- 1Department of Physical Education and Sports Teaching, Faculty of Sports Sciences, University of Cukurova, Adana, Türkiye
- 2Department of Physical Education and Sports Teaching, Faculty of Sports Sciences, University of Gedik, Istanbul, Türkiye
- 3Department of Coaching Education, Faculty of Sports Sciences, University of Cukurova, Adana, Türkiye
- 4Department of Physiology, Faculty of Medicine, University of Cukurova, Adana, Türkiye
- 5Department of Coaching Education, Faculty of Sports Sciences, University of Aksaray, Aksaray, Türkiye
Purpose: This study investigated the respiratory response and isocapnic buffering (IB) phase during an incremental exercise test to exhaustion in 16 child soccer players (11.9±0.9 years) and 18 youth soccer players (18.2±2.9 years).
Methods: The IB phase was calculated as the difference in oxygen uptake (VO2) between the respiratory compensation point (RCP) and metabolic threshold (MT) and expressed in either absolute or relative values.
Results: The maximal oxygen uptake (VO2max) was higher in youth players than in child players. For youth players, VO2max was measured at 55.9 ± 3.6 mL min−1 kg−1 and 74.9 ± 4.8 mL min−1 kg−0.75, while for child players, VO2max was 50.8 ± 4.1 mL min−1 kg−1 and 67.2 ± 6.1 mL min−1 kg−0.75 (p < 0.001). MT and RCP occurred at 69.8 ± 6.7% and 90.9 ± 6.9% of VO2max in child players and at 73.9 ± 5.1% and 91.5 ± 4.5% of VO2max in youth players, respectively. The two groups had no significant difference (p > 0.05). Absolute IB (10.6 ± 2.8 vs 9.7 ± 3.1 mL min−1 kg−1), relative IB (23.1 ± 5.7 vs 19.1 ± 6.1), and the ratio of RCP VO2 to MT VO2 (1.3 ± 0.09 vs 1.24 ± 0.09) were similar in child and youth players (p > 0.05). There was no difference in minute ventilation (V̇E, mL min−1 kg−1) and respiratory exchange ratio during exercise between the two groups (p > 0.05). During exercise, respiratory frequency, ventilatory equivalent for carbon dioxide (VE/VCO2) and oxygen (VE/VO2), VE/VCO2 slope, end-tidal O2 pressure were higher in child players than in youth players, while tidal volume (L kg−1), O2 pulse, and end-tidal CO2 pressure were lower (p < 0.05).
Conclusion: Despite differences in aerobic capacity and ventilatory response to exercise, child players showed similar IB phase as youth players. Although child players have lower ventilation efficiency than youth players, the higher ventilation response for a given VCO2 may provide an advantage in regulating acid-base balance during intense exercise.
Introduction
Maximal oxygen uptake (VO2max), ventilatory anaerobic threshold (metabolic threshold), and respiratory compensation point (RCP) are important physiological measurements used to determine aerobic fitness, training adaptations, and training planning in soccer players (Cunha et al., 2016). Metabolic threshold (MT) based on gas exchange measurements is considered the point at which the contribution of anaerobic metabolism to overall metabolism begins to increase during incremental exercise (Wasserman et al., 2012). When MT is exceeded during incremental exercise testing, non-oxidative CO2 production increases due to H+ buffering by blood bicarbonate (Wasserman et al., 2012). As exercise intensity increases further, the buffering system eventually becomes overwhelmed, causing a decrease in blood pH and further stimulation of hyperventilation (Binder et al., 2008; Oshima et al., 1998; Wasserman et al., 2012). This induces a second ventilatory threshold called the respiratory compensation point (RCP) (Meyer et al., 2004; Whipp et al., 1989). Physiologically, RCP marks the failure of the body’s buffering mechanism (Meyer et al., 2004). The period between MT and RCP is called the isocapnic buffering (IB) and represents the compensation phase for exercise-induced metabolic acidosis (Takano, 2000; Whipp et al., 1989). The period between the RCP and the exhaustion point of the exercise is called the hypocapnic hyperventilation (HHV) phase (Chicharro et al., 2000; Oshima et al., 1998).
The IB phase is assumed to be an indicator of cardiopulmonary function in athletes, healthy individuals, and patients with cardiac diseases (Yen et al., 2018). Although the IB phase is considered an indicator of endurance performance in healthy individuals and athletes (Oshima et al., 1998), it appears to be more associated with performance in activities that lead to a pronounced elevation in blood lactate and accompanying metabolic acidosis (Bentley et al., 2005). The range of the IB phase is related to factors such as buffering capacity, lactate kinetics, and sensitivity of the carotid bodies (Whipp et al., 1989). These factors are adaptive variables, and their response to physical training depends on exercise planning (e.g., intensity and volume) (Edge et al., 2006; Hasanli et al., 2015). Therefore, the IB phase can be a useful tool for coaches and physiologists to characterize metabolic adaptations induced by a specific training regimen (Hasanli et al., 2015). Although the IB phase decreases with age, it is longer in trained athletes independent of age (Lenti et al., 2011). While differences in respiratory response to maximal exercise have been documented between children and adults (Prado et al., 2010; Cooper et al., 1987; Weston et al., 2021), the IB phase has not received attention in children.
Ventilatory responses to exercise in the pediatric population appear to be maturity-dependent (Giardini et al., 2011; Rowland and Cunningham, 1997). Compared to teenagers, younger children have been reported to ventilate more during exercise to eliminate a given amount of CO2 to maintain PaCO2 at a lower level (Cooper et al., 1987; Gratas-Delamarche et al., 1993; Nagano et al., 1998). Smaller body size, smaller CO2 storage capacity, and immaturity of the respiratory center are considered responsible for age-related differences (Ohuchi et al., 1999; Cooper et al., 1987; Weston et al., 2021). Compared to adults, children show a greater increase in VE relative to VCO2 during exercise, reflecting lower ventilation efficiency (Takken et al., 2020; Giardini et al., 2011). Children’s respiratory centers have been suggested to be more sensitive to CO2 during exercise than adults (Gratas-Delamarche et al., 1993; Nagano et al., 1998). However, ventilatory regulation related to the change in acid-base balance induced by lactic acidosis has been shown to be more efficient in boys than men during strenuous exercise (Ratel et al., 2002).
Although it has been shown that aging reduces the IB phase (Lenti et al., 2011), to our knowledge, the IB phase in children has not been studied previously. Children may exhibit different IB phases compared to adults due to children’s immature glycolytic capacity (Reybrouck et al., 1985; Fellmann and Coudert, 1994). On the other hand, children have a higher ventilatory response during exercise (Cooper et al., 1987; Gratas-Delamarche et al., 1993), which may enhance the IB phase by assisting in the respiratory compensation of pH. We hypothesized that instead of VO2max, child soccer players might extend the IB phase by using the compensatory mechanisms mentioned above and increase their exercise tolerance compared to youth soccer players. This study aimed to examine the respiratory response and IB phase during incremental exercise in child and youth soccer players.
Materials and methods
Participants
Sixteen boy soccer players (11.9 ± 0.9 years, 150.6 ± 4.6 cm and 39.03 ± 5.3 kg) and eighteen youth male soccer players (18.2 ± 2.9 years, 174.5 ± 6.5 cm, 67.9 ± 7.3 kg) from the Turkish Regional Soccer League participated in the study. The child players had at least 1 year of soccer training and match experience. They had two soccer training sessions and one official match per week. The youth players had trained soccer 3–5 times weekly for at least 4 years. They also played at least one official match per week during the competitive season. Consent forms were reviewed and signed by the players and their parents in accordance with the Declaration of Helsinki. This study was approved by the Ethics Committee of the Çukurova University Faculty of Medicine (83/2018/22). Each player avoided any exercise until 24 h before the test measurement.
The age of peak linear growth (age at peak height velocity) is an indicator of somatic maturity and represents the time of maximum growth in height during adolescence. The biological maturity of child players was assessed noninvasively by calculating years from peak height velocity (PHV) using a sex-appropriate equation derived from body mass, standing height, sitting height, leg length measurements, and age (Mirwald et al., 2002). This method is a reliable (standard error of estimate ±0.592 years), nonintrusive, inexpensive, and simple way of assessing biological maturity and has the potential to be incorporated into methodologies for predicting adult height in adolescent children, free of growth-limiting diseases (Mirwald et al., 2002; Sherar et al., 2005). Maturity offset (years) was calculated by subtracting the chronological age at the time of measurement from the age in PHV, with negative values (−) interpreted as pre-PHV and positive values (+) as post-PHV. As a result of the calculations, the maturity offset of child players was found to be −1.72 ± 0.67 years.
Incremental exercise test
The test was performed on a treadmill (HP Cosmos, Nussdorf–Traunstein, Germany), and breath-by-breath gas measurements were taken throughout the exercise using an indirect calorimetric system (PFT Cosmed, Rome, Italy) calibrated before each session. The heart rate was recorded continuously by telemetry using a heart rate monitor (Cosmed, Rome, Italy). Each player performed a standardized warm-up consisting of a 5 min run at their own pace followed by about 3 min stretching. Following the warm-up period, players performed an incremental test protocol at a constant 1% grade incline, with speed increments of 1 km h−1 every minute until they could no longer keep the running pace. Child players started the test at 5 km h−1, while youth players started at 8 km h−1. The athletes were instructed to run until voluntary exhaustion and given strong verbal encouragement throughout the test to elicit their best performance. Attainment of volitional exhaustion was confirmed if two of the following criteria were satisfied: 1) a plateau in VO2 despite increasing speed (defined as an increase of no more than 2 mL kg−1 min−1), 2) a respiratory exchange ratio (RER) above 1.10, and 3) an HR within ten beats per minute of the age-predicted maximum HR (220 – age) (Trowbridge et al., 1997). Breath-by-breath data were averaged over 10-s periods and then smoothed by eight steps, and these values were used for further analysis. The VO2max was determined as the highest 10-s VO2 value reached during the test. Data corresponding to 1) baseline (beginning of the exercise), 2) 50% of the range from baseline to MT (50% MT), 3) MT, 4) 50% of the range from AT to RCP (50%∆ MT-RCP), 6) RCP and 6) exhaustion were used to evaluate responses to exercise. VE/VCO2 slope (VE versus VCO2 slope) was calculated using linear regression from the beginning of the exercise to exhaustion and from the beginning to RCP. Oxygen pulse (O2 pulse) was calculated as VO2/HR. VO2, VCO2, O2 pulse, VE, and tidal volume (TV) per kilogram of body mass were expressed with relative values. Additionally, VO2 at baseline, MT, RCP, and maximal was expressed according to an allometric scaling exponent of 0.75 for body mass (mL min−1 kg−0.75), as recommended in previous studies (Bergh et al., 1991; Cunha et al., 2008), to eliminate the effects of body mass on adults and children.
The MT and RCP were determined using the V-slope method (Beaver et al., 1986; Wasserman et al., 2012). The MT and RCP were defined as the VO2 value corresponding to the intersection of two linear regression lines, indicating the disproportionate increase in the VCO2 versus the VO2 and the VE versus the VCO2 relationships, respectively (Figure 1). Additionally, a visual identification technique was used to increase the identification accuracy of the MT and RCP. The MT was determined using the criterion of increases in the ventilatory equivalent for oxygen (VE/VO2) and end-tidal oxygen pressure (PETO2) without simultaneous changes in the ventilatory equivalent for carbon dioxide (VE/VCO2) and end-tidal carbon dioxide pressure (PETCO2), while the RCP corresponded to an increase in the VE/VCO2 and decrease in the PETCO2 (Wasserman et al., 2012; Binder et al., 2008). Two independent investigators performed data analysis. If there was disagreement, the opinion of a third investigator was sought.
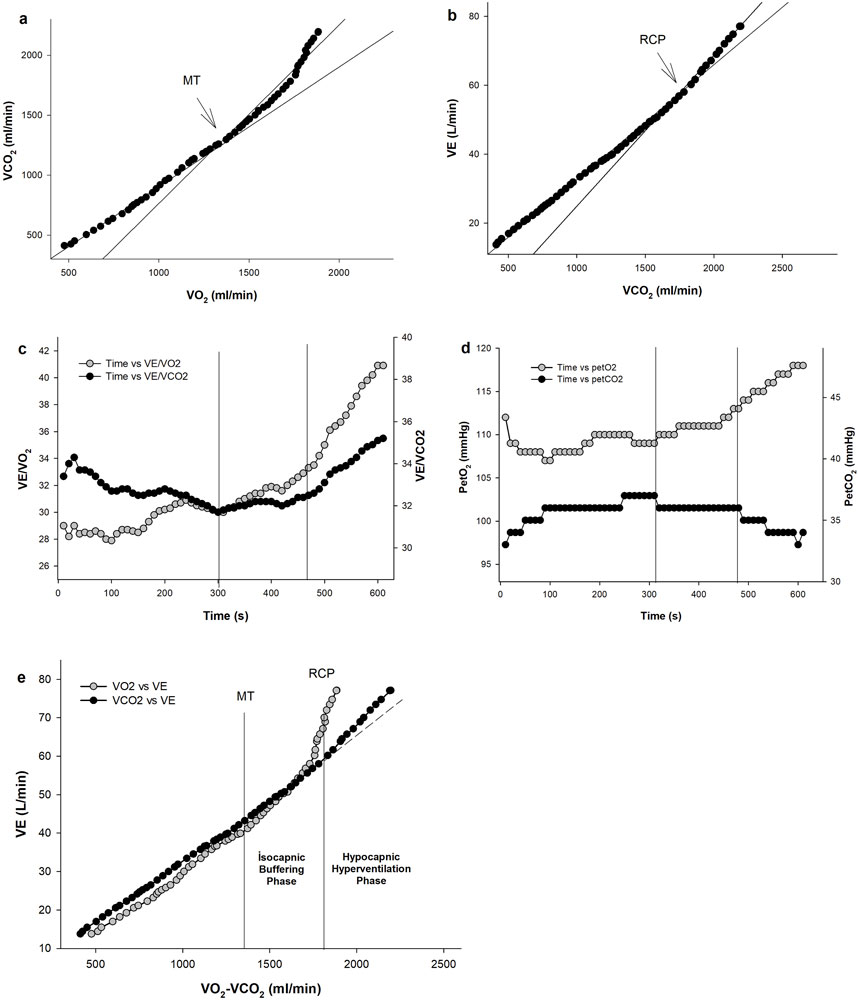
Figure 1. An example of gas exchange and ventilatory responses to incremental exercise in one representative child player. Determination of metabolic threshold (MT) and respiratory compensation point (RCP) using the V-slope method from VCO2 vs VO2 plot (A) and VE vs VCO2 plot (B). The ventilatory equivalent for O2 (VE/VO2) and CO2 (VE/VCO2) vs time plot (C), end-tidal O2 (PETO2) and CO2 pressure (PETCO2) vs time plot (D) were used as the secondary criteria to determine MT and RCP. Plot of ventilation (VE) vs VO2 and VCO2 (E) showing the isocapnic buffering and hypocapnic hyperventilation phases.
The IB was calculated as the difference in VO2 between the RCP and MT and expressed as an absolute value (Oshima et al., 1998). Additionally, relative IB was calculated as previously described by Röcker et al. (1994).
where
Statistical analyses
The assumption of normality was assessed through the Shapiro-Wilk test. The two groups were compared using an independent t-test and Mann-Whitney U test for normally and non-normally distributed data, respectively. Effect sizes were calculated for independent samples using Cohen’s d method (Thalheimer and Cook, 2002). Effect sizes were interpreted as negligible (d ≥ 0.2), small (0.2 ≤ d ≤ 0.5), medium (0.5 ≤ d ≤ 0.8) or large (0.8 ≥ d). Linear regression analyses were performed using the Sigma Plot program (Sigma Plot 12.0, Systat Software Inc., Chicago, United States). IBM SPSS 21 software (IBM SPSS Statistics 21 Inc., Chicago, IL) was used for the statistical analyses. Data are reported as the means ± standard deviation (SD). Statistical significance was accepted at p < 0.05.
Results
When the maturation status of child players was evaluated noninvasively, it was determined that they were in the pre-PHV period. VO2max, maximal VE, and exercise duration were higher in youth players compared with child players (p < 0.05) (Table 1). MT and RCP occurred at a similar percentage of VO2max in the two groups (p > 0.05). The absolute and mass-adjusted relative VO2 and VCO2 values at MT, RCP, and maximal were higher in youth players than in child players (p < 0.05). While relative VO2 and VCO2 at baseline were higher in child players than in youth players (p < 0.05), the absolute values did not differ between the two groups (p > 0.05). VO2 (mL min−1 kg−0.75), expressed according to allometric scaling, was higher in youth players than in child players at MT, RCP, and maximal, while it was higher in child players than in youth players at baseline (p < 0.05). RER values were not different between child and youth players (p > 0.05). The HR values were higher at baseline in child players and at MT in youth players (p < 0.05) and were not different between the two groups at RCP and maximal (p > 0.05).
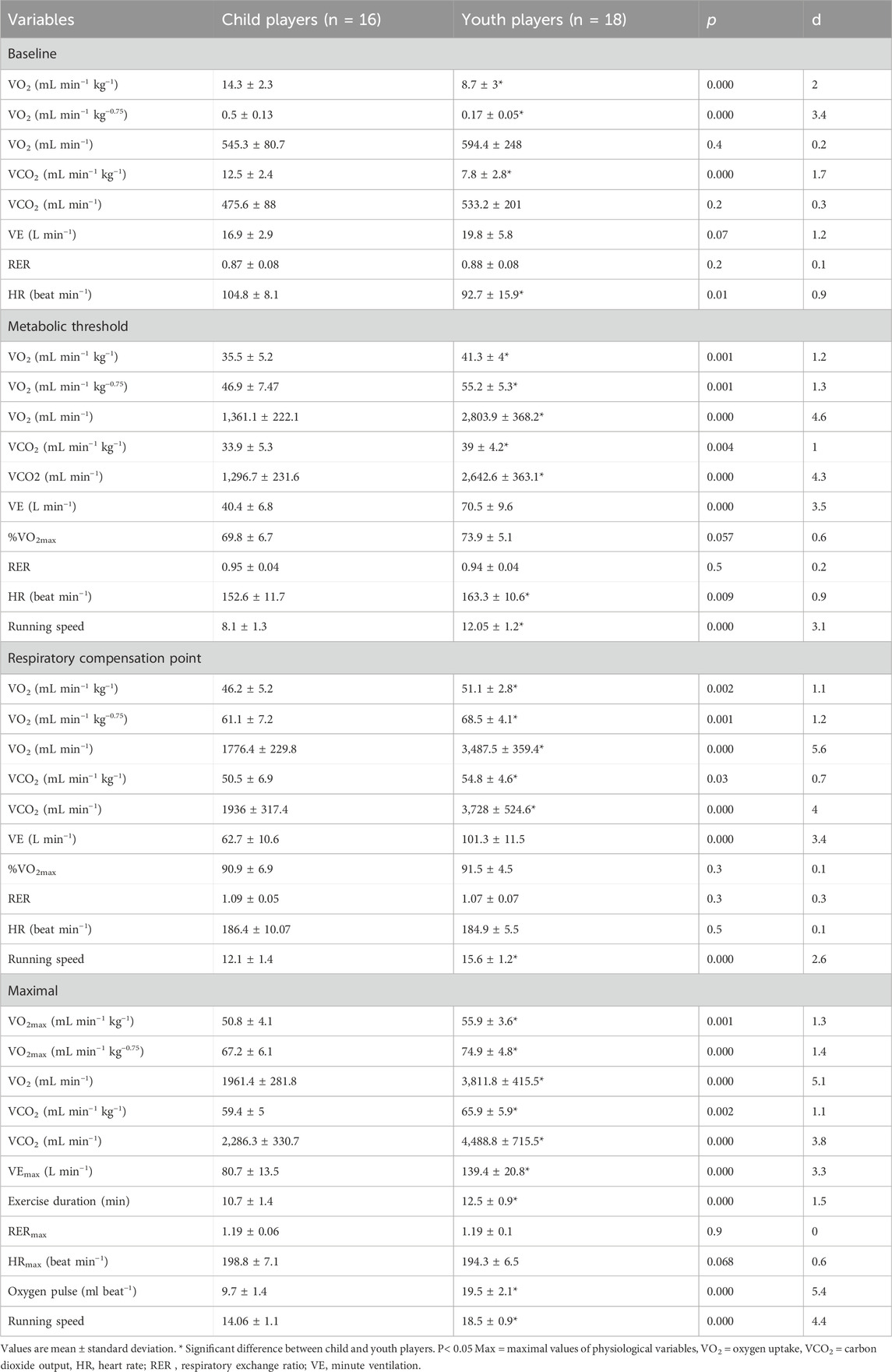
Table 1. Comparison of physiological parameters between child and youth players at baseline, anaerobic threshold, respiratory compensation point, and maximal.
Relative VE was higher in child players at baseline (p < 0.05), but there was no difference between the two groups at 50%MT, MT, 50%∆MT-RCP, RCP, and maximal (p > 0.05) (Figure 2). Youth players had greater relative TV than child players at 50%MT, MT, 50%∆MT-RCP, and RCP (p < 0.05). Child players had higher respiratory frequency than youth players at baseline, MT, 50%∆MT-RCP, RCP, and maximal (p < 0.05). Relative O2 pulse was higher in child players at baseline and higher in youth players at 50%MT, 50%∆MT-RCP, RCP, and maximal (p < 0.05).
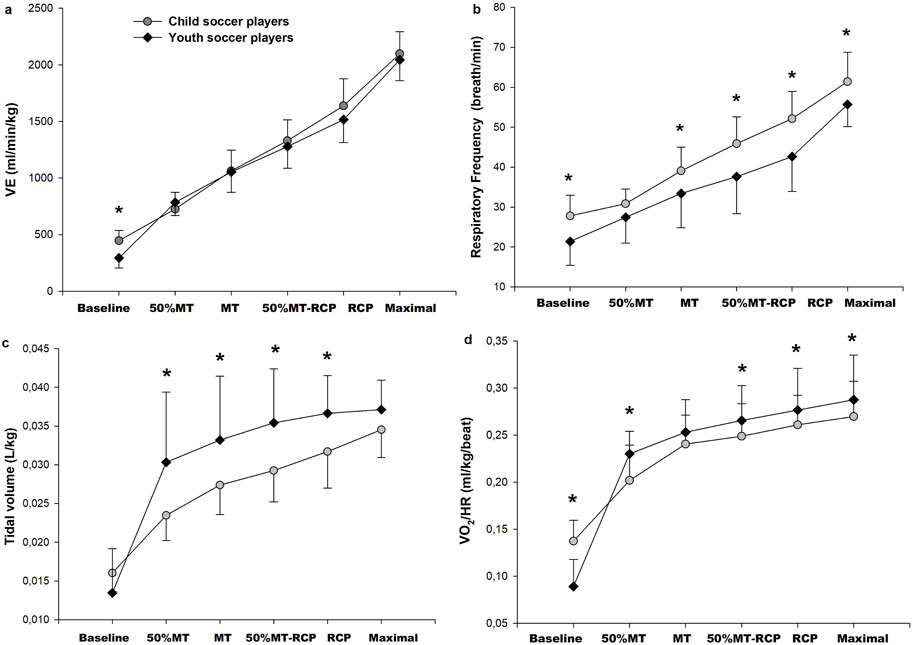
Figure 2. Mass-adjusted relative minute ventilation (VE) (A), respiratory frequency (B), relative tidal volume (C), O2 pulse (VO2 HR−1 mL kg−1 beat−1) (D) responses to incremental exercise to exhaustion in child players (n = 16; gray circles) and youth players (n = 18; black square). *Significant difference between child and youth players, p < 0.05.
Child players had lower PETCO2 and higher PETO2, VE/VCO2, and VE/VO2values compared to youth players during exercise (p < 0.05), with no difference between the two groups at baseline (p > 0.05) (Figure 3). There were no significant differences between child and youth players in RCP/MT VO2, absolute and relative IBP, and HHP (p > 0.05) (Table 2). VE/VCO2 slope and VE/VCO2RCP slope were higher in child players than in youth players (p < 0.05).
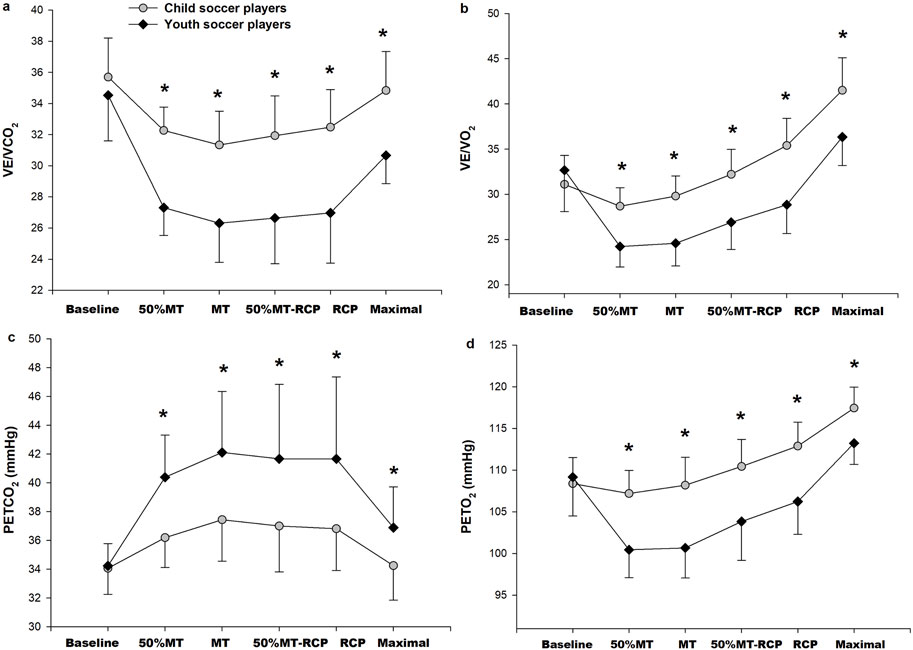
Figure 3. Ventilatory equivalent for CO2 (VE/VCO2) (A), Ventilatory equivalent for O2 (VE/VO2) (B), end-tidal CO2 pressure (PETCO2; (C) and end-tidal O2 pressure (PETO2) (D) responses to incremental exercise to exhaustion in child players (n = 16; gray circles) and youth players (n = 18; black square). *Significant difference between child and youth players, p < 0.05.
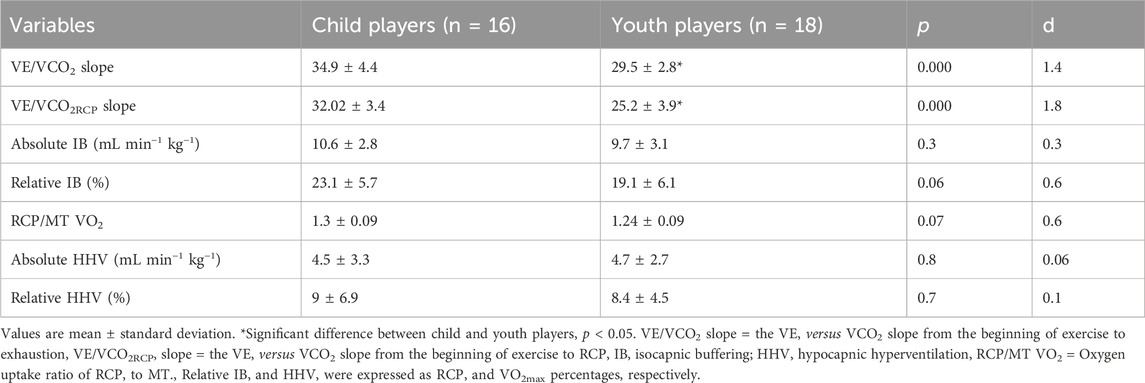
Table 2. Comparison of VE versus VCO2 slope, isocapnic buffering, and hypocapnic hyperventilation between child and youth players.
Discussion
The main findings of the present study are as follows: 1) child players showed an IB phase similar to youth players, 2) child players had lower aerobic capacity than youth players, and 3) child players had different ventilatory responses to incremental exercise compared to youth players. The results confirmed our hypothesis and indicated that child players exhibited similar IB phases despite having lower relative VO2max compared to youth players. MT and RCP occurred at a similar VO2max fraction in child and youth players, suggesting that both thresholds significantly impact the IB phase.
Respiratory frequency, VE/VCO2 slope, ventilatory equivalent for CO2 and O2, and PETO2 values of child players were higher, while TV and PETCO2 values were lower than youth players. These findings may be interpreted as child players’ lower ventilation efficiency during exercise than youth players (Ohuchi et al., 1999; Takken et al., 2020). Similar to the findings of previous studies (Prado et al., 2010; Gratas-Delamarche et al., 1993), the VE/VCO2 slope and the VE/VCO2 were found to be higher in child players than in youth players. Even though VE relative to body mass was not different between the two groups, lower relative VO2 and VCO2 values of child players throughout exercise indicate increased ventilatory response during exercise. Consistent with our findings, lower TV in children compared with adults during exercise is compensated by a higher respiratory frequency (Takken et al., 2020; Rowland and Cunningham, 1997; Prado et al., 2010). The lower PETCO2 observed in child players in this study compared to youth players during incremental exercise is consistent with previous studies (Prado et al., 2010; Ellis et al., 2017; Nagano et al., 1998; Cooper et al., 1987; Weston et al., 2021). Higher central ventilatory neural drive for children may lead to higher ventilatory rates for a given metabolic demand (Gratas-Delamarche et al., 1993).
The high VO2max values of youth players indicate that they had a better aerobic capacity than child players. VO2max may increase with biological maturation in regularly exercising individuals (Paterson et al., 1987; Baxter-Jones et al., 1993). Since child players have a shorter soccer training history than youth players, their lower VO2max may be an expected finding. Two other important variables related to aerobic fitness, MT and RCP, occurred at similar VO2max fractions in child and youth players. Consistent with our findings, studies have shown that relative RCP values in boys are similar to youth men (Cunha et al., 2008) or men (Klentrou et al., 2006), corresponding to approximately 80%–91% of VO2max (or VO2peak) (Weston et al., 2021; Cunha et al., 2008; Klentrou et al., 2006). In children, MT varies widely, with approximately 58%–73% of VO2max (Cunha et al., 2008; Paterson et al., 1987; Reybrouck et al., 1985; Mahon et al., 1998; Mahon and Vaccaro, 1989). However, active boys have a higher ventilatory anaerobic threshold than less active boys (Reybrouck et al., 1985; Weymans and Reybrouck, 1989). Although some studies have reported that MT occurs at a higher percentage of VO2max in children than in adults (Klentrou et al., 2006; Weston et al., 2021), other studies similar to our study found no difference in MT between groups (Mahon et al., 1998; Cunha et al., 2016). Individuals’ training status and training history from childhood appear to play an important role in MT values (Paterson et al., 1987). Although youth players had a twice higher weekly training volume than child players due to more training sessions, the relative intensity of each soccer training was similar. The metabolic pathways used during training sessions and matches were probably equivalent in terms of physiological strain. Since relative MT and RCP were similar between child and youth players, the physiological mechanisms that identify these thresholds may differ for these age groups, and exercise intensity may be the main determinant of these two variables.
During the IB phase, both aerobic and anaerobic systems simultaneously produce energy at varying rates (Hasanli et al., 2015). If the aerobic metabolic rate is relatively high after MT, the duration of the IB phase may be longer due to the slow increase in metabolic acidosis (Yen et al., 2018). The IB phase of youth soccer players is consistent with previous research on trained men (Lenti et al., 2011; Chicharro et al., 2000; Eryılmaz et al., 2018; Korkmaz Eryılmaz et al., 2018). We found that even though youth players had higher VO2max, the absolute and relative IB range was similar to that of child players. In addition to that, the ratio of RCP VO2 to MT VO2 can be used to compare buffering capacity between groups of different ages (Kominami et al., 2022), and we did not find any significant difference in this ratio between youth and child players in our study. The present and previously published data (Hasanli et al., 2015; Korkmaz Eryılmaz et al., 2018; Röcker et al., 1994) indicate that the VO2max value may not be enough to interpret IB and buffering capacity.
Physical training may directly affect the IB phase by changing MT and RC (Kominami et al., 2022; Lenti et al., 2011; Chicharro et al., 2000; Eryılmaz et al., 2018). Although there was no statistically significant difference in our study, child players tend to have a larger IB phase due to their lower relative MT than youth players. High-intensity training may enhance the IB phase by shifting RCP with minimal or no change in MT (Eryılmaz et al., 2018; Oshima et al., 1998). Several studies have shown higher lactate accumulation during the IB phase in anaerobic-trained athletes than in endurance-trained athletes (Hasanli et al., 2015; Röcker et al., 1994). Although we did not directly measure blood lactate, the maximal RER may reflect the lactate concentration (Stringier et al., 1995). Unlike adults, children have a muscle metabolic profile better equipped for the aerobic energy pathway rather than the glycolytic energy pathway (Fellmann and Coudert, 1994). Therefore, maximal RER values of children at the end of exercise can be expected to be lower than adults. RER values at the same relative exercise intensities did not differ significantly between child and youth players, and our data is consistent with other studies (Ohuchi et al., 1999; Giardini et al., 2011). Even though the training history of the child players was lower than that of the youth players, both groups were training specifically for soccer. It is known that regular physical training can increase the glycolytic capacity of skeletal muscles in boys (Eriksson, 1972; Paterson et al., 1987). Since youth soccer players have a more extended training history than child players, similar RER values indicate that training modalities are important for determining metabolic profiles.
Ratel et al. (2002) demonstrated a smaller decrease in blood pH relative to an increase in lactate in boys compared with men during repeated sprint exercise. This relatively small increase in blood [H+] in boys was explained by higher ventilatory regulation related to the change in acid-base balance compared to men during repeated sprints (Ratel et al., 2002). Children may be better able to tolerate lactic acidosis with a higher ventilatory response for a given CO2 (Ratel et al., 2002). This may explain the mechanism of similar IB phases between child players with lower aerobic capacity and youth players. Future studies are necessary to explain this physiological phenomenon in child and youth athletes with matched aerobic capacities or specific training programs.
Conclusion
Child players had lower aerobic capacity and hyperventilatory response to exercise than youth players. MT and RCP occurred at similar VO2max fractions in child and youth players, and both of these groups showed similar IB phases. Respiratory frequency, ventilatory equivalent for CO2 and O2, and PETO2 values of child players were higher than youth players. Hyperventilation reduced PETCO2 values, which may correspond to a reduction of PaCO2. Therefore, a higher ventilatory response during exercise may help children buffer pH. Longitudinal studies are needed to confirm the responsiveness of the IB phase to physical training in children.
Data availability statement
The raw data supporting the conclusions of this article will be made available by the authors, without undue reservation.
Ethics statement
The studies involving humans were approved by the Çukurova University Faculty of Medicine Clinical Research Ethics Committee. The studies were conducted in accordance with the local legislation and institutional requirements. Written informed consent for participation in this study was provided by the participants’ legal guardians/next of kin.
Author contributions
SKE: Conceptualization, Methodology, Writing–original draft, Writing–review and editing, Data curation, Formal Analysis, Project administration. SK: Data curation, Formal Analysis, Project administration, Validation, Writing–review and editing. CB: Data curation, Formal Analysis, Investigation, Methodology, Validation, Writing–review and editing. ÖG: Data curation, Formal Analysis, Investigation, Writing–original draft. AK: Data curation, Formal Analysis, Investigation, Methodology, Validation, Visualization, Writing–review and editing. ÇÖ: Data curation, Investigation, Validation, Visualization, Writing–review and editing. KÖ: Validation, Visualization, Writing–review and editing. MK: Data curation, Investigation, Visualization, Writing–review and editing. ÜA: Formal Analysis, Investigation, Writing–review and editing. SK: Conceptualization, Methodology, Supervision, Writing–review and editing.
Funding
The author(s) declare that no financial support was received for the research, authorship, and/or publication of this article.
Conflict of interest
The authors declare that the research was conducted in the absence of any commercial or financial relationships that could be construed as a potential conflict of interest.
Publisher’s note
All claims expressed in this article are solely those of the authors and do not necessarily represent those of their affiliated organizations, or those of the publisher, the editors and the reviewers. Any product that may be evaluated in this article, or claim that may be made by its manufacturer, is not guaranteed or endorsed by the publisher.
References
Baxter-Jones A., Goldstein H., Helms P. (1993). The development of aerobic power in young athletes. J. Appl. Physiol. 75 (3), 1160–1167. doi:10.1152/jappl.1993.75.3.1160
Beaver W. L., Wasserman K., Whipp B. J. (1986). A new method for detecting anaerobic threshold by gas exchange. J. Appl. Physiol. 60 (6), 2020–2027. doi:10.1152/jappl.1986.60.6.2020
Bentley D. J., Vleck V. E., Millet G. P. (2005). The isocapnic buffering phase and mechanical efficiency: relationship to cycle time trial performance of short and long duration. Can. J. Appl. Physiol. 30 (1), 46–60. doi:10.1139/h05-104
Bergh U., Sjodin B., Forsberg A., Svedenhag J. (1991). The relationship between body mass and oxygen uptake during running in humans. Med. Sci. Sports Exerc. 23 (2), 205–211. doi:10.1249/00005768-199102000-00010
Binder R. K., Wonisch M., Corra U., Cohen-Solal A., Vanhees L., Saner H., et al. (2008). Methodological approach to the first and second lactate threshold in incremental cardiopulmonary exercise testing. Eur. J. Prev. Cardiol. 15 (6), 726–734. doi:10.1097/HJR.0b013e328304fed4
Chicharro J. L., Hoyos J., Lucía A. (2000). Effects of endurance training on the isocapnic buffering and hypocapnic hyperventilation phases in professional cyclists. Br. J. Sports. Med. 34 (6), 450–455. doi:10.1136/bjsm.34.6.450
Cooper D. M., Kaplan M. R., Baumgarten L., Weiler-Ravell D., Whipp B. J., Wasserman K. (1987). Coupling of ventilation and CO2 production during exercise in children. Pediatr. Res. 21 (6), 568–572. doi:10.1203/00006450-198706000-00012
Cunha G. D. S., Vaz M. A., Geremia J. M., Leites G. T., Baptista R. R., Lopes A. L., et al. (2016). Maturity status does not exert effects on aerobic fitness in soccer players after appropriate normalization for body size. Pediatr. Exerc. 28 (3), 456–465. doi:10.1123/pes.2015–0133
Cunha G. S., Célia F. G., Ribeiro J. L., Oliveira A. R. (2008). Effects of the biological maturation on maximal oxygen uptake and ventilatory breakpoint of Brazilian soccer players. Gazz. Med. Ital-Arch. Sci. Med. 167 (2), 43–49.
Edge E. J., Bishop D., Hill-Haas S., Dawson B., Goodman C. (2006). Comparison of muscle buffer capacity and repeated-sprint ability of untrained, endurance-trained and team-sport athletes. Eur. J. Appl. Physiol. 96, 225–234. doi:10.1007/s00421-005-0056-x
Ellis L. A., Ainslie P. N., Armstrong V. A., Morris L. E., Simair R. G., Sletten N. R., et al. (2017). Anterior cerebral blood velocity and end-tidal CO2 responses to exercise differ in children and adults. Am. J. Physiol. Heart Circ. 312 (6), H1195–H1202. doi:10.1152/ajpheart.00034.2017
Eriksson B. O. (1972). Physical training, oxygen supply and muscle metabolism in 11-13-year-old boys. Acta Physiol. scund. Suppl. 384, 1–48.
Eryılmaz S. K., Kaynak K., Polat M., Aydoğan S. (2018). Effects of repeated sprint training on isocapnic buffering phase in volleyball players. Rev. Bras. Med. Esporte. 24, 286–290. doi:10.1590/1517-869220182404185842
Fellmann N., Coudert J. (1994). Physiology of muscular exercise in children. Arch. Pediatr. 1 (9), 827–840.
Giardini A., Odendaal D., Khambadkone S., Derrick G. (2011). Physiologic decrease of ventilatory response to exercise in the second decade of life in healthy children. Am. Heart J. 161 (6), 1214–1219. doi:10.1016/j.ahj.2011.03.008
Gratas-Delamarche A., Mercier J., Ramonatxo M., Dassonville J., Prefaut C. (1993). Ventilatory response of prepubertal boys and adults to carbon dioxide at rest and during exercise. Eur. J. Appl. Physiol. Occup. Physiol. 66 (1), 25–30. doi:10.1007/BF00863395
Hasanli M., Nikooie R., Aveseh M., Mohammad F. (2015). Prediction of aerobic and anaerobic capacities of elite cyclists from changes in lactate during isocapnic buffering phase. J. Strength Cond. Res. 29 (2), 321–329. doi:10.1519/JSC.0000000000000640
Klentrou P., Nishio M. L., Plyley M. (2006). Ventilatory breakpoints in boys and men. Pediatr. Exerc. 18 (2), 216–225. doi:10.1123/pes.18.2.216
Kominami K., Imahashi K., Katsuragawa T., Murakami M., Akino M. (2022). The ratio of oxygen uptake from ventilatory anaerobic threshold to respiratory compensation point is maintained during incremental exercise in older adults. Front. Physiol. 13, 769387. doi:10.3389/fphys.2022.769387
Korkmaz Eryılmaz S., Polat M., Soyal M., Aydoğan S. (2018). The relationship between the isocapnic buffering phase and ventilatory threshold in endurance athletes and team sport athletes during an incremental exercise test. Ann. Appl. Sport. Sci. 6 (1), 1–9. doi:10.29252/aassjournal.6.1.1
Lenti M., De Vito G., Di Palumbo A. S., Sbriccoli P., Quattrini F. M., Sacchetti M. (2011). Effects of aging and training status on ventilatory response during incremental cycling exercise. J. Strength Cond. Res. 25 (5), 1326–1332. doi:10.1519/JSC.0b013e3181d99061
Mahon A. D., Gay J. A., Stolen K. Q. (1998). Differentiated ratings of perceived exertion at ventilatory threshold in children and adults. Eur. J. Appl. Physiol. Occup. Physiol. 78, 115–120. doi:10.1007/s004210050395
Mahon A. D., Vaccaro P. (1989). Ventilatory threshold and VO2max changes in children following endurance training. Med. Sci. Sports Exerc. 21 (4), 425–431.
Meyer T., Faude O., Scharhag J., Urhausen A., Kindermann W. (2004). Is lactic acidosis a cause of exercise induced hyperventilation at the respiratory compensation point? Br. J. Sports Med. 38 (5), 622–625. doi:10.1136/bjsm.2003.007815
Mirwald R. L., Baxter-Jones A. D., Bailey D. A., Beunen G. P. (2002). An assessment of maturity from anthropometric measurements. Med. Sci. Sports. Exerc. 34 (4), 689–694. doi:10.1097/00005768-200204000-00020
Nagano Y., Baba R., Kuraishi K., Yasuda T., Ikoma M., Nishibata K., et al. (1998). Ventilatory control during exercise in normal children. Pediat. Res. 43 (5), 704–707. doi:10.1203/00006450-199805000-00021
Ohuchi H., Kato Y., Tasato H., Arakaki Y., Kamiya T. (1999). Ventilatory response and arterial blood gases during exercise in children. Pediat. Res. 45 (3), 389–396. doi:10.1203/00006450-199903000-00017
Oshima Y., Tanaka S., Miyamoto T., Wadazumi T., Kurihara N., Fujimoto S. (1998). Effects of endurance training above the anaerobic threshold on isocapnic buffering phase during incremental exercise in middle-distance runners. Jpn. J. Phys. Fit. Sports Med. 47 (1), 43–51. doi:10.7600/jspfsm1949.47.43
Paterson D. H., McLellan T. M., Stella R. S., Cunningham D. A. (1987). Longitudinal study of ventilation threshold and maximal O2 uptake in athletic boys. J. Appl. Physiol. 62 (5), 2051–2057. doi:10.1152/jappl.1987.62.5.2051
Prado D. M. L. D., Braga A. M. F. W., Rondon M. U. P., Azevedo L. F., Matos L. D., Negrão C. E., et al. (2010). Cardiorespiratory responses during progressive maximal exercise test in healthy children. Arq. Bras. Cardiol. 94, 493–499. doi:10.1590/S0066-782X2010005000007
Ratel S., Duche P., Hennegrave A., Van Praagh E., Bedu M. (2002). Acid-base balance during repeated cycling sprints in boys and men. J. Appl. Physiol. 92 (2), 479–485. doi:10.1152/japplphysiol.00495.2001
Reybrouck T., Weymans M., Stijns H., Knops J., Van der Hauwaert L. (1985). Ventilatory anaerobic threshold in healthy children: age and sex differences. Eur. J. Appl. Physiol. Occup. Physiol. 54 (3), 278–284. doi:10.1007/BF00426145
Röcker K., Striegel H., Freund T., Dickhuth H. H. (1994). Relative functional buffering capacity in 400-meter runners, long-distance runners and untrained individuals. Eur. J. Appl. Physiol. 68, 430–434. doi:10.1007/BF00843741
Rowland T. W., Cunningham L. N. (1997). Development of ventilatory responses to exercise in normal white children: a longitudinal study. Chest 111 (2), 327–332. doi:10.1378/chest.111.2.327
Sherar L. B., Mirwald R. L., Baxter-Jones A. D., Thomis M. (2005). Prediction of adult height using maturity-based cumulative height velocity curves. J. Pediatr. 147 (4), 508–514. doi:10.1016/j.jpeds.2005.04.041
Stringier W., Wasserman K., Casaburi R. (1995). The VCO2/V02 relationship during heavy, constant work rate exercise reflects the rate of lactic acid accumulation. Eur. J. Appl. Physiol. Occup. Physiol. 72 (1), 25–31. doi:10.1007/BF00964110
Takano N. (2000). Respiratory compensation point during incremental exercise as related to hypoxic ventilator chemosensitivity and lactate increase in man. Jpn. J. Physiol. 50, 449–455. doi:10.2170/jjphysiol.50.449
Takken T., Sonbahar Ulu H., Hulzebos E. H. (2020). Clinical recommendations for cardiopulmonary exercise testing in children with respiratory diseases. Expert Rev. Respir. Med. 14 (7), 691–701. doi:10.1080/17476348.2020.1752195
Thalheimer W., Cook S. (2002). How to calculate effect sizes from published research: a simplified methodology. Work-Learning Res., 1–9.
Trowbridge C. A., Gower B. A., Nagy T. R., Hunter G. R., Treuth M. S., Goran M. I. (1997). Maximal aerobic capacity in African-American and Caucasian prepubertal children. Am. J. Physiol. Endocrinol. Metab. 273 (4), E809–E814. doi:10.1152/ajpendo.1997.273.4.E809
Wasserman K., Hansen J. E., Sue D. Y., Stringer W. W., Whipp B. J. (2012). Principles of exercise testing and interpretation including pathophysiology and clinical applications. Philadelphia, PA: Lippincott Williams and Wilkins.
Weston M. E., Barker A. R., Tomlinson O. W., Coombes J. S., Bailey T. G., Bond B. (2021). Differences in cerebrovascular regulation and ventilatory responses during ramp incremental cycling in children, adolescents, and adults. J. Appl. Physiol. 131 (4), 1200–1210. doi:10.1152/japplphysiol.00182.2021
Weymans M., Reybrouck T. (1989). Habitual level of physical activity and cardiorespiratory endurance capacity in children. Eur. J. Appl. Physiol. Occup. Physiol. 58, 803–807. doi:10.1007/BF02332210
Whipp B. J., Davis J. A., Wasserman K. (1989). Ventilatory control of the ‘isocapnic buffering’region in rapidly-incremental exercise. Respir. Physiol. 76 (3), 357–367. doi:10.1016/0034-5687(89)90076-5
Keywords: metabolic threshold, respiratory compensation point, oxygen uptake, ventilation, cardiopulmonary exercise testing
Citation: Korkmaz Eryılmaz S, Karakaş S, Boyraz C, Günaştı Ö, Kılcı A, Özdemir Ç, Özgünen K, Koç M, Adaş Ü and Kurdak S (2024) Respiratory responses and isocapnic buffering phase in child and youth soccer players during an incremental exercise test. Front. Physiol. 15:1407759. doi: 10.3389/fphys.2024.1407759
Received: 27 March 2024; Accepted: 10 September 2024;
Published: 23 September 2024.
Edited by:
Muaz Belviranli, Selçuk University, TürkiyeReviewed by:
Danilo Marcelo Leite Do Prado, University Hospital of São Paulo, BrazilRobert Naeije, Université Libre de Bruxelles, Belgium
Copyright © 2024 Korkmaz Eryılmaz, Karakaş, Boyraz, Günaştı, Kılcı, Özdemir, Özgünen, Koç, Adaş and Kurdak. This is an open-access article distributed under the terms of the Creative Commons Attribution License (CC BY). The use, distribution or reproduction in other forums is permitted, provided the original author(s) and the copyright owner(s) are credited and that the original publication in this journal is cited, in accordance with accepted academic practice. No use, distribution or reproduction is permitted which does not comply with these terms.
*Correspondence: Selcen Korkmaz Eryılmaz, c2VsY2VuX2tvcmttYXpAeWFob28uY29t