- 1Department of Biomedical Engineering, School of Science and Engineering, Tulane University, New Orleans, LA, United States
- 2Department of Cell and Molecular Biology, School of Science and Engineering, Tulane University, New Orleans, LA, United States
- 3Department of Physiology, School of Medicine, Tulane University, New Orleans, LA, United States
Recent advances in organ chip (or, “organ-on-a-chip”) technologies and microphysiological systems (MPS) have enabled in vitro investigation of endothelial cell function in biomimetic three-dimensional environments under controlled fluid flow conditions. Many current organ chip models include a vascular compartment; however, the design and implementation of these vessel-on-a-chip components varies, with consequently varied impact on their ability to capture and reproduce hemodynamic flow and associated mechanosensitive signaling that regulates key characteristics of healthy, intact vasculature. In this review, we introduce organ chip and vessel-on-a-chip technology in the context of existing in vitro and in vivo vascular models. We then briefly discuss the importance of mechanosensitive signaling for vascular development and function, with focus on the major mechanosensitive signaling pathways involved. Next, we summarize recent advances in MPS and organ chips with an integrated vascular component, with an emphasis on comparing both the biomimicry and adaptability of the diverse approaches used for supporting and integrating intravascular flow. We review current data showing how intravascular flow and fluid shear stress impacts vessel development and function in MPS platforms and relate this to existing work in cell culture and animal models. Lastly, we highlight new insights obtained from MPS and organ chip models of mechanosensitive signaling in endothelial cells, and how this contributes to a deeper understanding of vessel growth and function in vivo. We expect this review will be of broad interest to vascular biologists, physiologists, and cardiovascular physicians as an introduction to organ chip platforms that can serve as viable model systems for investigating mechanosensitive signaling and other aspects of vascular physiology.
Introduction
The blood vasculature is an extensive organ system comprised of hierarchically organized blood vessels that circulate blood from the heart to all tissues of the body. The mechanical forces that blood flow exerts on vascular cells is a key mechanical signal for vascular cell function and homeostasis. Yet, the contribution of hemodynamic flow signaling to healthy and diseased vascular cell function can be difficult to capture experimentally, in part due to the nature of currently available in vitro and in vivo models of the blood vasculature. Here, we review the importance of hemodynamic flow in the blood vasculature and discuss how increasingly sophisticated microphysiological models of the vasculature, or so-called vessel-on-a-chip systems, may enable new study of flow-sensitive signaling and mechanotransduction in the blood vasculature.
Research on the biology of the blood vasculature has traditionally taken advantage of two general types of model systems. First, many studies use in vitro two-dimensional (2D) or three-dimensional (3D) cultures, with the latter often entailing co-culture of endothelial cells with vascular support cells, including fibroblasts, vascular smooth muscle cells, and/or pericytes. The simplicity and high throughput capacity of 2D cultures has enabled discovery of many fundamental aspects of endothelial cell biology, including response to shear stress in 2D cultures of endothelial cells in parallel plate flow chambers (James et al., 1995; Sedlak and Clyne, 2023). 3D cultures in hydrogels composed of natural ECM proteins are useful tools for modeling vasculogenesis and the impact of culture parameters on vascular network architecture. Vascular organoids generated with induced pluripotent stem cell derivatives are powerful tools for modeling key aspects of vascular niche formation including pericyte interactions and basement membrane synthesis (Mondrinos et al., 2014; Wimmer et al., 2019). Collectively, these evolving 2D and 3D culture models have advanced our understanding of vascular biology and the pharmacodynamics of drugs targeting the vasculature (Cochrane et al., 2019; Haas et al., 2020).
Regarding hemodynamics and more complex aspects of vascular physiology, most of the aforementioned in vitro cell culture systems fail to simultaneously capture the 3D architecture and flow perfusion of blood vessels in vivo. On the other hand, studies of intact blood vessels can be performed either in vivo in research animals (e.g., mice, rats, hamsters, etc.) or in ex vivo blood vessel explants. However, in vivo models can be expensive, time-consuming, and potentially incompatible with certain experimental approaches. Modeling the effects of different flow conditions in a reductionist manner while simultaneously controlling for other system elements is challenging unless isolated ex vivo vessel preparations are used (Adamson et al., 1994). Furthermore, differences between human and research animal physiology may further complicate interpretation of data for clinical translation (Seok et al., 2013).
In the last 20 years, new in vitro models—termed organ chip (or, “organ-on-a-chip”) technology and so-called microphysiological systems (MPS)—have emerged as powerful tools to complement existing in vitro and in vivo tissue models. MPS, which were originally conceived as “human surrogates” to serve as platforms for modeling pharmacokinetics and pharmacodynamics in vitro (Viravaidya and Shuler, 2004; Edington et al., 2018), can fill the gap between traditional culture systems and animal models by situating human cells in a more physiologically-relevant context with controlled biochemical and biophysical parameters (Bhatia and Ingber, 2014).
Early MPS designs focused on the use of printed microfluidic features to interconnect cultures of multiple human cell types and enable real-time communication via the transport of soluble factors. In 2004, Viravaidya et al. developed a “microscale cell culture analog” system in which a series of culture chambers containing lung and liver cells were connected by patterned microfluidic channels to “mimic the circulatory system.” (Viravaidya et al., 2004) While in this model an acellular circuit of microfluidic channels accomplishes the essential macroscale transport functions of the circulatory system, the endothelium is in itself an organ that influences tissue-specific and systemic physiology (Augustin and Koh, 2017). Thus, development of systems that faithfully recapitulate the vascular niche of intact blood vessels has long been considered a critical requirement for improving the physiological relevance of MPS (Ewald et al., 2021). In 2010, Huh and colleagues integrated a cellularized vascular compartment in their lung-on-a-chip model of the alveolar-capillary tissue-tissue interface. In this model, a lower tissue channel is lined with primary vascular endothelial cells to form an endothelial monolayer cultured under pump-driven flow and separated from an overlying lung epithelial monolayer by a porous elastomeric membrane that enables inter-tissue exchange of growth factors and nutrients (Huh, 2015). This pioneering microphysiological model of the blood-air interface of the lung has since been used to capture a variety of complex tissue interactions, including immune responses to respiratory virus infection (Huh, 2015; Si et al., 2021).
In the years since these early proof-of-concept prototypes, there has been a proliferation of unique organ chip models—all varying in their platform design and target tissue, as well as presence (or absence) of a vascular tissue component. These include (but are not limited to) working organ chip models of the gut and associated microbiome (Langer et al., 2019; Poceviciute and Ismagilov, 2019), the female reproductive system (Xiao et al., 2017), the pancreatic islet (Bender et al., 2024), the blood-brain barrier (Phan et al., 2017; Campisi et al., 2018), and cancer growth and metastasis (Jeon et al., 2015; Sobrino et al., 2016; Chen et al., 2017; Hachey et al., 2021; Hachey et al., 2024) among many others. Ongoing efforts to engineer body-on-a-chip systems will likely require capturing the physiology of a continuous intact vasculature under physiological flow to model systemic processes and pharmacokinetics (Vogt, 2022). In this review, we will focus on the importance of hemodynamic flow as a regulator of vascular physiology and disease, and explore the challenges of integrating these physiological flow forces in next-generation vessel-on-a-chip designs.
The blood vasculature and hemodynamic flow
Several specialized blood vessel types support efficient systemic circulation, including muscularized arteries and arterioles that constrict and dilate to control local and systemic blood flow; thinly-walled veins and venules that function as elastic capacitance reservoirs for excess blood volume, and intervening small-caliber capillaries (typically 8–10 µm in diameter) that connect the arteriolar and venous sides of the circulatory system and that function as the primary site of oxygen, nutrient, and waste exchange between blood and surrounding tissue. All blood vessels are lined on their luminal surface by endothelial cells—specialized epithelial cell types that form a continuous barrier between circulating blood and surrounding tissue. The apical, lumen-facing surface of vascular endothelium is enriched for proteoglycans and glycoproteins, which forms a protective and immunoregulatory layer known as the glycocalyx that interfaces directly with circulating blood (Figure 1). The basolateral side of endothelial cells, on the other hand, rests upon the tunica interna—a collagen-, laminin-, and fibronectin-rich basement membrane supported by an elastin-rich internal elastic lamina that confers radial elasticity onto the blood vessel. Beyond the tunica interna, perivascular cells—including contractile smooth muscle cells and vessel-stabilizing pericytes—form a medial layer that stabilizes blood vessels and—in the case of smooth muscle—constricts and relaxes to establish local vessel tone and regulate downstream blood flow. On the outer surface of the blood vessel is the tunica externa, a collagen-rich layer of extracellular matrix that in arteries also includes an external elastic lamina that confers additional vascular elasticity to the vessel. Mural cells are physically separated from the vascular endothelium by the tunica interna except via pores in the intervening extracellular matrix layer (termed myoendothelial junctions (Heberlein et al., 2009)) that enable endothelial-mural cell-cell contact and intracellular exchange of signaling molecules.
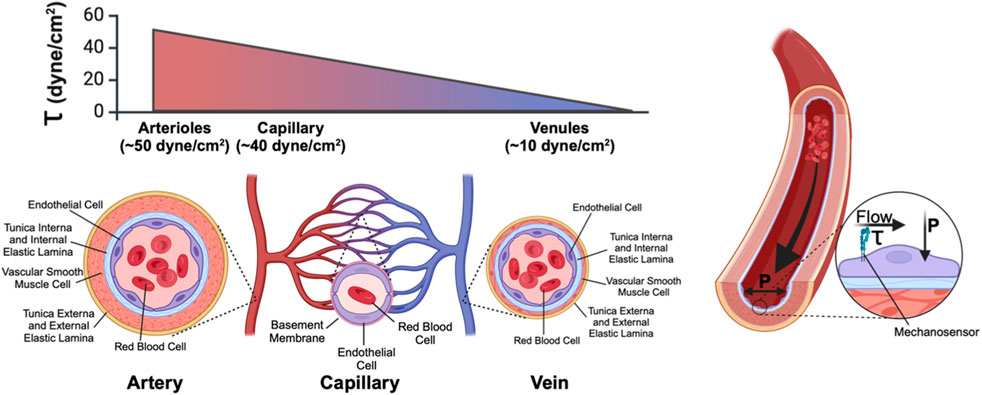
Figure 1. Anatomy of a blood vessel. Blood vessels are lined on their luminal surface by the vascular endothelium, which consists of a single continuous layer of endothelial cells. Surrounding the vascular endothelium is an extracellular matrix layer (tunica interna) which separates the endothelium from the medial layer, which consists of vascular smooth muscle cells (arteries/arterioles, and veins/venules) or pericytes (capillaries). The outermost layer of blood vessels is the tunica externa, another collagen-rich extracellular matrix layer. Within a microvascular network (in which blood flows from arteriole to capillary to venule) fluid shear stress (τ) (the frictional force of fluid flow along the luminal face of the vasculature and that is sensed by endothelial-expressed cell surface mechanoreceptors) is high in the arteriole and low in the venule. Transmural pressure (P), generated by the hydrostatic pressure imparted by fluid in the vessel, acts perpendicular to the endothelium.
Endothelial cells critically drive both the development and function of the blood vasculature (Garcia and Larina, 2014). During embryonic development, primordial endothelial cells coalesce into a primitive and lumenized vascular plexus (vasculogenesis), which subsequently expands by sprouting angiogenesis to establish new blood vessels in surrounding avascular tissue. Once blood vessels are established in the post-natal animal, vascular endothelium is highly specialized to perform key functions including to regulate blood flow distribution through local control of vessel tone; to mediate vascular-stromal exchange of oxygen, nutrients, and waste; and to regulate inflammation and immune cell responses. Under some physiological (e.g., menstruation) and pathological (e.g., cancer neovascularization, wet age-related macular degeneration, diabetes, etc.) conditions, the vascular endothelium may also reactivate pro-angiogenic signaling pathways to grow new blood vasculature.
As the interface between circulating blood and surrounding tissue, vascular endothelium is exposed to hemodynamic flow that exerts (and is thus sensed by primary mechanosensory cell surface receptors (Figure 1) as) mechanical friction forces (i.e., wall shear stress, WSS or τ) onto the lumen-facing (apical) surface of endothelial cells. Local WSS depends upon both vessel diameter and the flow characteristics of circulating blood. WSS can be calculated (Papaioannou and Stefanadis, 2005; Khankin et al., 2021) as:
In this equation, ƞ is blood viscosity, Q is fluid velocity, and r is vessel diameter—all are critical parameters that determine local WSS. Importantly, of these parameters, both fluid velocity and vessel diameter can vary significantly across the systemic circulation. While fluid velocity is directly correlated with shear, this equation underscores how even small changes in vessel diameter—such as what occurs in the hierarchal branching of the vascular tree—can produce large effects in WSS (Table 1). Endothelial cells of the aorta, for example, experience high-velocity pulsatile flow at magnitudes of ∼20 dynes/cm2 (∼2Pa) (Callaghan and Grieve, 2018). Pulsatile flow is defined as flow that oscillates between periods of high and low pressure, which corresponds to systole and diastole; flow pulsatility is quickly lost as the vessel wall dampens the pressure oscillations leading to steady downstream laminar flow. By contrast, WSS values for the microvessels are on average 40 dynes/cm2, but flow through these vessels is intermittent due to dynamic, moment-to-moment vasomotor changes that alter precapillary vessel diameter to control whether downstream capillary networks are perfused. Shearing forces are further compounded by the movement of circulating red blood cells as they squeeze through—and thus drag along the inner wall of—small caliber capillaries (Figure 1). Thus, capillary flow and WSS can vary by as much as 3–96 dynes/cm2 (0.3Pa–9.6Pa). Importantly, normal vascular WSS values are also species-specific: mice, for example, can experience WSS of ∼600 dynes/cm2 in the aorta, whereas such WSS forces are rarely found in humans (Suo et al., 2007).
WSS is a key biophysical signal for endothelial cells, and influences many aspects of vascular biology by altering endothelial cell shape, proliferation (Guo et al., 2007; Fang et al., 2017), migration (Hsu et al., 2001), gene expression and signaling activation (Guo et al., 2007; Fang et al., 2017; Chung et al., 2022; Mendez et al., 2022), and junctional permeability (Baeyens et al., 2016). During blood vessel development, WSS is a critical signal for proper vascular morphogenesis, and onset of systemic blood flow from the heart drives reorganization, remodeling, and mural cell recruitment to transform this plexus into a mature vascular network that includes arteries, capillaries, and veins (Lucitti et al., 2007; Garcia and Larina, 2014). For example, exposure of endothelial cells to arterial levels of flow drives expression of the arterial identity gene Cx40, and knockout of this gene leads to disrupted arteriogenesis (Buschmann et al., 2010; Fang et al., 2017). By contrast, vascular malformations in the congenital disease Hereditary Hemorrhagic Telangiectasia (HHT) occur primarily in high flow vessels (Larrivee et al., 2012), and appear to arise from defects in flow-sensitive endothelial cell migration (Jin et al., 2017). In post-natal vasculature, healthy vascular endothelial cell function remains tightly regulated by flow, especially in regard to vascular barrier function where flow induces profound changes in expression of junctional proteins [e.g., cadherins (Miao et al., 2005), gap junctions (Fang et al., 2017), and tight junctions (Yang et al., 2020)]. Flow also controls cell-cell signaling [e.g., Notch (Fang et al., 2017; Mack et al., 2017)], activates vasodilatory signals [e.g., eNOS (Sahni et al., 2023)], suppresses anti-inflammatory KLF2 signaling and increases production of circulating cytokines (Fledderus et al., 2007; Chen et al., 2021), and induces endothelial cell cycle arrest and specification (Fang et al., 2017). Abnormal WSS signaling is associated with endothelial cell dysfunction, and is a major contributor to the pathophysiology of vascular diseases such as atherosclerosis (Zhou et al., 2023). In the lymphatic circulation, WSS is similarly critical for lymphatic vascular development and function (Angeli and Lim, 2023).
Notably, endothelial cells are exposed to transmural blood-pressure mediated hydrostatic pressure (P, Figure 1) in addition to WSS. The innate pulsatile flow of blood throughout the cardiovascular system imparts a significant force against vascular walls. Like WSS, this additional pressure is a known modulator of endothelial cell behavior, both on the individual and tissue scales. Externally applied hydrostatic pressure in culture models has been shown to regulate endothelial cell proliferation, focal adhesion complexes, and integrin expression (Schwartz et al., 1999; Prystopiuk et al., 2018). Tuning the applied hydrostatic pressure to match known physiological ranges protects against barrier damage in endothelial perturbation studies (Muller-Marschhausen et al., 2008). Moreover, hydrostatic pressure has been shown to induce angiogenesis via YAP1 signaling in damaged lung tissues (Mammoto et al., 2022) and to promote vascular tube formation in vitro via Ras-ERK signaling (Yoshino et al., 2020). Hence, hydrostatic pressure in the vascular system is an important mechanical signal sensed by the endothelium in vivo and within the context of in vitro systems. Controlling and monitoring hydrostatic pressure should be considered along with applied shear stress in the design and implementation of vessel-on-a-chip systems. Technology for studying the effects of hydrostatic pressure relevant to vessel-on-a-chip systems include microfluidic culture devices with embedded pressure sensors designed to grant independent control of applied pressures and shear stresses (Liu et al., 2013). This review focuses on modeling and interrogating endothelial mechanosensing of shear forces associated with applied fluid flow.
Diversity in flow-sensitive signaling across endothelial cells
Endothelial cells exhibit distinct responses to the magnitude of WSS forces, indicating exquisite mechanosensing capabilities. Cleavage of the Notch intracellular domain, for example, is maximal in endothelial cells at 18 dynes/cm2, but reduced at either higher or lower shear, or under static conditions (Fang et al., 2017). By contrast, Smad1/5 phosphorylation occurs at low (1 dynes/cm2) shear stress, but this is suppressed at higher (3 dynes/cm2) shear (Mendez et al., 2022). In microfluidic channel models of the vasculature, only application of fluid flow above a minimum threshold induces angiogenic sprouting in an MMP1-dependent manner (Galie et al., 2014). Taken together, the findings that many mechanosensitive signals respond differently to different magnitudes of flow have led to the hypothesis that distinct endothelial cells maintain themselves at a specific fluid shear stress “setpoint” (Baeyens et al., 2015; Baeyens et al., 2016), and that this may be established in an organotypic and vessel-specific manner. Furthermore, the homeostatic regulation of setpoint signaling appears to be a key determinant for endothelial cell biology in health and disease (Baeyens et al., 2015), and may moreover differ across distinct endothelial cell subtypes. Indeed, recent next-generation sequencing analysis of the murine vasculature reveals profound heterogeneity in endothelial cell identity and gene expression (Kalucka et al., 2020), suggesting that fluid shear stress “setpoint” and mechanosensitive responses may also vary widely within an individual blood vessel, between blood vessels of different identity and caliber within a single network, and across distinct tissue-specific blood vasculatures. Lastly, heterogeneity of the endothelium is also heavily influenced by tissue-specific paracrine and ECM signals that function in concert with hemodynamic signals to confer local phenotypes (Aird, 2012; Gunawardana et al., 2021).
Several cell surface proteins have been reported to function as primary mechanosensors that sense and transduce WSS signals into biological cell signaling responses (Figure 1). Of these, the junctional complex comprised of VE-Cadherin/CD31/VEGFR2/3 (Tzima et al., 2005; Coon et al., 2015) has been well characterized and plays a critical role as a mechanosensor of WSS in endothelial cells. The non-selective Piezo1 cation channel (Hyman et al., 2017), Notch1 (Mack et al., 2017), S1PR1 (Cantalupo et al., 2017), and primary cilia structures have also been proposed to be flow mechanosensors (Luu et al., 2018), although how and under what circumstances these mechanosensory proteins and cell structures (individually and collectively) translate WSS signals to control endothelial cell biology in health and disease remains the subject of active research. The ability to precisely control and measure applied shear stress in vessel-on-a-chip systems can allow researchers to investigate shear sensing mechanisms in a physiologically relevant format using human cells. In summary, efforts to mimic the physiology of living blood vasculature in microphysiological systems will require careful consideration of applied flow and the resultant shear stresses that regulate endothelial cell forms and functions.
History of vessel-on-a-chip systems
Early efforts to harness microfluidic technologies for vascular engineering largely focused on integrating an artificially engineered circulatory system into bulk-engineered tissue constructs. Methods of patterning a template of channels included micromachining a series of channels in a rigid polymer bulk, or by manipulating the 3D architecture of more compliant extracellular matrix-derived substrates (Figure 2). In the latter approach, a sacrificial material (often made of sugar, alginate, or gelatin) was embedded within a bulk hydrogel in the pattern of the desired vascular structures. Subsequent dissolution of this matrix produced a network of perfusable channels to enable fluid circulation (Golden and Tien, 2007; Bellan et al., 2009; Norotte et al., 2009; Miller et al., 2012; Zervantonakis et al., 2012; Wang et al., 2014). Alternatively, photolithography-based methods could be used to generate micropatterned hydrogels with internal channels approximating vascular structures (Shevkoplyas et al., 2003; Moon et al., 2009; Du et al., 2011; Zheng et al., 2012) Further engineered efforts combined both micromachining and 3D matrix micromolding (Bertassoni et al., 2014). Overall, while such models were able to generate channel networks to perfuse bulk tissues, these printed channel designs were often not endothelialized and lacked many key biological characteristics such as structural heterogeneity of the native vasculature and the presence of stromal support cells.
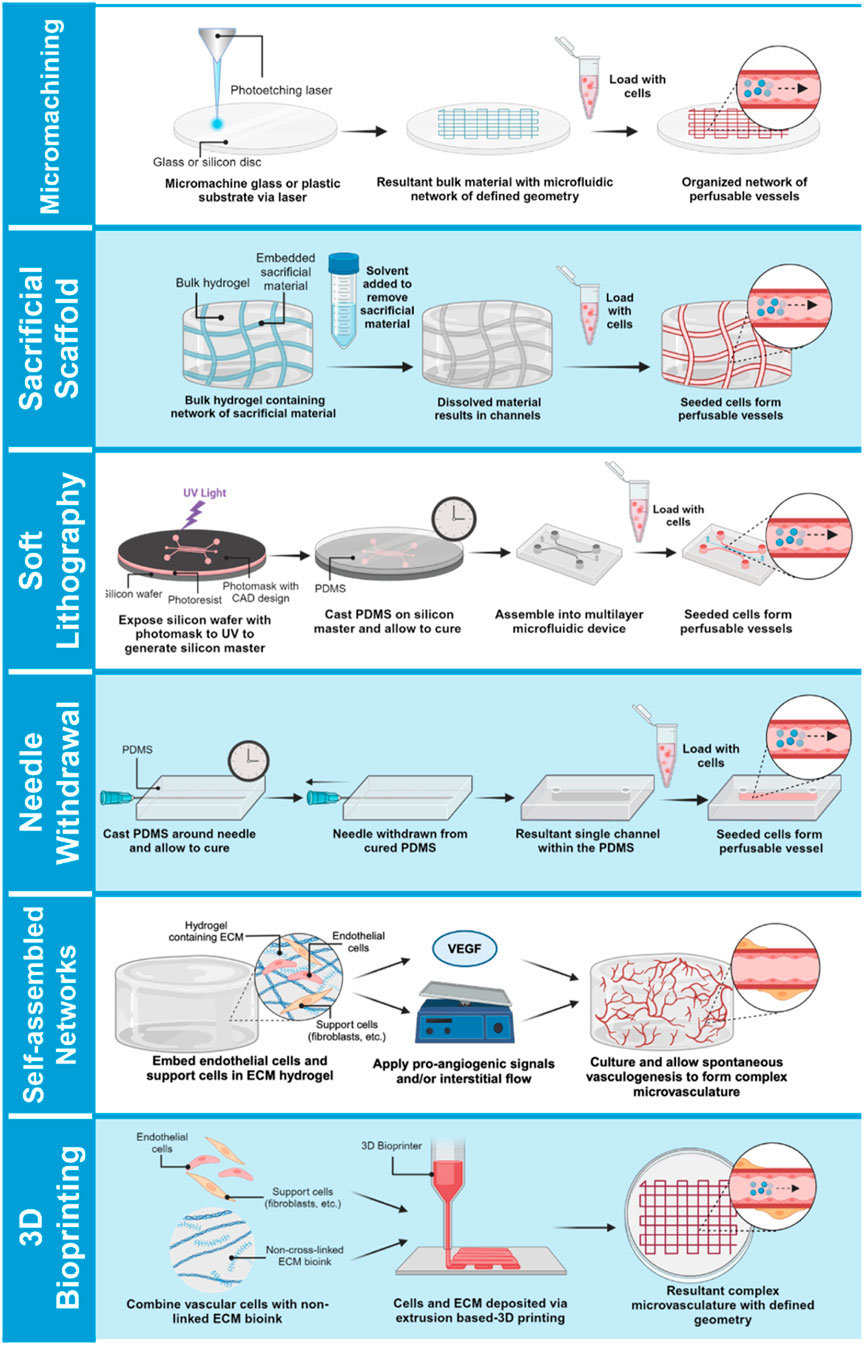
Figure 2. Approaches for bioengineering vessel-on-a-chip models. Micromachined or acellular gel-imprinted microfluidic channels circulate flow between distinct tissue compartments. Alternatively, complex microfluidic channel designs can be formed in a 3D hydrogel by soft lithography printing, or by a needle withdrawal method to generate a single-channel vessel-on-a-chip. Microvessel networks can be formed in organ chips either by embedding endothelial cells with support cells into a 3D hydrogel and inducing network self-assembly by application of interstitial flow, or vessel networks can be bioprinted onto a minimal scaffold, and then exposed to interstitial flow to promote lumenization.
In recent years, considerable efforts have been dedicated to engineering MPS models of the vasculature, either as a stand-alone vessel-on-a-chip platform or in the presence of organ-specific parenchymal cells (Torisawa et al., 2014; Huh, 2015; Blundell et al., 2016; Jang et al., 2019; Poceviciute and Ismagilov, 2019). Contemporary vessel-on-a-chip platforms vary widely in their design and ability to recapitulate aspects of the intact vascular niche (Table 2). A commonly used single vessel-on-a-chip platform involves fabrication of an extracellular matrix-comprised hydrogel cast within a mold fabricated from polydimethylsiloxane (PDMS) in the presence of a stainless steel needle or similar template (Figure 2). Subsequent withdrawal of the needle produces a templated channel that can then be seeded with endothelial cells to form a complete circumferential monolayer on the inner surface of the channel (Chrobak et al., 2006; Price et al., 2008; Seto et al., 2010; Wong et al., 2010; Sadr et al., 2011; Kusuma et al., 2013; Linville et al., 2016; Polacheck et al., 2017; Polacheck et al., 2019). This approach produces perfusable vessels as small as 160 µm in diameter (Polacheck et al., 2017; Polacheck et al., 2019)—within the range of small peripheral vessels in humans. Linville and colleagues showed that controlled patterns of interstitial pressure, stiffer hydrogel matrices, and high cAMP can enhance the generation of small diameter biomimetic vessels via this method (Linville et al., 2016). Hypothesis-driven studies using the needle withdrawal model have yielded insights into endothelial cell signaling mechanisms that control vessel barrier function (Wong et al., 2010; Polacheck et al., 2017; Polacheck et al., 2019). However, this vessel-on-a-chip is limited by difficulties in incorporating a well-organized outer mural cell layer. The single vessel format with constant diameter also lacks the morphology of fractal branching and tapered diameters that contribute to heterogenous flow patterns and gradients of WSS that are present in native vascular beds (Saqr et al., 2020).
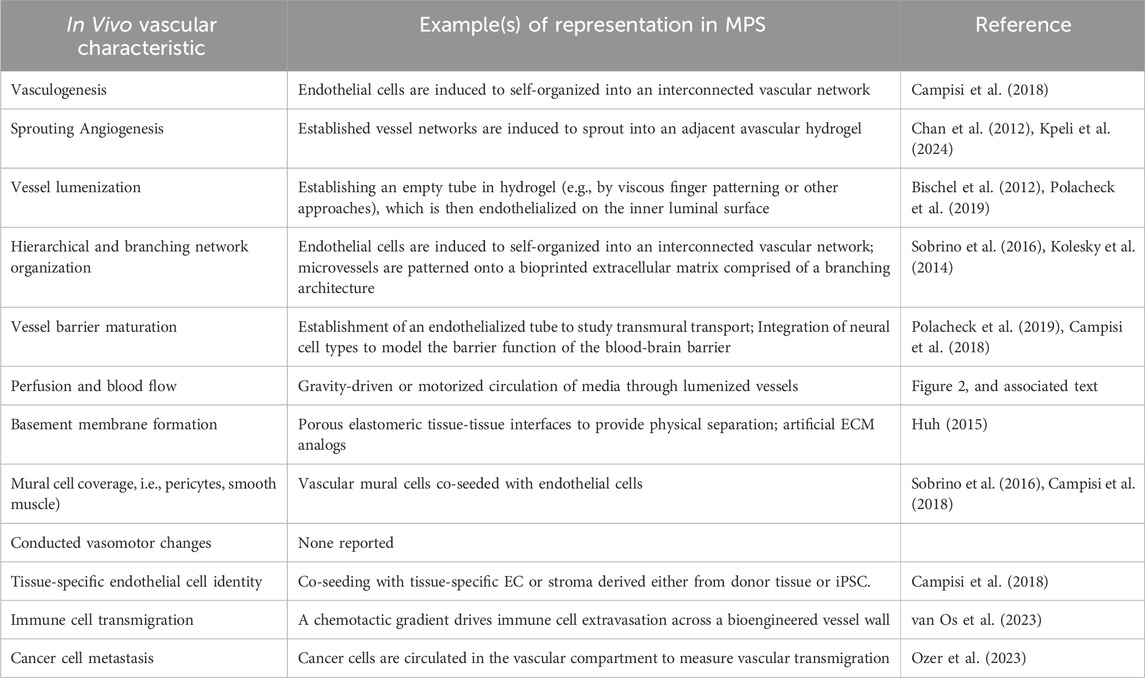
Table 2. Characteristics and Functions of In Vivo Vasculature and Examples of their Representation in MPS.
Organ chip models of entire vascular beds have been developed in attempts to create more biomimetic systems for investigating vascular physiology, drug delivery, and pathophysiological processes such as tumor cell metastasis (Haase et al., 2020; Del Piccolo et al., 2021; Chen et al., 2023) (Figure 1). In brief, human donor-derived (i.e., primary or iPSC-derived) endothelial and vascular support cells (e.g., fibroblasts, pericytes, vascular smooth muscle) are co-seeded in extracellular matrix hydrogels loaded in organ chip devices. These organ chips typically entail a “3-lane” design in which a central channel loaded with the 3D hydrogel mixture containing vascular cells is flanked by side channels that can be seeded with a confluent endothelial layer to aid anastomosis with the internal bulk vasculature (Wang et al., 2016). Various approaches have been reported to enhance anastomosis, such as the subsequent application of pro-angiogenic signals (e.g., exogenous VEGF) and/or interstitial flow that drives cytoskeletal rearrangement and increases nitric oxide (NO) synthesis, (Kim et al., 2016). Collectively, numerous approaches can facilitate the self-organization of vascular cells into a patent and perfusable microvascular bed, although defining the mechanism of anastomosis to achieve greater control will require further investigation. Most importantly, the resultant models exhibit organotypic vascular organization (i.e., appropriate lumen size, branching architecture, mural cell wrapping) and grant the ability to precisely control biophysical and biochemical signals for tuned mimicry of in vivo blood vessels (Nakatsu and Hughes, 2008; Newman et al., 2011; Chan et al., 2012; Kim et al., 2013). Reports of microvasculature-on-a-chip models outperforming conventional 2D and 3D models in their recapitulation of in vivo drug responses are a testament to the translational potential of these platforms (Hachey et al., 2021; Hachey et al., 2024).
Organ chip models of vascular beds that rely on vascular cell self-organization may be difficult to standardize due to variations in cell sourcing, purity, and quality. Recently, some groups have incorporated cutting-edge 3D bioprinting approaches to circumvent this requirement by artificially depositing vascular cells into pre-determined architectures within an extracellular matrix (Kolesky et al., 2014; Gao et al., 2017; Zhang G. et al., 2020; Zhang et al., 2021; Orellano et al., 2022; Zhang et al., 2022), and these models are capable of generating perfused vascular networks supported by minimal scaffolding material (Kolesky et al., 2014; Lee et al., 2014; Jia et al., 2016; Cao et al., 2019; Freeman et al., 2019; Gold et al., 2021). Unlike other models described thus far, bioprinted vessel characteristics are dependent on the mechanical properties of both the bioinks used as well as the surrounding hydrogel scaffold. For example, changing flow rate and print-head speed affects resulting bioprinted vessel lumen size (Attalla et al., 2016). Inclusion of solid frames within the hydrogel scaffold produces tensile forces that also influence vessel morphology (Zhang G. et al., 2020; Zhang et al., 2021; Zhang et al., 2022). While bioprinted platforms have the potential to precisely control 3D architecture, the complexity and technical demands of the approach currently limits it is broad adoption by basic scientists and preclinical researchers. Organ chip models and MPS allow researchers to utilize familiar 2D and 3D cell culture methods using fluidic device platforms with increasing commercial availability as “off the shelf” products to a wide-range of end users (Zhang and Radisic, 2017).
As with all MPS, engineering organ-specific vessel-on-a-chip models require precise control of cell type, microtissue architecture, extracellular matrix composition, and mechanical forces such as substrate stiffness and the shear stress applied by microfluidic flow. Importantly, these parameters are key signals for microvessel-on-a-chip network formation—which underscores the importance of these signals in the function of native vasculature—and are critical for capturing endothelial cell physiology in an in vitro setting. In a recent study by Hatch et al. to develop a COVID-on-a-chip vascular model, for example, endothelial cell expression of SARS-CoV2 target ACE2 was reported to be flow-dependent, and a SARS-CoV2 pseudovirus failed to efficiently infect primary endothelial cells except within a vessel-on-a-chip platform under flow (Hatch et al., 2024).
Optimization of chip biomaterials, organotypic cell combinations, extracellular matrix composition is a unique tissue engineering problem within each specific organ chip application. Most organ chips are fabricated out of optically transparent, biocompatible elastomers—most commonly PDMS. Pure PDMS has a relatively low elastic modulus (1.3–3 MPa) and tensile strength (3.5–5.1 MPa) (Ariati et al., 2021), meaning that it is a relatively soft and elastic elastomer when compared to other inorganic materials. Furthermore, the mechanical properties of PDMS can be controlled either by altering the curing conditions of pure PDMS or by including fillers to create PDMS composites that can vary widely in their mechanical properties (Ariati et al., 2021). Nonetheless, pure PDMS is inherently less elastic and more stiff than biological tissues (including intact vessel wall), and most PDMS composites increase—not decrease—these properties (Ebrahimi, 2009). The difference in PDMS stiffness relative to intact basement membrane will affect the resulting shear stress that is generated by fluid flow and sensed by endothelial cells—particularly with regard to organ chips in which endothelial cells are seeded directly onto PDMS-based (or other elastomeric) surfaces and where the PDMS provides direct mechanical support for the engineered endothelium.
Alternatively, blood vessels can be generated within extracellular matrix hydrogels seeded into microfluidic tissue compartments, such that the PDMS of the tissue chamber is not directly in contact with engineered blood vessels and is not directly contributing to mechanical wall stiffness. In these types of vessel-on-a-chip designs, the elastic modulus of vascular mural cells and surrounding hydrogel typically falls within the compliant range of soft tissues—i.e., 1 kPa or less—providing a more physiologically relevant microenvironment that better approximates the mechanical properties of intact blood vasculature. As has been previously shown to be the case in simpler 3D angiogenesis models (Newman et al., 2011), the presence of fibroblasts is essential for network formation. In the absence of perivascular support cells, networks fail to form or regress rapidly (Nakatsu et al., 2003; Newman et al., 2011; Whisler et al., 2014). More research is needed to determine the optimal types and ratios of support cells to produce coveted organ-specific endothelial phenotypes. Extracellular matrix protein composition and density also affects vascular network formation and maturation, likely through combined effects on cell-matrix signaling and matrix stiffness. For example, increased fibrinogen concentration increases the average diameter of resulting vessels (Whisler et al., 2014), and extent of gelatin methacrylate methylation dictates vessel outgrowth potential and vasculogenesis (Chen et al., 2012). Externally applied mechanical load alone also controls vessel architecture (Krishnan et al., 2008; Rosenfeld et al., 2016), suggesting that both cell-matrix signaling and mechanical properties of the extracellular space contribute to vascular morphogenesis in these models.
In summary, MPS research has delivered the foundation for accurate biomimicry of vascular beds, but future efforts will be needed to develop and implement protocols for the creation of organ-specific models of healthy and diseased vasculature.
Integrating physiological fluid flow into vessel-on-a-chip platforms
Of greatest relevance to this review, vessel-on-a-chip models enable study of bioengineered blood vessels under controlled flow, which promises to significantly advance our understanding of how hemodynamic flow contributes to healthy and diseased vascular biology in intact blood vessels. Thus, vessel-on-a-chip design and implementation demands careful attention to the method of introducing circulating flow, which entails a balance between ease of use and the degree to which researchers wish to mimic the in vivo milieu for a given application. This is especially important when considered in the context of the aforementioned “fluid shear setpoint” hypothesis advanced by Baeyens et al. (Baeyens et al., 2016). There is also inherent applied hydrostatic pressure that will vary with the total liquid volume, reservoir height, and layout of the fluidic channel circuit in each vessel-on-a-chip design. Within replicates of a single design exposed to the same fluid flow conditions, the applied hydrostatic pressure is relatively uniform, which allows for isolation of applied shear stress as an experimental variable by varying the flow rate (Alonzo et al., 2015; Lam et al., 2018). Altered hydrostatic pressure should be considered when moving between vessel chip platforms as changes to system parameters such as channel geometry, total liquid volume, and method of generating flow will impart varying hydrostatic pressure profiles.
Varying WSS experienced by endothelial cells in a vessel-on-a-chip platform is achieved via control of applied fluid flow. The primary strategies for controlling fluid flow in vessel-on-a-chip systems include: 1) motorized pumping, 2) motorized rocking of the platform to reset gravity-driven flow, or 3) non-motorized gravity-driven flow with manual reset (Figure 3). Motorized syringe pumps have the advantage of being able to readily achieve high circulating fluid velocity (and thus, correspondingly higher levels of WSS within the ranges seen in vivo, Table 1). In addition, motorized pumps (unlike gravity-driven models) allow users to program defined patterns of continuous, oscillatory, and/or pulsatile flow, which may be critical if modeling blood vessel structures in which complex flow profiles are typically observed in vivo. However, pump-based systems are mechanically complex, and require numerous accessory components to maintain flow through the pump system. This significantly increases the required volume of circulating media, limits the number of devices that can be run in parallel, and introduces points of potential device failure through fluid leak or microbial contamination. While syringe pumps are often used to provide programmable flow control, peristaltic pumps have become an attractive alternative that offer increased simplicity, lowered cost, and decreased risk of contamination due to the closed nature of recirculating peristaltic systems (Abello et al., 2022; Mohammed et al., 2019; Schneider et al., 2021; Zhang X. et al., 2020; O’Grady et al., 2018). A limitation of such pumps is the inherently pulsatile nature of the produced fluid flow. It is well documented that endothelial cells respond differentially to laminar versus pulsatile flow (Eskin et al., 1984; Helmlinger et al., 1991; Miao et al., 2005; Guo et al., 2007; Mohammed et al., 2019; Liu et al., 2021), thus pulsatile flow patterns will not impart physiological shear forces to small vessels which naturally feature continuous laminar flow in intact microvasculature. Efforts have been made to generate laminar flow via peristaltic pumps using pulse dampeners (Voyvodic et al., 2012; Pech et al., 2020; Abello et al., 2022). The transition from pulsatile flow in the macrovasculature to continuous flow in the microvascular beds in vivo should be considered when choosing a mechanical pumping approach for specific vessel-on-a-chip applications.
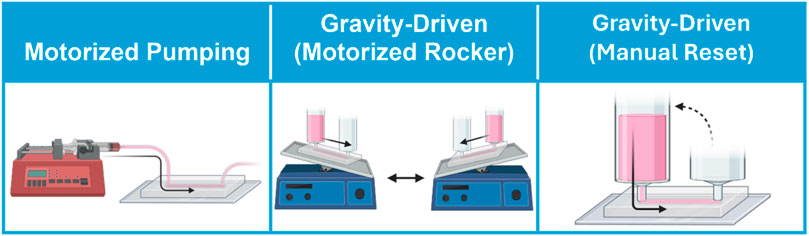
Figure 3. Approaches for circulating flow on vessel-on-a-chip models. Flow is circulated through vessel-on-a-chip platform through motorized pumping, or by gravity-driven flow in which motorized rockers automate hydrostatic pressure reset, or by manual pressure head reset.
On the other hand, pumpless gravity-driven models are mechanically simpler, and thus potentially easier to scale in studies in which large numbers of devices must be run in parallel (Figure 3). Nonetheless, gravity-driven models also have unique drawbacks. Gravity-driven flow depends upon the hydrostatic pressure gradient generated between two coupled media reservoirs. Thus, gravity-driven flow is initially high when the hydrostatic pressure gradient is largest, but this driving force decreases over time as the volumes of the two reservoirs equilibrate. Not only does this produce a changing flow profile over time, but also necessitates frequent reset of the hydrostatic pressure heads within the two media reservoirs to maintain flow through the system. This process can be performed manually through pipetting, or can be automated using a motorized rocker. Of these two strategies, motorized rockers typically produce bi-directional (i.e., alternating flow direction) gravity-driven fluid circulation, which does not accurately reflect the unidirectionality of blood flow in the intact vasculature. A recent pumpless gravity-driven system corrects this problem using a platform with additional supporting channels and valve features to create continuous unidirectional flow with rocking, although it is not yet in widespread use (Wang and Shuler, 2018). Alternatively, hydrostatic pressure heads can be manually reset through pipetting. While manual pipetting can be tedious, time-consuming, and inconsistent, it is likely that automated robotic pipetting will solve this problem for high-throughput applications, particularly in industrial settings (Langer and Joensson, 2020).
In addition to the above challenges, gravity-driven strategies are also typically limited by media reservoir size, which physically constrains the size of the hydrostatic pressure gradient that can be generated by this approach. As a result, it can be challenging to produce sufficiently high fluid velocity to capture high fluid flow and WSS using gravity-driven approaches. Since WSS depends heavily on vessel diameter, pump-driven models in which high fluid flow rates are introduced in large-diameter vessel compartments, the high fluid velocity may still be insufficient to produce physiological levels of WSS in some models. By contrast, the small vessel sizes (e.g., 25–30 µm in diameter) (Fang et al., 2024) that are often achievable in self-assembled microvasculature-on-a-chip models can bring even gravity-driven models into physiological ranges of WSS (Fang et al., 2024). Precise control of hemodynamic flow, regardless of the mode in which it is applied, in vessel-on-a-chip models is also constrained by the lack of widely adaptable tools for the study of real-time fluid flow. Precise measurement of flow rates and local fluid velocities within a 3D vascular architecture will be necessary for robust quality control and precise determination of WSS effects in functional assays. Fluid velocity can be measured in vessel-on-a-chip models by particle velocimetry, i.e., time-lapse imaging of perfused fluorescent particles from which particle trajectories and velocities can be used to calculate flow patterns and estimate applied WSS based on defined vessel geometry (Pitts and Fenech, 2013). Alternatively, fluid velocity and WSS can be estimated using in silico modeling based on the size and shape of bioengineered vessels (Fang et al., 2024; Hatch et al., 2024) using approaches similar to have been previously applied in intact tissue (Bernabeu et al., 2014), but this still presupposes that the pumping or rocking method used generates the desired flow rates within an engineered vessel or vascular bed. Empirical and computational approaches to quantify applied WSS will likely be combined in industrial settings, but these strategies currently present challenges to implementation in most research labs that use vessel-on-a-chip systems. Developing new tools for adaptable and scalable automation of real-time flow monitoring in MPS with complex 3D vasculature will enable modeling of hemodynamics in vitro with unprecedented biomimicry.
Another issue for vessel-on-a-chip models is endothelial cell source, particularly with regard to capturing tissue-specific endothelial cell mechanosensitive signaling. Early vessel-on-a-chip models including many described in this review rely on “generic” primary human endothelial cells selected for their ease-of-use and non-specific endothelial cell identity (e.g., human umbilical vein endothelial cells (HUVEC); circulating endothelial progenitor cells (EPC), etc.), with the idea that these “generic” endothelial cells would acquire more specialized identities when co-seeded with tissue-specific stromal cells in organ chip platforms. However, with recent studies further underscoring the broad heterogeneity of vascular endothelial cells (Kalucka et al., 2020)—with likely important implications for heterogeneity in mechanosensitive signaling—recent organ chip work has increasingly focused on integrating tissue-specific primary endothelial cells into organ chip settings, with these endothelial cells either isolated directly from tissue-specific vascular beds (e.g. (Park et al., 2019)) or generated from iPSC (e.g. (Vatine et al., 2019)). These latest efforts are more likely to recapitulate tissue-specific flow-sensitive signaling relative to platforms that were developed using “generic” endothelial cells. However, tissue-specific endothelial cells may lose their specialized endothelial cell identities with time in culture, and/or may have compromised vessel self-assembly properties that may limit their integration into platforms that rely upon this property to form vessel-on-a-chip networks. Ongoing work in the field is focused on addressing these various challenges.
A final consideration when incorporating physiological flow into vessel-on-a-chip platforms is the composition of the circulating media. Current vessel-on-a-chip models typically circulate growth factor-rich endothelial growth medium. However, endothelial growth medium often contains higher levels of pro-angiogenic growth factors and other supplements than is typically found in circulating blood. This alone would be hypothesized to drive endothelial cells in vessel-on-a-chip platforms towards a more activated, pro-inflammatory, and pro-angiogenic state more representative of disease, whereas endothelial cells in established and healthy (i.e., uninjured) blood vessels are typically non-proliferative and quiescent (Langille et al., 1986). In addition, the cell culture media typically circulated through vessel-on-a-chip models lacks the higher viscosity of blood and colloidal properties imparted by circulating blood cells—both properties that greatly influence vascular WSS experienced in vivo. Additional work remains to be done to improve our current “blood surrogates” to better mimic the biophysical and biochemical properties of in vivo blood (Marx et al., 2016). This will in turn further enhance the physiological relevance of circulating fluid flow in vessel-on-a-chip platforms to better recapitulate the effect of hemodynamic forces on intact vasculature.
Conclusion
Vascularized MPS (i.e., vessel-on-a-chip systems) are powerful in vitro models that enable precise control of cell type composition, 3D tissue architecture and layering, extracellular matrix composition, and intravascular fluid flow that regulates biotransport processes and mechanical forces such as applied shear stresses. Current vessel-on-a-chip systems take varied approaches to model the in vivo vascular niche, and have successfully recapitulated many of the hallmarks of intact blood vessels that remain elusive in conventional 2D cell culture, while still retaining many of the ease-of-use advantages of in vitro experimentation. Balancing complexity and ease-of-use by the widest range of possible end users will be a critical dimension of efforts to maximize translational impact of vessel-on-a-chip technologies. Studies using vessel-on-a-chip models have already produced novel insights into the role of fluid flow and WSS as key signals in vascular health and disease. However, efforts to fully capture the physiological hemodynamics in vascularized MPS remain challenging due to engineering considerations such as controlling and monitoring intravascular fluid flow. Future vessel-on-a-chip platforms must continue to consider how important parameters that determine WSS—including vessel diameter, flow velocity, and fluid viscosity—are represented in their chip designs to ensure that resulting on-chip flow fully captures the biomechanical properties of circulating blood and its corresponding signaling to regulate vascular physiology and function.
Author contributions
AM: Visualization, Writing–review and editing, Writing–original draft, Conceptualization. ZC: Writing–review and editing. HS: Writing–review and editing. MM: Writing–review and editing, Writing–original draft, Funding acquisition, Conceptualization. JF: Writing–review and editing, Writing–original draft, Visualization, Funding acquisition, Conceptualization.
Funding
The author(s) declare that financial support was received for the research, authorship, and/or publication of this article. This work was funded in part by a grant from the Eye, Ear, Nose and Throat Foundation of New Orleans (EE221102 to JF and MM).
Acknowledgments
The authors would like to acknowledge members of the Fang and Mondrinos labs for their collegiality and scientific discussions.
Conflict of interest
The authors declare that the research was conducted in the absence of any commercial or financial relationships that could be construed as a potential conflict of interest.
Publisher’s note
All claims expressed in this article are solely those of the authors and do not necessarily represent those of their affiliated organizations, or those of the publisher, the editors and the reviewers. Any product that may be evaluated in this article, or claim that may be made by its manufacturer, is not guaranteed or endorsed by the publisher.
References
Abello J., Raghavan S., Yien Y. Y., Stratman A. N. (2022). Peristaltic pumps adapted for laminar flow experiments enhance in vitro modeling of vascular cell behavior. J. Biol. Chem. 298, 102404. doi:10.1016/j.jbc.2022.102404
Adamson R. H., Lenz J. F., Curry F. E. (1994). Quantitative laser scanning confocal microscopy on single capillaries: permeability measurement. Microcirculation 1, 251–265. doi:10.3109/10739689409146752
Aird W. C. (2012). Endothelial cell heterogeneity. Cold Spring Harb. Perspect. Med. 2, a006429. doi:10.1101/cshperspect.a006429
Alonzo L. F., Moya M. L., Shirure V. S., George S. C. (2015). Microfluidic device to control interstitial flow-mediated homotypic and heterotypic cellular communication. Lab. Chip 15, 3521–3529. doi:10.1039/c5lc00507h
Angeli V., Lim H. Y. (2023). Biomechanical control of lymphatic vessel physiology and functions. Cell Mol. Immunol. 20, 1051–1062. doi:10.1038/s41423-023-01042-9
Ariati R., Sales F., Souza A., Lima R. A., Ribeiro J. (2021). Polydimethylsiloxane composites characterization and its applications: a review. Polym. (Basel) 13, 4258. doi:10.3390/polym13234258
Attalla R., Ling C., Selvaganapathy P. (2016). Fabrication and characterization of gels with integrated channels using 3D printing with microfluidic nozzle for tissue engineering applications. Biomed. Microdevices 18, 17–12. doi:10.1007/s10544-016-0042-6
Augustin H. G., Koh G. Y. (2017). Organotypic vasculature: from descriptive heterogeneity to functional pathophysiology. Science 357, eaal2379. doi:10.1126/science.aal2379
Baeyens N., Bandyopadhyay C., Coon B. G., Yun S., Schwartz M. A. (2016). Endothelial fluid shear stress sensing in vascular health and disease. J. Clin. Invest. 126, 821–828. doi:10.1172/JCI83083
Baeyens N., Nicoli S., Coon B. G., Ross T. D., Van den Dries K., Han J., et al. (2015). Vascular remodeling is governed by a VEGFR3-dependent fluid shear stress set point. Elife 4, e04645. doi:10.7554/eLife.04645
Ballermann B. J., Dardik A., Eng E., Liu A. (1998). Shear stress and the endothelium. Kidney Int. Suppl. 67, S100–S108. doi:10.1046/j.1523-1755.1998.06720.x
Bellan L. M., Singh S. P., Henderson P. W., Porri T. J., Craighead H. G., Spector J. A. (2009). Fabrication of an artificial 3-dimensional vascular network using sacrificial sugar structures. Soft Matter 5, 1354–1357. doi:10.1039/b819905a
Bender R. H. F., O’Donnell B. T., Shergill B., Pham B. Q., Tahmouresie S., Sanchez C. N., et al. (2024). A vascularized 3D model of the human pancreatic islet forex vivostudy of immune cell-islet interaction. Biofabrication 16, 025001. doi:10.1088/1758-5090/ad17d0
Bernabeu M. O., Jones M. L., Nielsen J. H., Kruger T., Nash R. W., Groen D., et al. (2014). Computer simulations reveal complex distribution of haemodynamic forces in a mouse retina model of angiogenesis. J. R. Soc. Interface 11, 20140543. doi:10.1098/rsif.2014.0543
Bertassoni L. E., Cecconi M., Manoharan V., Nikkhah M., Hjortnaes J., Cristino A. L., et al. (2014). Hydrogel bioprinted microchannel networks for vascularization of tissue engineering constructs. Lab. Chip 14, 2202–2211. doi:10.1039/c4lc00030g
Bhatia S. N., Ingber D. E. (2014). Microfluidic organs-on-chips. Nat. Biotechnol. 32, 760–772. doi:10.1038/nbt.2989
Bischel L. L., Lee S. H., Beebe D. J. (2012). A practical method for patterning lumens through ECM hydrogels via viscous finger patterning. J. Lab. Autom. 17, 96–103. doi:10.1177/2211068211426694
Blundell C., Tess E. R., Schanzer A. S., Coutifaris C., Su E. J., Parry S., et al. (2016). A microphysiological model of the human placental barrier. Lab. Chip 16, 3065–3073. doi:10.1039/c6lc00259e
Buschmann I., Pries A., Styp-Rekowska B., Hillmeister P., Loufrani L., Henrion D., et al. (2010). Pulsatile shear and Gja5 modulate arterial identity and remodeling events during flow-driven arteriogenesis. Development 137, 2187–2196. doi:10.1242/dev.045351
Callaghan F. M., Grieve S. M. (2018). Normal patterns of thoracic aortic wall shear stress measured using four-dimensional flow MRI in a large population. Am. J. Physiol. Heart Circ. Physiol. 315, H1174–H1181. doi:10.1152/ajpheart.00017.2018
Campisi M., Shin Y., Osaki T., Hajal C., Chiono V., Kamm R. D. (2018). 3D self-organized microvascular model of the human blood-brain barrier with endothelial cells, pericytes and astrocytes. Biomaterials 180, 117–129. doi:10.1016/j.biomaterials.2018.07.014
Cantalupo A., Gargiulo A., Dautaj E., Liu C., Zhang Y., Hla T., et al. (2017). S1PR1 (Sphingosine-1-Phosphate receptor 1) signaling regulates blood flow and pressure. Hypertension 70, 426–434. doi:10.1161/HYPERTENSIONAHA.117.09088
Cao X., Ashfaq R., Cheng F., Maharjan S., Li J., Ying G., et al. (2019). A tumor-on-a-chip system with bioprinted blood and lymphatic vessel pair. Adv. Funct. Mater. 29, 1807173. doi:10.1002/adfm.201807173
Chan J. M., Zervantonakis I. K., Rimchala T., Polacheck W. J., Whisler J., Kamm R. D. (2012). Engineering of in vitro 3D capillary beds by self-directed angiogenic sprouting. PLoS One 7, e50582. doi:10.1371/journal.pone.0050582
Chatterjee S. (2018). Endothelial mechanotransduction, redox signaling and the regulation of vascular inflammatory pathways. Front. Physiol. 9, 524. doi:10.3389/fphys.2018.00524
Chen C., Chen J., Tao X., Fu M., Cheng B., Chen X. (2021). Activation of GPR30 with G1 inhibits oscillatory shear stress-induced adhesion of THP-1 monocytes to HAECs by increasing KLF2. Aging (Albany NY) 13, 11942–11953. doi:10.18632/aging.202897
Chen M. B., Whisler J. A., Fröse J., Yu C., Shin Y., Kamm R. D. (2017). On-chip human microvasculature assay for visualization and quantification of tumor cell extravasation dynamics. Nat. Protoc. 12, 865–880. doi:10.1038/nprot.2017.018
Chen S. W., Blazeski A., Zhang S., Shelton S. E., Offeddu G. S., Kamm R. D. (2023). Development of a perfusable, hierarchical microvasculature-on-a-chip model. Lab. Chip 23, 4552–4564. doi:10.1039/d3lc00512g
Chen Y.-C., Lin R.-Z., Qi H., Yang Y., Bae H., Melero-Martin J. M., et al. (2012). Functional human vascular network generated in photocrosslinkable gelatin methacrylate hydrogels. Adv. Funct. Mater. 22, 2027–2039. doi:10.1002/adfm.201101662
Chrobak K. M., Potter D. R., Tien J. (2006). Formation of perfused, functional microvascular tubes in vitro. Microvasc. Res. 71, 185–196. doi:10.1016/j.mvr.2006.02.005
Chung J., Kim K. H., Yu N., An S. H., Lee S., Kwon K. (2022). Fluid shear stress regulates the landscape of microRNAs in endothelial cell-derived small extracellular vesicles and modulates the function of endothelial cells. Int. J. Mol. Sci. 23, 1314. doi:10.3390/ijms23031314
Cochrane A., Albers H. J., Passier R., Mummery C. L., van den Berg A., Orlova V. V., et al. (2019). Advanced in vitro models of vascular biology: human induced pluripotent stem cells and organ-on-chip technology. Adv. Drug Deliv. Rev. 140, 68–77. doi:10.1016/j.addr.2018.06.007
Coon B. G., Baeyens N., Han J., Budatha M., Ross T. D., Fang J. S., et al. (2015). Intramembrane binding of VE-cadherin to VEGFR2 and VEGFR3 assembles the endothelial mechanosensory complex. J. Cell Biol. 208, 975–986. doi:10.1083/jcb.201408103
Del Piccolo N., Shirure V. S., Bi Y., Goedegebuure S. P., Gholami S., Hughes C. C. W., et al. (2021). Tumor-on-chip modeling of organ-specific cancer and metastasis. Adv. Drug Deliv. Rev. 175, 113798. doi:10.1016/j.addr.2021.05.008
Du Y., Ghodousi M., Qi H., Haas N., Xiao W., Khademhosseini A. (2011). Sequential assembly of cell-laden hydrogel constructs to engineer vascular-like microchannels. Biotechnol. Bioeng. 108, 1693–1703. doi:10.1002/bit.23102
Ebrahimi A. P. (2009). Mechanical properties of normal and diseased cerebrovascular system. J. Vasc. Interv. Neurol. 2, 155–162.
Edington C. D., Chen W. L. K., Geishecker E., Kassis T., Soenksen L. R., Bhushan B. M., et al. (2018). Interconnected microphysiological systems for quantitative biology and pharmacology studies. Sci. Rep. 8, 4530. doi:10.1038/s41598-018-22749-0
Eskin S. G., Ives C. L., McIntire L. V., Navarro L. T. (1984). Response of cultured endothelial cells to steady flow. Microvasc. Res. 28, 87–94. doi:10.1016/0026-2862(84)90031-1
Ewald M. L., Chen Y. H., Lee A. P., Hughes C. C. W. (2021). The vascular niche in next generation microphysiological systems. Lab. Chip 21, 3244–3262. doi:10.1039/d1lc00530h
Fang J. S., Coon B. G., Gillis N., Chen Z., Qiu J., Chittenden T. W., et al. (2017). Shear-induced Notch-Cx37-p27 axis arrests endothelial cell cycle to enable arterial specification. Nat. Commun. 8, 2149. doi:10.1038/s41467-017-01742-7
Fang J. S., Hatch C. J., Andrejecsk J., van Trigt W., Juat D. J., Chen Y.-H., et al. (2024). A microphysiological HHT-on-a-chip platform recapitulates patient vascular lesions. bioRxiv. doi:10.21203/rs.3.rs-4578507/v1
Fledderus J. O., van Thienen J. V., Boon R. A., Dekker R. J., Rohlena J., Volger O. L., et al. (2007). Prolonged shear stress and KLF2 suppress constitutive proinflammatory transcription through inhibition of ATF2. Blood 109, 4249–4257. doi:10.1182/blood-2006-07-036020
Freeman S., Ramos R., Alexis Chando P., Zhou L., Reeser K., Jin S., et al. (2019). A bioink blend for rotary 3D bioprinting tissue engineered small-diameter vascular constructs. Acta Biomater. 95, 152–164. doi:10.1016/j.actbio.2019.06.052
Galie P. A., Nguyen D. H., Choi C. K., Cohen D. M., Janmey P. A., Chen C. S. (2014). Fluid shear stress threshold regulates angiogenic sprouting. Proc. Natl. Acad. Sci. U. S. A. 111, 7968–7973. doi:10.1073/pnas.1310842111
Gao Q., Liu Z., Lin Z., Qiu J., Liu Y., Liu A., et al. (2017). 3D bioprinting of vessel-like structures with multilevel fluidic channels. ACS Biomaterials Sci. Eng. 3, 399–408. doi:10.1021/acsbiomaterials.6b00643
Garcia M. D., Larina I. V. (2014). Vascular development and hemodynamic force in the mouse yolk sac. Front. Physiol. 5, 308. doi:10.3389/fphys.2014.00308
Gold K. A., Saha B., Rajeeva Pandian N. K., Walther B. K., Palma J. A., Jo J., et al. (2021). 3D bioprinted multicellular vascular models. Adv. Healthc. Mater. 10, 2101141. doi:10.1002/adhm.202101141
Golden A. P., Tien J. (2007). Fabrication of microfluidic hydrogels using molded gelatin as a sacrificial element. Lab. Chip 7, 720–725. doi:10.1039/b618409j
Gunawardana H., Romero T., Yao N., Heidt S., Mulder A., Elashoff D. A., et al. (2021). Tissue-specific endothelial cell heterogeneity contributes to unequal inflammatory responses. Sci. Rep. 11, 1949. doi:10.1038/s41598-020-80102-w
Guo D., Chien S., Shyy J. Y. (2007). Regulation of endothelial cell cycle by laminar versus oscillatory flow: distinct modes of interactions of AMP-activated protein kinase and Akt pathways. Circ. Res. 100, 564–571. doi:10.1161/01.RES.0000259561.23876.c5
Haas M. J., Feng V., Gonzales K., Onstead-Haas L., Mooradian A. D. (2020). High-throughput analysis identifying drugs that reduce oxidative and ER stress in human coronary artery endothelial cells. Eur. J. Pharmacol. 879, 173119. doi:10.1016/j.ejphar.2020.173119
Haase K., Offeddu G. S., Gillrie M. R., Kamm R. D. (2020). Endothelial regulation of drug transport in a 3D vascularized tumor model. Adv. Funct. Mater 30, 2002444. doi:10.1002/adfm.202002444
Hachey S. J., Hatch C. J., Gaebler D., Mocherla A., Nee K., Kessenbrock K., et al. (2024). Targeting tumor-stromal interactions in triple-negative breast cancer using a human vascularized micro-tumor model. Breast Cancer Res. 26, 5. doi:10.1186/s13058-023-01760-y
Hachey S. J., Movsesyan S., Nguyen Q. H., Burton-Sojo G., Tankanzyan A., Wu J., et al. (2021). Anin vitro vascularized micro-tumor model of human colorectal cancer recapitulates in vivo responses to standard-of-care therapy. Lab. Chip 21, 1333–1351. doi:10.1039/d0lc01216e
Hatch C. J., Piombo S. D., Fang J. S., Gach J. S., Ewald M. L., Van Trigt W. K., et al. (2024). SARS-CoV-2 infection of endothelial cells, dependent on flow-induced ACE2 expression, drives hypercytokinemia in a vascularized microphysiological system. Front. Cardiovasc Med. 11, 1360364. doi:10.3389/fcvm.2024.1360364
Heberlein K. R., Straub A. C., Isakson B. E. (2009). The myoendothelial junction: breaking through the matrix? Microcirculation 16, 307–322. doi:10.1080/10739680902744404
Helmlinger G., Geiger R. V., Schreck S., Nerem R. M. (1991). Effects of pulsatile flow on cultured vascular endothelial cell morphology. J. Biomech. Eng. 113, 123–131. doi:10.1115/1.2891226
Hosseinzadegan H., Tafti D. K. (2017). Modeling thrombus formation and growth. Biotechnol. Bioeng. 114, 2154–2172. doi:10.1002/bit.26343
Hsu P. P., Li S., Li Y. S., Usami S., Ratcliffe A., Wang X., et al. (2001). Effects of flow patterns on endothelial cell migration into a zone of mechanical denudation. Biochem. Biophys. Res. Commun. 285, 751–759. doi:10.1006/bbrc.2001.5221
Huh D. D. (2015). A human breathing lung-on-a-chip. Ann. Am. Thorac. Soc. 12 (Suppl. 1), S42–S44. doi:10.1513/AnnalsATS.201410-442MG
Hyman A. J., Tumova S., Beech D. J. (2017). Piezo1 channels in vascular development and the sensing of shear stress. Curr. Top. Membr. 79, 37–57. doi:10.1016/bs.ctm.2016.11.001
James N. L., Harrison D. G., Nerem R. M. (1995). Effects of shear on endothelial cell calcium in the presence and absence of ATP. FASEB J. 9, 968–973. doi:10.1096/fasebj.9.10.7615166
Jang K. J., Otieno M. A., Ronxhi J., Lim H. K., Ewart L., Kodella K. R., et al. (2019). Reproducing human and cross-species drug toxicities using a Liver-Chip. Sci. Transl. Med. 11, eaax5516. doi:10.1126/scitranslmed.aax5516
Jeon J. S., Bersini S., Gilardi M., Dubini G., Charest J. L., Moretti M., et al. (2015). Human 3D vascularized organotypic microfluidic assays to study breast cancer cell extravasation. Proc. Natl. Acad. Sci. U. S. A. 112, 214–219. doi:10.1073/pnas.1417115112
Jia W., Gungor-Ozkerim P. S., Zhang Y. S., Yue K., Zhu K., Liu W., et al. (2016). Direct 3D bioprinting of perfusable vascular constructs using a blend bioink. Biomaterials 106, 58–68. doi:10.1016/j.biomaterials.2016.07.038
Jin Y., Muhl L., Burmakin M., Wang Y., Duchez A. C., Betsholtz C., et al. (2017). Endoglin prevents vascular malformation by regulating flow-induced cell migration and specification through VEGFR2 signalling. Nat. Cell Biol. 19, 639–652. doi:10.1038/ncb3534
Kalucka J., de Rooij L., Goveia J., Rohlenova K., Dumas S. J., Meta E., et al. (2020). Single-cell transcriptome atlas of murine endothelial cells. Cell 180, 764–779. doi:10.1016/j.cell.2020.01.015
Khankin E. V., Ko N. L., Mandala M., Karumanchi S. A., Osol G. (2021). Normalization of wall shear stress as a physiological mechanism for regulating maternal uterine artery expansive remodeling during pregnancy. FASEB Bioadv 3, 702–708. doi:10.1096/fba.2021-00019
Kim S., Chung M., Ahn J., Lee S., Jeon N. L. (2016). Interstitial flow regulates the angiogenic response and phenotype of endothelial cells in a 3D culture model. Lab. Chip 16, 4189–4199. doi:10.1039/c6lc00910g
Kim S., Lee H., Chung M., Jeon N. L. (2013). Engineering of functional, perfusable 3D microvascular networks on a chip. Lab. Chip 13, 1489–1500. doi:10.1039/c3lc41320a
Kolesky D. B., Truby R. L., Gladman A. S., Busbee T. A., Homan K. A., Lewis J. A. (2014). 3D bioprinting of vascularized, heterogeneous cell-laden tissue constructs. Adv. Mater. 26, 3124–3130. doi:10.1002/adma.201305506
Koutsiaris A. G., Tachmitzi S. V., Batis N., Kotoula M. G., Karabatsas C. H., Tsironi E., et al. (2007). Volume flow and wall shear stress quantification in the human conjunctival capillaries and post-capillary venules in vivo. Biorheology 44, 375–386.
Kpeli G. W., Conrad K. M., Bralower W., Byrne C. E., Boue S. M., Burow M. E., et al. (2024). Xenohormetic phytochemicals inhibit neovascularization in microphysiological models of vasculogenesis and tumor angiogenesis. Adv. Biol. (Weinh) 8, e2300480. doi:10.1002/adbi.202300480
Krishnan L., Underwood C. J., Maas S., Ellis B. J., Kode T. C., Hoying J. B., et al. (2008). Effect of mechanical boundary conditions on orientation of angiogenic microvessels. Cardiovasc Res. 78, 324–332. doi:10.1093/cvr/cvn055
Kusuma S., Shen Y. I., Hanjaya-Putra D., Mali P., Cheng L., Gerecht S. (2013). Self-organized vascular networks from human pluripotent stem cells in a synthetic matrix. Proc. Natl. Acad. Sci. U. S. A. 110, 12601–12606. doi:10.1073/pnas.1306562110
Lam S. F., Shirure V. S., Chu Y. E., Soetikno A. G., George S. C. (2018). Microfluidic device to attain high spatial and temporal control of oxygen. PLOS ONE 13, e0209574. doi:10.1371/journal.pone.0209574
Langer K., Joensson H. N. (2020). Rapid production and recovery of cell spheroids by automated droplet microfluidics. SLAS Technol. 25, 111–122. doi:10.1177/2472630319877376
Langer V., Vivi E., Regensburger D., Winkler T. H., Waldner M. J., Rath T., et al. (2019). IFN-γ drives inflammatory bowel disease pathogenesis through VE-cadherin-directed vascular barrier disruption. J. Clin. Invest. 129, 4691–4707. doi:10.1172/JCI124884
Langille B. L., Reidy M. A., Kline R. L. (1986). Injury and repair of endothelium at sites of flow disturbances near abdominal aortic coarctations in rabbits. Arteriosclerosis 6, 146–154. doi:10.1161/01.atv.6.2.146
Larrivee B., Prahst C., Gordon E., del Toro R., Mathivet T., Duarte A., et al. (2012). ALK1 signaling inhibits angiogenesis by cooperating with the Notch pathway. Dev. Cell 22, 489–500. doi:10.1016/j.devcel.2012.02.005
Lee V. K., Kim D. Y., Ngo H., Lee Y., Seo L., Yoo S.-S., et al. (2014). Creating perfused functional vascular channels using 3D bio-printing technology. Biomaterials 35, 8092–8102. doi:10.1016/j.biomaterials.2014.05.083
Linville R. M., Boland N. F., Covarrubias G., Price G. M., Tien J. (2016). Physical and chemical signals that promote vascularization of capillary-scale channels. Cell. Mol. Bioeng. 9, 73–84. doi:10.1007/s12195-016-0429-8
Liu M. C., Shih H. C., Wu J. G., Weng T. W., Wu C. Y., Lu J. C., et al. (2013). Electrofluidic pressure sensor embedded microfluidic device: a study of endothelial cells under hydrostatic pressure and shear stress combinations. Lab. Chip 13, 1743–1753. doi:10.1039/c3lc41414k
Liu Z., Ruter D. L., Quigley K., Tanke N. T., Jiang Y., Bautch V. L. (2021). Single-cell RNA sequencing reveals endothelial cell transcriptome heterogeneity under homeostatic laminar flow. Arterioscler. Thromb. Vasc. Biol. 41, 2575–2584. doi:10.1161/ATVBAHA.121.316797
Lucitti J. L., Jones E. A., Huang C., Chen J., Fraser S. E., Dickinson M. E. (2007). Vascular remodeling of the mouse yolk sac requires hemodynamic force. Development 134, 3317–3326. doi:10.1242/dev.02883
Luu V. Z., Chowdhury B., Al-Omran M., Hess D. A., Verma S. (2018). Role of endothelial primary cilia as fluid mechanosensors on vascular health. Atherosclerosis 275, 196–204. doi:10.1016/j.atherosclerosis.2018.06.818
Mack J. J., Mosqueiro T. S., Archer B. J., Jones W. M., Sunshine H., Faas G. C., et al. (2017). NOTCH1 is a mechanosensor in adult arteries. Nat. Commun. 8, 1620. doi:10.1038/s41467-017-01741-8
Mammoto T., Hunyenyiwa T., Kyi P., Hendee K., Matus K., Rao S., et al. (2022). Hydrostatic pressure controls angiogenesis through endothelial YAP1 during lung regeneration. Front. Bioeng. Biotechnol. 10, 823642. doi:10.3389/fbioe.2022.823642
Marx U., Andersson T. B., Bahinski A., Beilmann M., Beken S., Cassee F. R., et al. (2016). Biology-inspired microphysiological system approaches to solve the prediction dilemma of substance testing. ALTEX 33, 272–321. doi:10.14573/altex.1603161
Mendez P. L., Obendorf L., Jatzlau J., Burdzinski W., Reichenbach M., Nageswaran V., et al. (2022). Atheroprone fluid shear stress-regulated ALK1-Endoglin-SMAD signaling originates from early endosomes. BMC Biol. 20, 210. doi:10.1186/s12915-022-01396-y
Miao H., Hu Y. L., Shiu Y. T., Yuan S., Zhao Y., Kaunas R., et al. (2005). Effects of flow patterns on the localization and expression of VE-cadherin at vascular endothelial cell junctions: in vivo and in vitro investigations. J. Vasc. Res. 42, 77–89. doi:10.1159/000083094
Miller J. S., Stevens K. R., Yang M. T., Baker B. M., Nguyen D.-H. T., Cohen D. M., et al. (2012). Rapid casting of patterned vascular networks for perfusable engineered three-dimensional tissues. Nat. Mater. 11, 768–774. doi:10.1038/nmat3357
Mohammed M., Thurgood P., Gilliam C., Nguyen N., Pirogova E., Peter K., et al. (2019). Studying the response of aortic endothelial cells under pulsatile flow using a compact microfluidic system. Anal. Chem. 91, 12077–12084. doi:10.1021/acs.analchem.9b03247
Mondrinos M. J., Jones P. L., Finck C. M., Lelkes P. I. (2014). Engineering de novo assembly of fetal pulmonary organoids. Tissue Eng. Part A 20, 2892–2907. doi:10.1089/ten.TEA.2014.0085
Moon J. J., Hahn M. S., Kim I., Nsiah B. A., West J. L. (2009). Micropatterning of poly(ethylene glycol) diacrylate hydrogels with biomolecules to regulate and guide endothelial morphogenesis. Tissue Eng. Part A 15, 579–585. doi:10.1089/ten.tea.2008.0196
Muller-Marschhausen K., Waschke J., Drenckhahn D. (2008). Physiological hydrostatic pressure protects endothelial monolayer integrity. Am. J. Physiol. Cell Physiol. 294, C324–C332. doi:10.1152/ajpcell.00319.2007
Nakatsu M. N., Hughes C. C. (2008). An optimized three-dimensional in vitro model for the analysis of angiogenesis. Methods Enzymol. 443, 65–82. doi:10.1016/S0076-6879(08)02004-1
Nakatsu M. N., Sainson R. C. A., Aoto J. N., Taylor K. L., Aitkenhead M., Pérez-del-Pulgar S., et al. (2003). Angiogenic sprouting and capillary lumen formation modeled by human umbilical vein endothelial cells (HUVEC) in fibrin gels: the role of fibroblasts and Angiopoietin-1. Microvasc. Res. 66, 102–112. doi:10.1016/s0026-2862(03)00045-1
Newman A. C., Nakatsu M. N., Chou W., Gershon P. D., Hughes C. C. (2011). The requirement for fibroblasts in angiogenesis: fibroblast-derived matrix proteins are essential for endothelial cell lumen formation. Mol. Biol. Cell 22, 3791–3800. doi:10.1091/mbc.E11-05-0393
Norotte C., Marga F. S., Niklason L. E., Forgacs G. (2009). Scaffold-free vascular tissue engineering using bioprinting. Biomaterials 30, 5910–5917. doi:10.1016/j.biomaterials.2009.06.034
O’Grady B. J., Wang J. X., Faley S. L., Balikov D. A., Lippmann E. S., Bellan L. M., et al. (2018). A customizable, low-cost perfusion system for sustaining tissue constructs. SLAS Technol. 23, 592–598. doi:10.1177/2472630318775059
Orellano I., Thomas A., Herrera A., Brauer E., Wulsten D., Petersen A., et al. (2022). Engineering vascular self-assembly by controlled 3D-printed cell placement. Adv. Funct. Mater. 32, 2208325. doi:10.1002/adfm.202208325
Ozer L. Y., Fayed H. S., Ericsson J., Al Haj Zen A. (2023). Development of a cancer metastasis-on-chip assay for high throughput drug screening. Front. Oncol. 13, 1269376. doi:10.3389/fonc.2023.1269376
Papaioannou T. G., Stefanadis C. (2005). Vascular wall shear stress: basic principles and methods. Hell. J. Cardiol. 46, 9–15.
Park T. E., Mustafaoglu N., Herland A., Hasselkus R., Mannix R., FitzGerald E. A., et al. (2019). Hypoxia-enhanced Blood-Brain Barrier Chip recapitulates human barrier function and shuttling of drugs and antibodies. Nat. Commun. 10, 2621. doi:10.1038/s41467-019-10588-0
Pech S., Richter R., Lienig J. (2020). “Peristaltic pump with continuous flow and programmable flow pulsation,” in 2020 IEEE 8th electronics system-integration technology conference, ESTC, Norway, 15-18 September 2020 (IEEE) 1–5.
Phan D. T., Bender R. H. F., Andrejecsk J. W., Sobrino A., Hachey S. J., George S. C., et al. (2017). Blood-brain barrier-on-a-chip: microphysiological systems that capture the complexity of the blood-central nervous system interface. Exp. Biol. Med. (Maywood) 242, 1669–1678. doi:10.1177/1535370217694100
Pitts K. L., Fenech M. (2013). Micro-particle image velocimetry for velocity profile measurements of micro blood flows. J. Vis. Exp. 10, e50314. doi:10.3791/50314
Poceviciute R., Ismagilov R. F. (2019). Human-gut-microbiome on a chip. Nat. Biomed. Eng. 3, 500–501. doi:10.1038/s41551-019-0425-0
Polacheck W. J., Kutys M. L., Tefft J. B., Chen C. S. (2019). Microfabricated blood vessels for modeling the vascular transport barrier. Nat. Protoc. 14, 1425–1454. doi:10.1038/s41596-019-0144-8
Polacheck W. J., Kutys M. L., Yang J., Eyckmans J., Wu Y., Vasavada H., et al. (2017). A non-canonical Notch complex regulates adherens junctions and vascular barrier function. Nature 552, 258–262. doi:10.1038/nature24998
Price G. M., Chrobak K. M., Tien J. (2008). Effect of cyclic AMP on barrier function of human lymphatic microvascular tubes. Microvasc. Res. 76, 46–51. doi:10.1016/j.mvr.2008.02.003
Prystopiuk V., Fels B., Simon C. S., Liashkovich I., Pasrednik D., Kronlage C., et al. (2018). A two-phase response of endothelial cells to hydrostatic pressure. J. Cell Sci. 131, jcs206920. doi:10.1242/jcs.206920
Reneman R. S., Hoeks A. P. (2008). Wall shear stress as measured in vivo: consequences for the design of the arterial system. Med. Biol. Eng. Comput. 46, 499–507. doi:10.1007/s11517-008-0330-2
Rosenfeld D., Landau S., Shandalov Y., Raindel N., Freiman A., Shor E., et al. (2016). Morphogenesis of 3D vascular networks is regulated by tensile forces. PNAS 113, 3215–3220. doi:10.1073/pnas.1522273113
Sadr N., Zhu M., Osaki T., Kakegawa T., Yang Y., Moretti M., et al. (2011). SAM-based cell transfer to photopatterned hydrogels for microengineering vascular-like structures. Biomaterials 32, 7479–7490. doi:10.1016/j.biomaterials.2011.06.034
Sahni J., Arshad M., Schake M. A., Brooks J. R., Yang R., Weinberg P. D., et al. (2023). Characterizing nuclear morphology and expression of eNOS in vascular endothelial cells subjected to a continuous range of wall shear stress magnitudes and directionality. J. Mech. Behav. Biomed. Mater 137, 105545. doi:10.1016/j.jmbbm.2022.105545
Saqr K. M., Tupin S., Rashad S., Endo T., Niizuma K., Tominaga T., et al. (2020). Physiologic blood flow is turbulent. Sci. Rep. 10, 15492. doi:10.1038/s41598-020-72309-8
Schneider S., Bubeck M., Rogal J., Weener H. J., Rojas C., Weiss M., et al. (2021). Peristaltic on-chip pump for tunable media circulation and whole blood perfusion in PDMS-free organ-on-chip and Organ-Disc systems. Lab. Chip 21, 3963–3978. doi:10.1039/d1lc00494h
Schwartz E. A., Bizios R., Medow M. S., Gerritsen M. E. (1999). Exposure of human vascular endothelial cells to sustained hydrostatic pressure stimulates proliferation. Involvement of the alphaV integrins. Circ. Res. 84, 315–322. doi:10.1161/01.res.84.3.315
Sedlak J., Clyne A. (2023). Application of shear stress to endothelial cells using a parallel plate flow chamber. Methods Mol. Biol. 2600, 81–90. doi:10.1007/978-1-0716-2851-5_5
Seok J., Warren H. S., Cuenca A. G., Mindrinos M. N., Baker H. V., Xu W., et al. (2013). Genomic responses in mouse models poorly mimic human inflammatory diseases. Proc. Natl. Acad. Sci. U. S. A. 110, 3507–3512. doi:10.1073/pnas.1222878110
Seto Y., Inaba R., Okuyama T., Sassa F., Suzuki H., Fukuda J. (2010). Engineering of capillary-like structures in tissue constructs by electrochemical detachment of cells. Biomaterials 31, 2209–2215. doi:10.1016/j.biomaterials.2009.11.104
Shevkoplyas S. S., Gifford S. C., Yoshida T., Bitensky M. W. (2003). Prototype of an in vitro model of the microcirculation. Microvasc. Res. 65, 132–136. doi:10.1016/s0026-2862(02)00034-1
Si L., Bai H., Rodas M., Cao W., Oh C. Y., Jiang A., et al. (2021). A human-airway-on-a-chip for the rapid identification of candidate antiviral therapeutics and prophylactics. Nat. Biomed. Eng. 5, 815–829. doi:10.1038/s41551-021-00718-9
Sobrino A., Phan D. T., Datta R., Wang X., Hachey S. J., Romero-Lopez M., et al. (2016). 3D microtumors in vitro supported by perfused vascular networks. Sci. Rep. 6, 31589. doi:10.1038/srep31589
Suo J., Ferrara D. E., Sorescu D., Guldberg R. E., Taylor W. R., Giddens D. P. (2007). Hemodynamic shear stresses in mouse aortas: implications for atherogenesis. Arterioscler. Thromb. Vasc. Biol. 27, 346–351. doi:10.1161/01.ATV.0000253492.45717.46
Torisawa Y. S., Spina C. S., Mammoto T., Mammoto A., Weaver J. C., Tat T., et al. (2014). Bone marrow-on-a-chip replicates hematopoietic niche physiology in vitro. Nat. Methods 11, 663–669. doi:10.1038/nmeth.2938
Tzima E., Irani-Tehrani M., Kiosses W. B., Dejana E., Schultz D. A., Engelhardt B., et al. (2005). A mechanosensory complex that mediates the endothelial cell response to fluid shear stress. Nature 437, 426–431. doi:10.1038/nature03952
van Os L., Yeoh J., Witz G., Ferrari D., Krebs P., Chandorkar Y., et al. (2023). Immune cell extravasation in an organ-on-chip to model lung inflammation. Eur. J. Pharm. Sci. 187, 106485. doi:10.1016/j.ejps.2023.106485
Vatine G. D., Barrile R., Workman M. J., Sances S., Barriga B. K., Rahnama M., et al. (2019). Human iPSC-derived blood-brain barrier chips enable disease modeling and personalized medicine applications. Cell Stem Cell 24, 995–1005. doi:10.1016/j.stem.2019.05.011
Viravaidya K., Shuler M. L. (2004). Incorporation of 3T3-L1 cells to mimic bioaccumulation in a microscale cell culture analog device for toxicity studies. Biotechnol. Prog. 20, 590–597. doi:10.1021/bp034238d
Viravaidya K., Sin A., Shuler M. L. (2004). Development of a microscale cell culture analog to probe naphthalene toxicity. Biotechnol. Prog. 20, 316–323. doi:10.1021/bp0341996
Vogt N. (2022). Modeling multi-organ systems on a chip. Nat. Methods 19, 641. doi:10.1038/s41592-022-01533-z
Voyvodic P. L., Min D., Baker A. B. (2012). A multichannel dampened flow system for studies on shear stress-mediated mechanotransduction. Lab. Chip 12, 3322–3330. doi:10.1039/c2lc40526a
Wang X., Phan D. T., Sobrino A., George S. C., Hughes C. C., Lee A. P. (2016). Engineering anastomosis between living capillary networks and endothelial cell-lined microfluidic channels. Lab. Chip 16, 282–290. doi:10.1039/c5lc01050k
Wang X.-Y., Jin Z.-H., Gan B.-W., Lv S.-W., Xie M., Huang W.-H. (2014). Engineering interconnected 3D vascular networks in hydrogels using molded sodium alginate lattice as the sacrificial template. Lab. Chip 14, 2709–2716. doi:10.1039/c4lc00069b
Wang Y. I., Shuler M. L. (2018). UniChip enables long-term recirculating unidirectional perfusion with gravity-driven flow for microphysiological systems. Lab. Chip 18, 2563–2574. doi:10.1039/c8lc00394g
Whisler J. A., Chen M. B., Kamm R. D. (2014). Control of perfusable microvascular network morphology using a multiculture microfluidic system. Tissue Eng. - Part C. Methods 20, 543–552. doi:10.1089/ten.TEC.2013.0370
Wimmer R. A., Leopoldi A., Aichinger M., Wick N., Hantusch B., Novatchkova M., et al. (2019). Human blood vessel organoids as a model of diabetic vasculopathy. Nature 565, 505–510. doi:10.1038/s41586-018-0858-8
Wong K. H., Truslow J. G., Tien J. (2010). The role of cyclic AMP in normalizing the function of engineered human blood microvessels in microfluidic collagen gels. Biomaterials 31, 4706–4714. doi:10.1016/j.biomaterials.2010.02.041
Xiao S., Coppeta J. R., Rogers H. B., Isenberg B. C., Zhu J., Olalekan S. A., et al. (2017). A microfluidic culture model of the human reproductive tract and 28-day menstrual cycle. Nat. Commun. 8, 14584. doi:10.1038/ncomms14584
Yang F., Zhang Y., Zhu J., Wang J., Jiang Z., Zhao C., et al. (2020). Laminar flow protects vascular endothelial tight junctions and barrier function via maintaining the expression of long non-coding RNA MALAT1. Front. Bioeng. Biotechnol. 8, 647. doi:10.3389/fbioe.2020.00647
Yoshino D., Funamoto K., Sato Kenry K., Sato M., Lim C. T. (2020). Hydrostatic pressure promotes endothelial tube formation through aquaporin 1 and Ras-ERK signaling. Commun. Biol. 3, 152. doi:10.1038/s42003-020-0881-9
Zervantonakis I. K., Hughes-Alford S. K., Charest J. L., Condeelis J. S., Gertler F. B., Kamm R. D. (2012). Three-dimensional microfluidic model for tumor cell intravasation and endothelial barrier function. Proc. Natl. Acad. Sci. U. S. A. 109, 13515–13520. doi:10.1073/pnas.1210182109
Zhang B., Radisic M. (2017). Organ-on-a-chip devices advance to market. Lab. Chip 17, 2395–2420. doi:10.1039/c6lc01554a
Zhang G., Cao G., Gu C., Fu Y., Jin G., Tang L., et al. (2022). Regulation of vascular branch formation in 3D bioprinted tissues using confining force. Appl. Mater. Today 26, 101240. doi:10.1016/j.apmt.2021.101240
Zhang G., Varkey M., Wang Z., Xie B., Hou R., Atala A. (2020a). ECM concentration and cell-mediated traction forces play a role in vascular network assembly in 3D bioprinted tissue. Biotechnol. Bioeng. 117, 1148–1158. doi:10.1002/bit.27250
Zhang G., Wang Z., Han F., Jin G., Xu L., Xu H., et al. (2021). Mechano-regulation of vascular network formation without branches in 3D bioprinted cell-laden hydrogel constructs. Biotechnol. Bioeng. 118, 3787–3798. doi:10.1002/bit.27854
Zhang X., Bishawi M., Zhang G., Prasad V., Salmon E., Breithaupt J. J., et al. (2020b). Modeling early stage atherosclerosis in a primary human vascular microphysiological system. Nat. Commun. 11, 5426. doi:10.1038/s41467-020-19197-8
Zheng Y., Chen J., Craven M., Choi N. W., Totorica S., Diaz-Santana A., et al. (2012). In vitro microvessels for the study of angiogenesis and thrombosis. PNAS 109, 9342–9347. doi:10.1073/pnas.1201240109
Keywords: organ chips, microphysiological systems, vessel-on-a-chip, fluid shear stress, wall shear stress, mechanotransduction, hemodynamic flow
Citation: Martier A, Chen Z, Schaps H, Mondrinos MJ and Fang JS (2024) Capturing physiological hemodynamic flow and mechanosensitive cell signaling in vessel-on-a-chip platforms. Front. Physiol. 15:1425618. doi: 10.3389/fphys.2024.1425618
Received: 06 May 2024; Accepted: 10 July 2024;
Published: 29 July 2024.
Edited by:
Nicolas Baeyens, Université libre de Bruxelles, BelgiumReviewed by:
Daisuke Yoshino, Tokyo University of Agriculture and Technology, JapanJulian Albarran Juarez, Aarhus University, Denmark
Copyright © 2024 Martier, Chen, Schaps, Mondrinos and Fang. This is an open-access article distributed under the terms of the Creative Commons Attribution License (CC BY). The use, distribution or reproduction in other forums is permitted, provided the original author(s) and the copyright owner(s) are credited and that the original publication in this journal is cited, in accordance with accepted academic practice. No use, distribution or reproduction is permitted which does not comply with these terms.
*Correspondence: J. S. Fang, amZhbmc1QHR1bGFuZS5lZHU=
†These authors have contributed equally to this work