- 1Dipartimento di Medicina e Chirurgia, Università Milano-Bicocca, Monza, Italy
- 2Department of Emergency and Intensive Care, Fondazione IRCCS San Gerardo dei Tintori, Monza, Italy
- 3Vicerrectorado de Investigación, Escuela de Postgrado Universidad Católica de Santa María, Arequipa, Peru
- 4Unidad de Cuidados Intensivos, Hospital Nacional Carlos Alberto Seguin Escobedo, Arequipa, Peru
- 5High Altitude Pulmonary and Pathology Institute (HAPPI-IPPA), La Paz, Bolivia
This is the first study to describe the daytime evolution of respiratory parameters in mechanically ventilated COVID-19 patients. The data base refers to patients hospitalised in the intensive care unit (ICU) at Arequipa Hospital (Peru, 2335 m) in 2021. In both survivors (S) and non-survivors (NS) patients, a remarkable decrease in respiratory compliance was observed, revealing a proportional decrease in inflatable alveolar units. The S and NS patients were all hyperventilated and their SatO2 was maintained at >90%. However, while S remained normocapnic, NS developed progressive hypercapnia. We compared the efficiency of O2 uptake and CO2 removal in the air blood barrier relying on a model allowing to partition between diffusion and perfusion limitations to gas exchange. The decrease in O2 uptake was interpreted as diffusion limitation, while the impairment in CO2 removal was modelled by progressive perfusion limitation. The latter correlated with the increase in positive end-expiratory pressure (PEEP) and plateau pressure (Pplat), leading to capillary compression, increased blood velocity, and considerable shortening of the air-blood contact time.
1 Introduction
Respiratory failure can develop when lung disease forces a patient to unsuccessfully adapt his ventilatory response to ensure gas exchange. The management of severe lung diseases involving loss of function in the alveolar units remains a challenge in intensive care units. From a pathophysiological point of view, the critical issue is that the spreading of lung disease can lead to the progressive loss of specific morpho-functional features of the air-blood barrier that normally ensure gas diffusion (Rezoagli et al., 2022). The efficiency of gas exchange is based not only on the morphological integrity of the alveolar-capillary membrane but also on the functional coupling between the gas diffusion capacity and perfusion capacity. The importance of this coupling has recently been emphasised, providing additional information on facing a perturbation in gas exchange (Beretta et al., 2019; Miserocchi et al., 2022; Miserocchi, 2023a). Based on our past work on the air blood barrier function, we have been invited to comment on a database of daytime evolution of respiratory parameters in mechanically ventilated SARS-CoV-2 patients. SARS-CoV-2 respiratory failure has led to a massive need for mechanical ventilatory support worldwide (Rezoagli et al., 2021). This study wishes to explore the impact of SARS-CoV-2 disease on the diffusion/perfusion function in mechanical ventilated patients.
2 Material and methods
This was a retrospective study based on data from adult COVID patients hospitalised in the intensive care unit (ICU) at Arequipa Hospital (Peru, 2335 m) in 2021. The study was conducted in accordance with the Declaration of Helsinki. Patient consent was waived owing to the observational nature of the study, and the Institutional Review Board of Arequipa Hospital approved the data collection. No patient identifiers were used in this study.
Patients with a clinical diagnosis of respiratory failure and with a positive confirmation at the PCR quantification of Sars-CoV2 infection by sample evaluation from airways (i.e., naso-pharingeal swabs, bronchoaspirate, bronchoalveolar lavage) were intubated according to Institution standard of care of the admitting Intensive Care Unit and were enrolled in the current analysis. No specific exclusion criteria were considered. Settings of mechanical ventilation were applied in accordance to the recommendations of protective mechanical ventilation as reported in the ARDS guidelines (Fan et al., 2017).
All patients were maintained in the supine position, 30° head up (Spooner et al., 2014). The following parameters were collected daily during mechanical ventilation in ICU: tidal volume (Vt), respiratory rate (RR), minute ventilation (
Statistics: descriptive data were reported as mean ± standard deviation; differences between continuous variables were reported as t-tests paired and unpaired as appropriate; correlations of continuous data were assessed by Pearson’s correlation coefficient; alpha level<0.05 was deemed significant (two-tailed). All statistical analyses were performed using Microsoft Excel (Version 16.81).
3 Results
Table 1 reports data for survivors (S, n = 30; 26 males, 87%) and non-survivors (NS, n = 16; 15 males, 94%) patients referring to the first day of admission in ICU.
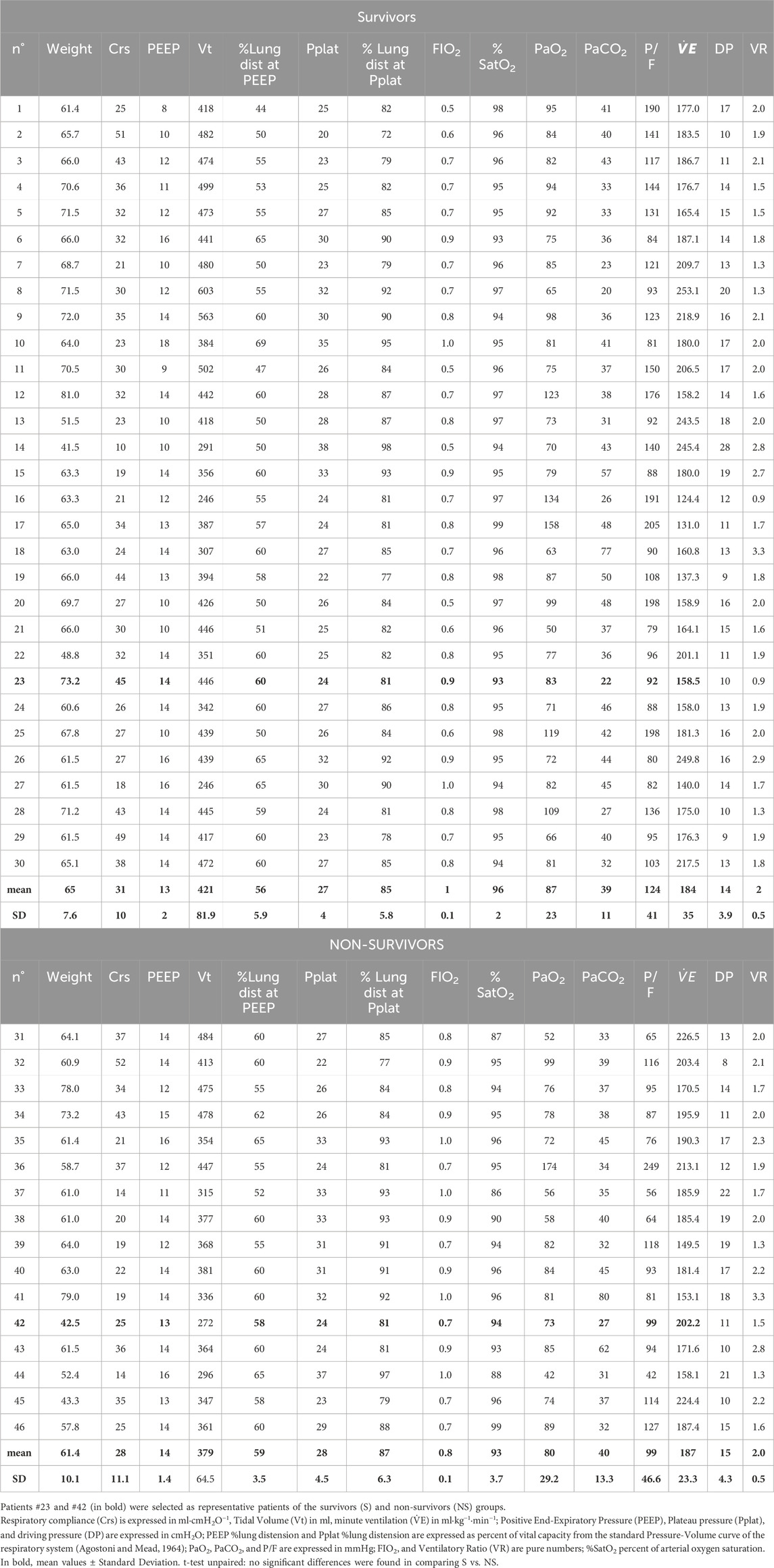
Table 1. Data for survivors (S) and non-survivors (NS) patients on the first day of admission in ICU.
Table 2 reports data for S and NS patients referring to the last day of ICU.
Comparing data on the first and last days of ICU stay (Tables 1, 2) in S (n = 30) patients, we found the following:
- No significant change in Crs, but a significant decrease in PEEP and Pplat (with a corresponding decrease in lung distension);
- All subjects were hyperventilated relative to the standard value of 100 mL kg−1·min−1.
- Significant increase in diffusion/perfusion efficiency of the air–blood barrier for O2 (increase in PaO2, P/F, and decrease in FIO2) and CO2 (decrease in PaCO2).
The same comparison for NS (n = 16) patients (Tables 1, 2) shows:
- Significant decrease in Crs with no change in PEEP and significant increase in Pplat (increase in lung distension).
- All subjects were hyperventilated.
- No change in diffusion/perfusion efficiency of the air–blood barrier for O2 (PaO2, P/F, and decrease in FIO2), but a considerable reduction in diffusion/perfusion efficiency for CO2 elimination (increase in PaCO2).
Upon comparing data from the first day between the S and NS patients, no significant differences were found. However, the same comparison for the last day showed significant differences for all parameters considered, except ventilation, revealing a considerable loss of diffusion/perfusion efficiency of the air–blood barrier concerning O2 and CO2 and greater overdistension of the lung at both PEEP and Pplat.
We decided to discuss two representative patients from the S and NS groups (patient #23 and #42 in Tables 1, 2, respectively). Both patients showed a comparable decrease in Crs, with opposite fates concerning the diffusion/perfusion efficiency of the air-blood barrier for O2 and CO2.
3.1 Respiratory mechanics and alveolar pressure during mechanical ventilation
The first row in Figures 1A–C shows the time course of Crs, PEEP, and Pplat in two representative patients: survivors (S, closed symbols) and non-survivors (NS, open symbols). In both patients, Crs decreased over time to a similar extent, although the Crs values in NS were lower (Panel A). A clear dissociation is seen in panel B, as PEEP increased in NS while decreasing in S. Panel C reports the Pplat values that increased over time in NS but remained essentially steady in S.
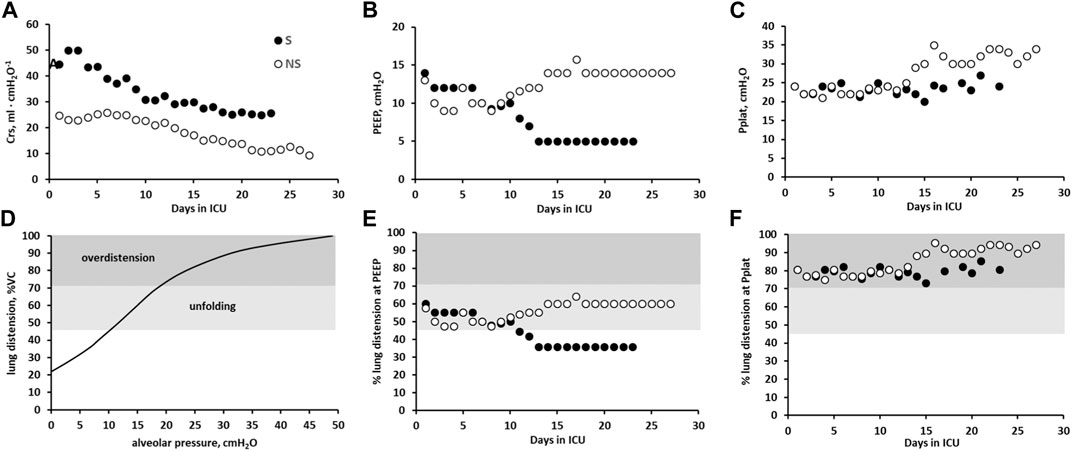
Figure 1. Respiratory parameters in 2 representative patients. Survivors (S, closed circles) and non-survivors (NS, open circles) in all panels. First row. Data referring to the time course of Crs (A), PEEP (B), and Pplat (C) Second row: Panel (D) reports the degree of lung distension based on the volume-pressure curve of the respiratory system in the supine position. Grey levels refer to the alveolar folding/unfolding and lung overdistension zones. Panels (E,F) report %lung distension for the two subjects at PEEP and Pplat, respectively.
Panel D in the second row of Figure 1 shows the pressure-volume relationship of the respiratory system (Agostoni and Mead, 1964), which is expressed as a percentage of the maximum. The maximum volume decreased with decreasing Crs, reflecting a decrease in inflatable alveolar units (IAU). If the mechanical properties of the residual IAU remained unchanged, the curve in Figure 1D reflects the specific compliance of the IAU. Based on this assumption, the ordinate can be used to express the corresponding degree of lung distension of the IAU as a function of the alveolar pressure. The light grey area includes the portion of the pressure-volume curve with the highest specific compliance, extending from 45% to 70% lung distension, corresponding to a range of alveolar pressures from approximately 10–20 cmH2O. In this range of pressures, the process of unfolding of the alveolar surface takes place on inspiration, reflecting the existence of a “reserve” surface area of the corrugated alveolar cells (Weibel, 2015). As the unfolding process develops, the parenchymal stretch gradually increases (from the light to the darker grey area), indicating lung overdistension. Under physiological conditions at rest, an increase in tidal volume during spontaneous breathing is achieved by an increase in transpulmonary pressure of approximately 5 cmH2O; accordingly, the same tidal volume in mechanical ventilation would be achieved by an alveolar pressure of approximately 5 cmH2O, corresponding to 30% lung distension, well below the saturation of the unfolding zone. Panels E and F show the degree of lung distention at PEEP and Pplat, respectively, for the two subjects. In the case of NS, lung distension at PEEP falls in the light grey area, whereas at Pplat, lung distension falls in the overdistension zone for both patients.
3.2 Gas exchange
Panel A in Figure 2 shows that the time course of P/F significantly decreased in both patients. As shown in Panel B, no significant differences in SatO2 were observed. Panels C and E again show a divergence in the time courses of FIO2 and PaCO2, despite displaying a similar time course for PaO2 and VE/kg (Panels D and F, respectively).
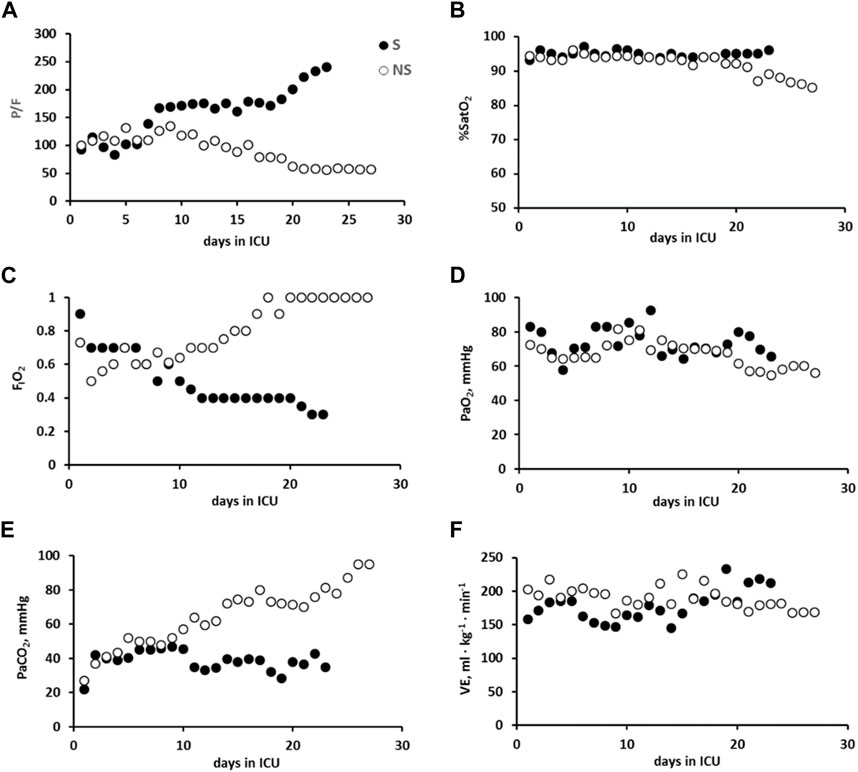
Figure 2. Data referring to the time course of gas exchange in the two representative patients: survivors, S (closed symbols), and non-survivors (NS, open symbols) in all panels.: P/F (A), SatO2 (B), FIO2 (C), PaO2 (D), PaCO2 (E), and VE/kg (F).
Figure 3 (Panel A) shows the relationships of P/F plotted vs. Crs; for a decrease in Crs, P/F increased in patient S but decreased in NS. Panel B shows a decrease in P/F with increasing PEEP in patient NS; conversely (Panel C), P/F increased in S with decreasing PEEP.
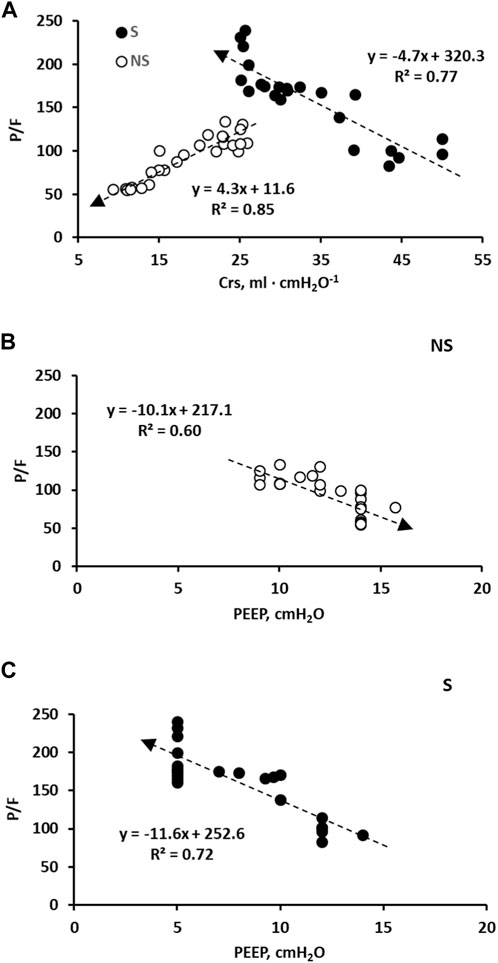
Figure 3. (A): correlation between day-time P/F vs. Crs in the two subjects S (closed symbols) and NS (open symbols). (B): correlation between day-time P/F vs. PEEP in subject NS. (C): correlation between day-time P/F vs. PEEP in subject S. Time dependence is expressed by the arrows in all panels.
Figure 4 shows the time course of tidal volume (Vt) and respiratory rate (RR) in S and NS patients (Panels A and B). By decreasing PEEP (Figure 1B), a higher driving pressure can increase Vt in S patient. Conversely, the opposite occurred in NS patient due to an increase in PEEP (Figure 1B), particularly considering the greater decrease in lung compliance (Figure 1A). Furthermore, it should be noted that in NS patients, the Vt approaches the anatomical dead space. The RR (Panel B) remained high for both subjects.
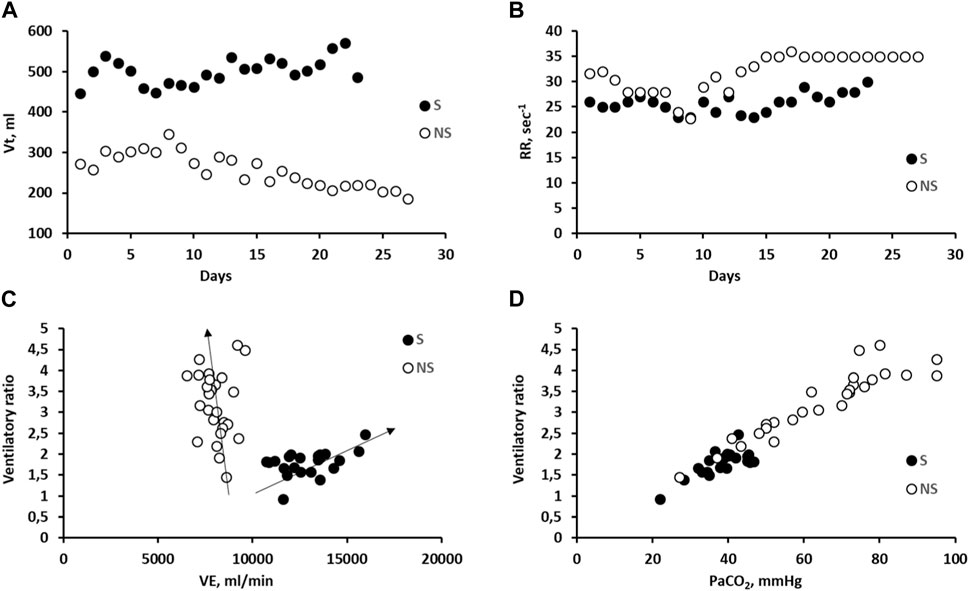
Figure 4. (A): correlation between tidal volume (Vt) vs. days in subjects S and NS; (B): correlation between respiratory rate (RR) vs. PEEP in subjects S and NS. (C): correlation between day-time Ventilatory ratio vs. Ventilation (VE) in the two representative subjects S and NS. (D): correlation between day-time Ventilatory ratio vs. PaCO2 in the two representative subjects S and NS.
Concerning the ventilatory ratio, a clear dependence on ventilation is observed, albeit in opposite directions, considering S and NS patients (panel C). Panel D shows the striking dependence of the ventilatory ratio on PaCO2; in the case of patient S, PaCO2 remained within the physiological range, while it increased remarkably in patient NS.
4 Discussion
To our knowledge, this is the first study to compare the evolution of respiratory parameters in survivors and non-survivors mechanically ventilated SARS-CoV-2 patients hospitalised in ICU. This is a physiologic study and not a clinical study with the ambition of a validation phase of the results. The novelty is the longitudinal physiological data granularity, the stratification by outcome. The data interpretation is made on physiological models that offer a mechanistic reading to the ventilatory data and gas exchange behaviour in patients who did or did not survive at ICU discharge. We will discuss the differences defining a computational biophysical model allowing to define potential diffusion/perfusion limitations of alveolar gas exchanges.
Figure 5 summarises the various conditions that may impact gas exchange at the alveolar level during the development of lung diseases, such as SARS-CoV-2 infection. Diffusion limitation may progress from a physiological condition (A) to interstitial oedema (B) and severe oedema with alveolar flooding (C). The development of oedema reflects an increase in microvascular permeability due to the progressive fragmentation of the proteoglycan component of the interstitial macromolecular network (Negrini et al., 1998; Negrini et al., 2008; Moriondo et al., 2012; Yi et al., 2016). The path from A to C indicates a progressive increase in the shunt effect. The path from A to E shows a case of perfusion limitation due to pulmonary capillary squeezing due to lung overdistension (D) or complete vessel closure due to thrombosis (E). The progression from A to D led to an increase in dead space. Red dashed arrows indicate mixed events that occur in severe lung pathology.
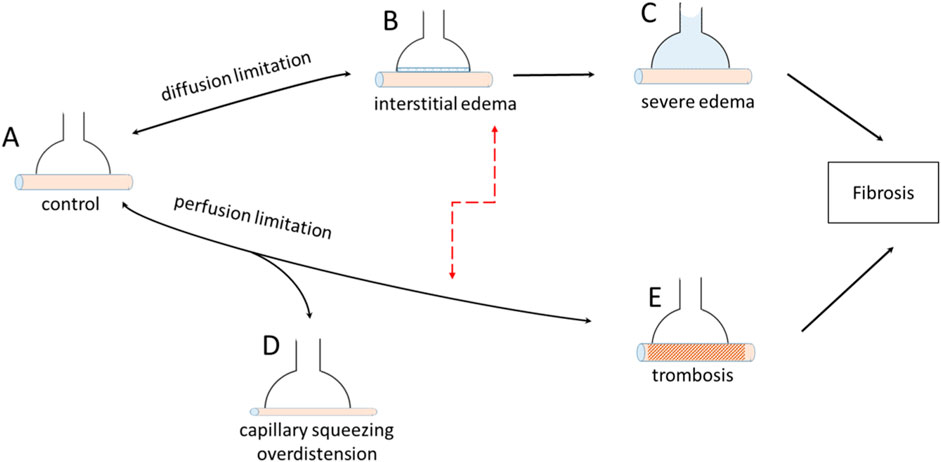
Figure 5. Various pathological conditions leading to diffusion limitation and perfusion limitation at the level of the air-blood barrier.
We interpret the decrease in Crs as mainly due to the loss of inflatable alveolar units (IAU) during disease progression and partly due to the increase in tissue elastance during the development of interstitial oedema (Dellacà et al., 2008). Gas exchange can occur only in the IAU, which retains its morphofunctional features to ensure gas diffusion.
4.1 Dependence of Vc on lung distension
An increase in alveolar pressure leads to a decrease in capillary blood volume (Vc), owing to the squeezing of capillaries (Figure 5D) caused by an increase in parenchymal stretching (Glazier et al., 1969; Brower et al., 1985; Nieman et al., 1988; Koyama and Hildebrandt, 1991; Miserocchi et al., 2008). This decrease was found to vary remarkably among subjects, depending on the individual morphofunctional assembly of the alveolar capillary unit. The latter is characterised by the ratio of Vc to the diffusion capacity of the alveolar membrane (Vc/Dm), which essentially compares inter-individual differences in the extension of the pulmonary alveolar capillary network to the alveolar size (Miserocchi et al., 2008). The present available data do not allow for the estimation of inter-individual differences in Vc/Dm among patients. Accordingly, Figure 6 shows three cases of Vc ranging at Functional Residual Capacity (FRC) from 150 to 300 mL (corresponding to different Vc/Dm ratios). The figure reports the expected decrease in Vc with increasing lung distension with a PEEP of 5 and 15 cmH2O (Miserocchi et al., 2008): clearly, the decrease in Vc (in absolute terms) is larger the greater the Vc value at FRC (Figure 5D).
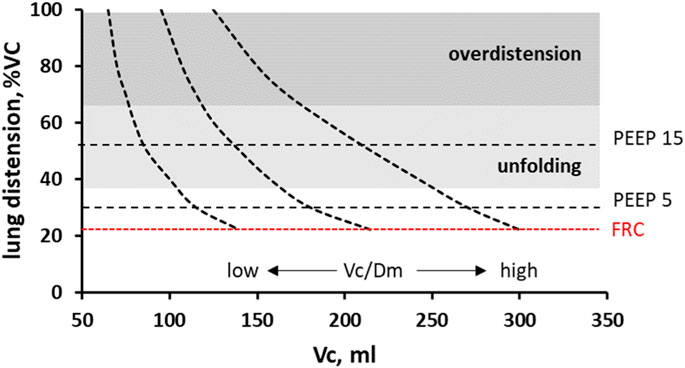
Figure 6. Decrease in capillary blood volume (Vc) on increasing lung distension, considering three cases of Vc values at Functional Residual Capacity (FRC) in the supine position. The Vc values correspond to either a low or high ratio to the diffusion capacity of the alveolar membrane (Vc/Dm), characterising inter-individual differences in alveolar morphology (from Miserocchi et al., 2008). Figure also refers to a PEEP of 5 and 10 cmH2O.
Interestingly, a decrease in pulmonary blood volume has been documented in post COVID-19 through Dual-energy CT scan not only in opacification areas but also in parenchyma of normal appearance in acute (Aydin et al., 2021; Ball et al., 2021) and post-acute phase (Mohamed et al., 2023) (Figure 5E), suggesting a potential limitation to perfusion.
4.2 Gas exchange
We have developed a model to estimate the dependence of alveolar gas exchange resulting from the functional coupling of blood capillary flow with gas diffusion flows (Beretta et al., 2019; Miserocchi et al., 2022; Miserocchi, 2023b). Our present aim is to rely on this model to compare two distinct conditions: hyperoxia and normocapnia in S patients, against hyperoxia and hypercapnia in NS patients. We shall briefly summarize the principles of the biophysical model.
Based on the gas mass conservation notion (Piiper and Scheid, 1981) and an exponential kinetics of the equilibration process, the alveolar-capillary equilibration for gas exchange reached at the exit of the blood from the capillary is mathematically defined as:
being Tt blood capillary transit time (also known as “capillary residence time” or “blood contact time”), and τ is the time constant of the exponential kinetics. Tt is the key parameter to switch from volumes to flows and can be estimated as the ratio of pulmonary blood capillary volume (Vc) to cardiac output (
The kinetics of the equilibration is defined by the time constant given by:
and
being DO2 and DCO2, the respective diffusive capacities, while β and α include gas solubility and transport capacity in blood.
Leq can vary from 0 (perfect equilibration) to 1 (total lack of equilibration) (Beretta et al., 2019; Miserocchi et al., 2022; Miserocchi, 2023b).
This approach provided supplementary information to the classic
4.3 Diffusion and perfusion limitation
The key issue on comparing S with NS is that both groups had a SatO2>90%; however, while the former remained normocapnic, the latter developed hypercapnia.
Diffusion limitation is a specific case occurring for oxygen, due to its low solubility-diffusion coefficients (Eq. 3).
Figure 7 shows that under physiological conditions, Leq = 0 at the exit of the pulmonary capillaries.
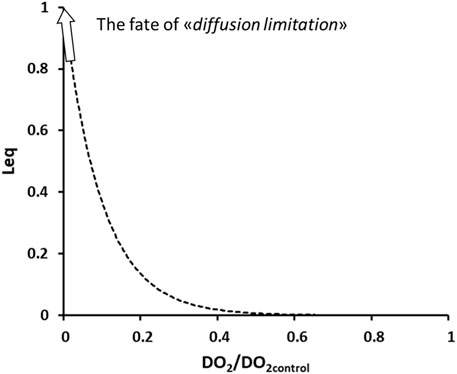
Figure 7. Exponential increase in Leq on decreasing diffusive capacitance for O2 relative to control.
The development of interstitial and severe alveolar edema (Figures 5B, C) represent the obvious case of diffusion limitation for O2 uptake, due to the decrease in DO2. As shown in Figure 7, an exponential loss of equilibration capacity occurred with a 5-times decrease in DO2, compatible with the observed average decrease in Crs in patients relative to a physiological value of approximately 100 mL/cmH2O. The loss of O2 equilibration capacity for the whole lung simulates a “shunt-like effect.”
Oxygen diffusion limitation is commonly compensated for by an increase in FIO2. One shall report that for FIO2 > 0.7 (Aggarwal et al., 2018), cellular (Kistler et al., 1967; Weibel, 1971) and tissue (Chow et al., 2003; Kallet and Matthay, 2013) damage in the lungs were reported, leading to increased alveolar permeability (Matalon and Egan, 1981; Kolliputi et al., 2010).
Conversely, a diffusion limitation is hardly conceivable for CO2 exchange considering its high solubility-diffusion coefficients. Accordingly, one can develop the hypothesis of perfusion limitation.
The aim of our study is to find a cause-effect relationship for developing hypercapnia. Figure 8 presents a computational (Eq. 1) estimate of the exponential increase in perfusion limitation for CO2 removal for Tt < 1 s by decreasing Vc (Eq. 2) (Figures 5D, E).
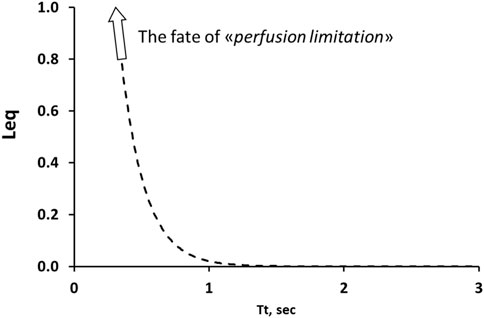
Figure 8. Computational model for cause-effect interpretation of perfusion limitation in CO2 removal. Perfusion limitation expressed by the increase in Leq on decreasing Tt. Note the exponential increase in Leq for Tt < 1 s.
Besides a decrease in Vc due to pulmonary stretching, a further factor arises from tissue compression in developing oedema (Figures 5B, C), that could actually lead to complete vessel closure (Mazzuca et al., 2019), functionally equivalent to the case of thrombosis (Figure 5E). Furthermore, studies on ECMO have confirmed that CO2 removal is hampered by low blood flow (Karagiannidis et al., 2017; Giraud et al., 2021; Zanella et al., 2022).
4.4 Lung fluid balance
An estimate of the Starling pressure gradient controlling the lung fluid balance, particularly alveolar pressure, is presented in Figure 9. We accounted for the hydraulic and colloidal osmotic pressure, reflection coefficient, and alveolar surface tension (Beretta et al., 2021).
Data in Figure 9 show that, for Palv>5 cmH2O, the transendothelial Starling gradient favours microvascular filtration and thus is an edemagenic factor (Miserocchi et al., 1993).
Lung overdistension has been found to increase microvascular filtration (Miserocchi et al., 1991), a finding confirmed by a computational model showing stress-dependent leak progression through an epithelial monolayer (Hamlington et al., 2016; Hamlington et al., 2018).
Notably, a decrease in Tt, resulting in an increase in blood velocity, leads to an increase in shear rate (Miserocchi et al., 2022), which in turn causes the increase in microvascular and protein permeability, thus favouring oedema (Sill et al., 1995; Lakshminarayanan et al., 2000; Mazzag et al., 2003; Barakat et al., 2006; Kang et al., 2014; Kolářová et al., 2014; Miserocchi and Beretta, 2023).
4.5 Study limitations
We have to acknowledge some study limitation. We did not have any specific exclusion criteria. However, we did not have information on the screening data of ICU admission, but this is a convenient sample size of patients admitted to ICU of the Arequipa Hospital in Perù with a clinical diagnosis of respiratory failure with a positive PCR confirmation of Sars-CoV2 infection that were enrolled from April 2020 to March 2021. This study is not designed to evaluate independent association of clinical variables on outcome by using multivariable models of association but aims at exploring the physiopathology of gas exchange in the air-blood barrier during acute respiratory failure. We relied on established computational physio-pathological models.
4.6 Concluding remarks
This paper deals with diffusion and perfusion limitation to alveolar gas exchanges in mechanically ventilated COVID-19 patients.
In the representative NS patient, P/F decreased in the first week of ICU stay (Figure 2A), clearly reflecting oxygen diffusion limitation compensated by an increase in PEEP and FIO2 (Figures 1B, 2C, respectively). This was in accordance to the PEEP:FIO2 tables (Brower et al., 2004) and the guidelines (Fan et al., 2017), the leading idea being to increase alveolar recruitment and ventilation to favor oxygen uptake, although a steady SatO2>90%. However, the increase in PEEP may contribute to a progressive increase in PaCO2 (Reazoagli and Bellani, 2022), due to perfusion limitation, hindering CO2 removal (Figure 2E) and leading to a remarkable increase in the ventilatory ratio – which -in turn – is a marker per se of the respiratory failure severity (Figure 4D).
In representative S patient, the increase in P/F over the first week (Figure 2A) led to the decision (Brower et al., 2004) to decrease both PEEP and FIO2 (Figures 1B, 2C). Consequently, this allowed ventilation of patient S with a progressively lower mean airway pressure, favoring CO2 removal, notwithstanding the potential for alveolar de-recruitment.
In NS group, the ventilatory strategy led to SatO2 >90% coupled with severe hypercapnia. The latter has been considered as a biomarker of increased dead space due to perfusion limitation, a condition associated independently with a high risk of mortality (Nuckton et al., 2002).
In fact, survivors or non-survivors are separated by a faint border considering that in both groups, lung compliance was decreased by the disease to approximately 1/5 of normal, meaning that the total number of alveolar units assuring gas exchange was decreased from a physiological value of approximately 500 (Ochs et al., 2004) to 100 million. One cannot exclude an overestimate of the decrease in Crs in the study population considering the possible presence of auto-PEEP, which may occur with an increasing respiratory rate (Marini, 2011). In severe cases of mechanically ventilated patients, a 70%–80% reduction in DLCO relative to the expected normal value was reported at 5–12 months (Krueger et al., 2023; van Willigen et al., 2023). Radiological pulmonary abnormalities have been described more than 100 days after the diagnosis of COVID-19 (Sonnweber et al., 2021). It appears reasonable to relate these decreased variables to pulmonary fibrosis development (Figure 5).
Several parameters obviously impact on the efficiency of gas exchanges in the air blood barrier.
The time dependent analysis that we performed allows an integrated view coherent with the parameters considered, highlighting the time dependence of gas exchanges in the air blood barrier, being diffusion limited for O2 and perfusion limited for CO2.
Data availability statement
The raw data supporting the conclusions of this article will be made available by the authors, without undue reservation.
Ethics statement
The requirement of ethical approval was waived by the Review Board of Arequipa Hospital for the studies involving humans because Data in the present paper refer to a retrospective study. The studies were conducted in accordance with the local legislation and institutional requirements. The ethics committee/institutional review board also waived the requirement of written informed consent for participation from the participants or the participants’ legal guardians/next of kin because observational nature of the study.
Author contributions
GM: Conceptualization, Data curation, Formal Analysis, Investigation, Methodology, Project administration, Supervision, Visualization, Writing–original draft, Writing–review and editing. ER: Conceptualization, Data curation, Formal Analysis, Investigation, Methodology, Project administration, Supervision, Visualization, Writing–original draft, Writing–review and editing. AM-D-C-T: Data curation, Investigation, Resources, Writing–review and editing. LP-Y: Data curation, Investigation, Resources, Writing–review and editing. NZ-D: Data curation, Investigation, Resources, Writing–review and editing. GZ-C: Data curation, Investigation, Resources, Writing–review and editing. EB: Conceptualization, Data curation, Formal Analysis, Investigation, Methodology, Project administration, Supervision, Visualization, Writing–original draft, Writing–review and editing.
Funding
The author(s) declare that no financial support was received for the research, authorship, and/or publication of this article.
Conflict of interest
The authors declare that the research was conducted in the absence of any commercial or financial relationships that could be construed as a potential conflict of interest.
The author(s) declared that they were an editorial board member of Frontiers, at the time of submission. This had no impact on the peer review process and the final decision.
Publisher’s note
All claims expressed in this article are solely those of the authors and do not necessarily represent those of their affiliated organizations, or those of the publisher, the editors and the reviewers. Any product that may be evaluated in this article, or claim that may be made by its manufacturer, is not guaranteed or endorsed by the publisher.
References
Aggarwal N. R., Brower R. G., Hager D. N., Thompson B. T., Netzer G., Shanholtz C., et al. (2018). Oxygen exposure resulting in arterial oxygen tensions above the protocol goal was associated with worse clinical outcomes in acute respiratory distress syndrome. Crit. Care Med. 46, 517–524. doi:10.1097/CCM.0000000000002886
Agostoni E., Mead J. (1964). “Statics of the respiratory system,” in Handbook of physiology respiration. Editors W. O. Fenn, and H. Rahn (Washington DC: American Physiological Society), 387–409.
Aydin S., Kantarci M., Karavas E., Unver E., Yalcin S., Aydin F. (2021). Lung perfusion changes in COVID-19 pneumonia: a dual energy computerized tomography study. Br. J. Radiol. 94 (1125), 20201380. doi:10.1259/bjr.20201380
Ball L., Robba C., Herrmann J., Gerard S. E., Xin Y., Mandelli M., et al. (2021). Lung distribution of gas and blood volume in critically ill COVID-19 patients: a quantitative dual-energy computed tomography study. Crit. Care. 25 (1), 214. doi:10.1186/s13054-021-03610-9
Barakat A. I., Lieu D. K., Gojova A. (2006). Secrets of the code: do vascular endothelial cells use ion channels to decipher complex flow signals? Biomaterials 27 (5), 671–678. doi:10.1016/j.biomaterials.2005.07.036
Beretta E., Grasso G. S., Forcaia G., Sancini G., Miserocchi G. (2019). Differences in alveolo-capillary equilibration in healthy subjects on facing O2 demand. Sci. Rep. 9 (1), 16693. doi:10.1038/s41598-019-52679-4
Beretta E., Romanò F., Sancini G., Grotberg J. B., Nieman G. F., Miserocchi G. (2021). Pulmonary interstitial matrix and lung fluid balance from normal to the acutely injured lung. Front. Physiol. 12, 781874. doi:10.3389/fphys.2021.781874
Brower R., Wise R. A., Hassapoyannes C., Bromberger-Barnea B., Permutt S. (1985). Effect of lung inflation on lung blood volume and pulmonary venous flow. J. Appl. Physiol. 58 (3), 954–963. doi:10.1152/jappl.1985.58.3.954
Brower R. G., Lanken P. N., MacIntyre N., Matthay M. A., Morris A., Ancukiewicz M., et al. (2004). Higher versus lower positive end-expiratory pressures in patients with the acute respiratory distress syndrome. N. Engl. J. Med. 351 (4), 327–336. doi:10.1056/NEJMoa032193
Chow C. W., Herrera Abreu M. T., Suzuki T., Downey G. P. (2003). Oxidative stress and acute lung injury. Am. J. Respir. Cell Mol. Biol. 29, 427–431. doi:10.1165/rcmb.F278
Dellacà R. L., Zannin E., Sancini G., Rivolta I., Leone B. E., Pedotti A., et al. (2008). Changes in the mechanical properties of the respiratory system during the development of interstitial lung edema. Respir. Res. 9 (1), 51. doi:10.1186/1465-9921-9-51
Fan E., Del Sorbo L., Goligher E. C., Hodgson C. L., Munshi L., Walkey A. J., et al. (2017). An official American thoracic society/European society of intensive care medicine/society of critical care medicine clinical practice guideline: mechanical ventilation in adult patients with acute respiratory distress syndrome. Am. J. Respir. Crit. Care Med. 195 (9), 1253–1263. doi:10.1164/rccm.201703-0548ST
Giraud R., Banfi C., Assouline B., De Charrière A., Bendjelid K. (2021). Very low blood flow carbon dioxide removal system is not effective in a chronic obstructive pulmonary disease exacerbation setting. Artif. organs 45 (5), 479–487. doi:10.1111/aor.13867
Glazier J. B., Hughes J. M., Maloney J. E., West J. B. (1969). Measurements of capillary dimensions and blood volume in rapidly frozen lungs. J. Appl. Physiol. 26 (1), 65–76. doi:10.1152/jappl.1969.26.1.65
Glenny R., Robertson H. T. (2011). Distribution of perfusion. Compr. Physiol. 1 (1), 245–262. doi:10.1002/cphy.c100012
Hamlington K. L., Bates J. H. T., Roy G. S., Julianelle A. J., Charlebois C., Suki B., et al. (2018). Alveolar leak develops by a rich-get-richer process in ventilator-induced lung injury. PloS one 13 (3), e0193934. doi:10.1371/journal.pone.0193934
Hamlington K. L., Ma B., Smith B. J., Bates J. H. (2016). Modeling the progression of epithelial leak caused by overdistension. Cell. Mol. Bioeng. 9 (1), 151–161. doi:10.1007/s12195-015-0426-3
Hopkins S. R. (2020). Ventilation/perfusion relationships and gas exchange: measurement approaches. Compr. Physiol. 10 (3), 1155–1205. doi:10.1002/cphy.c180042
Kallet R. H., Matthay M. A. (2013). Hyperoxic acute lung injury. Respir. Care 58, 123–141. doi:10.4187/respcare.01963
Kang H., Cancel L. M., Tarbell J. M. (2014). Effect of shear stress on water and LDL transport through cultured endothelial cell monolayers. Atherosclerosis 233, 682–690. doi:10.1016/j.atherosclerosis.2014.01.056
Karagiannidis C., Philipp A., Strassmann S., Schäfer S., Merten M., Windisch W. (2017). Extracorporeal CO2 elimination (ECCO2R) for hypercapnic respiratory failure: from pathophysiology to clinical application. Pneumologie 71 (4), 215–220. doi:10.1055/s-0042-124406
Kistler G. S., Caldwell P. R., Weibel E. R. (1967). Development of fine structural damage to alveolar and capillary lining cells in oxygen-poisoned rat lungs. J. Cell Biol. 32 (3), 605–628. doi:10.1083/jcb.32.3.605
Kolářová H., Ambrůzová B., Svihálková Šindlerová L., Klinke A., Kubala L. (2014). Modulation of endothelial glycocalyx structure under inflammatory conditions. Mediat. Inflamm. 2014, 694312. doi:10.1155/2014/694312
Kolliputi N., Shaik R. S., Waxman A. B. (2010). The inflammasome mediates hyperoxia-induced alveolar cell permeability. J. Immunol. 184, 5819–5826. doi:10.4049/jimmunol.0902766
Koyama S., Hildebrandt J. (1991). Air interface and elastic recoil affect vascular resistance in three zones of rabbit lungs. J. Appl. Physiol. 70 (6), 2422–2431. doi:10.1152/jappl.1991.70.6.2422
Krueger T., van den Heuvel J., van Kampen-van den Boogaart V., van Zeeland R., Mehagnoul-Schipper D. J., Barten D. G., et al. (2023). Pulmonary function three to five months after hospital discharge for COVID-19: a single centre cohort study. Sci. Rep. 13 (1), 681. doi:10.1038/s41598-023-27879-8
Lakshminarayanan S., Gardner T. W., Tarbell J. M. (2000). Effect of shear stress on the hydraulic conductivity of cultured bovine retinal microvascular endothelial cell monolayers. Curr. Eye Res. 21, 944–951. doi:10.1076/ceyr.21.6.944.6985
Marini J. J. (2011). Dynamic hyperinflation and auto-positive end-expiratory pressure: lessons learned over 30 years. Am. J. Respir. Crit. Care Med. 184 (7), 756–762. doi:10.1164/rccm.201102-0226PP
Matalon S., Egan E. A. (1981). Effects of 100% O2 breathing on permeability of alveolar epithelium to solute. J. Appl. Physiol. Respir. Environ. Exerc. Physiol. 50, 859–863. doi:10.1152/jappl.1981.50.4.859
Mazzag B. M., Tamaresis J. S., Barakat A. I. (2003). A model for shear stress sensing and transmission in vascular endothelial cells. Biophys. J. 84 (6), 4087–4101. doi:10.1016/S0006-3495(03)75134-0
Mazzuca E., Aliverti A., Miserocchi G. (2019). Understanding vasomotion of lung microcirculation by in vivo imaging. J. Imaging 5 (2), 22. doi:10.3390/jimaging5020022
Miserocchi G. (2023a). Early endothelial signaling transduction in developing lung edema. Life 13 (6), 1240. doi:10.3390/life13061240
Miserocchi G. (2023b). The impact of heterogeneity of the air-blood barrier on control of lung extravascular water and alveolar gas exchange. Front. Netw. Physiol. 3, 1142245. doi:10.3389/fnetp.2023.1142245
Miserocchi G., Beretta E. (2023). A century of exercise physiology: lung fluid balance during and following exercise. Eur. J. Appl. Physiol. 123 (1), 1–24. doi:10.1007/s00421-022-05066-3
Miserocchi G., Beretta E., Rivolta I., Bartesaghi M. (2022). Role of the air-blood barrier phenotype in lung oxygen uptake and control of extravascular water. Front. Physiol. 13, 811129. doi:10.3389/fphys.2022.811129
Miserocchi G., Messinesi G., Tana F., Passoni E., Adamo S., Romano R., et al. (2008). Mechanisms behind inter-individual differences in lung diffusing capacity. Eur. J. Appl. Physiol. 102 (5), 561–568. doi:10.1007/s00421-007-0625-2
Miserocchi G., Negrini D., Del Fabbro M., Venturoli D. (1993). Pulmonary interstitial pressure in intact in situ lung: transition to interstitial edema. J. Appl. Physiol. 74 (3), 1171–1177. doi:10.1152/jappl.1993.74.3.1171
Miserocchi G., Negrini D., Gonano C. (1991). Parenchymal stress affects interstitial and pleural pressures in in situ lung. J. Appl. Physiol. 71 (5), 1967–1972. doi:10.1152/jappl.1991.71.5.1967
Mohamed I., de Broucker V., Duhamel A., Giordano J., Ego A., Fonne N., et al. (2023). Pulmonary circulation abnormalities in post-acute COVID-19 syndrome: dual-energy CT angiographic findings in 79 patients. Eur. Radiol. 33 (7), 4700–4712. doi:10.1007/s00330-023-09618-9
Moriondo A., Marcozzi C., Bianchin F., Reguzzoni M., Severgnini P., Protasoni M., et al. (2012). Impact of mechanical ventilation and fluid load on pulmonary glycosaminoglycans. Respir. Physiol. Neurobiol. 181 (3), 308–320. doi:10.1016/j.resp.2012.03.013
Negrini D., Passi A., De Luca G., Miserocchi G. (1998). Proteoglycan involvement during development of lesional pulmonary edema. Am. J. Physiol. 274 (2), L203–L211. doi:10.1152/ajplung.1998.274.2.L203
Negrini D., Passi A., Moriondo A. (2008). The role of proteoglycans in pulmonary edema development. Intensive Care Med. 34 (4), 610–618. doi:10.1007/s00134-007-0962-y
Nieman G. F., Paskanik A. M., Bredenberg C. E. (1988). Effect of positive end-expiratory pressure on alveolar capillary perfusion. J. Thorac. Cardiovasc. Surg. 95 (4), 712–716. doi:10.1016/s0022-5223(19)35741-1
Nuckton T. J., Alonso J. A., Kallet R. H., Daniel B. M., Pittet J. F., Eisner M. D., et al. (2002). Pulmonary dead-space fraction as a risk factor for death in the acute respiratory distress syndrome. N. Engl. J. Med. 346 (17), 1281–1286. doi:10.1056/NEJMoa012835
Ochs M., Nyengaard J. R., Jung A., Knudsen L., Voigt M., Wahlers T., et al. (2004). The number of alveoli in the human lung. Am. J. Respir. Crit. Care Med. 169 (1), 120–124. doi:10.1164/rccm.200308-1107OC
Piiper J., Scheid P. (1981). Model for capillary-alveolar equilibration with special reference to O2 uptake in hypoxia. Respir. Physiol. 46 (3), 193–208. doi:10.1016/0034-5687(81)90121-3
Ramirez-Sandoval J. C., Castilla-Peón M. F., Gotés-Palazuelos J., Vázquez-García J. C., Wagner M. P., Merelo-Arias C. A., et al. (2016). Bicarbonate values for healthy residents living in cities above 1500 meters of altitude: a theoretical model and systematic review. High. Alt. Med. Biol. 17 (2), 85–92. doi:10.1089/ham.2015.0097
Rezoagli E., Bellani G. (2022). Monitoring lung injury severity and ventilation intensity during mechanical ventilation. Crit. Care. 23 (1), 412. doi:10.1186/s13054-019-2695-z
Rezoagli E., Laffey J. G., Bellani G. (2022). Monitoring lung injury severity and ventilation intensity during mechanical ventilation. Semin. Respir. Crit. Care Med. 43 (3), 346–368. doi:10.1055/s-0042-1748917
Rezoagli E., Magliocca A., Bellani G., Pesenti A., Grasselli G. (2021). Development of a critical care response - experiences from Italy during the coronavirus disease 2019 pandemic. Anesthesiol. Clin. 39 (2), 265–284. doi:10.1016/j.anclin.2021.02.003
Sill H. W., Chang Y. S., Artman J. R., Frangos J. A., Hollis T. M., Tarbell J. M. (1995). Shear stress increases hydraulic conductivity of cultured endothelial monolayers. Am. J. Physiol. 268 (2), H535–H543. doi:10.1152/ajpheart.1995.268.2.H535
Sinha P., Sanders R. D., Soni N., Vukoja M. K., Gajic O. (2013). Acute respiratory distress syndrome: the prognostic value of ventilatory ratio--a simple bedside tool to monitor ventilatory efficiency. Am. J. Respir. Crit. Care Med. 187 (10), 1150–1153. doi:10.1164/rccm.201211-2037LE
Sonnweber T., Sahanic S., Pizzini A., Luger A., Schwabl C., Sonnweber B., et al. (2021). Cardiopulmonary recovery after COVID-19: an observational prospective multicentre trial. Eur. Respir. J. 57 (4), 2003481. doi:10.1183/13993003.03481-2020
Spooner A. J., Corley A., Sharpe N. A., Barnett A. G., Caruana L. R., Hammond N. E., et al. (2014). Head-of-bed elevation improves end-expiratory lung volumes in mechanically ventilated subjects: a prospective observational study. Respir. Care 59 (10), 1583–1589. doi:10.4187/respcare.02733
van Willigen H. D. G., Wynberg E., Verveen A., Dijkstra M., Verkaik B. J., Figaroa O. J. A., et al. (2023). One-fourth of COVID-19 patients have an impaired pulmonary function after 12 months of disease onset. PloS one 18 (9), e0290893. doi:10.1371/journal.pone.0290893
Wagner P. D. (2008). The multiple inert gas elimination technique (MIGET). Intensive Care Med. 34 (6), 994–1001. doi:10.1007/s00134-008-1108-6
Weibel E. R. (1971). Oxygen effect on lung cells. Arch. Intern. Med. 128, 54–56. doi:10.1001/archinte.1971.00310190058005
Weibel E. R. (2015). On the tricks alveolar epithelial cells play to make a good lung. Am. J. Respir. Crit. Care Med. 191 (5), 504–513. doi:10.1164/rccm.201409-1663OE
Yi E., Sato S., Takahashi A., Parameswaran H., Blute T. A., Bartolák-Suki E., et al. (2016). Mechanical forces accelerate collagen digestion by bacterial collagenase in lung tissue strips. Front. Physiol. 7, 287. doi:10.3389/fphys.2016.00287
Keywords: dead space, respiratory compliance, gas exchanges, diffusion limitation, perfusion limitation, alveolar pressure, lung distension, mechanical ventilation
Citation: Miserocchi G, Rezoagli E, Muñoz-Del-Carpio-Toia A, Paricahua-Yucra LP, Zubieta-DeUrioste N, Zubieta-Calleja G and Beretta E (2024) Modelling lung diffusion-perfusion limitation in mechanically ventilated SARS-CoV-2 patients. Front. Physiol. 15:1408531. doi: 10.3389/fphys.2024.1408531
Received: 28 March 2024; Accepted: 18 June 2024;
Published: 12 July 2024.
Edited by:
Zhanqi Zhao, Guangzhou Medical University, ChinaReviewed by:
Yuxian Wang, Fudan University, ChinaCarmen Silvia Valente Barbas, University of São Paulo, Brazil
Copyright © 2024 Miserocchi, Rezoagli, Muñoz-Del-Carpio-Toia, Paricahua-Yucra, Zubieta-DeUrioste, Zubieta-Calleja and Beretta. This is an open-access article distributed under the terms of the Creative Commons Attribution License (CC BY). The use, distribution or reproduction in other forums is permitted, provided the original author(s) and the copyright owner(s) are credited and that the original publication in this journal is cited, in accordance with accepted academic practice. No use, distribution or reproduction is permitted which does not comply with these terms.
*Correspondence: Giuseppe Miserocchi, Z2l1c2VwcGUubWlzZXJvY2NoaUB1bmltaWIuaXQ=
†These authors share first authorship