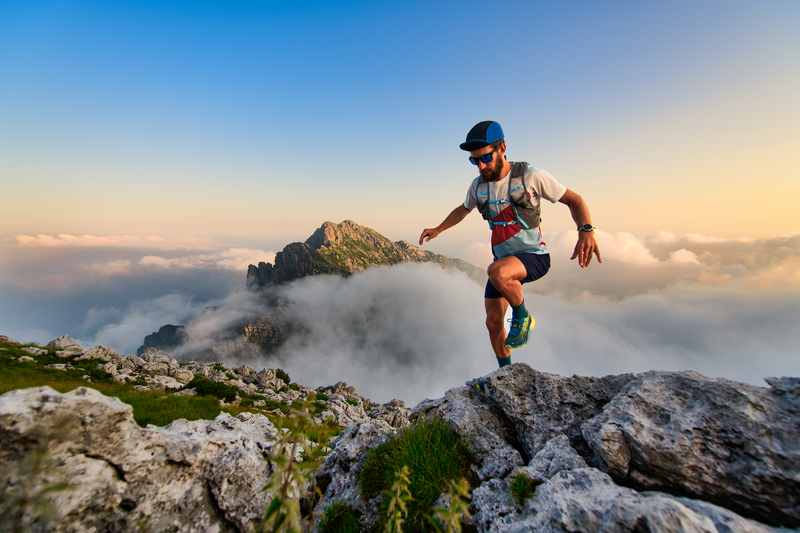
94% of researchers rate our articles as excellent or good
Learn more about the work of our research integrity team to safeguard the quality of each article we publish.
Find out more
REVIEW article
Front. Physiol. , 27 March 2024
Sec. Clinical and Translational Physiology
Volume 15 - 2024 | https://doi.org/10.3389/fphys.2024.1380992
Sepsis is a life-threatening organ dysfunction caused by a dysregulated host response to infection, and its morbidity and mortality rates are increasing annually. It is an independent risk factor for intensive care unit-acquired weakness (ICU-AW), which is a common complication of patients in ICU. This situation is also known as sepsis-associated acquired weakness (SAW), and it can be a complication in more than 60% of patients with sepsis. The outcomes of SAW are often prolonged mechanical ventilation, extended hospital stays, and increased morbidity and mortality of patients in ICUs. The pathogenesis of SAW is unclear, and an effective clinical treatment is not available. Ferroptosis is an iron-dependent type of cell death with unique morphological, biochemical, and genetic features. Unlike other forms of cell death such as autophagy, apoptosis, and necrosis, ferroptosis is primarily driven by lipid peroxidation. Cells undergo ferroptosis during sepsis, which further enhances the inflammatory response. This process leads to increased cell death, as well as multi-organ dysfunction and failure. Recently, there have been sporadic reports suggesting that SAW is associated with ferroptosis, but the exact pathophysiological mechanisms remain unclear. Therefore, we reviewed the possible pathogenesis of ferroptosis that leads to SAW and offer new strategies to prevent and treat SAW.
Sepsis is a life-threatening dysfunction caused by a dysregulated host response to infection. The World Health Organization categorizes it as a global health priority (Cecconi et al., 2018; Evans et al., 2021). Despite many advances in the clinical management of sepsis, treatments are limited to symptomatic treatments, such as fluid resuscitation and organ protective support (Evans et al., 2021). The prevalence of sepsis and septic shock has increased annually since it was initially defined. Among 49 million patients with sepsis in 2017, 11 million succumbed to avoidable deaths that accounted for almost 20% of all-cause deaths and the prevalence was higher in females than in males (World Health Organization, 2020). The mortality rate of sepsis in intensive care unit (ICU) is 42%, which is very high (Crawford et al., 2023).
Intensive care unit-acquired weakness (ICU-AW) is a frequent complication among critically ill patients. It is mainly characterized by generalized symmetrical decrease in proximal limb muscle tone and diminished or normal deep tendon reflexes, which are often cumulative in limb and respiratory muscles (Stevens et al., 2009; Latronico et al., 2017). Globally, 13–20 million patients annually require treatment in ICU and >1 million of them develop ICU-AW (Fan et al., 2014). The main etiologies of ICU-AW are sepsis, mechanical ventilation, long-term immobilization, hyperglycemia, advanced age, and malnutrition (Herridge et al., 2011; Jolley et al., 2016; Ozdemir et al., 2022). Sepsis is an independent risk factor for ICU-AW, affecting over 60% of sepsis patients (Latronico et al., 2017; Mitobe et al., 2019; Bellaver et al., 2023; Takahashi et al., 2023), and this condition is also known as sepsis-associated acquired weakness (SAW). The pathogenesis of SAW remains unclear and an effective clinical treatment does not exist. Ferroptosis is closely associated with sepsis, such as lipid peroxidation, reactive oxygen species (ROS), and cellular ion metabolism (Huang et al., 2023). Ferroptosis is involved in skeletal muscle atrophy and sarcopenia, and sepsis can cause acquired muscle weakness (Ikeda et al., 2016; Huang et al., 2021). Inflammation inhibits the expression of the ferroptosis biomarker cystine transporter solute carrier family 7 member 11 (SLC7A11) and GPX4 synthesis is blocked, thus promoting the occurrence of ferroptosis (Wang Y. et al., 2023; Wang Z. et al., 2023). However, whether ferroptosis is involved in the development of SAW is unclear. Therefore, we reviewed the mechanisms of ferroptosis that might be involved in SAW to generate new strategies that could prevent and treat SAW.
Ferroptosis is a unique form of iron-dependent non-apoptotic cell death that is morphologically, biochemically, and genetically distinct from apoptosis, necrosis, and autophagy (Dixon et al., 2012). The essence is that intracellular ROS and hydrogen peroxide (H2O2) produce lipid peroxides due to the action of iron and oxidized lipid membranes with polyunsaturated fatty acids (PUFAs). This causes membrane damage followed by cell death. The intracellular cystine/glutamic acid reverse transporter (SXc−), glutathione, and glutathione peroxidase 4 (GPX4) interact under physiological conditions to maintain homeostasis in cells and organisms (Dixon et al., 2012; Hassannia et al., 2019; Jiang et al., 2021). Mitochondria are not only involved in energy supply but also produce ROS, H202 in the process. In general, mitochondrial antioxidant enzymes, such as superoxide dismutase (SOD), glutathione peroxidase (GPX), catalase, etc., can scavenge excess ROS and H202 produced during metabolism, maintain mitochondrial homeostasis, and protect cellular activity (Paplou et al., 2021). Furthermore, the mitochondria-targeted antioxidant MitoTEMPO blocked adriamycin-induced cardiac ferroptosis in mice, providing strong in vivo evidence for a link between mitochondria and ferroptosis (Fang et al., 2019). Ferroptosis is involved in the development of various diseases such as sepsis, tumors, neurological disease, and cardiomyopathies (Qiu et al., 2020). When bacterial infections result in sepsis, bacteria rely on iron for growth and thus stimulate intracellular iron shedding and release. Moreover, bacteria enhance the production of ROS and unsaturated fatty acids that serve as additional resources for ferroptosis and as bacterial nutrients. This subsequently exacerbates infection, leading to multi-organ dysfunction and ICU-AW (Latronico et al., 2017; Lei et al., 2022; Ma et al., 2022).
Ferroptosis plays important roles in sepsis-induced lung and renal injury, cardiomyopathy (Li et al., 2020; Wang et al., 2020; Li et al., 2022; Qiongyue et al., 2022; Bayır et al., 2023; Fang et al., 2023) and in the pathological process of sarcopenia and skeletal muscle atrophy (Reardon and Allen, 2009; Huang et al., 2021). Ferroptosis in skeletal muscle cells might be involved in the development of SAW, and the mechanism might be associated with disordered iron metabolism, lipid peroxidation, and oxidative stress that inhibit GPX4 expression and promote ferroptosis in skeletal muscle cells (Li et al., 2023). We previously found that the AMP-activated protein kinase-peroxisome proliferator-activated receptor γ coactivator-1α-nicotinamide adenine dinucleotide (NAD)-dependent deacetylase sirtuin-3 (SIRT3) signaling pathway is involved in SAW development (Wang, 2021) and that SIRT3 upregulation inhibits p53-mediated ferroptosis (Jin et al., 2021). SIRT3 is mainly located on mitochondria (Zhou et al., 2022), which are impaired in muscle in sepsis patients, interfering with muscle function and metabolism (Fredriksson et al., 2008; Friedrich et al., 2015; Leduc-Gaudet et al., 2020). Mitochondrial dysfunction can also be considered a “catalyst” for skeletal muscle atrophy. Mitochondrial dysfunction is not limited to muscle atrophy, but also triggers a cascade of deleterious events, including impaired energy metabolism, increased oxidative stress, and ferroptosis (Owen et al., 2019).
It has also been found that in a mouse model of sepsis, mitochondrial ultrastructural features manifested as smaller mitochondria, increased membrane density, reduced or absent cristae and rupture of the outer membrane, providing additional evidence for iron-induced cell death in skeletal muscle cells of septic mice (Li et al., 2023). In addition, the researchers identified a set of genes associated with both mitochondrial dysfunction and ferroptosis in sepsis-affected skeletal muscle and subjected the muscle tissue to immunoblotting analyses that revealed significantly increased levels of ferroptosis marker proteins (Sheng et al., 2024). The morphological features of ferroptosis are mainly high mitochondrial membrane density, reduced volume and rupture of the outer membrane (Jiang et al., 2021). Cells undergoing ferroptosis are immunogenic and can amplify the inflammatory response, causing more cell death (Martin-Sanchez et al., 2017). We speculated that the common mechanism of ferroptosis leading to sepsis and muscle atrophy are closely associated with the pathogenesis of SAW. Therefore, this article focuses on relevant mechanisms that may contribute to the development of SAW, such as iron and lipid metabolic disorders, SXc−, and p53.
Iron is essential for life as it plays important roles in physiological and redox reactions, as well as DNA synthesis (Liu et al., 2021). Iron concentrations that exceed a threshold maintained by homeostatic mechanisms can trigger increased ROS production, oxidative stress, ferroptosis, activation of pro-inflammatory signaling pathways, and other toxic responses (Ward and Cloonan, 2019; Dutt et al., 2022). Most iron enters the body through dietary iron intake. Dietary iron is usually regarded as heme or non-heme and most of it enters the body in the ferric (Fe3+) form, which ferric reductase reduces to the ferrous (Fe2+) form. After binding to divalent metal-ion transporter 1 (DMT1) in the intestinal lumen, Fe2+ crosses the brush border membrane to reach the apical membranes of enterocytes. Ferroportin 1 (FPN1) transports Fe2+ across the basolateral membrane and into the blood circulation by cytosis and binds to plasma transferrin (TF) to form TF-Fe3+ complexes that enter cells through endocytosis by transferrin receptor 1 (TFR1). Some Fe3+ is stored in ferritin. Excess Fe3+ converted to Fe2+ by ferric reductases (6-transmembrane epithelial antigen of the prostate 3, STEAP3) enters the labile iron pool (LIP) (Anderson and Frazer, 2017) (Figure 1). Intracellular iron is normally in dynamic equilibrium and is used to meet the normal metabolic demands of organisms. However, due to repeated blood sampling or blood losses, anemia of inflammation (AI), along with blood transfusions and intravenous iron supplementation, ICU patients face challenges in maintaining a dynamic iron balance (Grange et al., 2023).
Figure 1. Metabolism of dietary iron derived from food. Ferric reductase converts Fe3+ absorbed through the duodenum to Fe2+, which binds to DMT1 for transport to enterocyte apical membranes. Endocytosis causes Fe2+ and FPN1 to enter blood circulation, and free Fe2+ binds to TF as TF-Fe3+. Thereafter, TFR1 transports Fe3+ through endocytosis into cells where some iron is stored as ferritin and some is converted to Fe2+ by STEAP3 and enters LIP. DMT1, divalent metal-ion transporter 1; Fe, iron; LIP, labile iron pool; STEAP3, 6-transmembrane epithelial antigen of the prostate 3; TF, transferrin; TFR1, transferrin receptor 1.
Under pathological conditions, proteins associated with iron and ferritin metabolism can become dysfunctional and augment biomarkers such as iron and ferritin that are associated with inflammation. Ferritin autophagy and iron deposition during inflammatory processes (Liu et al., 2021; Hamilton et al., 2023) result in excessive intracellular accumulation of Fe3+ and Fe2+. This excessive Fe3+ and Fe2+ accumulation thereby leads to ROS accumulation via the Fenton reaction, which generates lipid peroxides (PL-PUFA-OOH) that lead to ferroptosis (Xing et al., 2022). In addition, excessive iron in skeletal muscle induces skeletal muscle atrophy and oxidative stress (Reardon and Allen, 2009; Ikeda et al., 2016), such as the loss of skeletal muscle mass in aged rats (Huang et al., 2021). Both sepsis and skeletal muscle atrophy can lead to disordered iron metabolism, which is the mechanism of ferroptosis. We thus postulated that disrupted iron metabolism is one explanation for ferroptosis in SAW.
Lipids considerably impact cellular structure and functioning, including their involvement in biofilm composition and signaling processes. Polyunsaturated fatty acids are important components of the lipid bilayer in cell membranes, and ferroptosis is characterized by the generation of PL-PUFA-OOH, which is considered as the main executer of ferroptosis (Jiang et al., 2021). Free PUFAs synthesize lipids via synthases and integrate with phospholipids to form PUFA-PL to maintain cell membrane stability (Welch et al., 2022). Physiologically, cytochrome P450 oxidoreductase (POR) is located in the endoplasmic reticulum (Koppula et al., 2021; Rojas Velazquez et al., 2022). The electrons of nicotinamide dinucleotide phosphate hydrogen (NAD(P)H) are transferred to downstream CYP450 (Yan et al., 2021) via POR; however, some of these electrons react with oxygen during transfer, which results in H2O2 production. The subsequent Fenton reaction between H2O2 and Fe2+ leads to the generation of free hydroxyl radicals (-OH) that oxidatively react with PUFAs to yield PL-PUFA. Under the influence of Fe2+, PL-PUFA transforms into toxic PL-PUFA-OOH (Liang et al., 2022). Interaction between PL-PUFA-OOH and reduced glutathione (GSH) leads to their reduction via GPX4 to yield PUFA phospholipid alcohols (PL-PUFA-OH) and oxidized glutathione (GSSG). This enzymatic process helps preserve cell membrane integrity.
Pathological conditions such as sepsis and myasthenia, disrupt the equilibrium described above. That is, excessive -OH production and GPX4 depletion prevents the continued reduction of PL-PUFA-OOH and leads to oxidative rupture of the cell membrane (Ursini and Maiorino, 2020; Yan et al., 2021). Meanwhile, the expression of light chain SLC7A11 is suppressed by the activation of the nucleotide-binding domain, leucine-rich–containing family, pyrin domain–containing-3 (NLRP3) inflammasomes during sepsis (Liu et al., 2022; Wang C. et al., 2023). Downstream GPX4 levels are decreased, and inhibiting this pathway accelerates the onset of ferroptosis (Wang Y. et al., 2023; Wang Z. et al., 2023). Inflammation also contributes to the production and accumulation of ROS in the skeletal muscle, and this is a major characteristic of skeletal muscle atrophy (Eshima et al., 2023). Accumulated ROS leads to increased PL-PUFA-OOH level and inhibited GPX4 activity, which results in ferroptosis (Hassannia et al., 2019), thus exacerbating the development of sepsis. This process also accelerates GPX4 depletion, which facilitates ferroptosis (Figure 2). Thus, ferroptosis induced by disordered lipid metabolism disorders might be an additional pathogenetic mechanism of SAW.
Figure 2. Pathways of PUFAs involved in ferroptosis. Cytochrome P450 oxidoreductase on endoplasmic reticulum transfers electrons from NAD(P)H to CYP450 while some NAD(P)H transfers electrons to oxygen and produces H2O2. Hydrogen peroxide reacts with Fe2+ in the Fenton reaction to form -OH, which reacts with oxidized PUFAs to form PL-PUFA, which ROS and Fe2+ will convert to PL-PUFA-OOH. Intracellular GPX4 under physiological conditions will reduce PL-PUFA-OOH to PL-PUFA-OH. During sepsis, activated NLRP3 inhibits the expression of SLC7A11, which hinders cystine uptake, leaving insufficient raw material for GPX4 synthesis. Due to reduced levels of GPX4 synthesis, PL-PUFA-OOH cannot be reduced, which leads to ferroptosis. -OH, hydroxyl radical; CYP450, cytochrome P450; GPX4, glutathione, and glutathione peroxidase 4; H2O2, hydrogen peroxide; NAD(P)H, nicotinamide dinucleotide phosphate hydrogen; NLRP3, nucleotide-binding domain, leucine-rich–containing family, pyrin domain–containing-3; PL-PUFA, polyunsaturated-fatty-acid-containing phospholipid; PL-PUFA-OH, polyunsaturated-fatty-acid-containing oxidative phospholipid; PL-PUFA-OOH, polyunsaturated-fatty-acid-containing-phospholipid hydroperoxides; ROS, reactive oxygen species; SLC7A11, cystine transporter solute carrier family 7 member 11.
System Xc− consists of the protein subunits, SLC7A11 and heavy chain carrier family 3 member 2 (SLC3A2) that are amino acid transport proteins for cystine import and glutamate export (Dixon et al., 2014; Tang and Kroemer, 2020). Its role is to uptake cystine and excrete glutamate. Ingested cystine is reduced to cysteine then involved in glutathione synthesis (Liu et al., 2020a). Glutathione has reduced (GSH) and oxidized (GSSG) forms and GPX4 catalyzes the conversion of glutathione to GSSG. Normally, GSH plays antioxidant and protective roles by reacting with ROS (Stockwell et al., 2017). Glutathione peroxidase 4 is critical for cell survival and is a core regulator of ferroptosis, which degrades small molecule peroxides and PL-PUFA-OOH and inhibits lipid peroxidation. The knockdown and upregulation of GPX4 respectively induces and inhibits ferroptosis (Yang et al., 2014). Inhibiting SLC7A11 during sepsis leads to SXc− inactivation, the prevention of cystine translocation into cells (Wang et al., 2022; Zhang et al., 2022), and reduced glutathione synthesis from glutamate and cystine. Depleted glutathione causes a decline in GPX4 synthesis, which subsequently results in a diminished ability to convert H2O2 and hydroperoxides into water and a gradual increase of hydroperoxides. Accumulated hydroperoxides further react with PUFAs on lipid membranes and lead to ferroptosis via increased lipid peroxidation of cell membranes (Yang et al., 2014; Chen et al., 2021) (Figure 3).
Figure 3. Metabolic pathways of ferroptosis associated with sepsis. Glutathione synthesized via cystine uptake of SLC7A11 is an important raw material for synthesis of GPX4, which consumes ROS generated during normal metabolism. Suppressed SLC7A11 activity during sepsis leads to downregulated GPX4 expression and impaired PL-PUFA-OOH reduction that ultimately triggers intracellular ferroptosis. GPX4, glutathione, and glutathione peroxidase 4; PL-PUFA-OOH, polyunsaturated-fatty-acid-containing-phospholipid hydroperoxides; ROS, reactive oxygen species; SLC7A11, cystine transporter solute carrier family 7 member 11.
Sepsis is an independent risk factor for ICU-AW (Latronico et al., 2017; Mitobe et al., 2019; Bellaver et al., 2023; Takahashi et al., 2023), and SAW predominantly manifests as reduced protein synthesis coupled with increased degradation (Cohen et al., 2015). Transcription factors such as forkhead box O3 and nuclear factor kappa B required for muscle atrophy are activated during sepsis, and these transcription factors inhibit SLC7A11 expression (Zhong et al., 2023; Wu et al., 2024) which causes the inhibition of the SXc−-GSH-GPX4 signaling pathway (Wang et al., 2022; Zhang et al., 2022). Hence, ferroptosis facilitated by the SXc−-GSH-GPX4 pathway might function in the development of SAW.
The p53 tumor suppressor gene (Wang H. et al., 2023) is the most frequently mutated among the genes involved in human cancers (Levine, 2020). The proteins encoded by p53 control cell cycle arrest, apoptosis, and DNA repair (Hassin and Oren, 2023). Furthermore, p53 exerts its antiproliferative effects through an independent mechanism (Speidel, 2010), affects cytoplasm and almost all organelles, such as mitochondria, lysosomes, and endoplasmic reticulum (Hassin and Oren, 2023), and inhibits SLC7A11. These processes result in the inhibition of the SXc−-GSH-GPX4 pathway, leading to cellular ferroptosis (Jiang et al., 2015; Wang H. et al., 2023) (Figure 4).
Figure 4. Involvement of p53 activated during sepsis on ferroptosis. Inhibition of SLC7A11 expression by p53 reduces cystine uptake, leading to decreased GPX4 synthesis. Transcriptional target of p53, SAT1, is activated in sepsis, and SAT1 induces elevation of ALOX15, which oxidizes PUFAs that promote ferroptosis. Reduced GPX4 activity inevitably leads to ferroptosis. ALOX15, arachidonate 15-lipoxygenase; GPX4, glutathione, and glutathione peroxidase 4; p53, tumor protein 53; PUFAs, polyunsaturated fatty acids; SAT1, spermidine/spermine N1-acetyltransferase 1.
Patients with SAW in ICU are immobilized mostly due to factors such as disease states, prevention of catheter dislodgement, and falls from beds. The expression of p53 is increased in immobilization-induced skeletal muscle atrophy (Fox et al., 2014). Spermidine/spermine N1-acetyltransferase 1 (SAT1) acts as a transcriptional target of p53 that is activated by inflammation; it also induces the elevated expression of arachidonate 15-lipoxygenase (ALOX15), which oxidizes PUFAs that promote ferroptosis (Ou et al., 2016; Liu et al., 2020b). Therefore, p53 and SAT1 expression might be increased in patients with SAW, which in turn induces ferroptosis. However, the exact mechanism requires further in-depth investigation.
Sepsis-associated acquired weakness is a frequent complication in critically ill patients with sepsis that cannot be effectively treated or prevented. This type of weakness affects the quality of life of the patients and can lead to complete paralysis. Ferroptosis is a new pathway of programmed cell death in sepsis and skeletal muscle atrophy. Although there are sporadic reports suggesting that SAW is associated with ferroptosis, the mechanism remains unclear. Therefore, through this review, we described the common pathways through which ferroptosis leads to sepsis and muscle atrophy. These mechanisms potentially underpin the causative relationship between ferroptosis and SAW. However, this knowledge gap presents avenues for future investigation and serves as an impetus to guide investigators toward analyzing this situation.
JY: Writing–original draft, Conceptualization, Investigation. CY: Writing–original draft. SC: Writing–original draft. ML: Writing–original draft. YM: Writing–original draft. XM: Writing–original draft. JZ: Writing–original draft. PX: Data curation, Resources, Supervision, Writing–original draft, Writing–review and editing.
The author(s) declare financial support was received for the research, authorship, and/or publication of this article. This work was supported by the National Natural Science Foundation (Grant Nos 82060359 and 82360382) of China; Guizhou Province Social Development Project: Qiankehe [2021] General 088; Key Project of Guizhou Natural Science Foundation: Qiankehe Fundamentals ZK [2022] Key 049; Guizhou Province Excellent Youth Science and Technology Talent Project: Qiankehe Platform Talent [2021] No. 5648.
The authors would like to thank Xiaoming Zhang for her help in reviewing and proofreading this paper. The authors acknowledge the use of Figdraw (www.figdraw.com) to create all fgures.
The authors declare that the research was conducted in the absence of any commercial or financial relationships that could be construed as a potential conflict of interest.
All claims expressed in this article are solely those of the authors and do not necessarily represent those of their affiliated organizations, or those of the publisher, the editors and the reviewers. Any product that may be evaluated in this article, or claim that may be made by its manufacturer, is not guaranteed or endorsed by the publisher.
Anderson G. J., Frazer D. M. (2017). Current understanding of iron homeostasis. Am. J. Clin. Nutr. 106 (6), 1559S–1566s. doi:10.3945/ajcn.117.155804
Bayır H., Dixon S. J., Tyurina Y. Y., Kellum J. A., Kagan V. E. (2023). Ferroptotic mechanisms and therapeutic targeting of iron metabolism and lipid peroxidation in the kidney. Nat. Rev. Nephrol. 19 (5), 315–336. doi:10.1038/s41581-023-00689-x
Bellaver P., Schaeffer A. F., Leitao C. B., Rech T. H., Nedel W. L. (2023). Association between neuromuscular blocking agents and the development of intensive care unit-acquired weakness (ICU-AW): a systematic review with meta-analysis and trial sequential analysis. Anaesth. Crit. Care Pain Med. 42 (3), 101202. doi:10.1016/j.accpm.2023.101202
Cecconi M., Evans L., Levy M., Rhodes A. (2018). Sepsis and septic shock. Lancet 392 (10141), 75–87. doi:10.1016/S0140-6736(18)30696-2
Chen X., Kang R., Kroemer G., Tang D. (2021). Ferroptosis in infection, inflammation, and immunity. J. Exp. Med. 218 (6), e20210518. doi:10.1084/jem.20210518
Cohen S., Nathan J. A., Goldberg A. L. (2015). Muscle wasting in disease: molecular mechanisms and promising therapies. Nat. Rev. Drug Discov. 14 (1), 58–74. doi:10.1038/nrd4467
Crawford A. M., Shiferaw A. A., Ntambwe P., Milan A. O., Khalid K., Rubio R., et al. (2023). Global critical care: a call to action. Crit. Care 27 (1), 28. doi:10.1186/s13054-022-04296-3
Dixon S. J., Lemberg K. M., Lamprecht M. R., Skouta R., Zaitsev E. M., Gleason C. E., et al. (2012). Ferroptosis: an iron-dependent form of nonapoptotic cell death. Cell 149 (5), 1060–1072. doi:10.1016/j.cell.2012.03.042
Dixon S. J., Patel D. N., Welsch M., Skouta R., Lee E. D., Hayano M., et al. (2014). Pharmacological inhibition of cystine-glutamate exchange induces endoplasmic reticulum stress and ferroptosis. Elife 3, e02523. doi:10.7554/eLife.02523
Dutt S., Hamza I., Bartnikas T. B. (2022). Molecular mechanisms of iron and heme metabolism. Annu. Rev. Nutr. 42, 311–335. doi:10.1146/annurev-nutr-062320-112625
Eshima H., Shahtout J. L., Siripoksup P., Pearson M. J., Mahmassani Z. S., Ferrara P. J., et al. (2023). Lipid hydroperoxides promote sarcopenia through carbonyl stress. Elife 12, e85289. doi:10.7554/eLife.85289
Evans L., Rhodes A., Alhazzani W., Antonelli M., Coopersmith C. M., French C., et al. (2021). Executive summary: surviving sepsis campaign: international guidelines for the management of sepsis and septic shock 2021. Crit. Care Med. 49 (11), 1974–1982. doi:10.1097/CCM.0000000000005357
Fan E., Cheek F., Chlan L., Gosselink R., Hart N., Herridge M. S., et al. (2014). An official American Thoracic Society Clinical Practice guideline: the diagnosis of intensive care unit-acquired weakness in adults. Am. J. Respir. Crit. Care Med. 190 (12), 1437–1446. doi:10.1164/rccm.201411-2011ST
Fang X., Ardehali H., Min J., Wang F. (2023). The molecular and metabolic landscape of iron and ferroptosis in cardiovascular disease. Nat. Rev. Cardiol. 20 (1), 7–23. doi:10.1038/s41569-022-00735-4
Fang X., Wang H., Han D., Xie E., Yang X., Wei J., et al. (2019). Ferroptosis as a target for protection against cardiomyopathy. Proc. Natl. Acad. Sci. U. S. A. 116 (7), 2672–2680. doi:10.1073/pnas.1821022116
Fox D. K., Ebert S. M., Bongers K. S., Dyle M. C., Bullard S. A., Dierdorff J. M., et al. (2014). p53 and ATF4 mediate distinct and additive pathways to skeletal muscle atrophy during limb immobilization. Am. J. Physiol. Endocrinol. Metab. 307 (3), E245–E261. doi:10.1152/ajpendo.00010.2014
Fredriksson K., Tjader I., Keller P., Petrovic N., Ahlman B., Scheele C., et al. (2008). Dysregulation of mitochondrial dynamics and the muscle transcriptome in ICU patients suffering from sepsis induced multiple organ failure. PLoS One 3 (11), e3686. doi:10.1371/journal.pone.0003686
Friedrich O., Reid M. B., Van den Berghe G., Vanhorebeek I., Hermans G., Rich M. M., et al. (2015). The sick and the weak: neuropathies/myopathies in the critically ill. Physiol. Rev. 95 (3), 1025–1109. doi:10.1152/physrev.00028.2014
Grange C., Lux F., Brichart T., David L., Couturier A., Leaf D. E., et al. (2023). Iron as an emerging therapeutic target in critically ill patients. Crit. Care 27 (1), 475. doi:10.1186/s13054-023-04759-1
Hamilton F., Mitchell R., Ahmed H., Ghazal P., Timpson N. J. (2023). An observational and Mendelian randomisation study on iron status and sepsis. Sci. Rep. 13 (1), 2867. doi:10.1038/s41598-023-29641-6
Hassannia B., Vandenabeele P., Vanden Berghe T. (2019). Targeting ferroptosis to iron out cancer. Cancer Cell 35 (6), 830–849. doi:10.1016/j.ccell.2019.04.002
Hassin O., Oren M. (2023). Drugging p53 in cancer: one protein, many targets. Nat. Rev. Drug Discov. 22 (2), 127–144. doi:10.1038/s41573-022-00571-8
Herridge M. S., Tansey C. M., Matté A., Tomlinson G., Diaz-Granados N., Cooper A., et al. (2011). Functional disability 5 years after acute respiratory distress syndrome. N. Engl. J. Med. 364 (14), 1293–1304. doi:10.1056/NEJMoa1011802
Huang Q., Ding Y., Fang C., Wang H., Kong L. (2023). The emerging role of ferroptosis in sepsis, opportunity or challenge? Infect. Drug Resist 16, 5551–5562. doi:10.2147/idr.S419993
Huang Y., Wu B., Shen D., Chen J., Yu Z., Chen C. (2021). Ferroptosis in a sarcopenia model of senescence accelerated mouse prone 8 (SAMP8). Int. J. Biol. Sci. 17 (1), 151–162. doi:10.7150/ijbs.53126
Ikeda Y., Imao M., Satoh A., Watanabe H., Hamano H., Horinouchi Y., et al. (2016). Iron-induced skeletal muscle atrophy involves an Akt-forkhead box O3-E3 ubiquitin ligase-dependent pathway. J. Trace Elem. Med. Biol. 35, 66–76. doi:10.1016/j.jtemb.2016.01.011
Jiang L., Kon N., Li T., Wang S. J., Su T., Hibshoosh H., et al. (2015). Ferroptosis as a p53-mediated activity during tumour suppression. Nature 520 (7545), 57–62. doi:10.1038/nature14344
Jiang X., Stockwell B. R., Conrad M. (2021). Ferroptosis: mechanisms, biology and role in disease. Nat. Rev. Mol. Cell Biol. 22 (4), 266–282. doi:10.1038/s41580-020-00324-8
Jin Y., Gu W., Chen W. (2021). Sirt3 is critical for p53-mediated ferroptosis upon ROS-induced stress. J. Mol. Cell Biol. 13 (2), 151–154. doi:10.1093/jmcb/mjaa074
Jolley S. E., Bunnell A. E., Hough C. L. (2016). ICU-acquired weakness. Chest 150 (5), 1129–1140. doi:10.1016/j.chest.2016.03.045
Koppula P., Zhuang L., Gan B. (2021). Cystine transporter SLC7A11/xCT in cancer: ferroptosis, nutrient dependency, and cancer therapy. Protein Cell 12 (9), 599–620. doi:10.1007/s13238-020-00789-5
Latronico N., Herridge M., Hopkins R. O., Angus D., Hart N., Hermans G., et al. (2017). The ICM research agenda on intensive care unit-acquired weakness. Intensive Care Med. 43 (9), 1270–1281. doi:10.1007/s00134-017-4757-5
Leduc-Gaudet J. P., Mayaki D., Reynaud O., Broering F. E., Chaffer T. J., Hussain S. N. A., et al. (2020). Parkin overexpression attenuates sepsis-induced muscle wasting. Cells 9 (6), 1454. doi:10.3390/cells9061454
Lei X. L., Zhao G. Y., Guo R., Cui N. (2022). Ferroptosis in sepsis: the mechanism, the role and the therapeutic potential. Front. Immunol. 13, 956361. doi:10.3389/fimmu.2022.956361
Levine A. J. (2020). p53: 800 million years of evolution and 40 years of discovery. Nat. Rev. Cancer 20 (8), 471–480. doi:10.1038/s41568-020-0262-1
Li F., Wang S., Zhou Y., Gong Y. (2023). Signal transducer and activator of transcription 6 mediates skeletal muscle cell injury in septic mice by regulating ferroptosis. Zhonghua Wei Zhong Bing Ji Jiu Yi Xue 35 (8), 813–817. doi:10.3760/cma.j.cn121430-20230312-00170
Li J., Li M., Li L., Ma J., Yao C., Yao S. (2022). Hydrogen sulfide attenuates ferroptosis and stimulates autophagy by blocking mTOR signaling in sepsis-induced acute lung injury. Mol. Immunol. 141, 318–327. doi:10.1016/j.molimm.2021.12.003
Li N., Wang W., Zhou H., Wu Q., Duan M., Liu C., et al. (2020). Ferritinophagy-mediated ferroptosis is involved in sepsis-induced cardiac injury. Free Radic. Biol. Med. 160, 303–318. doi:10.1016/j.freeradbiomed.2020.08.009
Liang D., Minikes A. M., Jiang X. (2022). Ferroptosis at the intersection of lipid metabolism and cellular signaling. Mol. Cell 82 (12), 2215–2227. doi:10.1016/j.molcel.2022.03.022
Liu J., Xia X., Huang P. (2020a). xCT: a critical molecule that links cancer metabolism to redox signaling. Mol. Ther. 28 (11), 2358–2366. doi:10.1016/j.ymthe.2020.08.021
Liu J., Zhang C., Wang J., Hu W., Feng Z. (2020b). The regulation of ferroptosis by tumor suppressor p53 and its pathway. Int. J. Mol. Sci. 21 (21), 8387. doi:10.3390/ijms21218387
Liu Q., Wu J., Zhang X., Wu X., Zhao Y., Ren J. (2021). Iron homeostasis and disorders revisited in the sepsis. Free Radic. Biol. Med. 165, 1–13. doi:10.1016/j.freeradbiomed.2021.01.025
Liu Y., Wang D., Li T., Yang F., Li Z., Bai X., et al. (2022). The role of NLRP3 inflammasome in inflammation-related skeletal muscle atrophy. Front. Immunol. 13, 1035709. doi:10.3389/fimmu.2022.1035709
Ma R., Fang L., Chen L., Wang X., Jiang J., Gao L. (2022). Ferroptotic stress promotes macrophages against intracellular bacteria. Theranostics 12 (5), 2266–2289. doi:10.7150/thno.66663
Martin-Sanchez D., Ruiz-Andres O., Poveda J., Carrasco S., Cannata-Ortiz P., Sanchez-Nino M. D., et al. (2017). Ferroptosis, but not necroptosis, is important in nephrotoxic folic acid-induced AKI. J. Am. Soc. Nephrol. 28 (1), 218–229. doi:10.1681/ASN.2015121376
Mitobe Y., Morishita S., Ohashi K., Sakai S., Uchiyama M., Abeywickrama H., et al. (2019). Skeletal muscle index at intensive care unit admission is a predictor of intensive care unit-acquired weakness in patients with sepsis. J. Clin. Med. Res. 11 (12), 834–841. doi:10.14740/jocmr4027
Ou Y., Wang S. J., Li D., Chu B., Gu W. (2016). Activation of SAT1 engages polyamine metabolism with p53-mediated ferroptotic responses. Proc. Natl. Acad. Sci. U. S. A. 113 (44), E6806–e6812. doi:10.1073/pnas.1607152113
Owen A. M., Patel S. P., Smith J. D., Balasuriya B. K., Mori S. F., Hawk G. S., et al. (2019). Chronic muscle weakness and mitochondrial dysfunction in the absence of sustained atrophy in a preclinical sepsis model. Elife 8, e49920. doi:10.7554/eLife.49920
Ozdemir M., Bomkamp M. P., Hyatt H. W., Smuder A. J., Powers S. K. (2022). Intensive care unit acquired weakness is associated with rapid changes to skeletal muscle proteostasis. Cells 11 (24), 4005. doi:10.3390/cells11244005
Paplou V., Schubert N. M. A., Pyott S. J. (2021). Age-Related changes in the cochlea and vestibule: shared patterns and processes. Front. Neurosci. 15, 680856. doi:10.3389/fnins.2021.680856
Qiongyue Z., Xin Y., Meng P., Sulin M., Yanlin W., Xinyi L., et al. (2022). Post-treatment with irisin attenuates acute kidney injury in sepsis mice through anti-ferroptosis via the SIRT1/nrf2 pathway. Front. Pharmacol. 13, 857067. doi:10.3389/fphar.2022.857067
Qiu Y., Cao Y., Cao W., Jia Y., Lu N. (2020). The application of ferroptosis in diseases. Pharmacol. Res. 159, 104919. doi:10.1016/j.phrs.2020.104919
Reardon T. F., Allen D. G. (2009). Iron injections in mice increase skeletal muscle iron content, induce oxidative stress and reduce exercise performance. Exp. Physiol. 94 (6), 720–730. doi:10.1113/expphysiol.2008.046045
Rojas Velazquez M. N., Noebauer M., Pandey A. V. (2022). Loss of protein stability and function caused by P228L variation in NADPH-cytochrome P450 reductase linked to lower testosterone levels. Int. J. Mol. Sci. 23 (17), 10141. doi:10.3390/ijms231710141
Sheng Z., Yu Z., Wang M., Zhou R., Chen S., Yu X., et al. (2024). Targeting STAT6 to mitigate sepsis-induced muscle atrophy and weakness: modulation of mitochondrial dysfunction, ferroptosis, and CHI3L1-Mediated satellite cell loss. Biochem. Biophys. Rep. 37, 101608. doi:10.1016/j.bbrep.2023.101608
Speidel D. (2010). Transcription-independent p53 apoptosis: an alternative route to death. Trends Cell Biol. 20 (1), 14–24. doi:10.1016/j.tcb.2009.10.002
Stevens R. D., Marshall S. A., Cornblath D. R., Hoke A., Needham D. M., de Jonghe B., et al. (2009). A framework for diagnosing and classifying intensive care unit-acquired weakness. Crit. Care Med. 37 (10), S299–S308. doi:10.1097/CCM.0b013e3181b6ef67
Stockwell B. R., Friedmann Angeli J. P., Bayir H., Bush A. I., Conrad M., Dixon S. J., et al. (2017). Ferroptosis: a regulated cell death nexus linking metabolism, redox biology, and disease. Cell 171 (2), 273–285. doi:10.1016/j.cell.2017.09.021
Takahashi Y., Morisawa T., Okamoto H., Nakanishi N., Matsumoto N., Saitoh M., et al. (2023). Diaphragm dysfunction and ICU-acquired weakness in septic shock patients with or without mechanical ventilation: a pilot prospective observational study. J. Clin. Med. 12 (16), 5191. doi:10.3390/jcm12165191
Tang D., Kroemer G. (2020). Ferroptosis. Curr. Biol. 30 (21), R1292–r1297. doi:10.1016/j.cub.2020.09.068
Ursini F., Maiorino M. (2020). Lipid peroxidation and ferroptosis: the role of GSH and GPx4. Free Radic. Biol. Med. 152, 175–185. doi:10.1016/j.freeradbiomed.2020.02.027
Wang C., Liu Y., Zhang Y., Wang D., Xu L., Li Z., et al. (2023a). Targeting NAT10 protects against sepsis-induced skeletal muscle atrophy by inhibiting ROS/NLRP3. Life Sci. 330, 121948. doi:10.1016/j.lfs.2023.121948
Wang C., Yuan W., Hu A., Lin J., Xia Z., Yang C. F., et al. (2020). Dexmedetomidine alleviated sepsis-induced myocardial ferroptosis and septic heart injury. Mol. Med. Rep. 22 (1), 175–184. doi:10.3892/mmr.2020.11114
Wang H., Guo M., Wei H., Chen Y. (2023b). Targeting p53 pathways: mechanisms, structures, and advances in therapy. Signal Transduct. Target Ther. 8 (1), 92. doi:10.1038/s41392-023-01347-1
Wang J., Zhu Q., Li R., Zhang J., Ye X., Li X. (2022). YAP1 protects against septic liver injury via ferroptosis resistance. Cell Biosci. 12 (1), 163. doi:10.1186/s13578-022-00902-7
Wang W. (2021). Study on the mechanism of AMPK-PGC1α-SIRT3 signaling pathway involved in sepsis-related acquired myasthenia. master.
Wang Y., Duan H., Zhang J., Wang Q., Peng T., Ye X., et al. (2023c). YAP1 protects against PM2.5-induced lung toxicity by suppressing pyroptosis and ferroptosis. Ecotoxicol. Environ. Saf. 253, 114708. doi:10.1016/j.ecoenv.2023.114708
Wang Z., Li Y., Ye Y., Zhu H., Zhang J., Wang H., et al. (2023d). NLRP3 inflammasome deficiency attenuates cerebral ischemia-reperfusion injury by inhibiting ferroptosis. Brain Res. Bull. 193, 37–46. doi:10.1016/j.brainresbull.2022.11.016
Ward D. M., Cloonan S. M. (2019). Mitochondrial iron in human health and disease. Annu. Rev. Physiol. 81, 453–482. doi:10.1146/annurev-physiol-020518-114742
Welch B. M., McNell E. E., Edin M. L., Ferguson K. K. (2022). Inflammation and oxidative stress as mediators of the impacts of environmental exposures on human pregnancy: evidence from oxylipins. Pharmacol. Ther. 239, 108181. doi:10.1016/j.pharmthera.2022.108181
World Health Organization (2020). Global report on the epidemiology and burden of sepsis: current evidence, identifying gaps and future directions. Geneva: World Health Organization.
Wu S., Zhou Y., Liang J., Ying P., Situ Q., Tan X., et al. (2024). Upregulation of NF-κB by USP24 aggravates ferroptosis in diabetic cardiomyopathy. Free Radic. Biol. Med. 210, 352–366. doi:10.1016/j.freeradbiomed.2023.11.032
Xing G., Meng L., Cao S., Liu S., Wu J., Li Q., et al. (2022). PPARα alleviates iron overload-induced ferroptosis in mouse liver. EMBO Rep. 23 (8), e52280. doi:10.15252/embr.202052280
Yan B., Ai Y., Sun Q., Ma Y., Cao Y., Wang J., et al. (2021). Membrane damage during ferroptosis is caused by oxidation of phospholipids catalyzed by the oxidoreductases POR and CYB5R1. Mol. Cell 81 (2), 355–369.e10. doi:10.1016/j.molcel.2020.11.024
Yang W. S., SriRamaratnam R., Welsch M. E., Shimada K., Skouta R., Viswanathan V. S., et al. (2014). Regulation of ferroptotic cancer cell death by GPX4. Cell 156 (1-2), 317–331. doi:10.1016/j.cell.2013.12.010
Zhang J., Zheng Y., Wang Y., Wang J., Sang A., Song X., et al. (2022). YAP1 alleviates sepsis-induced acute lung injury via inhibiting ferritinophagy-mediated ferroptosis. Front. Immunol. 13, 884362. doi:10.3389/fimmu.2022.884362
Zhong S., Chen W., Wang B., Gao C., Liu X., Song Y., et al. (2023). Energy stress modulation of AMPK/FoxO3 signaling inhibits mitochondria-associated ferroptosis. Redox Biol. 63, 102760. doi:10.1016/j.redox.2023.102760
Keywords: sepsis-associated acquired weakness, ferroptosis, iron, lipid metabolic disorders, Sxc−, p53
Citation: Yang J, Yan C, Chen S, Li M, Miao Y, Ma X, Zeng J and Xie P (2024) The possible mechanisms of ferroptosis in sepsis-associated acquired weakness. Front. Physiol. 15:1380992. doi: 10.3389/fphys.2024.1380992
Received: 02 February 2024; Accepted: 18 March 2024;
Published: 27 March 2024.
Edited by:
Christina Maria Pabelick, Mayo Clinic, United StatesReviewed by:
Y. S. Prakash, Mayo Clinic, United StatesCopyright © 2024 Yang, Yan, Chen, Li, Miao, Ma, Zeng and Xie. This is an open-access article distributed under the terms of the Creative Commons Attribution License (CC BY). The use, distribution or reproduction in other forums is permitted, provided the original author(s) and the copyright owner(s) are credited and that the original publication in this journal is cited, in accordance with accepted academic practice. No use, distribution or reproduction is permitted which does not comply with these terms.
*Correspondence: Peng Xie, cHhpZTEyMzQ1QDEyNi5jb20=
†These authors have contributed equally to this work and share first authorship
Disclaimer: All claims expressed in this article are solely those of the authors and do not necessarily represent those of their affiliated organizations, or those of the publisher, the editors and the reviewers. Any product that may be evaluated in this article or claim that may be made by its manufacturer is not guaranteed or endorsed by the publisher.
Research integrity at Frontiers
Learn more about the work of our research integrity team to safeguard the quality of each article we publish.