- Department of Medicine, Division of Cardiovascular Medicine, Vanderbilt University Medical Center, Nashville, TN, United States
Salt-inducible kinases (SIKs) are serine/threonine kinases of the adenosine monophosphate-activated protein kinase family. Acting as mediators of a broad array of neuronal and hormonal signaling pathways, SIKs play diverse roles in many physiological and pathological processes. Phosphorylation by the upstream kinase liver kinase B1 is required for SIK activation, while phosphorylation by protein kinase A induces the binding of 14-3-3 protein and leads to SIK inhibition. SIKs are subjected to auto-phosphorylation regulation and their activity can also be modulated by Ca2+/calmodulin-dependent protein kinase in response to cellular calcium influx. SIKs regulate the physiological processes through direct phosphorylation on various substrates, which include class IIa histone deacetylases, cAMP-regulated transcriptional coactivators, phosphatase methylesterase-1, among others. Accumulative body of studies have demonstrated that SIKs are important regulators of the cardiovascular system, including early works establishing their roles in sodium sensing and vascular homeostasis and recent progress in pulmonary arterial hypertension and pathological cardiac remodeling. SIKs also regulate inflammation, fibrosis, and metabolic homeostasis, which are essential pathological underpinnings of cardiovascular disease. The development of small molecule SIK inhibitors provides the translational opportunity to explore their potential as therapeutic targets for treating cardiometabolic disease in the future.
Introduction
Salt-inducible kinases (SIKs), including SIK1, SIK2, and SIK3, are serine/threonine kinases of the adenosine monophosphate-activated protein kinase (AMPK) family (Jagannath et al., 2023). The founding member SIK1 was first described as a myocardial sucrose-nonfermenting 1 (SNF1)-like kinase (msk) involving mouse heart development (Ruiz et al., 1994). It was cloned later as a protein kinase of 776 amino acids from rats adrenal gland after high-salt diet treatment (Wang et al., 1999), thereby the name of salt-inducible kinase was first devised. The two additional members SIK2 and SIK3 were identified later through in silicon studies based on protein sequence similarity (Horike et al., 2003; Katoh et al., 2004a).
The three SIK isoforms are broadly expressed in vertebrate tissues. Based on the transcriptomic data from human and mouse tissues, SIK1, SIK2, and SIK3 are mostly enriched in the adrenal gland, the adipose tissue, and the brain, respectively (Fagerberg et al., 2014; Yue et al., 2014). SIK activities are dynamically regulated by multiple physiological cues through both transcriptional and post-translational mechanisms. SIK1 expression is regulated by cyclic AMP (cAMP) through the action of the cAMP responsive element binding (CREB) protein (Jagannath et al., 2013). The mRNA expression of SIK1 can be induced by many physiological cues, such as high-salt dietary intake (Wang et al., 1999), membrane depolarization (Feldman et al., 2000), adrenocorticotropic hormone (Lin et al., 2001), fasting (Koo et al., 2005), adrenergic stimulations (Kanyo et al., 2009), transforming growth factor β (TGFβ) signaling (Lönn et al., 2012), circadian clock (Jagannath et al., 2013), and hypertrophic cardiac stresses (Hsu et al., 2020). In contrast, SIK2 and SIK3 are constitutively expressed but their expression can be modulated under certain pathophysiological conditions. For example, SIK2 and SIK3 mRNA expression are downregulated in the adipose tissue of diabetic and obese individuals (Säll et al., 2016).
SIK proteins process an N-terminal serine/threonine kinase domain (KD), followed by a ubiquitin-associated (UBA) domain, and a C-terminal domain (CTD) (Figure 1). The KD is well-conserved among the three isoforms. It contains a liver kinase B1 (LKB1) phosphorylation threonine site in the activation loop (T-loop). The UBA domain is located within a SNF1 homolog (SNH) region following the KD. The regulatory CTD varies in length among the three isoforms and contains multiple protein kinase A (PKA) phosphorylation sites. SIK1 also has nuclear localization sequences (NLS) (Katoh et al., 2002) and it can repress CREB activity both in the nucleus and cytoplasm (Katoh et al., 2004b).
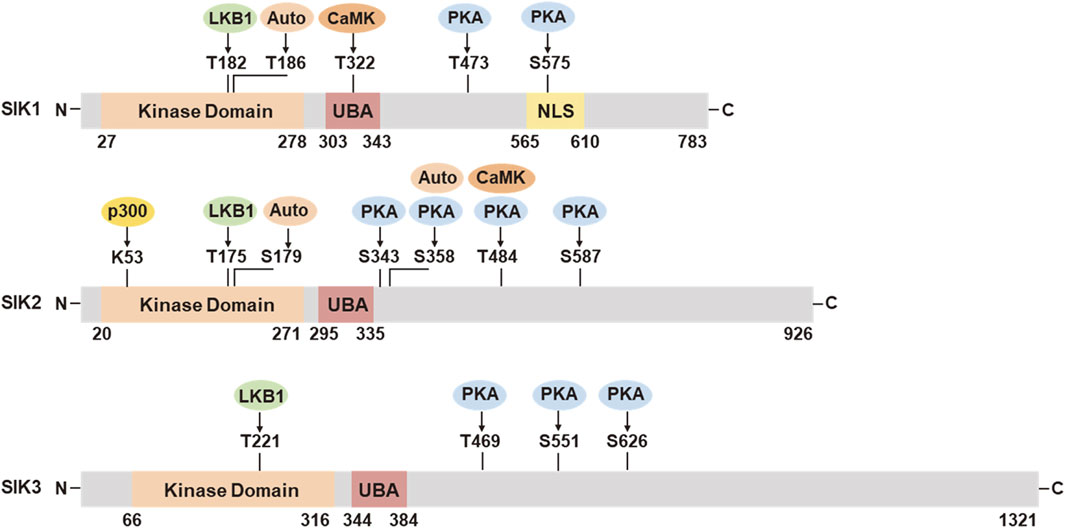
Figure 1. Functional domains and post-translational modifications in SIK1, SIK2, and SIK3. UBA, ubiquitin associated domain; NLS, nuclear location sequences; LKB1, liver kinase B1; Auto, autocatalysis; PKA, protein kinase A; CaMK, Ca2+/calmodulin dependent kinase; p300, histone acetyltransferase. The numbers of amino acid residues refer to human proteins SIK1 (NP_775490.2), SIK2 (NP_056006.1), and SIK3 (NP_079440.3), respectively.
SIK activities are tightly regulated through post-translational modification in response to hormonal stimulation and physiological alternations (Figure 1). The activation of SIK kinase activity requires the phosphorylation by LKB1 in the activation loop near the substrate binding sites (SIK1 Thr182, SIK2 Thr175, and SIK3 Thr221). The UBA domain assists the LKB1 phosphorylation and SIK kinase activation, potentially by preventing the binding of SIKs with the 14-3-3 phospho-binding proteins (Al-Hakim et al., 2005; Jaleel et al., 2006). Activation by LKB1 stimulates the autophosphorylation in the activation loop of SIK1 (Ser186) and SIK2 (Ser179), which are important for sustained kinase activity (Hashimoto et al., 2008). SIK2 can also be auto-phosphorylated at Ser358, which regulates it protein stability (Henriksson et al., 2012). SIK1 and SIK2 can be phosphorylated by Ca2+/calmodulin dependent kinase (CaMK). Intracellular sodium-mediated SIK1 activation requires its phosphorylation by CaMK I at Thr322 (Sjöström et al., 2007; Bertorello and Zhu, 2009). Phosphorylation of SIK2 by CaMK I/V at Ser484 promotes its protein degradation (Sasaki et al., 2011). PKA phosphorylation sites have been identified in SIK1 (Thr473, Ser575), SIK2 (Ser343, Ser358, Thr484, Ser587), and SIK3 (Thr469, Ser551, Ser626) (Sonntag et al., 2018). PKA phosphorylation facilitates the binding of 14-3-3 protein and leads to SIK inhibition. PKA-mediated SIK inhibition is underpinning a variety of neuronal and hormonal factor actions, such as glucagon, prostaglandins, parathyroid hormone, and α-melanocyte stimulation hormone (Sakamoto et al., 2018; Wein et al., 2018). SIKs are also subjected to additional post-translational modification (Sun et al., 2020), such as histone acetyltransferase (HAT) p300-mediated inhibitory acetylation and HDAC6-mediated deacetylation in SIK2 at Lys53 (K53) (Bricambert et al., 2010; Yang et al., 2013), and E3 ligase RNF2-mediated ubiquitination in SIK1 (Qu and Qu, 2017).
SIKs regulates physiological processes through direct phosphorylation of their substrates. The SIK phosphorylation sites have been defined through in vitro studies as a motif of LXB(S/T)XS*XXXL (B, basic amino acid; X, any amino acid) (Screaton et al., 2004). SIK phosphorylation leads to 14-3-3 binding and cytoplasmic retention of their substrates. Following PKA-mediated SIK inhibition, SIK substrates will become dephosphorylated and translocated to the nucleus to control the target gene program. The most well-characterized SIK substrates include the class IIa histone deacetylases (HDACs, HDAC4, 5, 7 and 9) and the CREB-regulated transcriptional coactivators (CRTCs, CRTC1, 2 and 3). Despite their name, the histone deacetylation activity of class IIa HDACs towards histones are relatively weak. This is due to the differences in amino acid composition in their active site as compared to the bona fide HDACs (Asfaha et al., 2019; Park and Kim, 2020). Instead, they can interact and remove the acetylation marks in a variety of non-histone proteins or transcriptional coregulators and hereby control the transcriptional activity of these targets. In addition, class IIa HDACs may associate with HDAC3 (a member of the class I HDACs that possesses bona fide histone deacetylation activity) and nuclear co-repressors and transcription factors NCoR/SMRT as an active complex that can drive epigenetic changes (Fischle et al., 2002). In their dephosphorylated states, CRTCs are translocated to the nucleus and interact with CREB via the basic leucine zipper (bZIP) domain to promote the CREB-dependent transcriptional gene program (Altarejos and Montminy, 2011). Mechanistically, the interaction with CRTCs increase the stability of CREB and binding to target gene promoters (Katoh et al., 2006). CRTCs also facilitate the recruitment of HAT p300 for CREB transcription activity (Altarejos and Montminy, 2011).
In response to external hormonal and neuronal factor stimulation, the second messenger cAMP signaling stimulates PKA-mediated phosphorylation and leads to SIK inhibition. This will release the cytosolic retention of SIK substrates and facilitate their nuclear translocation to control target gene program. Class IIa HDACs functions as a potent suppressor of the myocyte enhancer factor 2 (MEF2)-dependent gene program, which controls a variety of pathological and developmental processes, including cardiac hypertrophy (Zhang et al., 2002), muscle development and fiber-type formation (Edmondson et al., 1994; Handschin et al., 2003), myocyte survival and growth (Shen et al., 2006; Berdeaux et al., 2007; Stewart et al., 2012), craniofacial development (Verzi et al., 2007), and bone formation (Arnold et al., 2007; Collette et al., 2012). CRTCs functions as a coactivator to promote the CREB-dependent gene program, which involves in many metabolic pathways, such as the glucose uptake (Qi et al., 2009; Weems et al., 2012; Henriksson et al., 2015; Nixon et al., 2015), lipogenesis (Yoon et al., 2009; Bricambert et al., 2010; Park et al., 2014), gluconeogenesis (Patel et al., 2014; Itoh et al., 2015), steroidogenesis (Doi et al., 2002; Lee et al., 2016), and inflammatory cytokine production (Clark et al., 2012; MacKenzie et al., 2012). Furthermore, the transcription of Sik1 mRNA is regulated in a CREB-dependent manner (Jagannath et al., 2013), suggesting a negative feedback regulation between SIK1 and the CRTCs-CREB pathway.
Although HDACs and CRTCs are well-described SIK substrates, additional substrates have been identified. SIK1 can phosphorylate phosphatase methylesterase-1 (PME-1) of the protein phosphatase 2A (PP2A) complex to activate the activity of Na+/K+-ATPase (NKA) (Sjöström et al., 2007) (more details in next section). SIK1 functions downstream of TGFβ to negatively regulate type I receptor kinase signaling (Kowanetz et al., 2008; Lönn et al., 2012). Mechanistically, SIK1 forms a complex with SMAD7 (mothers against decapentaplegic homolog 7) and the E3 ubiquitin-protein ligase SMURF2 (SMAD-specific E3 ubiquitin-protein ligase 2) to promotes protein degradation of the activated type-I TGFβ receptor (TGFBR1 or ALK5) (Kowanetz et al., 2008; Lönn et al., 2012). SIK1 can phosphorylate the polarity protein PAR3 (Partitioning defective 3) to regulate tight junction assembly (Vanlandewijck et al., 2017). SIK1 can phosphorylate and activate the nucleotide release channel Pannexin 1 (PANX1) at Ser205, which involves the inflammatory responses of T cell to restrict the severity of allergic airway inflammation (Medina et al., 2021). In addition, SIK2 can form a complex with the cyclin-dependent kinase 5 (CDK5) regulatory subunit 1 (CDK5R1, also known as p35) and the E3 ligase PJA2 (praja ring finger ubiquitin ligase 2) to promotes insulin secretion (Sakamaki et al., 2014).
Acting as mediators of a broad array of neuronal and hormonal signaling pathways, SIKs play diverse roles in many physiological and pathological processes. Earlier studies and recent progress have demonstrated that they are critical regulators of the pathophysiological processes in cardiovascular and metabolic disease. This review will summarize our current understanding of the cardiovascular function of SIKs, with a focus on their pathophysiological roles in sodium handling, vascular remodeling, pulmonary arterial hypertension, cardiac hypertrophy and ischemia, inflammation, and fibrosis (Figure 2).
Sodium sensing and salt intake
The Na+/K+-ATPase (NKA) is a sodium/potassium-transporting ATPase wildly expressed on the outer plasma membrane. For each ATP consumed per transport cycle, NKA transports 3 sodium out of the cell and 2 potassium into the cell (Pirahanchi et al., 2024). Structurally, the NKA is composed of three subunits assembled in a 1:1:1 stoichiometry–the catalytic alpha (α)-subunit, the extracellular beta (β)-subunit, and the regulatory FXYD subunit (Nguyen et al., 2022). NKA activity helps to maintain the ion concentration gradient and cellular membrane potential, hereby the sodium and potassium gradients across the plasma membrane can be utilized by animal cells in various physiological processes for specialized functions (Jaitovich and Bertorello, 2010). As such, the range of the gradient demands requires NKA activity to be fine-tuned to coordinate different cellular needs (Jaitovich and Bertorello, 2010).
SIK1 involves in an intracellular sodium sensing network that controls NKA activity through a calcium-dependent process (Sjöström et al., 2007) (Figure 3). SIK1 was associated with NKA in the renal epithelium cells and participates in the regulation of NKA activity in response to sodium changes (Sjöström et al., 2007). Increases in intracellular sodium are coupled with calcium influx through the reversible Na+/Ca2+ exchanger (NCX), which is co-localized with NKA (Moore et al., 1993; Dostanic et al., 2004; Iwamoto et al., 2004). The sodium-induced calcium influx leads to the activation of SIK1 by CaMK-dependent phosphorylation at Thr322 (Sjöström et al., 2007). In its inactive form, the NKA catalytic α-subunit is constitutively associates with the protein phosphatase 2A (PP2A)/phosphatase methylesterase-1 (PME-1) complex (Sjöström et al., 2007). Activated SIK1 phosphorylates PME-1 possibly at Ser72 (Jaitovich and Bertorello, 2010), leading to its dissociation from the NKA/PP2A complex and allowing the dephosphorylation of NKA by PP2A to attain its catalytic activity (Sjöström et al., 2007). In addition, NKA activity can be regulated at the gene expression levels (Taub, 2018). The expression of ATP1B1 gene, encoding the NKA β1-subunit, is transcriptionally regulated by CRTCs (Taub, 2018). SIKs promote the cytoplasmic retention of CRTCs and hereby negatively regulates ATP1B1 expression (Taub et al., 2010). Extracellular stimulations by renal effectors such as norepinephrine, dopamine, and prostaglandins leads to the inhibition of SIK1 and nuclear translocation of CRTCs to promote ATP1B1 transcription via CREB (Taub et al., 2015). Furthermore, SIK1 also involves in sodium-independent hormonal regulation of NKA activity such as the dopamine and angiotensin pathways to control blood pressure homeostasis (Jaitovich and Bertorello, 2010).
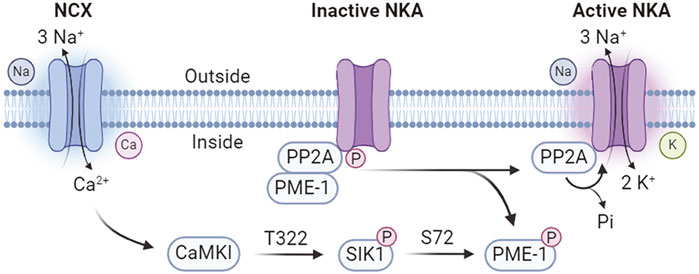
Figure 3. Calcium-dependent SIK1 regulation of NKA activity. NCX, Na+/Ca2+ exchanger; NKA, Na+/K+-ATPase; PP2A, protein phosphatase 2A; PME-1, phosphatase methylesterase-1; CaMKI, Ca2+/calmodulin dependent kinase I.
Genetic knockout mouse models have demonstrated the important roles of SIK1 in the pathological vascular adaptations to high-salt (HS) intake. HS intake induces Sik1 expression in the vascular smooth muscle cell (VSMCs) layer of the aorta (Bertorello et al., 2015). Under normal salt condition, Sik1 deletion does not affect blood pressure (BP) but increase collagen deposition in the aorta (Bertorello et al., 2015). On HS condition, Sik1 deletion leads to increased BP and vascular remodeling, as indicated by higher systolic BP, upregulation of TGFβ1 signaling, increased expression of endothelin-1 and genes related to VSMC contraction, and signs of cardiac hypertrophy (Bertorello et al., 2015). In in vitro cell models, Sik1 knockdown increases collagen in aortic adventitial fibroblasts and enhances the expression of endothelin-1 and contractile markers in VSMCs (Bertorello et al., 2015). These data suggested that vascular SIK1 prevents salt-induced hypertension by regulating vasoconstriction via downregulation of TGFβ1 signaling (Bertorello et al., 2015). Furter study showed that HS intake induced hypertension in Sik1−/− mice is associated with overactivity of the sympathetic nervous system (SNS), as indicated by increased levels of urinary L-3,4-dihydroxyphenylalanine (L-DOPA) and noradrenaline, and higher adrenal dopamine β-hydroxylase (DβH) activity (Pires et al., 2019). Preadministration of etamicastat, a peripheral reversible DβH inhibitor, prevents the HS-induced systolic BP increase, suggesting that SNS overactivity is a key mediator of salt-induced hypertension in Sik1−/− mice (Pires et al., 2019).
SIK1 and SIK2 play distinct roles in mediating the HS-induced high BP and cardiac hypertrophy in mouse models. Sik2 deletion does not affect the BP but prevents the development of left ventricular hypertrophy (LVH) on chronic HS intake (Popov et al., 2014). Instead, combined Sik1/2 deletion leads to higher BP but does not alter LVH on chronic HS intake, suggesting that SIK1 is required for maintaining normal BP while SIK2 is required for HS-induced cardiac hypertrophy independent of high BP (Pires et al., 2021). Further evidence also supports a pathological role for SIK2 in cardiac hypertrophy. For example, the hypertensive variant of α-adducin is associated with higher Sik2 expression and LVH in hypertensive Milan rats (Popov et al., 2014). In addition, the mRNA expression of SIK2, α-adducin, and genes related to cardiac hypertrophy are also positively correlated in human cardiac biopsies (Popov et al., 2014).
Vascular remodeling
The blood vessel is an autocrine-paracrine complex composed of VSMCs, endothelium cells, and fibroblasts (Gibbons and Dzau, 1994). In response to the hemodynamic alterations, the blood vessels undergo a structural remodeling processes involving in cell growth, death, migration, and production or degradation of the extracellular matrix (ECM) (Gibbons and Dzau, 1994). SIK1 plays an important role in maintaining vascular homeostasis. SIK1 is localized in human VSMCs and endothelial cells (Popov et al., 2011). Lower SIK1 activity leads to decreased NKA activity in VSMCs and is associated with increased vascular tone (Popov et al., 2011; Bertorello et al., 2015). A polymorphism in SIK1 gene encoding a Gly15 to Ser (G15S) missense mutation increases plasma membrane NKA activity in cultured VSMCs and is associated with lower blood pressure and reduced left ventricle (LV) mass in two separate Swedish and Japanese cohorts (Popov et al., 2011). In addition, SIK1 also involves in the negative feedback regulation of the TGFβ1 signaling and SIK1 deletion increases the expression of genes related to ECM remodeling (Bertorello et al., 2015). Furthermore, SIK1 regulates the expression of E-cadherin and contribute to LKB1-mediated regulation of cell polarity and intercellular junction stability (Eneling et al., 2012).
SIKs have been implicated in the pathological vascular remodeling process. Vascular calcification is a pathologic osteochondrogenic process of the blood vessels and is widely used as a clinical indicator of atherosclerosis (Abedin et al., 2004). SIKs promote vascular calcification by inducing cytoplasmic retention of HDAC4 (Abend et al., 2017). HDAC4 is induced in early calcification process, and it acts with the cytoplasmic adaptor protein ENIGMA (Pdlim7) to promote the VSMC calcification phenotypes (Abend et al., 2017). Pharmacologic SIK inhibition facilitates HDAC4 nuclear translocation and leads to the suppression of calcification process (Abend et al., 2017). Arterial restenosis is a pathological arterial narrowing process that results from a combination of elastic recoil, thrombosis, remodeling and intimal hyperplasia (Kenagy, 2011). SIK3 promotes arterial restenosis by stimulating VSMC proliferation and neointima formation (Cai et al., 2021). SIK3 is highly expressed in proliferating VSMCs and is also highly induced by growth medium in vitro and in neointimal lesions in vivo (Cai et al., 2021). Inactivation of SIKs attenuates the proliferation and migration of VSMCs, and reduced neointima formation and vascular inflammation in a femoral artery wire injury model (Cai et al., 2021).
Pulmonary arterial hypertension
Pulmonary arterial hypertension (PAH) is a high blood pressure condition in the arteries of lung. The pathogenesis of PAH involves in progressive narrowing of the arteries due to vasoconstriction, thrombosis, and vascular remodeling (Lan et al., 2018). Narrowing of the pulmonary arteries induces higher flow resistance and arterial pressure which cause right ventricle (RV) overload and ultimately lead to RV failure (António et al., 2022). Vascular remodeling is a hallmark feature in PAH and is characterized by excessive cell proliferation, abnormal release of inflammatory cytokines such as interleukin 1 (IL-1), IL-6, and tumor necrosis factor alpha (TNFα), and the upregulation of growth factors such as TGFβ (António et al., 2022). PAH is a multifactorial disease. The familiar form of PAH has been linked with mutations in genes of the TGFβ signaling pathway (Liu et al., 2011; Reynolds et al., 2011; Frydman et al., 2012) and mutations in ion channels such as KCNK3 (K+ channel subfamily K member 3) (Ma et al., 2013) and ATP1A2 (NKA α2-subunit) (Montani et al., 2013). As SIKs coordinate signaling pathways implicated in vasoconstriction, inflammation, and vascular remodeling, it has been proposed that the dysregulation of SIK pathways might underpin the pathophysiology of PAH (António et al., 2022).
Aberrant proliferation of the pulmonary artery endothelial cells (PAECs) plays an important role in the pathogenesis of PAH. It has been shown that the transcriptional factor MEF2 regulates expression of a number of transcriptional targets involved in pulmonary vascular homeostasis (Kim et al., 2014). The activity of MEF2 is significantly impaired in the PAECs derived from subjects with PAH due to the excess nuclear accumulation of HDAC4 and HDAC5 (Kim et al., 2014). Pharmacological inhibition by a selective class IIa HDACs inhibitor MC1568 restores MEF2 activity and transcriptional target expression, suppresses cell migration and proliferation, and rescues pulmonary hypertension in experimental mouse models (Kim et al., 2014). As SIKs are known to inhibit type IIa HDACs and able to restore MEF2-mediated transcription, it has been proposed that maneuvers that upregulate SIK activity could offer new opportunity to explore disease-modifying strategy for PAH (António et al., 2022).
Arterial remodeling in PAH also involves in the excessive proliferation and migration of pulmonary artery smooth muscle cells (PASMCs). A recent study showed that SIK1 deficiency stimulates PASMC proliferation via upregulation of the yes-associated protein (YAP) pathway and promotes vascular remodeling in PAH (Pu et al., 2022). The expression of SIK1, but not SIK2 or SIK3, is suppressed in the lung tissues of experimental PAH mice and in cultured human PASMCs under hypoxia (Pu et al., 2022). Pharmacological SIK inhibition or AAV9-mediated Sik1 knockdown in smooth muscles aggravates RV hypertrophy and pulmonary arterial remodeling in a hypoxia-induced PAH mouse model (Pu et al., 2022). Sik1 knockdown or inhibition promote the nuclear accumulation of YAP and stimulate proliferation and migration of human PASMCs under hypoxia condition (Pu et al., 2022). This study supported an anti-proliferative role for SIK1 in hypoxia-induced PAH remodeling and suggested that SIK1 might be a potential target for the treatment of PAH (Pu et al., 2022). However, the pathological role for SIK1 in alternative PAH models and in PAH patients is yet to be further explored.
Cardiac hypertrophy and ischemia
SIK1 was originally identifies as a myocardial SNF1-like kinase (msk) in a screen for novel protein kinases involving mouse heart development (Ruiz et al., 1994). Sik1 mRNA expression is restricted in the myocardial progenitor cells and marks the promotive ventricle and atrium in the developing heart (Ruiz et al., 1994), suggesting a possible role for SIK1 in embryonic myocardial cell differentiation and primitive heart formation (Ruiz et al., 1994). A later study further showed that Sik1 deletion in mouse embryonic stem cells postpones cardiomyocyte differentiation possibly by downregulation of the cyclin-dependent kinase inhibitor protein (Romito et al., 2010).
SIKs involve in high-salt (HS)-induced cardiac hypertrophy. HS intake is associated with cardiac hypertrophy both in humans (Du Cailar et al., 1992; Frohlich and Varagic, 2004) and animal models (Ferreira et al., 2010). A HS diet is associated with hallmark features of pathological cardiac remodeling, such as alterations in myocardial mechanical performance, dysregulation of the α/β-myosin heavy chain (MHC) expression, and alterations in calcium homeostasis and myocardial contractility (Patel and Joseph, 2020). Increases in intracellular sodium activates SIK1 and increases MEF2 transcriptional activity and cardiac hypertrophy signature gene expression in myocardial cells (Popov et al., 2012). The salt-induced activation of SIK1 and MEF2 is coupled by intracellular calcium influx through the NCX and activation of CaMKI (Popov et al., 2012). In addition, systemic hypertension also leads to a shift in the isoform distribution towards a decrease in the α/β-MHC ratio (Patel and Joseph, 2020). Cardiac hypertrophy of the spontaneous hypertensive rats is associated with decreased cardiac expression of SIK1 and SIK3 (Pinho et al., 2012). In contrast, SIK2 activation has been implicated in cardiac hypertrophy. SIK2 deletion prevents HS-induced cardiac hypertrophy independent of high BP (Pires et al., 2021). In hypertensive Milan rats, the hypertensive variant of α-adducin is associated with higher Sik2 expression and LVH (Popov et al., 2014). Furthermore, the mRNA expression of SIK2, α-adducin, and genes related to cardiac hypertrophy are positively correlated in human cardiac biopsies (Popov et al., 2014).
SIKs have been implicated in pressure overload-induced hypertrophic cardiac remodeling. In adult mice, Sik1 deletion protects against hypertrophic cardiac remodeling and heart failure following pressure overload via transverse aortic constriction (Hsu et al., 2020). Mechanistically, SIK1 phosphorylates and stabilizes HDAC7 to increase the expression of c-Myc, which in turn promotes the pro-hypertrophic gene program (Hsu et al., 2020) (Figure 4). In contrast, other class IIa HDACs, including HDAC4, HDAC5, and HDAC9, are regulated by stress-induced CaMKII and protein kinase D (PKD) phosphorylation for nuclear export in cardiomyocytes, which will de-suppress MEF2 to stimulate pro-hypertrophic gene expression (Zhang et al., 2002; Chang et al., 2004; Lehmann et al., 2017; Travers et al., 2020) (Figure 4). This suggests the functional divergence of HDAC7 from the other class IIa HDACs in cardiomyocyte remodeling (Hsu et al., 2020; Travers et al., 2020). A recent study further reported that the SIK1 expression is potentially regulated by the master circadian rhythm factor BMAL1 and Sik1 knockdown in neonatal rat ventricular myocytes (NRVMs) reduces myocyte size and hypertrophic gene expression in response to phenylephrine stimulation (Arrieta et al., 2023). Unlike the pro-hypertrophic role of SIK1, SIK3 functions as a suppressor of cardiac hypertrophy (Hsu, 2022). Loss of SIK3 induces hypertrophic phenotypes in cultured NRVMs and in adult mouse hearts (Hsu, 2022). Mechanically, SIK3 deletion reduces ARHGAP21 (Rho GTPase activating protein 21) protein and promotes the nuclear translocation of MRTF-A (myocardin related transcription factor A) to stimulate hypertrophic gene program (Hsu, 2022).
SIKs also involve in the pathological cardiac remodeling following myocardial infarction (MI). A recent study reported that intramyocardial transplantation of embryonic cardiopulmonary progenitors can target the SIK1-CREB1 axis via exosomal transfer of miR-27b-3p to facilitate cardiac repair and improve cardiac function in a mouse model of MI (Xiao et al., 2024). Ischemia-reperfusion (IR) injury induces the expression of SIK2 in myocardial tissues (Liu et al., 2022). Overexpression of SIK2 in NRVMs increases AKT phosphorylation but suppresses the activity of YAP, one of the downstream effectors of Hippo pathway (Facchi et al., 2022). However, SIK2 overexpression does not impact NRVM proliferation or survival, but leads to an increase in cardiomyocyte hypertrophy (Facchi et al., 2022). Sik2 deletion suppresses hypertrophic response and reduces infarct area and cardiac fibrosis in MI induced by coronary artery ligation, suggesting that SIK2 deficiency protects against cardiac ischemia (Facchi et al., 2022). However, the detailed mechanisms by which SIK2 promotes the hypertrophic gene program is yet to be revealed.
Inflammation
Inflammation is a coordinated immune response of the body to defense against injuries, infection, and stress (Pahwa et al., 2024). It is an essential pathological component closely associated with cardiovascular disease. For example, atherogenesis is a process associated with endothelial cell dysfunction that leads to atherosclerotic plaque formation and coronary arterial heart disease (Alfaddagh et al., 2020). It is considered that these pathological processes are driven by the cytokines, interleukins (ILs), and cellular constituents of the inflammatory response (Alfaddagh et al., 2020). Pathological cardiac remodeling is a process involving cardiac myocyte growth and death, vascular rarefaction, fibrosis, inflammation, and electrophysiological remodeling (Burchfield et al., 2013). There are growing evidences showing that elevated inflammatory biomarkers, implicating innate immune cells such as macrophages, are associated with worsened clinical outcomes in heart failures (HF) (Kalogeropoulos et al., 2010; Chen and Frangogiannis, 2016; Hage et al., 2017; DeBerge et al., 2019).
SIKs regulate the production of inflammatory cytokines, such as TNFα and ILs, to coordinate the innate immune responses (Figure 5). The endogenous SIK activity suppresses the anti-inflammatory signaling in macrophages. Pharmacological SIK inhibition increases secretion of anti-inflammatory cytokine IL-10 but suppresses proinflammatory cytokines TNFα, IL-6, and IL-12 in macrophage after toll-like receptor (TLR) stimulation by lipopolysaccharide (LPS) (Clark et al., 2012). The anti-inflammatory IL-10 expression depends on the CRTC3-CREB transcription activity (Clark et al., 2012), while the proinflammatory cytokine expression depends on HDAC4-mediated regulation of the nuclear factor-κB (NF-κB) pathway (Luan et al., 2014). SIKs mediate the prostaglandin E2 (PGE2) signaling to promote IL-10 production and macrophage polarization (MacKenzie et al., 2012). Combined stimulation with PGE2 and LPS leads to IL-10 expression and an anti-inflammatory phenotype in macrophages (Darling et al., 2016). Similarly, PGE2 stimulates IL-10 mRNA expression through CRTC3-CREB-mediated transcription and either genetic or pharmacological SIK inhibition mimics the effect of PGE2 on IL-10 production (Darling et al., 2016). Characterization of the primary macrophages carrying a catalytically inactive mutation in individual SIK gene further revealed that all three SIK isoforms contribute to macrophage polarization, with a major role for SIK2 and SIK3 (Darling et al., 2016). However, conflicting results have shown that SIKs act to suppress the pro-inflammatory signaling in Raw 264.7 macrophage cells. Overexpression of SIK1 and SIK3, but not SIK2, inhibits LPS-induced NF-κB activity and impacts proinflammatory cytokine expression (Kim et al., 2013). Knockdown of SIK1 and SIK3 in Raw 264.7 cells leads to activation of NF-κB pathway (Kim et al., 2013). Another study reported that SIK3 deficiency exacerbates LPS-induced endotoxicity and is associated with higher expression of proinflammatory cytokines (Sanosaka et al., 2015). Thus, it remains to be understood for the isoform specific function of endogenous SIKs in regulating the proinflammatory cytokine expression (Jagannath et al., 2023).
In addition to their immunoregulatory roles in macrophages, SIKs also involve in zymosan-stimulated cytokine production in mouse bone marrow-derived dendritic cells (Sundberg et al., 2014), LPS or IL-1β induced cytokine modulation in human myeloid cells (Lombardi et al., 2016), and IL-33-stumulated cytokine secretion in mast cells (Darling et al., 2021). Furthermore, SIKs also modulate adaptive immunity by regulating T cell lineage commitment, differentiation, and survival via a MEF2C-dependent mechanism (Nefla et al., 2021; Canté-Barrett et al., 2022).
Fibrosis
Fibrosis is a pathological process resulted from tissue injury or chronic inflammation (Henderson et al., 2020). It is characterized by the activation of fibroblasts and access accumulation of ECM proteins such as collagen and glycosaminoglycans (Henderson et al., 2020). Fibrosis is a hallmark feature of many cardiovascular disease. In pathological cardiac remodeling, myocardial fibrosis occurs as deposition of ECM proteins by activated fibroblast, which results in the expansion of cardiac interstitium and ultimately leads to systolic and diastolic dysfunction (Frangogiannis, 2021). Fibrogenic growth factors such as TGFβ, cytokines including TNF-α, IL-1, IL-6, IL-10, and IL-4, and neurohormonal pathways play essential roles in the pathogenesis of fibrosis (Frangogiannis, 2021).
SIK1 involves in a negative feedback regulation of the TGFβ signaling (Figure 6). TGFβ signals through type I and type II receptor serine/threonine kinases and intracellular Smad proteins to ultimately regulate the transcriptional program (Kowanetz et al., 2008). SIK1 is an inducible target of TGFβ pathway and acts to negatively regulate type I receptor kinase signaling (Kowanetz et al., 2008). Mechanistically, SIK1 forms a complex with Smad7 to downregulate the activated type I receptor TGFBR1 (ALK5) by increasing ubiquitination dependent receptor degradation (Kowanetz et al., 2008). Further study showed that TGFβ can direct regulate the transcription of Sik1, which involves in the binding of SMAD protein to the promoter of Sik1 (Lönn et al., 2012). SIK1 forms a complex with the ubiquitin ligase Smurf2 to regulate type I receptor turnover (Lönn et al., 2012). Sik1 deletion in mice induces collagen deposition and expression of genes related to ECM remodeling in the aorta (Bertorello et al., 2015). Furthermore, SIKs also regulate the TGFβ-targeted transcriptional gene program. Small molecule SIK inhibition or genetic SIK inactivation attenuates TGFβ-mediated transcription of PAI-1 (plasminogen activator inhibitor-1) and enhances cell apoptosis response (Hutchinson et al., 2020).
Pharmacological SIK inhibitors have demonstrated anti-fibrosis effects in mouse models. Earlier studies showed that dasatinib attenuates pulmonary fibrosis in bleomycin-exposed mice (Yilmaz et al., 2015) and in acute experimental silicosis (Cruz et al., 2016). However, whether the anti-fibrosis of dasatinib depends on SIKs is unclear. A recently study showed that SIK2 expression and activity is increased in fibrotic lung tissues and activated fibroblasts (Zou et al., 2022). A selective SIK2 inhibitor ARN-3236 restricts fibroblasts differentiation and ECM gene expression in human fetal lung fibroblasts and attenuates bleomycin-induced pulmonary fibrosis in mice (Zou et al., 2022), supporting as pathological role for SIK2 in pulmonary fibrosis.
Metabolic syndrome
As major risk factors for cardiovascular disease, diabetes, obesity, and metabolic syndrome negatively impact cardiovascular function (Strain and Paldánius, 2018; Powell-Wiley et al., 2021). The sodium/glucose cotransporter 2 (SGLT2) inhibitor empagliflozin and glucagon-like peptide-1 receptor (GLP1R) agonist semaglutide, medications originally developed to treat diabetes and/or obesity, have demonstrated clinically significant cardiovascular benefits (Cowie and Fisher, 2020; Sattar et al., 2021; Lincoff et al., 2023). It has been increasingly appreciated that targeting diabetes, obesity, and related metabolic dysfunction could be a disease modifying strategy for cardiovascular disease. SIKs are broadly expressed in the metabolic relevant tissues and play essential roles in mediating insulin action, maintaining glucose hemostasis, and controlling lipid metabolism (Figure 7). It has been appreciated that dysregulation of SIK function could underpin the pathophysiology of insulin resistance, dyslipidemia, and metabolic syndrome. In addition, SIKs are also expressed in the central nervous system and play essential roles in the regulation of circadian rhythm, sleep needs, and many other neurophysiological functions (Jagannath et al., 2023). Dysregulation of these neurophysiological processes could also have major impacts on systemic metabolic homeostasis and potentially contributes the cardiometabolic disease (Chellappa et al., 2019; Fagiani et al., 2022).
SIKs are critical regulators of hepatic gluconeogenesis. After feeding, insulin-stimulated FOXO (forkhead box O) phosphorylation by AKT leads to 14-3-3 binding and cytoplasmic restriction of FOXO, and thus inhibits the gluconeogenesis gene program (Wein et al., 2018). Under fasting condition, glucagon induces the PKA-mediated phosphorylation of CREB and recruitment of the p300 HAT and CREB binding protein (p300/CBP) to activate the gluconeogenesis gene expression (Wein et al., 2018). Glucagon action leads to PKA-mediated phosphorylation and inactivation of SIKs, which results in the dephosphorylation and nuclear translocation of CRTCs and class IIa HDACs. CRTCs bind to CREB and act as coactivator to stimulate the gluconeogenesis gene expression. Class IIa HDACs can recruit HDAC3 to the target gene promoters to regulate the acetylation of FOXO, which further activate the transcription of gluconeogenesis genes (Wein et al., 2018). In mouse models, liver specific ablation of LKB1, the upstream activating kinase for SIKs, leads to increased glucose production in hepatocytes. Pharmacological pan-SIK inhibition phenocopies LKB1 deficient hepatocytes, including the dephosphorylations in CRTC2/3 and HDAC4/5 and increased gluconeogenesis gene expression and glucose production (Foretz et al., 2010; Patel et al., 2014). However, deleting either Sik1 or Sik2 alone in the liver does not impact hepatic gluconeogenesis (Patel et al., 2014; Nixon et al., 2015), suggesting SIKs play redundant roles in hepatic gluconeogenesis.
SIKs also control hepatic lipogenesis. Hepatic lipogenesis gene expression is controlled by master transcription factors, including SREBP-1c (sterol regulatory element-binding protein 1c) and ChREBP (carbohydrate response element binding protein) (Wang et al., 2015). Hepatic Sik1 knockdown increases lipogenic gene expression (Yoon et al., 2009). SIK1 suppresses hepatic lipogenesis gene expression by inhibitory phosphorylation of SREBP-1c at Ser329 (Yoon et al., 2009). Sik2 knockdown in the liver also increases hepatic lipogenesis gene expression and steatosis (Bricambert et al., 2010). Mechanistically, SIK2 suppresses p300 HAT activity by inhibitory phosphorylation at Ser89, which in turn leads to decreased acetylation in ChREBP and reduced transcriptional activity of lipogenesis genes (Bricambert et al., 2010). Global Sik3 deletion leads to neonatal death and skeletal malformations in survived adults (Uebi et al., 2012). The survived adult Sik3 knockout mice show lipodystrophy phenotypes with altered cholesterol and bile acid metabolism in the liver (Uebi et al., 2012), indicating the involvement of SIK3 in lipid metabolism.
SIKs control a broad array of metabolic pathways in the adipose tissue. Among all the three SIK isoforms, SIK2 is the most abundant both in rodent and human adipocytes (Horike et al., 2003; Säll et al., 2016). SIK2 expression is robustly induced during adipocyte differentiation and its deletion leads to increased adipocyte differentiation in cell culture (Horike et al., 2003; Du et al., 2008; Gormand et al., 2014; Park et al., 2014). SIK2 can directly phosphorylates IRS-1 (insulin receptor substrate 1) in vitro and in cultured adipocytes, suggesting its involvement in insulin signaling (Horike et al., 2003). Insulin-stimulated AKT phosphorylation is reduced in the adipose tissue of global Sik2 knockout mice (Park et al., 2014) and in human adipocytes treated with pan-SIK inhibitor (Säll et al., 2016). SIK2 activity is increased in the adipose tissues from diabetic db/db mice (Du et al., 2008). However, SIK2 mRNA expression and activity is downregulated in the adipose tissue of obese individuals (Säll et al., 2016). Global Sik2 knockout mice show impaired glucose and insulin tolerance, hypertriglyceridemia, and adipose tissue dysfunction, such as increased lipolysis and macrophage infiltration and decreased GLUT4 and adiponectin expression (Park et al., 2014). The downstream molecular targets of SIK2 include HDAC4, CRTC2, and CRTC3 in adipocytes and support a role for SIK2 in regulating GLUT4 expression and adipocyte glucose uptake (Park et al., 2014; Henriksson et al., 2015). Lipogenesis gene expression is increased in the adipose tissue of Sik2 knockout mice (Park et al., 2014). It has been shown that SIK2 inhibits the expression of lipogenic genes potentially by regulating SREBP1c in adipocytes (Du et al., 2008).
SIKs play redundant roles as suppressors of the adipocyte thermogenic program. The adipocyte thermogenic program is orchestrated by the β-adrenergic receptor signaling cascade to drive the transcriptional machinery for thermogenic gene expression (Shi and Collins, 2017; 2023). Combined deletion of Sik1 and Sik2, but not deletion of Sik1 or Sik2 alone, increases the expression of thermogenic gene uncoupling protein 1 (Ucp1) in subcutaneous inguinal while adipose tissue (iWAT) (Wang et al., 2017). Knockdown of either Sik1, Sik2, or Sik3 increases Ucp1 expression in mouse brown adipocytes (Shi et al., 2023). In addition, treatment with a pan-SIK inhibitor YKL-05-099 in mice stimulates the thermogenic gene expression in iWAT (Shi et al., 2023). Furthermore, adipose tissue specific ablation of LKB1, the upstream kinase for SIK activation, promotes brown fat expansion and WAT browning (Shan et al., 2016; Wang et al., 2017). The adipose browning phenotypes of LKB1 adipose knockout mice are mediated by the action of SIK substrates CRTC3 and HDAC4 (Shan et al., 2016; Wang et al., 2017).
SIK1 involves in the CREB-dependent myogenic gene program. SIK1 is induced by the CREB transcription activity in skeletal myocytes and it promotes MEF2-dependent myogenic program by suppressing the class IIa HDAC activity (Berdeaux et al., 2007). SIK1 protein abundance is also dynamically controlled in response to cAMP stimulation during myogenesis (Stewart et al., 2012). Sik1 depletion in myogenic precursors profoundly impairs MEF2 protein accumulation and myogenesis (Stewart et al., 2012). Overexpression of SIK1 reduces the muscle dystrophy caused by a dominant negative A-CREB mutation (Berdeaux et al., 2007). In adult mice, skeletal muscle Sik1 expression can be induced by exercise training (Bruno et al., 2014), acute muscle injury (Stewart et al., 2011), and obesity (Horike et al., 2003; Nixon et al., 2015). Interestingly, global Sik1 knockout mice are protected against high-fat diet induced insulin resistance (Nixon et al., 2015). Sik1 deletion in skeletal muscle, but not liver or adipose tissue phenocopies the whole-body knockout mice, suggesting skeletal muscle Sik1 contribute to diet-induced insulin resistance through a tissue autonomous mechanism (Nixon et al., 2015).
SIKs involve in the regulation of insulin secretion in pancreatic β-cells. Sik1 deletion enhances glucose-stimulated insulin secretion in β-cells (Kim et al., 2015). Mechanistically, Sik1 directly phosphorylates phosphodiesterase 4D (PDE4D) at Ser136/Ser141, which leads to reduction in cellular cAMP and attenuation of insulin secretion (Kim et al., 2015). In contrast, β-cell specific Sik2 deletion reduces glucose-stimulated insulin secretion (Sakamaki et al., 2014). SIK2 phosphorylates CDK5R1 (p35) to induce its ubiquitination and proteasomal degradation, which leads to the suppression of CDK5 activity and activation of the voltage-dependent Ca2+ channels (VDCC) (Sakamaki et al., 2014). VDCC activation in turn induces Ca2+ influx and potentiates exocytosis and insulin vesicle release (Sakamaki et al., 2014). It was also proposed that SIK2 is involved in the β-cell compensation during hyperglycemia and loss of SIK2 contributes to β-cell failure and diabetes (Sakamaki et al., 2014).
Development of SIK inhibitors
Small molecule SIK inhibitors (SIKi) have been developed as tools to probe the function of SIKs and have also shown therapeutic potential in various disease models, including osteoporosis, leukemia, inflammatory and fibrotic disease, and certain types of cancer (Wein et al., 2018; Sun et al., 2020; Darling and Cohen, 2021; António et al., 2022; Jagannath et al., 2023). HG-9-91-01 is the first widely used pan-SIKi for in vitro models as it can be rapidly degraded by mouse liver microsomes (Sundberg et al., 2016). HG-9-91-01 targets a hydrophobic pocket created by the presence of a small amino acid residue at the gatekeeper site in the SIK kinase domain (Clark et al., 2012). Mutation of threonine residue within the gatekeeper sites impairs the accessibility of inhibitor and confers resistance to HG-9-91-01 inhibition (Clark et al., 2012). Using HG-9-91-01 as a starting point, YKL-05-099 was development and it shows increased selectivity for SIKs and enhanced pharmacokinetic properties (Sundberg et al., 2016). A well-tolerated dose of YKL-05-099 in vivo achieves free serum concentrations above its IC50 for SIK2 inhibition for >16 h, making it the first pan-SIKi widely used for in vivo studies (Sundberg et al., 2016). YKL-05-099 has been shown to modulate immunoregulatory cytokine production (Sundberg et al., 2016), suppress acute myeloid leukemia progression (Tarumoto et al., 2020), and increase bone formation (Wein et al., 2016; Tang et al., 2021). Our group showed that YKL-05-099 stimulates adipocyte thermogenic gene expression and promotes adipose tissue browning in vivo (Shi et al., 2023), suggesting a role for SIKs in regulating energy hemostasis and their potential as therapeutic targets of obesity.
The three SIK isoforms are broadly expressed. Each SIK isoform could have both unique and redundant functions in a variety of physiological processes (Wein et al., 2018; Sun et al., 2020; Darling and Cohen, 2021; António et al., 2022; Jagannath et al., 2023). As such, both pan and isoform specific SIKi are desired to better approach these targets in specific disease contexts. Newer SIKi with better potency, specificity and oral bioavailability have been developed. GLPG3312 is a pan-SIKi developed by Galapagos NV that demonstrated both anti-inflammatory and immunoregulatory activities in vitro in human primary myeloid cells and in vivo in mouse models (Temal-Laib et al., 2023). JRD-SIK1/2i-4 is a selective SIK1/2 inhibitor that modulates innate immune activation and suppresses intestinal inflammation (Babbe et al., 2024). SK-124 is an orally available SIK2/3 inhibitor that stimulates bone formation without evidence of short-term toxicity in mice (Sato et al., 2022). GLPG3970 is a SIK2/3 inhibitor developed by Galapagos NV for the treatment of auto-immune and inflammatory diseases (Peixoto et al., 2024). Selective SIK2 inhibitors have been developed based on the binding pose of GLPG-3970 by the application of AlphaFold structures and generative models (Zhu et al., 2023).
ARN-3236 is an orally active and selective SIK2 inhibitor (Lombardi et al., 2016; Zhou et al., 2017). ARN-3236 induces an anti-inflammatory phenotype in human myeloid cells by modulating cytokine production after TLR agonist stimulation (Lombardi et al., 2016). ARN-3236 has also been shown to inhibit ovarian cancer growth and enhancing paclitaxel chemosensitivity in preclinical models (Zhou et al., 2017). Pterosin B is an indanone found in bracken fern (pteridium aquilinum) and a selective SIK3 inhibitor (Itoh et al., 2015; Yahara et al., 2016). It has been shown that pterosin B stimulates hepatic glucose production (Itoh et al., 2015) and prevents chondrocyte hypertrophy and osteoarthritis in mice (Yahara et al., 2016). However, our knowledge on the in vivo effects of pterosin B is very limited. More recently, OMX-0407 was reported as an orally available, single-digit nanomolar inhibitor of SIK3 (Hartl et al., 2022). OMX-0407 suppresses intratumoral NF-κB activity and inhibits tumor cell growth in vivo (Hartl et al., 2022). Inhibition of SIK1 activity is considered undesirable due to its essential role in blood pressure control and vascular remodeling (Bertorello et al., 2015). However, selective SIK1 inhibition might be beneficial in certain disease conditions (Kim et al., 2015; Hsu et al., 2020). Instead, phanginin A, a diterpenoid from the seeds of Caesalpinia sappan Linn (Yodsaoue et al., 2008), has been reported as a SIK1 activator to inhibit hepatic gluconeogenesis by increasing PDE4 activity and suppressing the cAMP signaling pathway (Liu et al., 2020).
Conclusion remarks
Studies of genetic mouse models have demonstrated that SIKs are important regulators in the cardiovascular system and metabolic pathways (summarized in Table 1). It has been appreciated that SIKs could have both redundant and isoform-distinct functions in these pathophysiological processes. As such, the in vivo studies of genetic mouse models with SIK deletions might be challenging as efforts have to be made to delineate the redundant and distinct roles of each SIK protein in each tissue. These studies could be further complicated by the additional efforts required to identify the specific SIK substrate and their mechanisms of regulation in each physiological context. It has also been proposed that dysregulation of SIKs is associated with cardiometabolic disease. As mentioned above, a polymorphism in SIK1 gene encoding a G15S missense mutation is associated with lower blood pressure and reduced cardiac hypertrophy in humans (Popov et al., 2011; Bertorello et al., 2015). The expression and activity of SIK2 and SIK3 are downregulated in the adipose tissue of people with obesity and diabetes (Säll et al., 2016). Genetic variants in SIK genes also have been linked with alterations in blood lipid panels, suggesting the potential clinical relevance of SIK genes for dyslipidemia (Chasman et al., 2008; Teslovich et al., 2010; Braun et al., 2012; Willer and Mohlke, 2012; Ko et al., 2014; Karjalainen et al., 2020). For the translational development of SIKi, their cardiovascular and metabolic effects need to be considered and carefully evaluated. Understanding the cardiometabolic function of SIKs would not only offer new knowledge but also provide opportunity to better approach these targets and pathways for intervention and treatment of disease in the future.
Author contributions
FS: Conceptualization, Funding acquisition, Visualization, Writing–original draft, Writing–review and editing.
Funding
The author(s) declare that financial support was received for the research, authorship, and/or publication of this article. FS is supported by the American Heart Association Career Development Award 23CDA1048341.
Acknowledgments
The figures were created with BioRender.com.
Conflict of interest
The author declares that the research was conducted in the absence of any commercial or financial relationships that could be construed as a potential conflict of interest.
Publisher’s note
All claims expressed in this article are solely those of the authors and do not necessarily represent those of their affiliated organizations, or those of the publisher, the editors and the reviewers. Any product that may be evaluated in this article, or claim that may be made by its manufacturer, is not guaranteed or endorsed by the publisher.
Abbreviations
SIKs, salt-inducible kinases; SNF1, sucrose-nonfermenting 1; HDACs, histone deacetylases; CREB, cAMP responsive element binding protein; CRTCs, CREB-regulated transcriptional coactivators; MEF2, myocyte enhancer factor 2; LKB1, liver kinase B1; PKA, protein kinase A; AMPK, adenosine monophosphate-activated protein kinase; CaMK, Ca2+/calmodulin dependent kinase; PKD, protein kinase D; TGFβ, transforming growth factor β; PI3K, phosphoinositide 3-kinase; NKA, Na+/K+-ATPase; NCX, Na+/Ca2+ exchanger, VDCC, voltage-dependent Ca2+ channels; HAT, histone acetyltransferase; MI, myocardial infarction; PAH, pulmonary arterial hypertension; ECM, extracellular matrix; PAECs, pulmonary artery endothelial cells; PASMCs, pulmonary artery smooth muscle cells; SREBP-1c, sterol regulatory element-binding protein 1c; ChREBP, carbohydrate response element binding protein; MHC, myosin heavy chain; NF-κB, nuclear factor-κB; PME-1, phosphatase methylesterase-1, NRVMs, neonatal rat ventricular myocytes.
References
Abedin M., Tintut Y., Demer L. L. (2004). Vascular calcification: mechanisms and clinical ramifications. Arterioscler. Thromb. Vasc. Biol. 24 (7), 1161–1170. doi:10.1161/01.Atv.0000133194.94939.42
Abend A., Shkedi O., Fertouk M., Caspi L. H., Kehat I. (2017). Salt-inducible kinase induces cytoplasmic histone deacetylase 4 to promote vascular calcification. EMBO Rep. 18 (7), 1166–1185. doi:10.15252/embr.201643686
Alfaddagh A., Martin S. S., Leucker T. M., Michos E. D., Blaha M. J., Lowenstein C. J., et al. (2020). Inflammation and cardiovascular disease: from mechanisms to therapeutics. Am. J. Prev. Cardiol. 4, 100130. doi:10.1016/j.ajpc.2020.100130
Al-Hakim A., Göransson O., Deak M., Toth R., Campbell D. G., Morrice N. A., et al. (2005). 14-3-3 cooperates with LKB1 to regulate the activity and localization of QSK and SIK. J. Cell Sci. 118 (23), 5661–5673. doi:10.1242/jcs.02670
Altarejos J. Y., Montminy M. (2011). CREB and the CRTC co-activators: sensors for hormonal and metabolic signals. Nat. Rev. Mol. Cell Biol. 12 (3), 141–151. doi:10.1038/nrm3072
António T., Soares-da-Silva P., Pires N. M., Gomes P. (2022). Salt-inducible kinases: new players in pulmonary arterial hypertension? Trends Pharmacol. Sci. 43 (10), 806–819. doi:10.1016/j.tips.2022.06.008
Arnold M., Kim Y., Czubryt M. P., Phan D., McAnally J., Qi X., et al. (2007). MEF2C transcription factor controls chondrocyte hypertrophy and bone development. Dev. Cell 12 (3), 377–389. doi:10.1016/j.devcel.2007.02.004
Arrieta A., Chapski D., Reese A., Rosa-Garrido M., Vondriska T. M. (2023). Abstract P1133: bmal1 drives postnatal cardiac hypertrophy via circadian chromatin remodeling of the pro-hypertrophic gene Sik1. Circulation Res. 133 (1), AP1133. doi:10.1161/res.133.suppl_1.P1133
Asfaha Y., Schrenk C., Alves Avelar L. A., Hamacher A., Pflieger M., Kassack M. U., et al. (2019). Recent advances in class IIa histone deacetylases research. Bioorg Med. Chem. 27 (22), 115087. doi:10.1016/j.bmc.2019.115087
Babbe H., Sundberg T. B., Tichenor M., Seierstad M., Bacani G., Berstler J., et al. (2024). Identification of highly selective SIK1/2 inhibitors that modulate innate immune activation and suppress intestinal inflammation. Proc. Natl. Acad. Sci. U. S. A. 121 (1), e2307086120. doi:10.1073/pnas.2307086120
Berdeaux R., Goebel N., Banaszynski L. A., Takemori H., Wandless T. J., Shelton G. D., et al. (2007). SIK1 is a class II HDAC kinase that promotes survival of skeletal myocytes. Nat. Med. 13 (5), 597–603. doi:10.1038/nm1573
Bertorello A. M., Pires N., Igreja B., Pinho M. J., Vorkapic E., Wågsäter D., et al. (2015). Increased arterial blood pressure and vascular remodeling in mice lacking salt-inducible kinase 1 (SIK1). Circ. Res. 116 (4), 642–652. doi:10.1161/circresaha.116.304529
Bertorello A. M., Zhu J.-K. (2009). SIK1/SOS2 networks: decoding sodium signals via calcium-responsive protein kinase pathways. Pflugers Archiv Eur. J. physiology 458 (3), 613–619. doi:10.1007/s00424-009-0646-2
Braun T. R., Been L. F., Singhal A., Worsham J., Ralhan S., Wander G. S., et al. (2012). A replication study of GWAS-derived lipid genes in Asian Indians: the chromosomal region 11q23.3 harbors loci contributing to triglycerides. PloS one 7 (5), e37056–NA. doi:10.1371/journal.pone.0037056
Bricambert J., Miranda J., Benhamed F., Girard J., Postic C., Dentin R. (2010). Salt-inducible kinase 2 links transcriptional coactivator p300 phosphorylation to the prevention of ChREBP-dependent hepatic steatosis in mice. J. Clin. investigation 120 (12), 4316–4331. doi:10.1172/jci41624
Bruno N. E., Kelly K. A., Hawkins R., Bramah-Lawani M., Amelio A. L., Nwachukwu J. C., et al. (2014). Creb coactivators direct anabolic responses and enhance performance of skeletal muscle. Embo J. 33 (9), 1027–1043. doi:10.1002/embj.201386145
Burchfield J. S., Xie M., Hill J. A. (2013). Pathological ventricular remodeling: mechanisms: part 1 of 2. Circulation 128 (4), 388–400. doi:10.1161/circulationaha.113.001878
Cai Y., Wang X. L., Lu J., Lin X., Dong J., Guzman R. J. (2021). Salt-inducible kinase 3 promotes vascular smooth muscle cell proliferation and arterial restenosis by regulating AKT and PKA-CREB signaling. Arterioscler. Thromb. Vasc. Biol. 41 (9), 2431–2451. doi:10.1161/atvbaha.121.316219
Canté-Barrett K., Meijer M. T., Cordo V., Hagelaar R., Yang W., Yu J., et al. (2022). MEF2C opposes Notch in lymphoid lineage decision and drives leukemia in the thymus. JCI insight 7 (13), e150363–NA. doi:10.1172/jci.insight.150363
Chang S., McKinsey T. A., Zhang C. L., Richardson J. A., Hill J. A., Olson E. N. (2004). Histone deacetylases 5 and 9 govern responsiveness of the heart to a subset of stress signals and play redundant roles in heart development. Mol. Cell. Biol. 24 (19), 8467–8476. doi:10.1128/mcb.24.19.8467-8476.2004
Chasman D. I., Paré G., Zee R. Y., Parker A. N., Cook N. R., Buring J. E., et al. (2008). Genetic loci associated with plasma concentration of low-density lipoprotein cholesterol, high-density lipoprotein cholesterol, triglycerides, apolipoprotein A1, and Apolipoprotein B among 6382 white women in genome-wide analysis with replication. Circ. Cardiovasc Genet. 1 (1), 21–30. doi:10.1161/circgenetics.108.773168
Chellappa S. L., Vujovic N., Williams J. S., Scheer F. (2019). Impact of circadian disruption on cardiovascular function and disease. Trends Endocrinol. Metab. 30 (10), 767–779. doi:10.1016/j.tem.2019.07.008
Chen B., Frangogiannis N. G. (2016). Macrophages in the remodeling failing heart. Circ. Res. 119 (7), 776–778. doi:10.1161/circresaha.116.309624
Clark K., MacKenzie K. F., Petkevicius K., Kristariyanto Y. A., Zhang J., Choi H. G., et al. (2012). Phosphorylation of CRTC3 by the salt-inducible kinases controls the interconversion of classically activated and regulatory macrophages. Proc. Natl. Acad. Sci. U. S. A. 109 (42), 16986–16991. doi:10.1073/pnas.1215450109
Collette N. M., Genetos D. C., Economides A. N., Xie L., Shahnazari M., Yao W., et al. (2012). Targeted deletion of Sost distal enhancer increases bone formation and bone mass. Proc. Natl. Acad. Sci. U. S. A. 109 (35), 14092–14097. doi:10.1073/pnas.1207188109
Cowie M. R., Fisher M. (2020). SGLT2 inhibitors: mechanisms of cardiovascular benefit beyond glycaemic control. Nat. Rev. Cardiol. 17 (12), 761–772. doi:10.1038/s41569-020-0406-8
Cruz F. F., Horta L. F., Maia Lde A., Lopes-Pacheco M., da Silva A. B., Morales M. M., et al. (2016). Dasatinib reduces lung inflammation and fibrosis in acute experimental silicosis. PLoS One 11 (1), e0147005. doi:10.1371/journal.pone.0147005
Darling N. J., Arthur J. S. C., Cohen P. (2021). Salt-inducible kinases are required for the IL-33-dependent secretion of cytokines and chemokines in mast cells. J. Biol. Chem. 296 (NA), 100428. doi:10.1016/j.jbc.2021.100428
Darling N. J., Cohen P. (2021). Nuts and bolts of the salt-inducible kinases (SIKs). Biochem. J. 478 (7), 1377–1397. doi:10.1042/bcj20200502
Darling N. J., Toth R., Arthur J. S. C., Clark K. (2016). Inhibition of SIK2 and SIK3 during differentiation enhances the anti-inflammatory phenotype of macrophages. Biochem. J. 474 (4), 521–537. doi:10.1042/bcj20160646
DeBerge M., Shah S. J., Wilsbacher L., Thorp E. B. (2019). Macrophages in heart failure with reduced versus preserved ejection fraction. Trends Mol. Med. 25 (4), 328–340. doi:10.1016/j.molmed.2019.01.002
Doi J., Takemori H., Lin X. Z., Horike N., Katoh Y., Okamoto M. (2002). Salt-inducible kinase represses cAMP-dependent protein kinase-mediated activation of human cholesterol side chain cleavage cytochrome P450 promoter through the CREB basic leucine zipper domain. J. Biol. Chem. 277 (18), 15629–15637. doi:10.1074/jbc.m109365200
Dostanic I., Schultz Jel J., Lorenz J. N., Lingrel J. B. (2004). The alpha 1 isoform of Na,K-ATPase regulates cardiac contractility and functionally interacts and co-localizes with the Na/Ca exchanger in heart. J. Biol. Chem. 279 (52), 54053–54061. doi:10.1074/jbc.M410737200
Du J., Chen Q., Takemori H., Xu H. (2008). SIK2 can be activated by deprivation of nutrition and it inhibits expression of lipogenic genes in adipocytes. Obes. (Silver Spring) 16 (3), 531–538. doi:10.1038/oby.2007.98
Du Cailar G., Ribstein J., Daures J. P., Mimran A. (1992). Sodium and left ventricular mass in untreated hypertensive and normotensive subjects. Am. J. physiology 263 (1), H177–H181. doi:10.1152/ajpheart.1992.263.1.h177
Edmondson D. G., Lyons G. E., Martin J. F., Olson E. N. (1994). Mef2 gene expression marks the cardiac and skeletal muscle lineages during mouse embryogenesis. Development 120 (5), 1251–1263. doi:10.1242/dev.120.5.1251
Eneling K., Brion L., Pinto V., Pinho M. J., Sznajder J. I., Mochizuki N., et al. (2012). Salt-inducible kinase 1 regulates E-cadherin expression and intercellular junction stability. FASEB J. 26 (8), 3230–3239. doi:10.1096/fj.12-205609
Facchi C., Zi M., Prehar S., King K., Njegic A., Wang X., et al. (2022). Salt-inducible kinase 2 (SIK2) modulates cardiac remodeling following ischemic pathological stress. J. Mol. Cell. Cardiol. 173, S39. doi:10.1016/j.yjmcc.2022.08.077
Fagerberg L., Hallström B. M., Oksvold P., Kampf C., Djureinovic D., Odeberg J., et al. (2014). Analysis of the human tissue-specific expression by genome-wide integration of transcriptomics and antibody-based proteomics. Mol. Cell Proteomics 13 (2), 397–406. doi:10.1074/mcp.M113.035600
Fagiani F., Di Marino D., Romagnoli A., Travelli C., Voltan D., Di Cesare Mannelli L., et al. (2022). Molecular regulations of circadian rhythm and implications for physiology and diseases. Signal Transduct. Target Ther. 7 (1), 41. doi:10.1038/s41392-022-00899-y
Feldman J. D., Vician L., Crispino M., Hoe W., Baudry M., Herschman H. R. (2000). The salt-inducible kinase, SIK, is induced by depolarization in brain. J. Neurochem. 74 (6), 2227–2238. doi:10.1046/j.1471-4159.2000.0742227.x
Ferreira D. N., Katayama I. A., Oliveira I. B., Rosa K. T., Furukawa L. N. S., Coelho M. S., et al. (2010). Salt-induced cardiac hypertrophy and interstitial fibrosis are due to a blood pressure–independent mechanism in Wistar rats. J. Nutr. 140 (10), 1742–1751. doi:10.3945/jn.109.117473
Fischle W., Dequiedt F., Hendzel M. J., Guenther M. G., Lazar M. A., Voelter W., et al. (2002). Enzymatic activity associated with class II HDACs is dependent on a multiprotein complex containing HDAC3 and SMRT/N-CoR. Mol. Cell 9 (1), 45–57. doi:10.1016/s1097-2765(01)00429-4
Foretz M., Hébrard S., Leclerc J., Zarrinpashneh E., Soty M., Mithieux G., et al. (2010). Metformin inhibits hepatic gluconeogenesis in mice independently of the LKB1/AMPK pathway via a decrease in hepatic energy state. J. Clin. investigation 120 (7), 2355–2369. doi:10.1172/jci40671
Frangogiannis N. G. (2021). Cardiac fibrosis. Cardiovasc Res. 117 (6), 1450–1488. doi:10.1093/cvr/cvaa324
Frohlich E. D., Varagic J. (2004). The role of sodium in hypertension is more complex than simply elevating arterial pressure. Nat. Clin. Pract. Cardiovasc. Med. 1 (1), 24–30. doi:10.1038/ncpcardio0025
Frydman N., Steffann J., Girerd B., Frydman R., Munnich A., Simonneau G., et al. (2012). Pre-implantation genetic diagnosis in pulmonary arterial hypertension due to BMPR2 mutation. Eur. Respir. J. 39 (6), 1534–1535. doi:10.1183/09031936.00185011
Gibbons G. H., Dzau V. J. (1994). The emerging concept of vascular remodeling. N. Engl. J. Med. 330 (20), 1431–1438. doi:10.1056/nejm199405193302008
Gormand A., Berggreen C., Amar L., Henriksson E., Lund I., Albinsson S., et al. (2014). LKB1 signalling attenuates early events of adipogenesis and responds to adipogenic cues. J. Mol. Endocrinol. 53 (1), 117–130. doi:10.1530/jme-13-0296
Hage C., Michaëlsson E., Linde C., Donal E., Daubert J. C., Gan L. M., et al. (2017). Inflammatory biomarkers predict heart failure severity and prognosis in patients with heart failure with preserved ejection fraction: a holistic proteomic approach. Circ. Cardiovasc Genet. 10 (1), e001633. doi:10.1161/circgenetics.116.001633
Handschin C., Rhee J., Lin J., Tarr P. T., Spiegelman B. M. (2003). An autoregulatory loop controls peroxisome proliferator-activated receptor gamma coactivator 1alpha expression in muscle. Proc. Natl. Acad. Sci. U. S. A. 100 (12), 7111–7116. doi:10.1073/pnas.1232352100
Hartl C., Maser I.-P., Michels T., Milde R., Klein V., Beckhove P., et al. (2022). Abstract 3708: OMX-0407, a highly potent SIK3 inhibitor, sensitizes tumor cells to cell death and eradicates tumors in combination with PD-1 inhibition. Cancer Res. 82 (12), 3708. doi:10.1158/1538-7445.Am2022-3708
Hashimoto Y. K., Satoh T., Okamoto M., Takemori H. (2008). Importance of autophosphorylation at Ser186 in the A-loop of salt inducible kinase 1 for its sustained kinase activity. J. Cell. Biochem. 104 (5), 1724–1739. doi:10.1002/jcb.21737
Henderson N. C., Rieder F., Wynn T. A. (2020). Fibrosis: from mechanisms to medicines. Nature 587 (7835), 555–566. doi:10.1038/s41586-020-2938-9
Henriksson E., Jones H. A., Patel K. A., Peggie M., Morrice N. A., Sakamoto K., et al. (2012). The AMPK-related kinase SIK2 is regulated by cAMP via phosphorylation at Ser358 in adipocytes. Biochem. J. 444 (3), 503–514. doi:10.1042/bj20111932
Henriksson E., Säll J., Gormand A., Wasserstrom S., Morrice N. A., Fritzen A. M., et al. (2015). SIK2 regulates CRTCs, HDAC4 and glucose uptake in adipocytes. J. Cell Sci. 128 (3), 472–486. doi:10.1242/jcs.153932
Horike N., Takemori H., Katoh Y., Doi J., Min L., Asano T., et al. (2003). Adipose-specific expression, phosphorylation of Ser794 in insulin receptor substrate-1, and activation in diabetic animals of salt-inducible kinase-2. J. Biol. Chem. 278 (20), 18440–18447. doi:10.1074/jbc.m211770200
Hsu A. (2022). “Identifying Signaling and gene regulatory Mechanisms of pathologic cardiac Remodeling and heart failure pathogenesis,”. Ph.D. (San Francisco: University of California).
Hsu A., Duan Q., McMahon S., Huang Y., Wood S. A., Gray N. S., et al. (2020). Salt-inducible kinase 1 maintains HDAC7 stability to promote pathologic cardiac remodeling. J. Clin. Invest. 130 (6), 2966–2977. doi:10.1172/jci133753
Hutchinson L. D., Darling N. J., Nicolaou S., Gori I., Squair D. R., Cohen P., et al. (2020). Salt-inducible kinases (SIKs) regulate TGFβ-mediated transcriptional and apoptotic responses. Cell death Dis. 11 (1), 49. doi:10.1038/s41419-020-2241-6
Itoh Y., Sanosaka M., Fuchino H., Yahara Y., Kumagai A., Takemoto D., et al. (2015). Salt-inducible kinase 3 signaling is important for the gluconeogenic programs in mouse hepatocytes. J. Biol. Chem. 290 (29), 17879–17893. doi:10.1074/jbc.M115.640821
Iwamoto T., Kita S., Zhang J., Blaustein M. P., Arai Y., Yoshida S., et al. (2004). Salt-sensitive hypertension is triggered by Ca2+ entry via Na+/Ca2+ exchanger type-1 in vascular smooth muscle. Nat. Med. 10 (11), 1193–1199. doi:10.1038/nm1118
Jagannath A., Butler R., Sih G., Couch Y., Brown L. A., Vasudevan S. R., et al. (2013). The CRTC1-SIK1 pathway regulates entrainment of the circadian clock. Cell 154 (5), 1100–1111. doi:10.1016/j.cell.2013.08.004
Jagannath A., Taylor L., Ru Y., Wakaf Z., Akpobaro K., Vasudevan S., et al. (2023). The multiple roles of salt-inducible kinases in regulating physiology. Physiol. Rev. 103 (3), 2231–2269. doi:10.1152/physrev.00023.2022
Jaitovich A., Bertorello A. M. (2010). Intracellular sodium sensing: SIK1 network, hormone action and high blood pressure. Biochimica biophysica acta 1802 (12), 1140–1149. doi:10.1016/j.bbadis.2010.03.009
Jaleel M., Villa F., Deak M., Toth R., Prescott A. R., van Aalten D. M. F., et al. (2006). The ubiquitin-associated domain of AMPK-related kinases regulates conformation and LKB1-mediated phosphorylation and activation. Biochem. J. 394 (3), 545–555. doi:10.1042/bj20051844
Kalogeropoulos A., Georgiopoulou V., Psaty B. M., Rodondi N., Smith A. L., Harrison D. G., et al. (2010). Inflammatory markers and incident heart failure risk in older adults: the Health ABC (Health, Aging, and Body Composition) study. J. Am. Coll. Cardiol. 55 (19), 2129–2137. doi:10.1016/j.jacc.2009.12.045
Kanyo R., Price D. M., Chik C. L., Ho A. K. (2009). Salt-inducible kinase 1 in the rat pinealocytes: adrenergic regulation and role in arylalkylamine N-acetyltransferase gene transcription. Endocrinology 150 (9), 4221–4230. doi:10.1210/en.2009-0275
Karjalainen M. K., Holmes M. V., Wang Q., Anufrieva O., Kähönen M., Lehtimäki T., et al. (2020). Apolipoprotein A-I concentrations and risk of coronary artery disease: a Mendelian randomization study. Atherosclerosis 299, 56–63. doi:10.1016/j.atherosclerosis.2020.02.002
Katoh Y., Takemori H., Doi J., Okamoto M. (2002). Identification of the nuclear localization domain of salt-inducible kinase. Endocr. Res. 28 (4), 315–318. doi:10.1081/erc-120016802
Katoh Y., Takemori H., Horike N., Doi J., Muraoka M., Min L., et al. (2004a). Salt-inducible kinase (SIK) isoforms: their involvement in steroidogenesis and adipogenesis. Mol. Cell. Endocrinol. 217 (1), 109–112. doi:10.1016/j.mce.2003.10.016
Katoh Y., Takemori H., Lin X.-z., Tamura M., Muraoka M., Satoh T., et al. (2006). Silencing the constitutive active transcription factor CREB by the LKB1-SIK signaling cascade. FEBS J. 273 (12), 2730–2748. doi:10.1111/j.1742-4658.2006.05291.x
Katoh Y., Takemori H., Min L., Muraoka M., Doi J., Horike N., et al. (2004b). Salt-inducible kinase-1 represses cAMP response element-binding protein activity both in the nucleus and in the cytoplasm. Eur. J. Biochem. 271 (21), 4307–4319. doi:10.1111/j.1432-1033.2004.04372.x
Kenagy R. D. (2011). “Biology of restenosis and targets for intervention,” in Mechanisms of vascular disease: a reference book for vascular specialists. Editors R. Fitridge, and M. Thompson (Adelaide (AU): University of Adelaide Press).
Kim J., Hwangbo C., Hu X., Kang Y., Papangeli I., Mehrotra D., et al. (2014). Restoration of impaired endothelial myocyte enhancer factor 2 function rescues pulmonary arterial hypertension. Circulation 131 (2), 190–199. doi:10.1161/circulationaha.114.013339
Kim M. J., Park S. K., Lee J. H., Jung C. Y., Sung D. J., Park J.-H., et al. (2015). Salt-inducible kinase 1 terminates cAMP signaling by an evolutionarily conserved negative-feedback loop in β-cells. Diabetes 64 (9), 3189–3202. doi:10.2337/db14-1240
Kim S. Y., Jeong S., Chah K.-H., Jung E., Baek K.-H., Kim S.-T., et al. (2013). Salt-inducible kinases 1 and 3 negatively regulate Toll-like receptor 4-mediated signal. Mol. Endocrinol. 27 (11), 1958–1968. doi:10.1210/me.2013-1240
Ko A., Cantor R. M., Weissglas-Volkov D., Nikkola E., Reddy P. M., Sinsheimer J. S., et al. (2014). Amerindian-specific regions under positive selection harbour new lipid variants in Latinos. Nat. Commun. 5, 3983. doi:10.1038/ncomms4983
Koo S. H., Flechner L., Qi L., Zhang X., Screaton R. A., Jeffries S., et al. (2005). The CREB coactivator TORC2 is a key regulator of fasting glucose metabolism. Nature 437 (7062), 1109–1111. doi:10.1038/nature03967
Kowanetz M., Lönn P., Vanlandewijck M., Kowanetz K., Heldin C.-H., Moustakas A. (2008). TGFbeta induces SIK to negatively regulate type I receptor kinase signaling. J. Cell Biol. 182 (4), 655–662. doi:10.1083/jcb.200804107
Lan N. S. H., Massam B. D., Kulkarni S. S., Lang C. C. (2018). Pulmonary arterial hypertension: pathophysiology and treatment. Diseases 6 (2), 38. doi:10.3390/diseases6020038
Lee J., Yamazaki T., Dong H., Jefcoate C. R. (2016). A single cell level measurement of StAR expression and activity in adrenal cells. Mol. Cell. Endocrinol. 441 (NA), 22–30. doi:10.1016/j.mce.2016.08.015
Lehmann L. H., Jebessa Z. H., Kreusser M. M., Horsch A., He T., Kronlage M., et al. (2017). A proteolytic fragment of histone deacetylase 4 protects the heart from failure by regulating the hexosamine biosynthetic pathway. Nat. Med. 24 (1), 62–72. doi:10.1038/nm.4452
Lin X.-z., Takemori H., Katoh Y., Doi J., Horike N., Makino A., et al. (2001). Salt-inducible kinase is involved in the ACTH/cAMP-dependent protein kinase signaling in Y1 mouse adrenocortical tumor cells. Mol. Endocrinol. 15 (8), 1264–1276. doi:10.1210/mend.15.8.0675
Lincoff A. M., Brown-Frandsen K., Colhoun H. M., Deanfield J., Emerson S. S., Esbjerg S., et al. (2023). Semaglutide and cardiovascular outcomes in obesity without diabetes. N. Engl. J. Med. 389 (24), 2221–2232. doi:10.1056/NEJMoa2307563
Liu D., Qq L., Eyries M., Wu W.-H., Yuan P., Zhang R., et al. (2011). Molecular genetics and clinical features of Chinese idiopathic and heritable pulmonary arterial hypertension patients. Eur. Respir. J. 39 (3), 597–603. doi:10.1183/09031936.00072911
Liu S., Huang S., Wu X., Feng Y., Shen Y., Zhao Q. S., et al. (2020). Activation of SIK1 by phanginin A inhibits hepatic gluconeogenesis by increasing PDE4 activity and suppressing the cAMP signaling pathway. Mol. Metab. 41, 101045. doi:10.1016/j.molmet.2020.101045
Liu X., Xu L., Wu J., Zhang Y., Wu C., Zhang X. (2022). Down-regulation of SIK2 expression alleviates myocardial ischemia-reperfusion injury in rats by inhibiting autophagy through the mTOR-ULK1 signaling pathway. Nan Fang. Yi Ke Da Xue Xue Bao 42 (7), 1082–1088. doi:10.12122/j.issn.1673-4254.2022.07.18
Lombardi M. S., Gilliéron C., Dietrich D., Gabay C. (2016). SIK inhibition in human myeloid cells modulates TLR and IL-1R signaling and induces an anti-inflammatory phenotype. J. Leukoc. Biol. 99 (5), 711–721. doi:10.1189/jlb.2A0715-307R
Lönn P., Vanlandewijck M., Raja E., Kowanetz M., Watanabe Y., Kowanetz K., et al. (2012). Transcriptional induction of salt-inducible kinase 1 by transforming growth factor β leads to negative regulation of type I receptor signaling in cooperation with the Smurf2 ubiquitin ligase. J. Biol. Chem. 287 (16), 12867–12878. doi:10.1074/jbc.m111.307249
Luan B., Goodarzi M. O., Phillips N. G., Guo X., Chen Y.-D. I., Yao J., et al. (2014). Leptin-mediated increases in catecholamine signaling reduce adipose tissue inflammation via activation of macrophage HDAC4. Cell metab. 19 (6), 1058–1065. doi:10.1016/j.cmet.2014.03.024
Ma L., Roman-Campos D., Austin E. D., Eyries M., Sampson K. J., Soubrier F., et al. (2013). A novel channelopathy in pulmonary arterial hypertension. N. Engl. J. Med. 369 (4), 351–361. doi:10.1056/nejmoa1211097
MacKenzie K. F., Clark K., Naqvi S., McGuire V. A., Nöehren G., Kristariyanto Y. A., et al. (2012). PGE2 induces macrophage IL-10 production and a regulatory-like phenotype via a protein kinase A–SIK–CRTC3 pathway. J. Immunol. 190 (2), 565–577. doi:10.4049/jimmunol.1202462
Medina C. B., Chiu Y.-H., Stremska M. E., Lucas C. D., Poon I. K. H., Tung K. S. K., et al. (2021). Pannexin 1 channels facilitate communication between T cells to restrict the severity of airway inflammation. Immunity 54 (8), 1715–1727.e7. doi:10.1016/j.immuni.2021.06.014
Montani D., Girerd B., Günther S., Riant F., Tournier-Lasserve E., Magy L., et al. (2013). Pulmonary arterial hypertension in familial hemiplegic migraine with ATP1A2 channelopathy. Eur. Respir. J. 43 (2), 641–643. doi:10.1183/09031936.00147013
Moore E. D., Etter E. F., Philipson K. D., Carrington W. A., Fogarty K. E., Lifshitz L. M., et al. (1993). Coupling of the Na+/Ca2+ exchanger, Na+/K+ pump and sarcoplasmic reticulum in smooth muscle. Nature 365 (6447), 657–660. doi:10.1038/365657a0
Nefla M., Darling N. J., van Gijsel Bonnello M., Cohen P., Arthur J. S. C. (2021). Salt inducible kinases 2 and 3 are required for thymic T cell development. Sci. Rep. 11 (1), 21550–NA. doi:10.1038/s41598-021-00986-0
Nguyen P. T., Deisl C., Fine M., Tippetts T. S., Uchikawa E., Bai X. C., et al. (2022). Structural basis for gating mechanism of the human sodium-potassium pump. Nat. Commun. 13 (1), 5293. doi:10.1038/s41467-022-32990-x
Nixon M., Stewart-Fitzgibbon R., Fu J., Akhmedov D., Rajendran K., Mendoza-Rodriguez M. G., et al. (2015). Skeletal muscle salt inducible kinase 1 promotes insulin resistance in obesity. Mol. Metab. 5 (1), 34–46. doi:10.1016/j.molmet.2015.10.004
Pahwa R., Goyal A., Jialal I. (2024). “Chronic inflammation,” in StatPearls (Treasure Island (FL): StatPearls Publishing).
Park J.-Y., Yoon Y. S., Han H. S., Kim Y.-H., Ogawa Y., Park K.-G., et al. (2014). SIK2 is critical in the regulation of lipid homeostasis and adipogenesis in vivo. Diabetes 63 (11), 3659–3673. doi:10.2337/db13-1423
Park S. Y., Kim J. S. (2020). A short guide to histone deacetylases including recent progress on class II enzymes. Exp. Mol. Med. 52 (2), 204–212. doi:10.1038/s12276-020-0382-4
Patel K. A., Foretz M., Marion A., Campbell D. G., Gourlay R., Boudaba N., et al. (2014). The LKB1-salt-inducible kinase pathway functions as a key gluconeogenic suppressor in the liver. Nat. Commun. 5 (1), 4535. doi:10.1038/ncomms5535
Patel Y., Joseph J. (2020). Sodium intake and heart failure. Int. J. Mol. Sci. 21 (24), 9474. doi:10.3390/ijms21249474
Peixoto C., Joncour A., Temal-Laib T., Tirera A., Dos Santos A., Jary H., et al. (2024). Discovery of clinical candidate GLPG3970: a potent and selective dual SIK2/SIK3 inhibitor for the treatment of autoimmune and inflammatory diseases. J. Med. Chem. 67, 5233–5258. doi:10.1021/acs.jmedchem.3c02246
Pinho M. J., Bertorello A. M., Silva P.S.-d.-. (2012). Abstract 325: cardiac hypertrophy in spontaneous hypertensive rats (SHR) is associated with decreased cardiac expression of SIK1 and SIK3 isoforms. Hypertension 60 (1), A325. doi:10.1161/hyp.60.suppl_1.A325
Pirahanchi Y., Jessu R., Aeddula N. R. (2024). “Physiology, sodium potassium pump,” in StatPearls (Treasure Island (FL): Ineligible companies).
Pires N., Igreja B., Soares-da-Silva P. (2021). Antagonistic modulation of SIK1 and SIK2 isoforms in high blood pressure and cardiac hypertrophy triggered by high-salt intake. Clin. Exp. Hypertens. 43 (5), 428–435. doi:10.1080/10641963.2021.1896728
Pires N. M., Igreja B., Serrão M. P., Matias E. F., Moura E., António T., et al. (2019). Acute salt loading induces sympathetic nervous system overdrive in mice lacking salt-inducible kinase 1 (SIK1). Hypertens. Res. 42 (8), 1114–1124. doi:10.1038/s41440-019-0249-z
Popov S., Silveira A., Wågsäter D., Takemori H., Oguro R., Matsumoto S., et al. (2011). Salt-inducible kinase 1 influences Na(+),K(+)-ATPase activity in vascular smooth muscle cells and associates with variations in blood pressure. J. Hypertens. 29 (12), 2395–2403. doi:10.1097/hjh.0b013e32834d3d55
Popov S., Takemori H., Tokudome T., Mao Y., Otani K., Mochizuki N., et al. (2014). Lack of salt-inducible kinase 2 (SIK2) prevents the development of cardiac hypertrophy in response to chronic high-salt intake. PLoS One 9 (4), e95771. doi:10.1371/journal.pone.0095771
Popov S., Venetsanou K., Chedrese P. J., Pinto V., Takemori H., Franco-Cereceda A., et al. (2012). Increases in intracellular sodium activate transcription and gene expression via the salt-inducible kinase 1 network in an atrial myocyte cell line. Am. J. physiology. Heart circulatory physiology 303 (1), H57–H65. doi:10.1152/ajpheart.00512.2011
Powell-Wiley T. M., Poirier P., Burke L. E., Després J. P., Gordon-Larsen P., Lavie C. J., et al. (2021). Obesity and cardiovascular disease: a scientific statement from the American heart association. Circulation 143 (21), e984–e1010. doi:10.1161/cir.0000000000000973
Pu J., Wang F., Ye P., Jiang X., Zhou W., Gu Y., et al. (2022). Salt-inducible kinase 1 deficiency promotes vascular remodeling in pulmonary arterial hypertension via enhancement of yes-associated protein-mediated proliferation. Heliyon 8 (10), e11016. doi:10.1016/j.heliyon.2022.e11016
Qi L., Saberi M., Zmuda E., Wang Y., Altarejos J. Y., Zhang X., et al. (2009). Adipocyte CREB promotes insulin resistance in obesity. Cell metab. 9 (3), 277–286. doi:10.1016/j.cmet.2009.01.006
Qu C., Qu Y. (2017). Down-regulation of salt-inducible kinase 1 (SIK1) is mediated by RNF2 in hepatocarcinogenesis. Oncotarget 8 (2), 3144–3155. doi:10.18632/oncotarget.13673
Reynolds A. M., Holmes M., Danilov S. M., Reynolds P. N. (2011). Targeted gene delivery of BMPR2 attenuates pulmonary hypertension. Eur. Respir. J. 39 (2), 329–343. doi:10.1183/09031936.00187310
Romito A., Lonardo E., Roma G., Minchiotti G., Ballabio A., Cobellis G. (2010). Lack of sik1 in mouse embryonic stem cells impairs cardiomyogenesis by down-regulating the cyclin-dependent kinase inhibitor p57kip2. PLoS One 5 (2), e9029. doi:10.1371/journal.pone.0009029
Ruiz J. C., Conlon F. L., Robertson E. J. (1994). Identification of novel protein kinases expressed in the myocardium of the developing mouse heart. Mech. Dev. 48 (3), 153–164. doi:10.1016/0925-4773(94)90056-6
Sakamaki J., Fu A., Reeks C., Baird S., Depatie C., Al Azzabi M., et al. (2014). Role of the SIK2-p35-PJA2 complex in pancreatic β-cell functional compensation. Nat. Cell Biol. 16 (3), 234–244. doi:10.1038/ncb2919
Sakamoto K., Bultot L., Goransson O. (2018). The salt-inducible kinases: emerging metabolic regulators. Trends Endocrinol. Metab. 29 (12), 827–840. doi:10.1016/j.tem.2018.09.007
Säll J., Pettersson A. M. L., Björk C., Henriksson E., Wasserstrom S., Linder W., et al. (2016). Salt-inducible kinase 2 and -3 are downregulated in adipose tissue from obese or insulin-resistant individuals: implications for insulin signalling and glucose uptake in human adipocytes. Diabetologia 60 (2), 314–323. doi:10.1007/s00125-016-4141-y
Sanosaka M., Fujimoto M., Ohkawara T., Nagatake T., Itoh Y., Kagawa M., et al. (2015). Salt-inducible kinase 3 deficiency exacerbates lipopolysaccharide-induced endotoxin shock accompanied by increased levels of pro-inflammatory molecules in mice. Immunology 145 (2), 268–278. doi:10.1111/imm.12445
Sasaki T., Takemori H., Yagita Y., Terasaki Y., Uebi T., Horike N., et al. (2011). SIK2 is a key regulator for neuronal survival after ischemia via TORC1-CREB. Neuron 69 (1), 106–119. doi:10.1016/j.neuron.2010.12.004
Sato T., Andrade C. D. C., Yoon S. H., Zhao Y., Greenlee W. J., Weber P. C., et al. (2022). Structure-based design of selective, orally available salt-inducible kinase inhibitors that stimulate bone formation in mice. Proc. Natl. Acad. Sci. U. S. A. 119 (50), e2214396119. doi:10.1073/pnas.2214396119
Sattar N., Lee M. M. Y., Kristensen S. L., Branch K. R. H., Del Prato S., Khurmi N. S., et al. (2021). Cardiovascular, mortality, and kidney outcomes with GLP-1 receptor agonists in patients with type 2 diabetes: a systematic review and meta-analysis of randomised trials. Lancet Diabetes Endocrinol. 9 (10), 653–662. doi:10.1016/s2213-8587(21)00203-5
Screaton R. A., Conkright M. D., Katoh Y., Best J. L., Canettieri G., Jeffries S., et al. (2004). The CREB coactivator TORC2 functions as a calcium- and cAMP-sensitive coincidence detector. Cell 119 (1), 61–74. doi:10.1016/j.cell.2004.09.015
Shan T., Xiong Y., Zhang P., Li Z., Jiang Q., Bi P., et al. (2016). Lkb1 controls brown adipose tissue growth and thermogenesis by regulating the intracellular localization of CRTC3. Nat. Commun. 7, 12205. doi:10.1038/ncomms12205
Shen H., McElhinny A. S., Cao Y., Gao P., Liu J., Bronson R. T., et al. (2006). The Notch coactivator, MAML1, functions as a novel coactivator for MEF2C-mediated transcription and is required for normal myogenesis. Genes and Dev. 20 (6), 675–688. doi:10.1101/gad.1383706
Shi F., Collins S. (2017). Second messenger signaling mechanisms of the brown adipocyte thermogenic program: an integrative perspective. Horm. Mol. Biol. Clin. Investig. 31 (2). doi:10.1515/hmbci-2017-0062
Shi F., Collins S. (2023). Regulation of mTOR signaling: emerging role of cyclic nucleotide-dependent protein kinases and implications for cardiometabolic disease. Int. J. Mol. Sci. 24 (14), 11497. doi:10.3390/ijms241411497
Shi F., de Fatima Silva F., Liu D., Patel H. U., Xu J., Zhang W., et al. (2023). Salt-inducible kinase inhibition promotes the adipocyte thermogenic program and adipose tissue browning. Mol. Metab. 74, 101753. doi:10.1016/j.molmet.2023.101753
Sjöström M., Stenström K., Eneling K., Zwiller J., Katz A. I., Takemori H., et al. (2007). SIK1 is part of a cell sodium-sensing network that regulates active sodium transport through a calcium-dependent process. Proc. Natl. Acad. Sci. U. S. A. 104 (43), 16922–16927. doi:10.1073/pnas.0706838104
Sonntag T., Vaughan J., Montminy M. (2018). 14-3-3 proteins mediate inhibitory effects of cAMP on salt-inducible kinases (SIKs). FEBS J. 285 (3), 467–480. doi:10.1111/febs.14351
Stewart R., Akhmedov D., Robb C., Leiter C., Berdeaux R. (2012). Regulation of SIK1 abundance and stability is critical for myogenesis. Proc. Natl. Acad. Sci. U. S. A. 110 (1), 117–122. doi:10.1073/pnas.1212676110
Stewart R., Flechner L., Montminy M., Berdeaux R. (2011). CREB is activated by muscle injury and promotes muscle regeneration. PLoS One 6 (9), e24714. doi:10.1371/journal.pone.0024714
Strain W. D., Paldánius P. M. (2018). Diabetes, cardiovascular disease and the microcirculation. Cardiovasc Diabetol. 17 (1), 57. doi:10.1186/s12933-018-0703-2
Sun Z., Jiang Q., Li J., Guo J. (2020). The potent roles of salt-inducible kinases (SIKs) in metabolic homeostasis and tumorigenesis. Signal Transduct. Target Ther. 5 (1), 150. doi:10.1038/s41392-020-00265-w
Sundberg T. B., Choi H. G., Song J. H., Russell C. N., Hussain M. M., Graham D. B., et al. (2014). Small-molecule screening identifies inhibition of salt-inducible kinases as a therapeutic strategy to enhance immunoregulatory functions of dendritic cells. Proc. Natl. Acad. Sci. U. S. A. 111 (34), 12468–12473. doi:10.1073/pnas.1412308111
Sundberg T. B., Liang Y., Wu H., Choi H. G., Kim N. D., Sim T., et al. (2016). Development of chemical probes for investigation of salt-inducible kinase function in vivo. ACS Chem. Biol. 11 (8), 2105–2111. doi:10.1021/acschembio.6b00217
Tang C. C., Castro Andrade C. D., O'Meara M. J., Yoon S. H., Sato T., Brooks D. J., et al. (2021). Dual targeting of salt inducible kinases and CSF1R uncouples bone formation and bone resorption. Elife 10, e67772. doi:10.7554/eLife.67772
Tarumoto Y., Lin S., Wang J., Milazzo J. P., Xu Y., Lu B., et al. (2020). Salt-inducible kinase inhibition suppresses acute myeloid leukemia progression in vivo. Blood 135 (1), 56–70. doi:10.1182/blood.2019001576
Taub M. (2018). Gene level regulation of Na,K-ATPase in the renal proximal tubule is controlled by two independent but interacting regulatory mechanisms involving salt inducible kinase 1 and CREB-regulated transcriptional coactivators. Int. J. Mol. Sci. 19 (7), 2086. doi:10.3390/ijms19072086
Taub M., Garimella S., Kim D., Rajkhowa T., Cutuli F. (2015). Renal proximal tubule Na,K-ATPase is controlled by CREB-regulated transcriptional coactivators as well as salt-inducible kinase 1. Cell. Signal. 27 (12), 2568–2578. doi:10.1016/j.cellsig.2015.09.015
Taub M., Springate J. E., Cutuli F. (2010). Targeting of renal proximal tubule Na,K-ATPase by salt-inducible kinase. Biochem. biophysical Res. Commun. 393 (3), 339–344. doi:10.1016/j.bbrc.2010.02.037
Temal-Laib T., Peixoto C., Desroy N., De Lemos E., Bonnaterre F., Bienvenu N., et al. (2023). Optimization of selectivity and pharmacokinetic properties of salt-inducible kinase inhibitors that led to the discovery of pan-SIK inhibitor GLPG3312. J. Med. Chem. 67, 380–401. doi:10.1021/acs.jmedchem.3c01428
Teslovich T. M., Musunuru K., Smith A. V., Edmondson A. C., Stylianou I. M., Koseki M., et al. (2010). Biological, clinical and population relevance of 95 loci for blood lipids. Nature 466 (7307), 707–713. doi:10.1038/nature09270
Travers J. G., Hu T., McKinsey T. A. (2020). The black sheep of class IIa: HDAC7 SIKens the heart. J. Clin. Invest. 130 (6), 2811–2813. doi:10.1172/jci137074
Uebi T., Itoh Y., Hatano O., Kumagai A., Sanosaka M., Sasaki T., et al. (2012). Involvement of SIK3 in glucose and lipid homeostasis in mice. PloS one 7 (5), e37803–NA. doi:10.1371/journal.pone.0037803
Vanlandewijck M., Dadras M. S., Lomnytska M., Mahzabin T., Miller M. L., Busch C., et al. (2017). The protein kinase SIK downregulates the polarity protein Par3. Oncotarget 9 (5), 5716–5735. doi:10.18632/oncotarget.23788
Verzi M. P., Agarwal P., Brown C., McCulley D. J., Schwarz J. J., Black B. L. (2007). The transcription factor MEF2C is required for craniofacial development. Dev. Cell 12 (4), 645–652. doi:10.1016/j.devcel.2007.03.007
Wang Y., Paulo E., Wu D., Wu Y., Huang W., Chawla A., et al. (2017). Adipocyte liver kinase b1 suppresses beige adipocyte renaissance through class IIa histone deacetylase 4. Diabetes 66 (12), 2952–2963. doi:10.2337/db17-0296
Wang Y., Viscarra J., Kim S. J., Sul H. S. (2015). Transcriptional regulation of hepatic lipogenesis. Nat. Rev. Mol. Cell Biol. 16 (11), 678–689. doi:10.1038/nrm4074
Wang Z. n., Takemori H., Halder S. K., Nonaka Y., Okamoto M. (1999). Cloning of a novel kinase (SIK) of the SNF1/AMPK family from high salt diet-treated rat adrenal. FEBS Lett. 453 (1), 135–139. doi:10.1016/s0014-5793(99)00708-5
Weems J. C., Griesel B. A., Olson A. L. (2012). Class II histone deacetylases downregulate GLUT4 transcription in response to increased cAMP signaling in cultured adipocytes and fasting mice. Diabetes 61 (6), 1404–1414. doi:10.2337/db11-0737
Wein M. N., Foretz M., Fisher D. E., Xavier R. J., Kronenberg H. M. (2018). Salt-inducible kinases: physiology, regulation by cAMP, and therapeutic potential. Trends Endocrinol. Metab. 29 (10), 723–735. doi:10.1016/j.tem.2018.08.004
Wein M. N., Liang Y., Goransson O., Sundberg T. B., Wang J., Williams E. A., et al. (2016). SIKs control osteocyte responses to parathyroid hormone. Nat. Commun. 7, 13176. doi:10.1038/ncomms13176
Willer C. J., Mohlke K. L. (2012). Finding genes and variants for lipid levels after genome-wide association analysis. Curr. Opin. Lipidol. 23 (2), 98–103. doi:10.1097/MOL.0b013e328350fad2
Xiao Y. Y., Xia L. X., Jiang W. J., Qin J. F., Zhao L. X., Li Z., et al. (2024). Cardiopulmonary progenitors facilitate cardiac repair via exosomal transfer of miR-27b-3p targeting the SIK1-CREB1 axis. Cell Prolif. 57, e13593. doi:10.1111/cpr.13593
Yahara Y., Takemori H., Okada M., Kosai A., Yamashita A., Kobayashi T., et al. (2016). Pterosin B prevents chondrocyte hypertrophy and osteoarthritis in mice by inhibiting Sik3. Nat. Commun. 7, 10959. doi:10.1038/ncomms10959
Yang F.-C., Tan B.C.-M., Chen W. H., Lin Y. H., Huang J. Y., Chang H. Y., et al. (2013). Reversible acetylation regulates salt-inducible kinase (SIK2) and its function in autophagy. J. Biol. Chem. 288 (9), 6227–6237. doi:10.1074/jbc.m112.431239
Yilmaz O., Oztay F., Kayalar O. (2015). Dasatinib attenuated bleomycin-induced pulmonary fibrosis in mice. Growth factors 33 (5-6), 366–375. doi:10.3109/08977194.2015.1109511
Yodsaoue O., Cheenpracha S., Karalai C., Ponglimanont C., Chantrapromma S., Fun H. K., et al. (2008). Phanginin A-K, diterpenoids from the seeds of Caesalpinia sappan Linn. Phytochemistry 69 (5), 1242–1249. doi:10.1016/j.phytochem.2007.11.013
Yoon Y. S., Seo W. Y., Lee M. W., Kim S.-T., Koo S. H. (2009). Salt-inducible kinase regulates hepatic lipogenesis by controlling SREBP-1c phosphorylation. J. Biol. Chem. 284 (16), 10446–10452. doi:10.1074/jbc.m900096200
Yue F., Cheng Y., Breschi A., Vierstra J., Wu W., Ryba T., et al. (2014). A comparative encyclopedia of DNA elements in the mouse genome. Nature 515 (7527), 355–364. doi:10.1038/nature13992
Zhang C. L., McKinsey T. A., Chang S., Antos C. L., Hill J. A., Olson E. N. (2002). Class II histone deacetylases act as signal-responsive repressors of cardiac hypertrophy. Cell 110 (4), 479–488. doi:10.1016/s0092-8674(02)00861-9
Zhou J., Alfraidi A., Zhang S., Santiago-O'Farrill J. M., Yerramreddy Reddy V. K., Alsaadi A., et al. (2017). A novel compound ARN-3236 inhibits salt-inducible kinase 2 and sensitizes ovarian cancer cell lines and xenografts to paclitaxel. Clin. Cancer Res. 23 (8), 1945–1954. doi:10.1158/1078-0432.Ccr-16-1562
Zhu W., Liu X., Li Q., Gao F., Liu T., Chen X., et al. (2023). Discovery of novel and selective SIK2 inhibitors by the application of AlphaFold structures and generative models. Bioorg Med. Chem. 91, 117414. doi:10.1016/j.bmc.2023.117414
Keywords: salt-inducible kinases, cardiovascular disease, metabolic syndrome, inflammation, fibrosis, SIK inhibitors
Citation: Shi F (2024) Understanding the roles of salt-inducible kinases in cardiometabolic disease. Front. Physiol. 15:1426244. doi: 10.3389/fphys.2024.1426244
Received: 01 May 2024; Accepted: 26 June 2024;
Published: 16 July 2024.
Edited by:
Curtis C. Hughey, University of Minnesota Twin Cities, United StatesReviewed by:
Roxana Carbó, Instituto Nacional de Cardiología Ignacio Chávez, MexicoYujun Cai, Yale University, United States
Copyright © 2024 Shi. This is an open-access article distributed under the terms of the Creative Commons Attribution License (CC BY). The use, distribution or reproduction in other forums is permitted, provided the original author(s) and the copyright owner(s) are credited and that the original publication in this journal is cited, in accordance with accepted academic practice. No use, distribution or reproduction is permitted which does not comply with these terms.
*Correspondence: Fubiao Shi, fubiao.shi@vumc.org