5 Statistical analysis
POLO Plus software was used to calculate the median lethal concentration (LC50), with corresponding 95% confidence interval (CI) and chi-square (χ2) of the probit regression equation. For data on larval weight and enzyme activity assay, statistical analysis was performed using one-way analysis of variance (ANOVA) with LSD/Duncan pairwise comparison testing.
3 Results
3.1 Bioactivity of plumbagin against Spodoptera frugiperda larvae
The toxicity of plumbagin to S. frugiperda was determined using second or third instar larvae. The results showed that plumbagin exhibited significant insecticidal activity against S. frugiperda larvae, and the LC50 values for second instar and third instar were 0.573 and 2.676 mg/g, respectively (Table 1). The mortality rate of 1.5 mg/g plumbagin treatment after 7 days was 91.53% (Figure 1A). The survival larvae during 1.5 mg/g plumbagin treatment displayed lower weight compared to controls, with weight reduction from 43.57 to 15.90 mg after 4 days (Figure 1B).
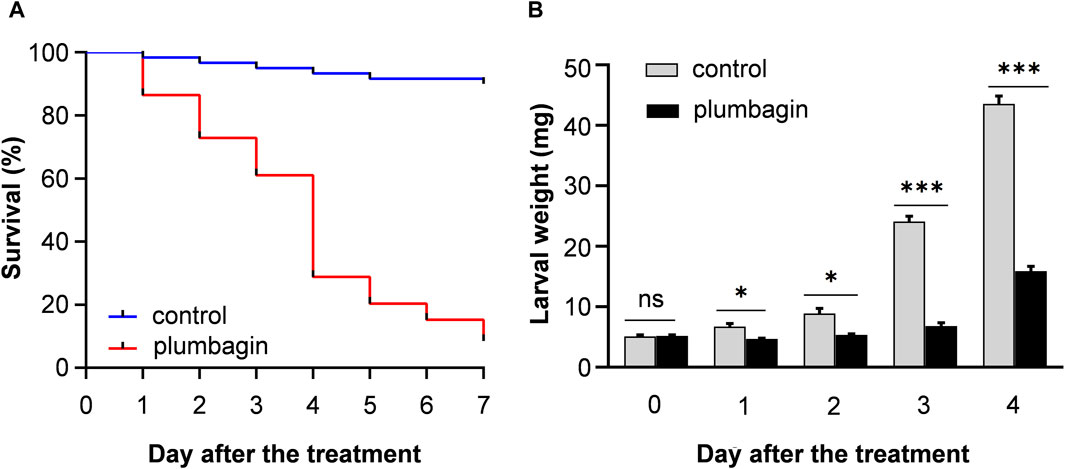
Figure 1. The survival rate (A) and larval weight (B) of S. frugiperda after 1.5 mg/g plumbagin treatment.
3.2 Enzyme activities of two detoxification enzymes in S. frugiperda larvae after plumbagin exposure
Furthermore, enzyme activity assays revealed that 1.5 mg/g plumbagin treatment significantly improved the activities of the two detoxification enzymes CarE and P450 compared with the control, with a 4.13- and 1.72-fold increase, respectively (Figure 2).
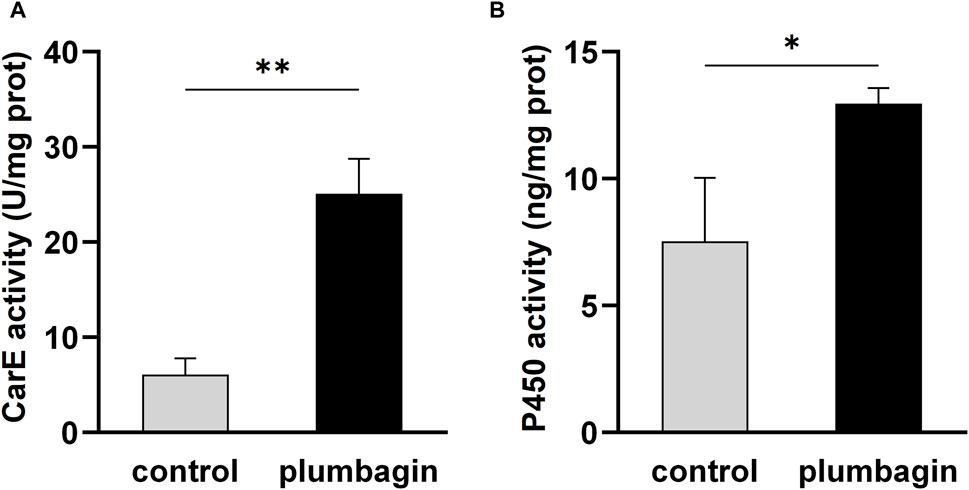
Figure 2. The activities of two detoxification enzymes in third-instar S. frugiperda larvae after 1.5 mg/g plumbagin treatment. (A) Carboxylesterase (CarE) activity. (B) P450 activity.
3.3 Global transcriptome changes in S. frugiperda larvae after plumbagin exposure
Transcriptome sequencing of S. frugiperda larvae upon plumbagin exposure was conducted to explore the molecular mechanisms underlying the larvicidal activity of plumbagin. Nine independent cDNA libraries were generated, comprising four for the plumbagin treatment group and five for the control group. The raw reads number ranged from 41,489,334 to 45,978,878, while high quality clean reads varied between 40,213,076 and 45,148,130. The proportion of mapped clean reads in each sample ranged from 77.56% to 80.91% (Supplementary Table S2). Correlation within each group of experimental and control samples were generally high (Figure 3A). Principal component analysis (PCA) showed that the plumbagin-exposed samples and controls formed distinct clusters, indicating plumbagin exposure led to aberrant expression of genes in S. frugiperda larvae (Figure 3B).
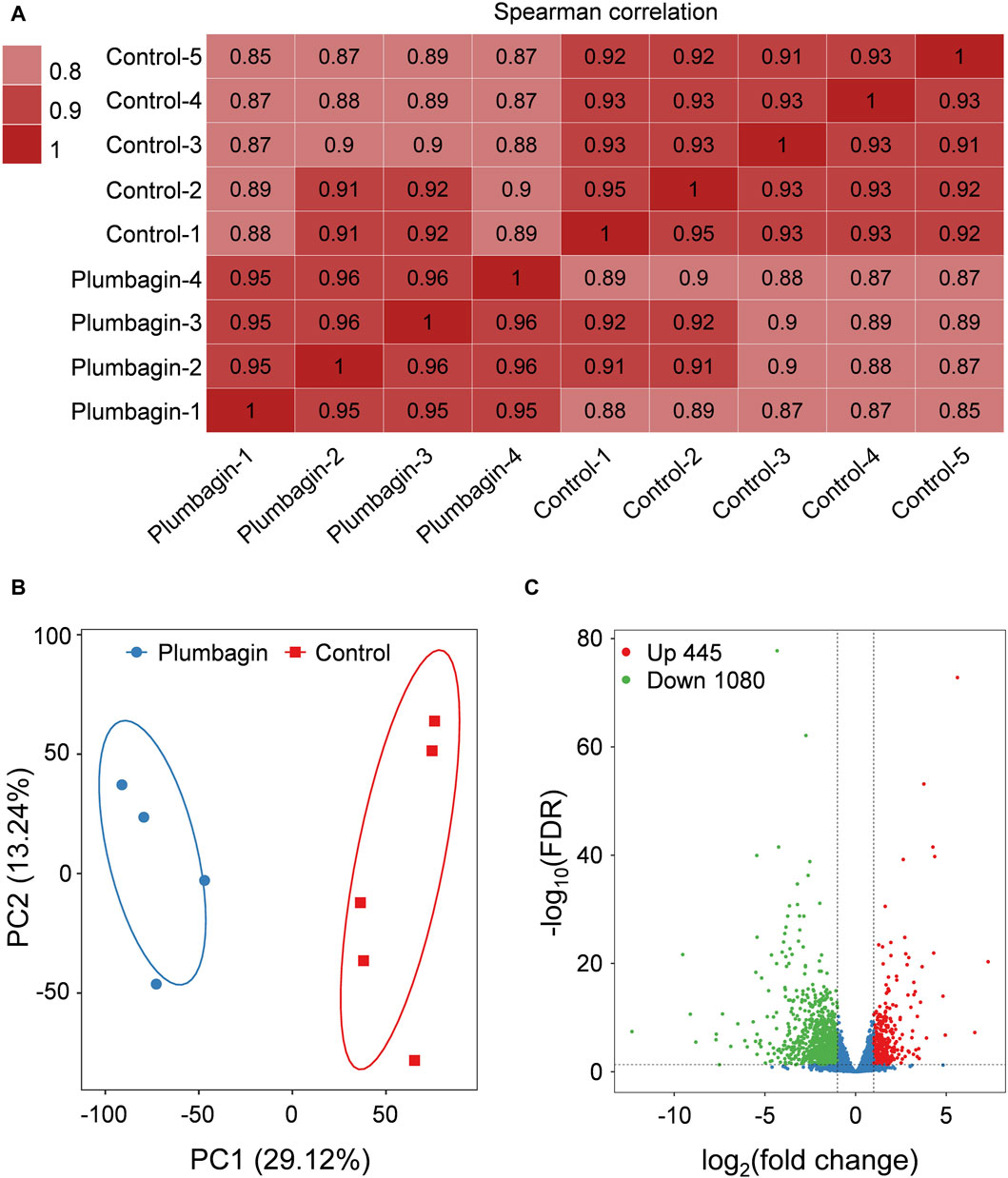
Figure 3. Transcriptome profile of RNA-Seq data. (A) Correlation matrix of the samples utilizing Spearman’s correlation coefficients. (B) Principal component analysis of the samples. (C) Volcano plot of RNA-Seq data.
3.4 Functional category of plumbagin-responsive DEGs in S. frugiperda larvae
After plumbagin exposure, a total of 1,614 genes with a log2|fold change| > 1 and FDR < 0.05 were identified as differentially expressed genes (DEGs) in S. frugiperda larvae. Among these DEGs, 445 were upregulated and 1,080 were downregulated (Figure 3C).
Functional enrichment analysis including GO annotation enrichment, KOG functional enrichment and KEGG pathway analysis were conducted to explore the functional categories of these DEGs (Figures 4, 5), which showed that the plumbagin treatment exhibited profound strong adverse effects on many essential genes involved in amino acid transport and metabolism (75 out of 104 DEGs), carbohydrate transport and metabolism (43 out of 54 DEGs), energy production and conversion (23 out of 28 DEGs), lipid transport and metabolism (51 out of 69 DEGs), nucleotide transport and metabolism (15 out of 23 DEGs), and replication, recombination and repair (31 out of 35 DEGs). Also, most DEGs related to humoral immune response (38 out of 50 DEGs), insect cuticle protein (21 out of 27 DEGs), insect hormone (27 out of 38 DEGs), detoxification enzymes (49 out of 74 DEGs) and digestive enzymes (69 out of 81 DEGs) were downregulated. It is noteworthy that all DEGs associated with chitin synthesis and degradation (8 of 8) and chitin-binding proteins (15 of 15) were downregulated, while all of the 10 DEGs related to endocytosis were upregulated (Figures 4, 5).
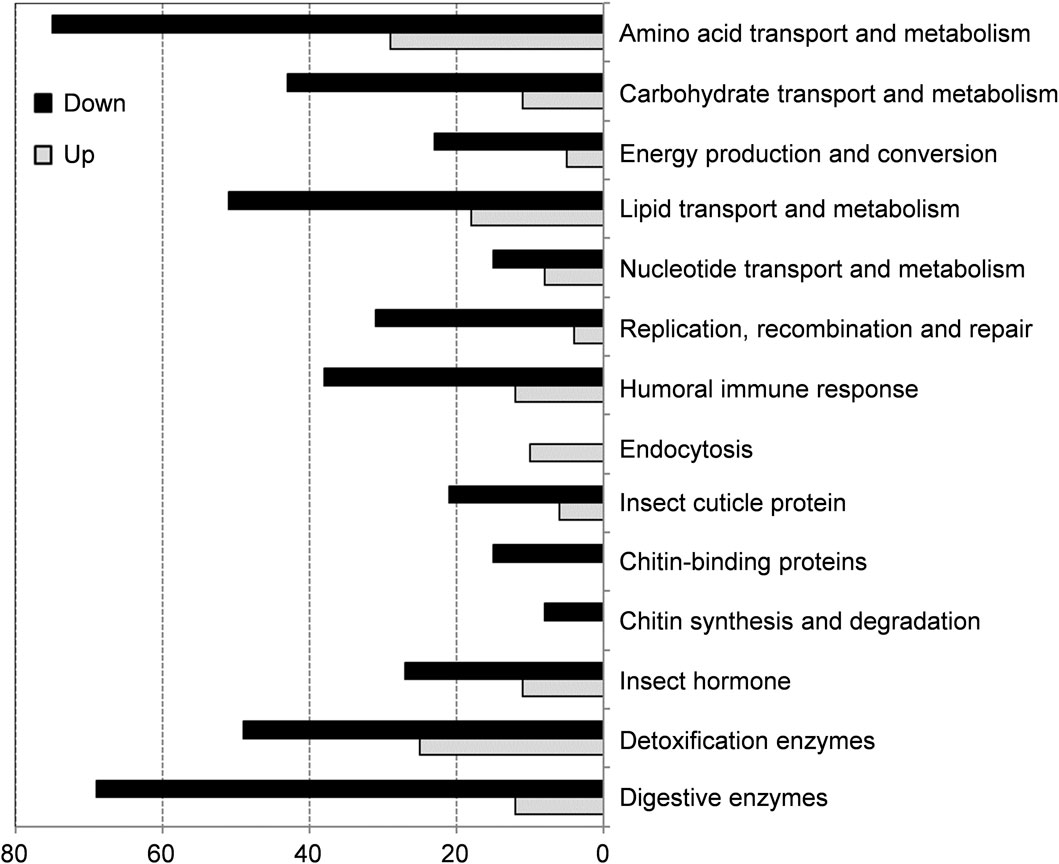
Figure 4. Enrichment analyses of differentially expressed genes (DEGs) in S. frugiperda larvae after 1.5 mg/g plumbagin treatment.
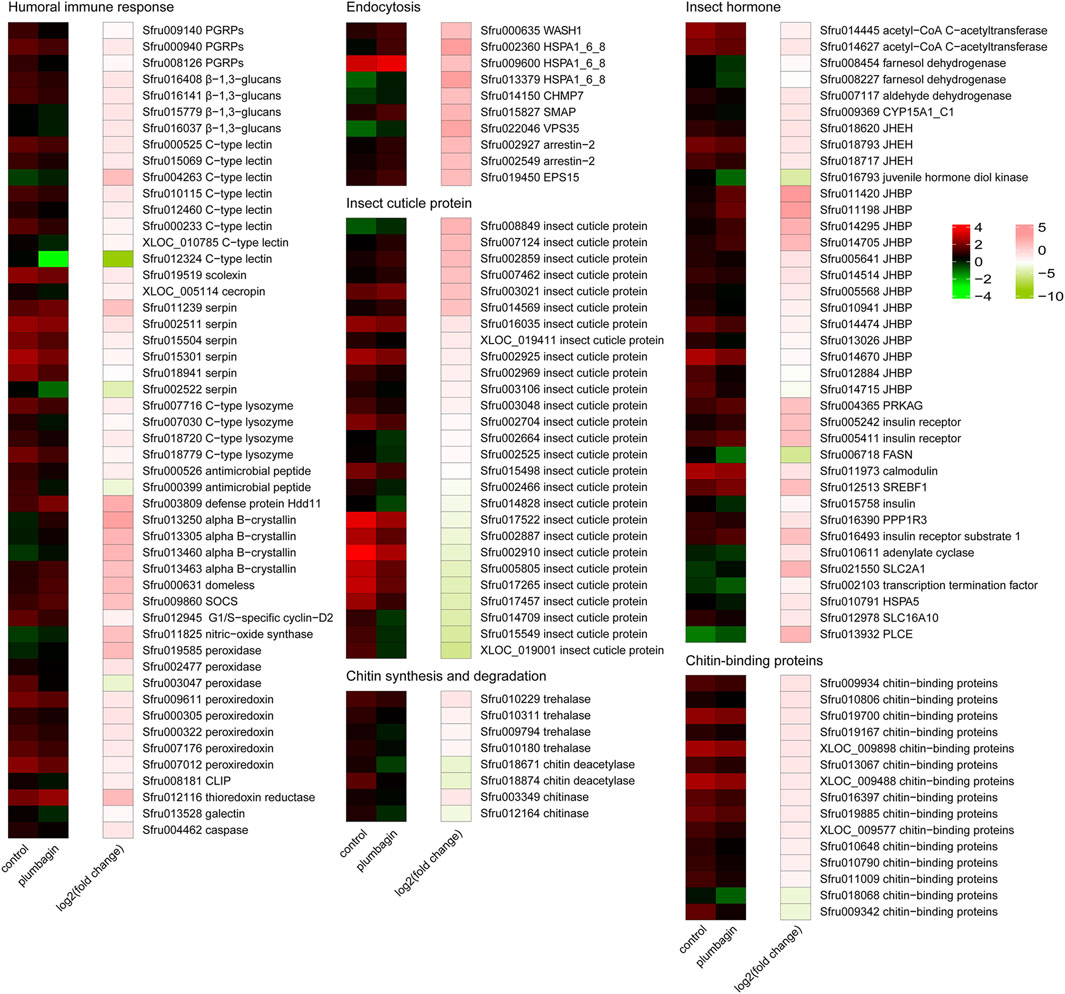
Figure 5. Heatmap analysis of differentially expressed genes (DEGs) in S. frugiperda larvae after plumbagin treatment. CLIP, clip domain serine protease; SOCS, suppressor of cytokine signaling 2; PGRPs, peptidoglycan recognition proteins; CHMP7, charged multivesicular body protein 7; HSPA1_6_8, heat shock 70 kDa protein 1/2/6/8; SMAP, stromal membrane-associated protein; VPS35, vacuolar protein sorting-associated protein 35; EPS15, epidermal growth factor receptor substrate 15; JHEH, juvenile hormone epoxide hydrolase; JHBP, haemolymph juvenile hormone binding protein; PRKAG, 5′-AMP-activated protein kinase, regulatory gamma subunit; FASN, fatty acid synthase, animal type; SREBF1, sterol regulatory element-binding transcription factor 1; PPP1R3, protein phosphatase 1 regulatory subunit 3A/B/C/D/E; SLC2A1, solute carrier family 2 (facilitated glucose transporter), member 1; HSPA5, heat shock 70 kDa protein 5; SLC16A10, solute carrier family 16 (monocarboxylic acid transporters), member 10; PLCE, phosphatidylinositol phospholipase C, epsilon; CYP15A1_C1, methyl farnesoate epoxidase/farnesoate epoxidase; WASH1, WAS protein family homolog 1.
3.5 Validation of transcriptomic data by qPCR
To validate the RNA-seq results, nine genes were analyzed by qPCR, which are involved in humoral immune response (XLOC_005114 and Sfru004462), insect hormone (Sfru007117) chitin synthesis and degradation (Sfru010180 and Sfru012164) detoxification enzymes (Sfru020044, XLOC_004422 and Sfru016345) digestive enzymes (Sfru017470) were selected and analyzed by qPCR. The qPCR results exhibited a high level of concordance with the transcriptomic data (Figure 6), confirming the reproducibility and reliability of the transcriptomic data.
4 Discussion
In this study, the bioassay experiments demonstrated that plumbagin, a natural small molecule naphthoquinone compound in plants, exhibited a high insecticidal activity against the larvae of the distributed destructive lepidopteran pest S. frugiperda, consistent with previously reported results that the secondary metabolite has high toxicity to several other lepidopteran pests (Tokunaga et al., 2004; Akhtar et al., 2012a; Rajendra Prasad et al., 2012; Hu et al., 2018; Shang et al., 2019; Dávila-Lara et al., 2021; Rahman-Soad et al., 2021). The findings demonstrated that plumbagin has great potential to serve as a botanical pesticide for controlling second or third instar larvae. However, according to the Globally Harmonized System (GHS) of Classification and Labelling of Chemicals, the toxicity and LC50 value of plumbagin against S. frugiperda classify it as a Class 4 acute toxicant for humans. This classification may restrict its use as a pesticide. To overcome this limitation, the encapsulation of plumbagin in nanocarriers could be a promising method, enhancing its bioactivity and allowing for reduced dosages and frequencies, thereby effectively minimizing harm to humans and mammals.
To better understand molecular mechanisms underlying the insecticidal effects of plumbagin against S. frugiperda, RNA-seq analysis and subsequent qPCR experiments were conducted, which demonstrated that plumbagin treatment could stimulate complex, global transcriptome changes in S. frugiperda larvae. Transcriptomic analysis indicated that plumbagin exposure had profound adverse effects on biological and metabolic processes of three nutrients (proteins, lipids and carbohydrates), which are the principal sources of nutrients and energy for insects, and are essential for their normal growth and development (Toprak et al., 2020). Most DEGs related to amino acid transport and metabolism (75 out of 104 genes), lipid transport and metabolism (51 out of 69 genes), and carbohydrate transport and metabolism (43 out of 54 genes) were downregulated (Figures 4, 5). Moreover, most DEGs involved in protein digestion (7 of 8), lipid digestion (13 of 21), and carbohydrate digestion (49 of 52), were downregulated in S. frugiperda larvae after plumbagin treatment, which are important for absorption and utilization of these three nutrients. The general suppression of these growth-related genes indicated that plumbagin exposure displayed strong adverse effects on larval growth and development, strongly correlating with bioassay results. Similarly, carvacrol treatment significantly affect the expression levels of genes involved in the metabolism carbohydrates, lipids and proteins in Lymantria dispar (Chen et al., 2022).
Transcriptomic analysis showed that plumbagin treatment strongly altered the expression of genes encoding detoxification enzymes/proteins, including CarEs (5 up- and 17 downregulated genes), P450s (6 up- and 19 downregulated genes), Glutathione S-transferases (GSTs; 4 up- and 5 downregulated genes), UDP-glycosyltransferases (UGTs; 5 up- and 6 downregulated genes), alkaline phosphatases (2 downregulated genes), and ABC transporters (5 upregulated genes). These detoxification enzymes have been reported to play multiple roles in metabolic detoxification of a variety of xenobiotics (Amezian et al., 2021; Giraudo et al., 2015; Li et al., 2007; Vandenhole et al., 2021). For example, the UGT33 family member SfUGT33F28 in S. frugiperda is responsible for detoxification of the major maize defensive compound DIMBOA (2,4-dihydroxy-7-methoxy-1,4-benzoxazin-3-one) (Israni et al., 2020). CYP9A subfamily genes in two insect pests Spodoptera exigua and S. frugiperda collectively metabolize two furanocoumarin plant defense compounds (imperatorin and xanthotoxin) and three insecticides (pyrethroids, avermectins, and oxadiazines) (Shi et al., 2023). The carboxylesterase SexiCXE11 in S. exigua is able to degrade two plant allelochemicals pentyl acetate and (Z)-3-hexenyl caproate with >50% degradation (He et al., 2020). Although most DEGs responsible for CarEs and P450s were downregulated in S. frugiperda larvae (Figures 4, 5), enzyme activity assays revealed that plumbagin treatment led to a significant increase in enzyme activities of both CarEs and P450 (Figure 2). Possible explanations for this phenomenon could be the involvement of post-transcriptional regulatory mechanisms and the contributions of enzyme isoforms with different expression and activity profiles. The inconsistency between RNA-seq data at the transcriptional level and enzyme assay results at the protein level are also reported in previous studies. For example, toosendanin exposure inhibited the expression of most DEGs encoding lipases, while it increased lipase enzyme activity in S. frugiperda larvae to overcome the adverse effects of the xenobiotic toosendanin (Lin et al., 2023). Notably, the six upregulated P450s (Sfru004887, Sfru020044, Sfru009692, Sfru004769, Sfru011158 and Sfru011561) are mainly concentrated in the CYP6 family and CYP301 family, which are also induced by phytochemicals in other insects, such as H. armigera and Aphis gossypii, and are associated with plant allelochemical detoxification in insects (Vandenhole et al., 2021). The expression level of one CYP301A1 gene (Sfru020044) were upregulated by 1.55- and 2.97 in RNA-seq and qPCR data, respectively, which was also induced by the phenolic monoterpenoid carvacrol in S. frugiperda larvae (Liu et al., 2023). Interestingly, all of five DEGs for the detoxification enzyme ABC transporters (Sfru016345, Sfru007970, Sfru007513, Sfru007645 and Sfru007518) and all of two DEGs for sodium channel protein (XLOC_025616 and Sfru014814), one type of the insecticide targets, were upregulated in plumbagin-treated larvae, highlighting their potential roles in S. frugiperda larvae in response to plumbagin exposure.
Plumbagin treatment led to the downregulation of many DEGs related to humoral immune responses. Almost all DEGs (14 of 15) associated with pattern recognition proteins/receptors including peptidoglycan recognition (Sfru009140, Sfru000940 and Sfru008126), protein beta-glucan recognition protein (Sfru016408, Sfru016141, Sfru015779 and Sfru016037) and c-type lectin including Sfru000525, Sfru015069 and Sfru004263, which plays critical roles in initiating humoral immune responses (Tsakas et al., 2010), were downregulated. Besides, several other immune-related genes such as one scolexin (Sfru019519), one cecropin (XLOC_005114), four C-type lysozymes (Sfru007716, Sfru007030, Sfru018720 and Sfru018779), two antimicrobial peptides (Sfru000526 and Sfru000399), five peroxiredoxin (Sfru009611, and Sfru000305, Sfru000322, Sfru007176 and Sfru007012) were also downregulated. Serpin (serine protease inhibitor) is a key negative regulator of melanization responses (Jiravanichpaisal et al., 2006). Almost all DEGs (5 of 6) for serpin, including Sfru002511, Sfru015504, Sfru015301, Sfru018941 and Sfru002522, were downregulated, which suggested that serpin-mediated immunity might be activated by plumbagin treatment. Moreover, all of 10 endocytosis-related genes (Sfru000635, Sfru002360, Sfru009600, Sfru013379, Sfru014150, Sfru015827, Sfru022046, Sfru002927, Sfru002549 and Sfru019450), all of 4 alpha-crystallin (Sfru013250, Sfru013305, Sfru013460 and Sfru013463), and almost all DEGs (3 out of 4) involved in JAK-STAT signaling pathway (Sfru000631, Sfru009860 and Sfru009860) were upregulated. These findings indicated that plumbagin treatment triggered complex immune responses in S. frugiperda larvae.
Cuticle proteins and chitin are the major components of insect cuticle and midgut peritrophic membrane (Shu et al., 2021b). After plumbagin treatment, most DEGs (21 of 27) for insect cuticle proteins, such as Sfru008849, Sfru007124 and Sfru002859, and one DEG for skin secretory protein (Sfru015138) were downregulated. Similar to our results, Shu et al. (2022) reported that azadirachtin treatment led to the downregulation of many genes for cuticle proteins S. frugiperda larvae, whereas the DEGs related to cuticle proteins and skin secretory proteins were upregulated in P. tsushimanus larvae after linalool exposure to decrease the penetration of this volatile compound (Li et al., 2023). Besides, plumbagin inhibited the expression of all DEGs (8 genes) involved in chitin synthesis and degradation pathway, including four trehalase (Sfru010229, Sfru010311, Sfru009794 and Sfru010180), two chitin deacetylase (Sfru018671 and Sfru018874) and two chitinase A (Sfru003349 and Sfru012164). Trehalase is the first enzyme in chitin biosynthesis, and suppression of trehalase resulted in lethality and morphological defects in several insects (Liu et al., 2019). In present study, all of 4 trehalases were downregulated, which might disrupt chitin synthesis and degradation, and consequently led to a decrease in chitin content. Also, all of 15 DEGs associated with chitin-binding proteins (Sfru009934, Sfru010806, Sfru019700, Sfru019167, XLOC_009898, Sfru013067, XLOC_009488, Sfru016397, Sfru019885, XLOC_009577, Sfru010648, Sfru010790, Sfru011009, Sfru018068 and Sfru009342) were downregulated, which play essential roles in forming and maintaining of chitin-containing structures (Tetreau et al., 2015).
5 Conclusion
In summary, our results illustrated that plumbagin treatment exhibited a high larvicidal activity against S. frugiperda larvae in the bioassay experiments. Consistently, a large number of the DEGs responsible for nutrient and energy metabolism, humoral immune response, insect cuticle protein, chitin-binding proteins, chitin synthesis and degradation, insect hormone, and xenobiotic detoxification were suppressed in RNA-seq data. Conversely, the expression of DEGs involved in endocytosis and the activities of the two detoxification enzymes CarE and P450 were enhanced after plumbagin treatment. These results will help to elucidate insecticidal mechanisms of botanical insecticides against pests.
Data availability statement
The transcriptome sequencing data presented in this study are deposited in the NCBI BioProject database (https://www.ncbi.nlm.nih.gov/bioproject), accession number PRJNA1119741. Further inquiries can be directed to the corresponding author.
Ethics statement
The manuscript presents research on animals that do not require ethical approval for their study.
Author contributions
XS: Investigation, Methodology, Writing–original draft. WL: Investigation, Methodology, Writing–original draft, Writing–review and editing. SY: Formal Analysis, Writing–original draft. XN: Formal Analysis, Writing–original draft. SH: Resources, Writing–original draft. MW: Resources, Writing–original draft. CZ: Conceptualization, Writing–original draft, Writing–review and editing. XH: Conceptualization, Writing–original draft, Writing–review and editing.
Funding
The author(s) declare that financial support was received for the research, authorship, and/or publication of this article. This work was supported by the National Key Research and Development Program of China (2021YFD1400701).
Conflict of interest
The authors declare that the research was conducted in the absence of any commercial or financial relationships that could be construed as a potential conflict of interest.
Publisher’s note
All claims expressed in this article are solely those of the authors and do not necessarily represent those of their affiliated organizations, or those of the publisher, the editors and the reviewers. Any product that may be evaluated in this article, or claim that may be made by its manufacturer, is not guaranteed or endorsed by the publisher.
Supplementary material
The Supplementary Material for this article can be found online at: https://www.frontiersin.org/articles/10.3389/fphys.2024.1427385/full#supplementary-material
References
Akhtar Y., Isman M. B., Lee C. H., Lee S. G., Lee H. S. (2012b). Toxicity of quinones against two-spotted spider mite and three species of aphids in laboratory and greenhouse conditions. Ind. Crop Prod. 37 (1), 536–541. doi:10.1016/j.indcrop.2011.07.033
Akhtar Y., Isman M. B., Niehaus L. A., Lee C. H., Lee H. S. (2012a). Antifeedant and toxic effects of naturally occurring and synthetic quinones to the cabbage looper, Trichoplusia ni. Crop Prot. 31 (1), 8–14. doi:10.1016/j.cropro.2011.09.009
Amezian D., Nauen R., Le Goff G. (2021). Comparative analysis of the detoxification gene inventory of four major Spodoptera pest species in response to xenobiotics. Insect biochem. Mol. Biol. 138, 103646. doi:10.1016/j.ibmb.2021.103646
Chen Y. Z., Li T., Yang J., Li Q. M., Zhang G. C., Zhang J. (2022). Transcriptomic analysis of interactions between Lymantria dispar larvae and carvacrol. Pestic. Biochem. Physiol. 181, 105012. doi:10.1016/j.pestbp.2021.105012
Dávila-Lara A., Rahman-Soad A., Reichelt M., Mithöfer A. (2021). Carnivorous Nepenthes x ventrata plants use a naphthoquinone as phytoanticipin against herbivory. PLoS One 16 (10), e0258235. doi:10.1371/journal.pone.0258235
de Oliveira P. M. C., Sousa J. P. B., Albernaz L. C., Coelho-Ferreira M., Espindola L. S. (2022). Bioprospection for new larvicides against Aedes aegypti based on ethnoknowledge from the Amazonian Sao Sebastiao de Marinau riverside community. J. Ethnopharmacol. 293, 115284. doi:10.1016/j.jep.2022.115284
Giraudo M., Hilliou F., Fricaux T., Audant P., Feyereisen R., Le Goff G. (2015). Cytochrome P450s from the fall armyworm (Spodoptera frugiperda): responses to plant allelochemicals and pesticides. Insect molec. Biol. 24 (1), 115–128. doi:10.1111/imb.12140
Guo J. F., Shi J. Q., Han H. L., Rwomushana I., Ali A., Myint Y., et al. (2023). Competitive interactions between invasive fall armyworm and Asian corn borer at intraspecific and interspecific level on the same feeding guild. Insect Sci. doi:10.1111/1744-7917.13300
He P., Mang D. Z., Wang H., Wang M. M., Ma Y. F., Wang J., et al. (2020). Molecular characterization and functional analysis of a novel candidate of cuticle carboxylesterase in Spodoptera exigua degradating sex pheromones and plant volatile esters. Pestic. Biochem. Physiol. 163, 227–234. doi:10.1016/j.pestbp.2019.11.022
Hu W. Y., Du W. C., Bai S. M., Lv S. T., Chen G. (2018). Phenoloxidase, an effective bioactivity target for botanical insecticide screening from green walnut husks. Nat. Prod. Res. 32 (23), 2848–2851. doi:10.1080/14786419.2017.1380015
Huang Y., Liao M., Yang Q. Q., Xiao J. J., Hu Z. Y., Zhou L. J., et al. (2018). Transcriptome profiling reveals differential gene expression of detoxification enzymes in Sitophilus zeamais responding to terpinen-4-ol fumigation. Pestic. Biochem. Physiol. 149, 44–53. doi:10.1016/j.pestbp.2018.05.008
Israni B., Wouters F. C., Luck K., Seibel E., Ahn S. J., Paetz C., et al. (2020). The fall armyworm Spodoptera frugiperda utilizes specific UDP-glycosyltransferases to inactivate maize defensive benzoxazinoids. Front. Physiol. 11, 604754. doi:10.3389/fphys.2020.604754
Jiravanichpaisal P., Lee B. L., Söderhäll K. (2006). Cell-mediated immunity in arthropods: hematopoiesis, coagulation, melanization and opsonization. Immunobiology 211 (4), 213–236. doi:10.1016/j.imbio.2005.10.015
Kenis M., Benelli G., Biondi A., Calatayud P. A., Day R., Desneux N., et al. (2023). Invasiveness, biology, ecology, and management of the fall armyworm, Spodoptera frugiperda. Entomol. Gen. 43 (2), 187–241. doi:10.1127/entomologia/2022/1659
Li S. Y., Li H., Chen C., Hao D. J. (2023). Tolerance to dietary linalool primarily involves co-expression of cytochrome P450s and cuticular proteins in Pagiophloeus tsushimanus (Coleoptera: Curculionidae) larvae using SMRT sequencing and RNA-seq. BMC Genomics 24 (1), 34. doi:10.1186/s12864-023-09117-7
Li X. C., Schuler M. A., Berenbaum M. R. (2007). Molecular mechanisms of metabolic resistance to synthetic and natural xenobiotics. Annu. Rev. Entomol. 52, 231–253. doi:10.1146/annurev.ento.51.110104.151104
Lin D. J., Zhang Y. X., Fang Y., Gao S. J., Wang R., Wang J. D. (2023a). The effect of chlorogenic acid, a potential botanical insecticide, on gene transcription and protein expression of carboxylesterases in the armyworm (Mythimna separata). Pestic. Biochem. Physiol. 195, 105575. doi:10.1016/j.pestbp.2023.105575
Lin Y. Z., Huang Y. T., Liu J. F., Liu L. Y., Cai X. M., Lin J. T., et al. (2023b). Characterization of the physiological, histopathological, and gene expression alterations in Spodoptera frugiperda larval midguts affected by toosendanin exposure. Pestic. Biochem. Physiol. 195, 105537. doi:10.1016/j.pestbp.2023.105537
Liu J. F., Lin Y. Z., Huang Y. T., Liu L. Y., Cai X. M., Lin J. T., et al. (2023). The effects of carvacrol on development and gene expression profiles in Spodoptera frugiperda. Pestic. Biochem. Physiol. 195, 105539. doi:10.1016/j.pestbp.2023.105539
Liu X. J., Cooper A. M. W., Zhang J. Z., Zhu K. Y. (2019). Biosynthesis, modifications and degradation of chitin in the formation and turnover of peritrophic matrix in insects. J. Insect Physiol. 114, 109–115. doi:10.1016/j.jinsphys.2019.03.006
Pavela R. (2013). Efficacy of naphthoquinones as insecticides against the house fly, Musca domestica L. Ind. Crop Prod. 43, 745–750. doi:10.1016/j.indcrop.2012.08.025
Rahman-Soad A., Dávila-Lara A., Paetz C., Mithöfer A. (2021). Plumbagin, a potent naphthoquinone from Nepenthes plants with growth inhibiting and larvicidal activities. Molecules 26, 825. doi:10.3390/molecules26040825
Rajendra Prasad K., Suresh Babu K., Ranga Rao R., Suresh G., Rekha K., Madhusudana Murthy J., et al. (2012). Synthesis and insect antifeedant activity of plumbagin derivatives. Med. Chem. Res. 21, 578–583. doi:10.1007/s00044-011-9559-7
Shang X. F., Zhao Z. M., Li J. C., Yang G. Z., Liu Y. Q., Dai L. X., et al. (2019). Insecticidal and antifungal activities of Rheum palmatum L. anthraquinones and structurally related compounds. Ind. Crop Prod. 137, 508–520. doi:10.1016/j.indcrop.2019.05.055
Shi Y., Liu Q., Lu W., Yuan J., Yang Y., Oakeshott J., et al. (2023). Divergent amplifications of CYP9A cytochrome P450 genes provide two noctuid pests with differential protection against xenobiotics. Proc. Natl. Acad. Sci. U. S. A. 120 (37), e2308685120. doi:10.1073/pnas.2308685120
Shu B. S., Lin Y. Z., Qian G. Z., Cai X. M., Liu L. Y., Lin J. T. (2022). Integrated miRNA and transcriptome profiling to explore the molecular mechanism of Spodoptera frugiperda larval midgut in response to azadirachtin exposure. Pestic. Biochem. Physiol. 187, 105192. doi:10.1016/j.pestbp.2022.105192
Shu B. S., Yang X. M., Dai J. H., Yu H. K., Yu J. K., Li X. L., et al. (2021a). Effects of camptothecin on histological structures and gene expression profiles of fat bodies in Spodoptera frugiperda. Ecotox. Environ. Safe. 228, 112968. doi:10.1016/j.ecoenv.2021.112968
Shu B. S., Yu H. K., Li Y. N., Zhong H. X., Li X. L., Cao L., et al. (2022). Identification of azadirachtin responsive genes in Spodoptera frugiperda larvae based on RNA-seq. Pestic. Biochem. Physiol. 172, 104745. doi:10.1016/j.pestbp.2020.104745
Shu B. S., Zou Y., Yu H. K., Zhang W. Y., Li X. L., Cao L., et al. (2021b). Growth inhibition of Spodoptera frugiperda larvae by camptothecin correlates with alteration of the structures and gene expression profiles of the midgut. BMC Genomics 22 (1), 391. doi:10.1186/s12864-021-07726-8
Su C. Y., Liu S. S., Sun M. X., Yu Q. L., Li C. Y., Graham R. I., et al. (2023). Delivery of methoprene-tolerant dsRNA to improve RNAi efficiency by modified liposomes for pest control. ACS Appl. Mater. Inter. 15, 13576–13588. doi:10.1021/acsami.2c20151
Sun X. X., Hu C. X., Jia H. R., Wu Q. L., Shen X. J., Zhao S. Y., et al. (2021). Case study on the first immigration of fall armyworm, Spodoptera frugiperda invading into China. J. Integr. Agr. 20 (3), 664–672. doi:10.1016/S2095-3119(19)62839-X
Tay W. T., Meagher R. L., Czepak C., Groot A. T. (2023). Spodoptera frugiperda: ecology, evolution, and management options of an invasive species. Annu. Rev. Entomol. 68, 299–317. doi:10.1146/annurev-ento-120220-102548
Tetreau G., Dittmer N. T., Cao X. L., Agrawal S., Chen Y. R., Muthukrishnan S., et al. (2015). Analysis of chitin-binding proteins from Manduca sexta provides new insights into evolution of peritrophin A-type chitin-binding domains in insects. Insect biochem. Mol. Biol. 62, 127–141. doi:10.1016/j.ibmb.2014.12.002
Tokunaga T., Takada N., Ueda M. (2004). Mechanism of antifeedant activity of plumbagin, a compound concerning the chemical defense in carnivorous plant. Tetrahedron Lett. 45 (38), 7115–7119. doi:10.1016/j.tetlet.2004.07.094
Toprak U., Hegedus D., Doğan C., Güney G. (2020). A journey into the world of insect lipid metabolism. Insect Biochem. Physiol. 104 (2), e21682. doi:10.1002/arch.21682
Tsakas S., Marmaras V. J. (2010). Insect immunity and its signalling: an overview. Invert. Surviv. J. 7 (2), 228–238.
Vandenhole M., Dermauw W., Van Leeuwen T. (2021). Short term transcriptional responses of P450s to phytochemicals in insects and mites. Curr. Opin. Insect Sci. 43, 117–127. doi:10.1016/j.cois.2020.12.002
Wang H. H., Zhao R., Gao J., Zhang L., Zhang S., Liang P., et al. (2023). Genetic architecture and insecticide resistance in Chinese populations of Spodoptera frugiperda. J. Pest Sci. 96 (4), 1595–1610. doi:10.1007/s10340-022-01569-2
Zhao R., Wang H. H., Gao J., Zhang Y. J., Li X. C., Zhou J. J., et al. (2022). Plant volatile compound methyl benzoate is highly effective against Spodoptera frugiperda and safe to non-target organisms as an eco-friendly botanical-insecticide. Ecotox. Environ. Safe. 245, 114101. doi:10.1016/j.ecoenv.2022.114101
Keywords: Spodoptera frugiperda, plumbagin, RNA-seq, detoxification enzyme, toxicological mechanism
Citation: Sun X, Li W, Yang S, Ni X, Han S, Wang M, Zhen C and Huang X (2024) Insecticidal activity and underlying molecular mechanisms of a phytochemical plumbagin against Spodoptera frugiperda. Front. Physiol. 15:1427385. doi: 10.3389/fphys.2024.1427385
Received: 03 May 2024; Accepted: 31 May 2024;
Published: 21 June 2024.
Edited by:
Bin Tang, Hangzhou Normal University, ChinaReviewed by:
Agata Kaczmarek, Polish Academy of Sciences, PolandSaif Ul Malook, University of Florida, United States
Copyright © 2024 Sun, Li, Yang, Ni, Han, Wang, Zhen and Huang. This is an open-access article distributed under the terms of the Creative Commons Attribution License (CC BY). The use, distribution or reproduction in other forums is permitted, provided the original author(s) and the copyright owner(s) are credited and that the original publication in this journal is cited, in accordance with accepted academic practice. No use, distribution or reproduction is permitted which does not comply with these terms.
*Correspondence: Xinzheng Huang, aHVhbmd4aW56aGVuZ0BjYXUuZWR1LmNu; Cong’ai Zhen, emhlbmNvbmdhaUAxMjYuY29t
†These authors have contributed equally to this work