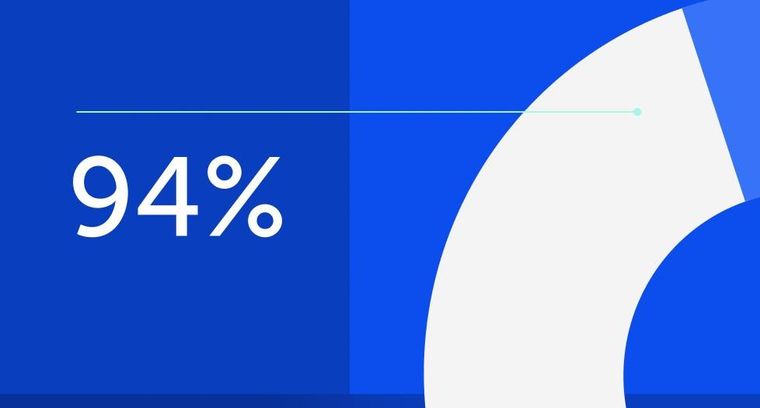
94% of researchers rate our articles as excellent or good
Learn more about the work of our research integrity team to safeguard the quality of each article we publish.
Find out more
ORIGINAL RESEARCH article
Front. Physiol., 09 April 2024
Sec. Biophysics
Volume 15 - 2024 | https://doi.org/10.3389/fphys.2024.1354091
This article is part of the Research TopicQuantum-based Effects on Cell PhysiologyView all 7 articles
The effects of lithium (Li) isotopes and their impact on biological processes have recently gained increased attention due to the significance of Li as a pharmacological agent and the potential that Li isotopic effects in neuroscience contexts may constitute a new example of quantum effects in biology. Previous studies have shown that the two Li isotopes, which differ in mass and nuclear spin, have unusual different effects in vivo and in vitro and, although some molecular targets for Li isotope fractionation have been proposed, it is not known whether those result in observable downstream neurophysiological effects. In this work we studied fluxes of Li+, sodium (Na+) and calcium (Ca2+) ions in the mitochondrial sodium/calcium/lithium exchanger (NCLX), the only transporter known with recognized specificity for Li+. We studied the effect of Li+ isotopes on Ca2+ efflux from heart mitochondria in comparison to natural Li+ and Na+ using Ca2+-induced fluorescence and investigated a possible Li isotope fractionation in mitochondria using inductively coupled plasma mass spectrometry (ICP-MS). Our fluorescence data indicate that Ca2+ efflux increases with higher concentrations of either Li+ or Na+. We found that the simultaneous presence of Li+ and Na+ increases Ca2+ efflux compared to Ca2+ efflux caused by the same concentration of Li+ alone. However, no differentiation in the Ca2+ efflux between the two Li+ isotopes was observed, either for Li+ alone or in mixtures of Li+ and Na+. Our ICP-MS data demonstrate that there is selectivity between Na+ and Li+ (greater Na+ than Li+ uptake) and, most interestingly, between the Li+ isotopes (greater 6Li+ than 7Li+ uptake) by the inner mitochondrial membrane. In summary, we observed no Li+ isotope differentiation for Ca2+ efflux in mitochondria via NCLX but found a Li+ isotope fractionation during Li+ uptake by mitochondria with NCLX active or blocked. Our results suggest that the transport of Li+ via NCLX is not the main pathway for Li+ isotope fractionation and that this differentiation does not affect Ca2+ efflux in mitochondria. Therefore, explaining the puzzling effects of Li+ isotopes observed in other contexts will require further investigation to identify the molecular targets for Li+ isotope differentiation.
Lithium (Li), administered in the form of simple lithium carbonate or citrate salts, has been a forefront medication in the treatment of bipolar disorder for decades (Shorter, 2009). Despite its long-standing clinical use, the precise mechanism of action of Li, acting as a simple Li ion (Li+), in treating this disorder remains poorly understood (Kerr, Bjedov, and Sofola-Adesakin, 2018). From an entirely different perspective, Li, due to reported unusual isotope effects, has recently attracted a renewed attention from researchers (Fisher, 2015; Weingarten, Doraiswamy, and Fisher, 2016; Ettenberg et al., 2020; Deline et al., 2023; Livingstone et al., 2023). Li has two stable isotopes, 6Li and 7Li, with different atomic masses and nuclear spins. Specifically, 6Li has an atomic mass of 6.0151223 atomic mass units (amu) and a nuclear spin of 1, while 7Li has an atomic mass of 7.016004 amu and a nuclear spin of 3/2. Previous studies showed that Li+ isotopes have different effects on animal behaviour and activity (Lieberman, Alexander, and Stokes, 1979; Sechzer et al., 1986; Ettenberg et al., 2020), on electrical response in neuronal tissues (Esmaeilpour et al., 2023), on uptake by the cortex (Sherman, Munsell, and Wong, 1984), on animal lethality (Alexander et al., 1982), on mitochondria calcium (Ca2+) buffering capacity and on the properties of amorphous calcium phosphate aggregates (ACP) in vitro (Deline et al., 2023; Fisher, 2023). A recent study reported that the sodium (Na+)/proton (H+) exchangers in fibroblast cells fractionate Li+ isotopes (Poet et al., 2023). On the other hand, other studies have found no difference in biochemical or cellular processes (Parthasarathy and Eisenberg Jr, 1984; Livingstone et al., 2023).
While certain studies (Lieberman, Alexander, and Stokes, 1979; Alexander et al., 1982; Sherman, Munsell, and Wong, 1984; Ettenberg et al., 2020; Deline et al., 2023) highlight some difference in Li+ isotope effects, a subset of the research results on lithium isotope effects in biological systems stands out in having revealed significant and contrasting impacts by each Li+ isotope. For example, in the study by Sechzer et al. (1986) the two Li+ isotopes were reported to provoke opposite maternal behaviour while, in a very recent study, Esmaeilpour et al. (2023) found a large and opposite effect on the electrical response in neuronal rat hippocampal tissues. The overarching question that these studies raise is: what are the possible molecular targets that may be responsible for such differentiation in Li+ isotope effects that may lead to such substantial and opposing outcomes? It is in this vein that we investigated whether the two Li+ isotopes may affect differently the Li+-induced Ca2+ efflux in heart mitochondria.
The specific mechanisms and the level at which Li+ isotopes act to manifest their different effects are unclear. The possibility of different Li+ isotope effects in neuroscience contexts is both puzzling and intriguing and has led to the formulation of new theoretical hypotheses invoking various quantum properties of the Li isotopes, such as its nuclear spin, that may determine their relative specific activity (Fisher, 2015; Weingarten, Doraiswamy, and Fisher, 2016; Zadeh-Haghighi and Simon, 2021). Recent work (Poet et al., 2023) reported Li+ isotope fractionation via the Na+/H+ exchanger in fibroblast cells, rationalizing the phenomenon in terms of a kinetic isotope effect involving the mass difference between the two isotopes. From a broader perspective, were neurophysiological Li+ isotope effects to be vindicated, it would constitute a new example of quantum effects arising in biology—i.e., within the field of quantum biology (Abbott, Davies, and Pati, 2008; Mohseni et al., 2014; Marais et al., 2018).
Although Li has long had clinical applications and is present in various natural sources, the full extent of its molecular targets in organisms has not been fully identified. While many Li+ targets have been recognized and investigated, sometimes with consideration of Li+ isotope-dependent processes (Parthasarathy and Eisenberg Jr, 1984; Parthasarathy et al., 1992; Livingstone et al., 2023), a complete knowledge and understanding of which ones are crucial for their neurological potency is still lacking. One of the primary pathways for Li+ entry into cells is through sodium (Na+) channels, where Li+ can compete with Na+ for intra-channel binding sites (Richelson, 1977; Thomsen and Shirley, 2006), thus indicating that Li+ and Na+ share affinities for these specific locations. It is worth noting that Li+ can also pass through potassium (K+) channels and interfere with K+ transport (Thompson et al., 2009). Furthermore, Li+ can enter Ca2+ channels and inhibit Ca2+ flux (Kuo and Hess, 1993). In addition to ion channels, Li+ can also be transported by and regulate the activity of the mitochondrial sodium/calcium/lithium exchanger (NCLX), which was identified as not only a Na+ to Ca2+ exchanger, but also as a Li+ to Ca2+ exchanger (Boyman et al., 2013). Mitochondrial Ca2+ transport regulates cell bioenergetics, Ca2+ signaling, and cell death. The influx and accumulation of Ca2+ in mitochondria is facilitated by the mitochondrial Ca2+ uniporter (MCU), while Ca2+ is extruded through the mitochondrial NCLX and H+/Ca2+ exchangers (Figure 1A). It was shown that liver has significantly higher NCLX expression levels than brain or heart mitochondria (Rysted et al., 2021). However, these last authors suggested that NCLX is responsible for Ca2+ extrusion from the mitochondria in the brain and heart, while playing only a minimal role, if any, in liver mitochondria. The NCLX facilitates the exchange of Na+ or Li+ for Ca2+ across the mitochondrial inner membrane, initiating Ca2+ efflux. Ca2+ is a crucial signaling ion in the brain, playing a pivotal role in various neuronal processes. It serves as a second messenger in intracellular signaling cascades and participates in neurotransmitter release, synaptic plasticity, and neuronal excitability. The precise regulation of Ca2+ levels is essential for proper neuronal function and communication. As Li+ is known to compete with other ions and influence calcium signaling (Dubovsky and Franks, 1983; Glen, 1985; Helmeste and Tang, 1998; Bosche et al., 2013; Harrison et al., 2021), we wanted to test whether Li+ isotopes differently affect Na+/Ca2+/Li+ exchange in NCLX.
Figure 1. (A) Schematics of mitochondrion with MCU and NCLX channels. MCU passes Ca2+ inside the mitochondrion (Ca2+ influx); NCLX passes Na+ or Li+ inside the mitochondrion in exchange for Ca2+ transport outside (Ca2+ efflux) (Rossi, Pizzo, and Filadi, 2019; Walters and Usachev, 2023). (B) Ca2+-induced fluorescence as a measure of Ca2+ influx through MCU and Ca2+ efflux through NCLX in exchange for Na+. Mitochondria were added to the buffer with/without CGP-37157 and were allowed to equilibrate. Then, 20 μM of CaCl2 was added and allowed to equilibrate, which resulted in a sharp increase of fluorescence in the first portion of the plot (around 50–60 s), followed by a fluorescence decrease due to Ca2+ uptake by mitochondria via MCU channel, from 100 to 200 s (Ca2+ influx). 10 μM Ru360 was added to inhibit MCU and, approximately 60 s after, 20 mM of NaCl was added to initiate Na+ exchange for Ca2+ via NCLX. The increase in fluorescence (green) is visible after 260 s when NCLX is functional and almost no increase (black) when NCLX is inhibited by CGP-37157. Panel (B) shows a representative trace of mitochondrial extract from one animal with one technical replicate.
The fact that NCLX, an ion exchanger of biological relevance, has been given a dedicated acronym that reflects its established ability to transport Li+ naturally motivates one to consider it as a natural target to explore in terms of its response to the two Li+ isotopes. In this vein, our study aimed to uncover the effects of different Li+ isotopes on the NCLX exchanger in mouse heart mitochondria. Specifically, we measured the rates of Ca2+ efflux associated with NCLX using Ca2+-induced fluorescence and investigated a possible Li+ isotope fractionation within the mitochondrial matrix using inductively coupled plasma mass spectrometry (ICP-MS). We selected heart mitochondria as our model for several reasons. Firstly, heart mitochondria are the most extensively studied among mitochondria from various tissues. Secondly, the extraction of heart mitochondria is rather straightforward, providing a higher yield for multiple experiments and minimizing animal usage, in line with animal ethics guidelines. Finally, heart mitochondria bear significant similarities to brain mitochondria and, perhaps most importantly, are an exceedingly well-established model for studying NCLX and mitochondrial processes (Rysted et al., 2021). Thus the heart mitochondrion constitutes an excellent test candidate for a first exploration of the proposal that NCLX transport may be differently affected by the two Li+ isotopes.
Li salts, 6LiCl (95% 6Li) and 7LiCl (99% 7Li), as well as natural LiCl salt (with natural abundance - 6Li at 7.49% and 7Li at 92.51%) were purchased from Sigma-Aldrich. TMRE (T669), Calcium Green 5-N (C3737) and trace-metal grade HNO3 were purchased from Fisher Scientific. Percoll was purchased from Cedarlane. All other reagents were purchased from Sigma-Aldrich.
All procedures using mice were performed at the University of Waterloo (UW) according to animal use protocols that received ethics approval by the UW Animal Care Committee (i.e., AUPP#43325, approved 3 Jun 2021, and AUPP#44128, approved 11 March 2022), and complied with Canadian Council on Animal Care guidelines. Mice were housed in a temperature- and humidity-controlled environment in same-sex groups under a 12:12-h light/dark cycle, with free access to standard rodent chow (Teklad 22/5 Rodent diet from Envigo, Haslett, MI, USA) and water. Enrichment materials were provided. Cardiac mitochondria were isolated from male and female adult (i.e., 9–12 weeks old) C57BL/6J mice using a standard protocol (Elustondo et al., 2015; Rysted et al., 2021). In brief, whole hearts were harvested immediately after euthanasia by cervical dislocation, and the tissue was minced, washed with 1 × PBS containing 10 mM EDTA buffer (pH = 7.4), and digested with trypsin for 15 min, then homogenized in ice-chilled mitochondria isolation buffer (100 mM KCl, 50 mM MOPS, 5 mM MgSO4-7H2O, 1 mM EGTA, 0.1% BSA, pH 7.4 with KOH). The homogenate was centrifuged at 500 × g for 5 min, and then the supernatant was centrifuged at 10,000 × g for 10 min. The pellet was then resuspended in an isolation buffer, and layered on top of a Percoll gradient that was centrifuged at 7000 × g for 5 min. The final pellet was resuspended in sucrose wash buffer (250 mM sucrose, 10 mM HEPES, 0.1% BSA, pH 7.2), and the protein content of the sample was determined using the BCA method (Zhou et al., 2023). All pH adjustments were made with KOH and HCl to exclude the addition of extra Na+, which affect experimental results.
Mitochondrial Ca2+ flux was monitored using the low-affinity fluorescent label Calcium Green 5N, as a Ca2+ indicator (Kd∼14 µM) and measuring changes in the extramitochondrial Ca2+ concentration. Specifically, mitochondria were suspended in a respiration buffer (5 mM succinate, 300 mM sucrose, 20 mM TRIS, 0.2 mM KH2PO4, 1 µM Ca Green, 0.8 µM Rotenone, 5 µM CSA) in a 96 well, black plate. Ca Green 5N was excited at 500 nm and fluorescence collected at 541 nm using a Cytation5 Reader. For a standard experiment, mitochondria were added to the respiration buffer and equilibrated. Then, they were loaded with Ca2+ (20 µM), to enable uptake of Ca2+ by mitochondria via mitochondrial calcium uniporter (MCU), followed by the addition of the MCU inhibitor Ru360 (0.1 µM) to prevent further mitochondrial Ca2+ transport via MCU, thereby enabling the study of mitochondrial Ca2+ efflux via NCLX only. To probe for the role of NCLX, 20 mM of NaCl or 20 mM LiCl was added to mitochondria in the continuous presence of Ru360. For the inhibited NCLX study, CGP-37157 (30 µM) was added to the respiration buffer.
The rate of Ca2+ efflux was quantified as Ca2+ fluorescence per minute per mg of mitochondrial protein during the first minute of any given condition.
For the ICP-MS experiments, mitochondria were added to the respiration buffer and equilibrated. They were then loaded with Ca2+ (20 µM), followed by the addition of the MCU inhibitor Ru360 (0.1 µM) to prevent mitochondrial Ca2+ uptake. To determine the difference in Li+ isotopes and natLi+ versus Na+ passages, mitochondria were treated with a 20 mM Li+ isotope mix (10 mM 6Li+ and 10 mM 7Li+), or a 15 mM Na+/Li+ mix (5 mM Na+ and 10 mM natLi+) in the continuous presence of Ru360. To scrutinize the passage of Li+ in NCLX, in some of the experiments, NCLX was blocked by the addition of CGP-37157 (30 μM) to the respiration buffer. After 10 min, the mitochondria solutions were centrifuged (7,000 × g for 5 min), then supernatants and mitochondria pellets were separated and used for further analysis. The mitochondria pellets were triple-washed with resuspension to remove trapped Li+ or Na+ between mitochondria and in the intermembrane mitochondrial space with respiration buffer and inhibitors (Ru360 and CGP-37157). To confirm the effective elimination of Li+ or Na+ trapped in mitochondria pellets, the supernatant washes obtained after centrifugation (in the absence of mitochondrial samples) were subjected to ICP-MS analysis. The results revealed a rapid reduction in ion concentrations, meaning that trapped ions were successfully removed. The concentration of Li+ in the third wash was measured to be ∼104 times lower than in the supernatant, approaching a zero-value baseline, while the concentration of the Na+ also decreased, albeit not as drastic as for Li+, returning to the buffer nonzero base level (data not presented).
Next, purified mitochondria pellets and supernatants were analyzed with ICP-MS for Li+ and Na+ contents. The samples were treated as previously described (Deline et al., 2023). Briefly, each sample was digested in 1 mL concentrated (67%–70%, Fisher Scientific trace metal grade) HNO3 and 1 mL 30% H2O2 (Millipore Suprapur) for 1 h at 110°C to eliminate organic matter. Samples were evaporated to dryness and then diluted in 3 mL of 2% trace metal grade HNO3. The concentrations of 6Li, 7Li, and Na were determined using an Agilent 8800 triple quadrupole inductively coupled plasma mass spectrometer (QQQ-ICP-MS). Each sample solution was measured ten times, with each measurement comprising 1000 sweeps of the mass spectrum and 2-second total integration times for each Li isotope. Instrument drift was corrected using scandium (Sc) as the internal element standard. Instrumental accuracy was verified using multiple United States Geological Survey (USGS) and NIST SRM 1643f water standards and 7Li, 6Li, natLi, and mixed Li (∼47%/53% mix of 6Li and 7Li) control standards.
All Ca2+ fluorescence data were collected from a minimum of 3 biological replicates (defined as mitochondria extracts from a whole individual mouse heart) and 2 technical replicates (defined as multiple experiments from a given mouse heart). Data were analyzed by one-way ANOVA with Bonferroni’s post hoc test (for comparing >2 groups of data). Means ± SEM are plotted throughout, and the following significance levels are reported: n.s. (p > 0.05), p < 0.05, p < 0.01, p < 0.001, and p < 0.0001.
ICP-MS data were collected from 2 to 3 biological replicates (defined as mitochondria extracts from a whole individual mouse heart). Measurements resulting in less than 1 ppb Li were discarded since they did not yield reproducible results as the accuracy of standard Li isotope ratios degrades at low Li concentrations.
Recapping what was stated in the Introduction, mitochondria are known for storing Ca2+ and releasing it by exchanging it with Na+ or Li+ through NCLX. Two naturally occurring isotopes, 6Li and 7Li, have been observed to produce distinct effects on animal behaviour and activity (Lieberman, Alexander, and Stokes, 1979; Sechzer et al., 1986; Ettenberg et al., 2020), on electrical response in neuronal tissues (Esmaeilpour et al., 2023), and on mitochondria calcium buffering capacity (Deline et al., 2023). Most recently, the two Li+ isotopes have been found to differently affect the formation and properties of ACP in vitro (Fisher, 2023). Given the established role of Ca2+ in neuronal processes, we hypothesize that Li+ isotopes may have varying effects on Ca2+ release in mitochondria, ultimately leading to different downstream outcomes. Henceforth, for compactness, we refer to lithium salt samples with the natural isotopic abundance (6Li at 7.49% and 7Li at 92.51%) simply as “natural lithium.”
To measure Ca2+ efflux, we used the Ca Green-5N fluorescence label, which selectively fluoresces when bound to free Ca2+. Ca Green-5N was added to the buffer outside the mitochondria and, as it is unable to permeate into mitochondria, it provides a means to quantify the Ca2+ efflux by measuring fluorescence intensity. The fluorescence signal increases with elevated levels of free Ca2+ outside the mitochondria.
To establish a control experiment (Figure 1B) the fluorescence was measured to follow both Ca2+ influx and efflux: the mitochondria were introduced to the respiration buffer and allowed to equilibrate (Figure 1B, pre-time = 0). Subsequently, they were loaded with 20 µM Ca2+ (Figure 1B, indicated by the arrow at time = 100 s), followed by the addition of the MCU inhibitor Ru360 (Figure 1B, indicated by the arrow at time = 200 s) at 0.1 µM to prevent mitochondrial Ca2+ uptake. This design enabled the investigation of mitochondrial Ca2+ efflux.
Next, 20 mM of the selected salt (NaCl, in this example, indicated by the arrow at time = 280 s) was introduced to the mitochondria (Figure 1B, green curve). An increase in fluorescence during the time period spanning 280–800 s indicates Ca2+ efflux.
To inhibit the transport of ions through the NCLX channel, 30 µM CGP-37157 (NCLX inhibitor) was introduced to the respiration buffer. With the presence of CGP-37157, we observe a stable background fluorescence signal that just slightly increases overtime, indicating the essentially complete absence of Ca2+ release (Figure 1B, black curve).
With this experiment, we established our control and confirmed that the process of Ca2+ influx and efflux and Na+ to Ca2+ exchange via NCLX is operating in agreement with previously reported (Rysted et al., 2021) protocols in mice heart mitochondria at similar conditions.
These protocols were then applied in the following experiments to monitor Ca2+ efflux as a result of exchange between Ca2+ and Li+, as well as between Ca2+ and each of the two Li+ isotopes.
To assess NCLX activity, we examined Ca2+-induced fluorescence in the presence of different salts: NaCl, KCl, and natural LiCl (natLiCl) — both with and without CGP-37157 inhibitor. We anticipated that Na+ would induce a higher Ca2+ efflux than Li+ (Rysted et al., 2021), while K+ was not expected to elicit any Ca2+ release and was employed as a negative control. Following the control experimental methodology developed with Na+, described in Section 3.1 and Figure 1B, and through titration experiments with Na+ and Li+, we determined that a salt concentration of 20 mM yielded maximum Ca2+ efflux and was consequently utilized in all experiments (refer to Supplementary Figures S1A–D). While all procedures with Na+, K+ and Li+ were followed exactly as in the control experiment shown in Figure 1B, only the Ca2+ efflux portion of the experiments is presented and discussed in the plots below.
Upon introducing 20 mM NaCl (Figure 2A, green) or LiCl (Figure 2A, yellow) to the extramitochondrial solution, a pronounced increase in fluorescence was observed, signifying an induced Ca2+ efflux from the mitochondria, and thus indicating a successful exchange of Ca2+ for Na+ or Li+ via NCLX. To quantify and compare the Ca2+ effluxes, we utilized the Ca2+ efflux rate, measured as Ca2+-induced fluorescence per minute per mg of mitochondrial protein during the initial minute of any given condition (maximal slope). The Ca2+ efflux rate triggered by Li+ was significantly slower (p < 0.0001) than that induced by Na+ (Figure 2B, yellow and green, respectively). Notably, this Na+/Li+-induced Ca2+ efflux was inhibited by the application of the NCLX inhibitor CGP-37157 (Figure 2B, yellow and green, dashed). Conversely, the introduction of 20 mM KCl (Figures 2A, B, blue) did not stimulate mitochondrial Ca2+ efflux, as evidenced by the absence of increased fluorescence.
Figure 2. Ca2+-induced fluorescence as a probe of NCLX activity in the presence of Na+, Li+ and K+. (A) Representative Ca2+ efflux measures from isolated heart mitochondria during a 10 min period where NCLX is functional in the presence of 20 mM of Na+, natLi+, K+, or mitochondria alone. (B) Quantification of the maximal rates of mitochondrial Ca2+ efflux induced by Ru360 alone, 20 mM Na+, natLi+, or K+ in the continuous presence of Ru360 in case of functional or inhibited NCLX.
These results indicate that Na+ and Li+ trigger Ca2+ efflux via NCLX, and that Ca2+ efflux in response to Na+ is significantly higher than in response to Li+. CGP-37157 efficiently inhibits Ca2+ efflux triggered by the presence of Li+ or Na+. As expected, K+ do not exchange for Ca2+ via NCLX.
Next, we explored Ca2+ efflux stimulated by the simultaneous presence of Na+ and Li+. Previous studies have proposed distinct binding sites for Na+ and Li+ (Giladi et al., 2022), so we sought to examine how the simultaneous presence of Na+ and Li+ would influence Ca2+ efflux.
The experimental protocol outlined in Section 3.1 (Figure 1B), was adapted to incorporate varying concentrations of LiCl (5 and 10 mM) alongside 5 mM NaCl (Figures 3A, B). The results indicate that the Ca2+ efflux rate triggered by the simultaneous presence of Na+ and Li+ is significantly higher than the Ca2+ efflux rate triggered by Li+ alone: 5 mM Na+ and 10 mM Li+ results in 0.39 Ca2+ fl min−1 mg prot-1 compared to 0.17 Ca2+ fl min−1 mg prot−1 triggered by 15 mM Li+ (p < 0.01, Figure 3B; Supplementary Figures S1A, B); 5 mM Na+ and 5 mM Li+ results in 0.39 Ca2+ fl min−1 mg prot−1 compared to 0.12 Ca2+ fl min−1 mg prot−1 triggered by 10 mM Li+ (p < 0.001, Figure 3B). However, there is no statistically significant difference (p > 0.5) between the Ca2+ efflux rate triggered by the simultaneous presence of Na+ and Li+ and the Ca2+ efflux rate triggered by Na+ alone: 5 mM Na+ and 5 mM Li+ results in 0.39 Ca2+ fl min−1 mg prot-1 (Figure 3B) compared to 0.33 Ca2+ fl min−1 mg prot−1 triggered by 10 mM Na+ (Supplementary Figure S1B).
Figure 3. Ca2+-induced fluorescence as a measure of Ca2+efflux in response to 5 mM Na+ and different concentrations of natLi+ (5, 10 and 15 mM). (A) Representative Ca2+ efflux measurements from isolated heart mitochondria during a 10 min period where NCLX is functional in the presence of 5 mM Na+ and different concentrations of natLi+. (B) Quantification of the maximal rates of mitochondrial Ca2+ efflux induced by 5 mM Na+ and different concentrations of natLi+ in the continuous presence of Ru360.
Following the examination of NCLX properties, parallel studies were conducted using 6LiCl and 7LiCl salts. The introduction of 20 mM 6LiCl or 7LiCl to the extra-mitochondrial solution resulted in an observable increase in Ca2+ fluorescence, indicating an enhancement in Ca2+ efflux from the mitochondria. Figure 4A illustrates that mitochondrial Ca2+ efflux rates triggered by either Li+ isotope were comparable to the Ca2+ efflux induced by natLi salts. To test the specific role of NCLX in this process the NCLX exchanger was either left functional (without CGP-37157) or inhibited by CGP-37157 (Figure 4A, dashed).
Figure 4. Ca2+-induced fluorescence as a measure of Ca2+ efflux in response to Li+ isotope presence. (A) Quantification of the maximal rates of mitochondrial Ca2+ efflux induced by 20 mM natLi+, 6Li+ and 7Li+ in the continuous presence of Ru360 in the case of functional and inhibited NCLX. (B) Quantification of the maximal rates of mitochondrial Ca2+ efflux induced by 5 mM Na+ and different concentrations of natLi+, 6Li+ and 7Li+ in the continuous presence of Ru360 in the case of functional (i.e., uninhibited) NCLX.
Furthermore, as depicted in Figure 4B, there was no statistically significant difference (p > 0.5) observed in Ca2+ efflux rates between the simultaneous presence of 5 mM Na+ and 0–10 mM natural Li+ or either Li+ isotope.
Thus, fluorescence data show that there is no difference in Ca2+ efflux rate triggered by natLi+, 6Li+ or 7Li+ when Li salts were applied alone or in combination with 5 mM Na+. The CGP-37157 inhibitor efficiently inhibits Ca2+ efflux triggered by the presence of natLi+, 6Li+ or 7Li+, irrespective of which isotope is present.
We utilized ICP-MS to investigate the uptake level of Li+ and Na+ across the mitochondrial inner membrane—in the matrix. This analytical approach allows for the precise quantification of element concentrations and ratios within a sample. We hypothesized that there might be a different uptake of Na+ and Li+, and thus the ratio of Li+/Na+ inside the mitochondrion matrix would deviate from the original ratio in the extra-mitochondrial buffer.
Mitochondria were exposed to a respiration buffer containing 5 mM NaCl and 10 mM natLiCl for 10 min, enabling NCLX to uptake Na+ and natLi+ by exchanging for Ca2+ and thus releasing it. Subsequently, samples underwent centrifugation, and both the buffer and washed pellet (matrix) were collected for analysis of each ion content ratio inside and outside mitochondria. To quantify the relative uptake of natLi+ versus Na+, we define a natLi+/Na+ quantity. We calculated that natLi+/Na+ = 0.036 ± 0.050 in matrix compared to natLi+/Na+ = 0.448 ± 0.159 in buffer (SD, n = 3, Figure 5A; Supplementary Table S1). These results suggest that mitochondria preferentially uptake Na+ over natLi+.
Figure 5. Ion ratios inside and outside of mitochondria measured by ICP-MS. (A) Mitochondria were treated with a mixture of natLi+ and Na+. The graph shows the ratio of natLi+/Na+ inside (mitochondrial matrix) and outside (buffer) of mitochondria in the case of functional NCLX. (B) Mitochondria were treated with an approximate 47%/53% solution of 6Li+/7Li+. The graph shows the ratio of 7Li+/6Li+ inside (matrix) and outside (buffer) of mitochondria with and without NCLX inhibition.
In a similar experiment, mitochondria were exposed to a respiration buffer containing 20 mM LiCl with close to equimolar isotope abundance (47% 6Li and 53% 7Li), as determined by ICP-MS. These solutions were applied to mitochondria, where NCLX was either inhibited by CGP-37157 or not. To quantify the Li+ isotope fractionation between the mitochondrion matrix and buffer, we calculated 7Li+/6Li+. For the functional NCLX, we determined that 7Li+/6Li+ = 1.122 ± 0.001 (SD, n = 3) in buffer and 7Li+/6Li+ = 0.681 ± 0.019 (SD, n = 3) in mitochondrial matrix. For the inhibited NCLX, 7Li+/6Li+ = 1.124 ± 0.001 (SD, n = 2) in buffer and 7Li+/6Li+ = 0.537 ± 0.029 (SD, n = 2) in mitochondrial matrix (Figure 5B; Supplementary Table S2). These results suggest that mitochondria preferentially uptake 6Li+ over 7Li+ with or without the presence of CGP-37157, with 6Li+ uptake more strongly favored in the presence of CGP-37157 blocking NCLX.
Lithium (Li), administered in the form of carbonate or citrate salts, has long been used to treat bipolar disorder (Shorter, 2009), although its exact mechanism of action remains unclear (Kerr, Bjedov, and Sofola-Adesakin, 2018). Li has two stable isotopes, 6Li and 7Li, with varying effects on animal behaviour (Lieberman, Alexander, and Stokes, 1979; Sechzer et al., 1986; Ettenberg et al., 2020), electrical response in neuronal tissues (Esmaeilpour et al., 2023), mitochondrial Ca2+ buffering capacity (Deline et al., 2023), and the properties of amorphous calcium phosphate (ACP) clusters and their formation (Deline et al., 2023; Fisher, 2023). A recent study also reported that the Na+/H+ exchangers in fibroblast cells fractionate Li+ isotopes (Poet et al., 2023). The possibility of strong isotopic effects in neuroscience is highly unexpected. Were such isotopic differences to be rigorously demonstrated experimentally, those would have to be assigned to one, or both, of the two distinct properties of the two isotopes: their different mass and their different nuclear spin (note that nuclear magnetic dipole and electric quadrupole moments of the two isotopes also differ). Naively, on the basis of conventional atomic physics, and thus chemistry and biochemistry, those nuclear properties would have, evolutionary speaking, already been “folded in” the rather small differences each isotope would cause in terms of molecular bonding and ion channel transport when considering experiments with a natural abundance of the two Li isotopes. As such, any large experimental differences between the two Li isotopes would suggest that heretofore underappreciated significant quantum effects may be at play in Li’s neurological activity (Fisher, 2015; Weingarten, Doraiswamy, and Fisher, 2016; Ettenberg et al., 2020; Zadeh-Haghighi and Simon, 2021; Deline et al., 2023; Livingstone et al., 2023). Our own recent work (Esmaeilpour et al., 2023) demonstrates that a large and opposite effect of Li+ isotopes is observed in electrical activity (evoked field postsynaptic potential measurements) of animal brain slices, indicating that fast electrochemical processes associated with ion channels or synaptic transmission may be involved. The works by Deline et al. (2023) and Fisher (2023) indicate that the two Li+ isotopes differentially affect the properties of ACP and mitochondrial calcium buffering capacity, which suggests that downstream Ca2+ signaling could be affected by such. Processes of Ca2+ aggregation and storage in mitochondria are important in Ca2+ regulation and its dysregulation has been linked to various neurological disorders (Khacho, Harris, and Slack, 2019; Calvo-Rodriguez et al., 2020; Cascella and Cecchi, 2021).
Beyond Li, results from experiments considering isotopes of other elements have recently been found to challenge the traditional view that the different isotopes of a given element should minimally impact biology –– the case of proton (H) versus deuterium (D) is a well-known counterexample finding its origin in the large kinetic isotope effect driven by the 100% mass difference between H and D (Di Martino, Maxwell, and Pirali, 2023). Stable oxygen isotope fractionation in chiral environments has been reported (Vardi et al., 2023). Intriguingly, the efficacy of xenon-induced general anesthesia has recently been reported to exhibit a strong dependence on the xenon isotope considered (Li et al., 2018). Integrating these recent observations along with the aforementioned reports of Li isotope effects in a number of experiments of neurological context emphasizes that various isotopes of a given element, not just Li, may have distinct effects. These observations could pave the way for uncovering unexpected quantum effects emerging at temperature scales and environmental conditions pertaining to biological systems, but more experiments are needed.
Intriguing theoretical hypotheses have been advanced to explain the striking differences between the two Li+ isotopes (Fisher, 2015; Weingarten, Doraiswamy, and Fisher, 2016; Zadeh-Haghighi and Simon, 2021), but experimental evidence thus far available has not been sufficient to elucidate the mechanism of such isotope action or to identify the molecular site(s) or target(s) responsible for such downstream Li isotope neurological effects, or to ascertain whether the isotopic difference is caused by the relatively large (∼15%) mass difference between the two isotopes (e.g., as in the kinetic isotope effect or other known mass-independent nuclear quantum effects) or their different nuclear spin (i.e., spin 1 and 3/2 for 6Li and 7Li, respectively). This is a challenging task since essentially all knowledge regarding Li biochemistry has been established on the basis of studies involving natural Li (natLi) salts, which are mixtures of the two Li isotopes. Compounding the challenge, while Li’s clinical applications are established, the full set of its identified biomolecular targets are neither entirely known nor are all mechanisms fully understood. In the search of the molecular targets for Li+ action, many directions have been considered. In this regard, we chose to explore the role of mitochondrial NCLX in its capacity to exchange Ca2+ for Li+ and as a potential target for Li+ isotope differentiation. It has been shown that Li+ modulates the activity of the NCLX (Boyman et al., 2013) and, by doing so, could potentially influence Ca2+ signaling in the brain. It has been proposed that NCLX has distinct binding sites for Na+ and Li+ and it was shown that the NCLX_Mj mutant can alternatively bind either one ion of Ca2+ or 2 Na+/2 Li+ ions at different stages of the transport cycle (Giladi et al., 2022). Given the crucial role of Ca2+ in the regulation of neuronal processes (Brini et al., 2014), the effects of Li+ could occur through modulating Ca2+ levels, emphasizing its significance in neurotransmitter release, synaptic plasticity, and overall neuronal function.
In this work, we measured mitochondrial Ca2+ efflux in the presence of natLi+, Na+, or K+, both under functional and inhibited NCLX conditions. Our findings for natLi and Na salts correlate well with available published data (Ruiz, Alberdi, and Matute, 2014; Rysted et al., 2021). Titration experiments confirm the effects of 0–20 mM Na+ or Li+ salts on Ca2+ efflux rate (Boyman et al., 2013). Interestingly, we observed that the rate of Ca2+ efflux drops significantly at increased concentrations of Na+ or Li+ (at around 30–45 mM) (Supplementary Figures S1A–D). Further, our data demonstrate that the simultaneous presence of Na+ and Li+ salts results in the enhancement of Ca2+ efflux when compared to Li+ alone but is similar when only Na+ is used. In regard to the latter results, our initial hypothesis was that the interaction of one of the Li+ isotopes with NCLX might influence Na+-induced Ca2+ release differently from that of the other Li+ isotope. If occurring, such an effect may be studied in Na+/Li+ mixtures given the documented distinctions in binding sites for Na+ and Li+ in NCLX (Giladi et al., 2022). The existence of different binding sites is noteworthy, as these sites may exhibit distinct affinities, potentially influencing the exchanger’s capacity, ionic exchange rate, and/or ionic selectivity. We were unable to detect a significant difference between Ca2+ efflux evoked by Na+ alone or the efflux caused by the combination of Na+ and Li+, for a same total concentration of ions, possibly due to the intrinsic limitations in the fluorescence method employed and the small signal induced by Li+. However, we observed a difference in Ca2+ efflux evoked by Li+ acting alone compared to the efflux caused by Li+ and Na+ together, again for a same ionic concentration. These results suggest a potential cumulative effect of Na+ and Li+.
While there is a pronounced effect of Na+ and Li+ on Ca2+ transport via NCLX, the role of Li+ isotopes had previously not been investigated in this regard. Our studies demonstrate no discernible difference in Ca2+ efflux rates triggered by natLi+, 6Li+, or 7Li+. We found that CGP-37157 efficiently inhibits Ca2+ efflux irrespective of which isotope is used. We also observed no difference between the Ca2+ efflux rate triggered by the simultaneous presence of Na+ and various concentrations of natLi+, 6Li+ or 7Li+. Based on Ca2+ fluorescence results, we conclude that NCLX does not differentiate between the two Li+ isotopes, suggesting an equivalence in transport function and binding site interactions for 6Li+ or 7Li+, or an undetectable small difference between the two isotopes, under our experimental conditions and with the methods used in this work. Having found that NCLX itself does not differentiate between the Li+ isotopes, we explored whether there exists an overall differentiation between the two Li+ isotopes at the level of the inner mitochondrial membrane, as it is known that Li+ can penetrate different biological membranes differently (Sherman, Munsell, and Wong, 1984; Hughes and Birch, 1992; Poet et al., 2023). We used the ICP-MS technique to test whether the two Li+ isotopes penetrate mitochondrial membranes differently by measuring the amount of each Li+ isotope inside and outside mitochondria.
To establish a baseline for our experiments, we started with Na+ and natLi+ simultaneously present and compared these with Li+ isotopes. We found that mitochondria uptake Na+ preferentially over Li+ (natLi+/Na+ = 0.036 ± 0.050 in matrix compared to natLi+/Na+ = 0.448 ± 0.159 in buffer). Here, we need to highlight the point that since experiments with Na+ were performed only in the case of functional NCLX, we cannot assume that NCLX is the sole path for Na+ entry into mitochondria leading to Na+ versus Li+ differentiation. Indeed, it is known that there are other ion exchangers in the mitochondrial inner membrane, such as the Na+-H+ exchanger (Numata et al., 1998), that might likely preferentially uptake Na+ over Li+. Therefore, the possibility does exist that Na+ is preferentially taken up over Li+ due to the availability of multiple pathways for Na+ entry.
In the ICP-MS measurements, we found that 6Li+ is enriched across the mitochondria inner membrane regardless of the presence of CGP-37157 (7Li+/6Li+ = 1.122 ± 0.001 in buffer vs. 7Li+/6Li+ = 0.681 ± 0.019 in mitochondrial matrix for functional NCLX and 7Li+/6Li+ = 1.124 ± 0.001 in buffer vs. 7Li+/6Li+ = 0.537 ± 0.029 in mitochondrial with NCLX blocked by CGP-37157). Interestingly, we find a rather large degree of 7Li+/6Li+ fractionation in the mitochondrial matrix with functional NCLX, which is even higher when NCLX is inhibited. These results suggest that not only are there other Li+ transport pathways in mitochondria that differentiate between the Li+ isotopes, but also that NCLX either does not differentiate between the Li+ isotopes or has less preference for 6Li+ compared to other pathways. It would seem likely that Li+ isotope differentiation occurs via Na+ exchangers, which are present in the mitochondrial inner membrane, since it is well known that Li+ can enter Na+ channels (Richelson, 1977; Thomsen and Shirley, 2006). We thus conclude that the inner mitochondrial membrane differentiates between Li+ isotopes within the precision and accuracy of the ICP-MS method used in the present work, but that this differentiation is not solely due to NCLX, if NCLX fractionates Li isotopes at all. Moreover, and perhaps most importantly, this 6Li+ enrichment does not affect the Ca2+ release via NCLX, as was shown by our Ca2+ fluorescence experiments. From the perspective of our work, these results would suggest that the Li+ isotope fractionation exposed through the ICP-MS measurements does not translate into a downstream effect via Ca2+ release through the NCLX. This is noteworthy considering that Ca2+ is a crucial ion associated with electrochemical neuronal signaling. Thus, our results would suggest that NCLX function cannot be directly related to the Li+ isotope effects seen in animal and tissue experiments (Sechzer et al., 1986; Esmaeilpour et al., 2023).
It is of interest to contrast the large Li isotope fractionation we find in the present work with two recent reports on Li isotope fractionation by Deline et al. (2023); Poet et al. (2023). In their work and ours, Li+ isotope fractionation by the mitochondrial membrane, using ICP-MS, and Ca2+ release, using Ca2+-induced fluorescence, were assessed. Deline et al. (2023) reported a Li+ isotope effect on Ca2+ buffering capacity as signaled by the mitochondria permeability transition pore (mPTP) opening event while they did not find a differential Li+ isotope uptake by mitochondria. Conversely, we tested NCLX, and observed that Li+ isotope partitioning in mitochondria did not affect Ca2+ efflux though NCLX. The experimental design as well as the types of mitochondria (brain and liver) used by Deline et al. (2023) differ from those considered in the present work, and it is therefore not possible at this time to explicitly state the specific reason(s) why the results differ. However, we believe that this question is interesting and worth exploring further using other experimental designs and utilizing more precise methods [e.g., Li purification by ion-exchange chromatography followed by MC-ICP-MS analysis as carried out by Poet et al. (2023)].
Another point of contrast warrants mention. In the recent work of Poet et al. (2023), it was shown that the Na+-H+ exchanger differentiates between Li+ isotopes and transports 6Li+ at a higher rate than 7Li+. Similarly to the Poet et al. (2023) results, we see an enrichment of 6Li+ in mitochondrial matrix compared to an enrichment of 6Li+ in fibroblast cells. We must point out, however, that our values are not directly comparable — as we utilized different systems, experimental designs and different ways of calibrating the ICP-MS measurements (natLi standard versus 6Li/7Li 47%/53% mixture). For example, in our case, we are studying isolated heart mitochondria that have large negative membrane potential (−180 mV), while Poet et al. (2023) studied fibroblast cells. We believe that the pathway of Li+ isotopes differentiation should be studied more extensively and propose this as a future research project.
Although we observed a discernable Li+ isotope differentiation by the mitochondrial membrane, we do not think that these results readily explain those from tissues and animals studies (Sechzer et al., 1986; Esmaeilpour et al., 2023). The lithium isotope effects found by Esmaeilpour et al. (2023) may be due to electrochemical activity which involves ion transport and signaling. As Ca2+ signaling is one of the main mechanisms to initiate the neuronal response in the brain, the NCLX, which transports Ca2+ in exchange for Na+ and Li+, may have been considered as a natural route/mechanism where Li+ isotopes can affect Ca2+ transport and would be a target to differentiate Li+ isotopes. However, our Ca2+-induced fluorescence measurements reveal no detectable isotope effects on the Ca2+ efflux through NCLX, undermining the credibility of NCLX being the origin of the effects seen in brain tissues (Esmaeilpour et al., 2023) and animal behaviour (Sechzer et al., 1986). It would therefore be interesting to investigate alternative mechanisms and pathways to test the effects of Li+ isotopes on Ca2+ signaling. This may include inositol monophosphatase (IMPase) within the hosphatidylinositol (PI) signaling pathway (Berridge, Downes, and Hanley, 1989), and the effect of Li+ on Ca2+ channels activity (McCarthy et al., 2016), endoplasmic reticulum (He et al., 2017), and the mitochondrial respiratory chain (Maurer, Schippel, and Volz, 2009). Given Li’s ability to act on multiple targets simultaneously, it might also be worthwhile to explore Ca2+ flux as a function of Li+ concentration in vivo using live cell model systems. It is worth noting that known Li+ effects may be attributed to further downstream neuronal or behavioural effects (Pasquali et al., 2010) arising from subtle Li+ actions on a variety of molecular targets, which would explain why drastic Li+ isotope effects can be observed at the tissue and animal levels but not at the subcellular/cellular levels.
In conclusion, we report a Li+ isotope differentiation in heart mouse mitochondria, determined by ICP-MS, that does not affect Ca2+ efflux via the sodium/calcium/lithium exchanger (NCLX), measured by Ca2+-induced fluorescence. Future research focused on already acknowledged targets for natural isotopic abundance Li may provide promising avenues for exploring Li isotope effects in the realm of neuroscience. However, were such molecular levels of Li isotope action to be convincingly exposed, their consequential downstream effects in neuronal activity will necessitate comprehensive investigations.
The raw data supporting the conclusion of this article will be made available by the authors, without undue reservation.
The animal study was approved by UW Animal Care Committee (i,e., AUPP#43325, approved 3 Jun 2021, and AUPP#44128, approved 11 March 2022). The study was conducted in accordance with the local legislation and institutional requirements.
IB: Data curation, Formal Analysis, Investigation, Methodology, Project administration, Validation, Visualization, Writing–original draft, Writing–review and editing, Conceptualization. FR: Methodology, Writing–review and editing. BK: Data curation, Formal Analysis, Investigation, Methodology, Resources, Supervision, Validation, Writing–review and editing. RD: Resources, Writing–review and editing, Methodology. JQ: Resources, Writing–review and editing, Methodology. EP: Conceptualization, Methodology, Project administration, Supervision, Validation, Writing–review and editing. MG: Conceptualization, Funding acquisition, Supervision, Writing–review and editing. ZL: Conceptualization, Funding acquisition, Project administration, Supervision, Writing–review and editing, Validation.
The author(s) declare that financial support was received for the research, authorship, and/or publication of this article: New Frontiers in Research Fund (NFRF), grant # 50383-10011, Ionis Pharmaceutical and Quantum Brain Network, grant #52504-10001, Natural Sciences and Engineering Research Council of Canada (NSERC), grants #RGPIN-2019-05642 and RGPAS-2019-00008.
The authors acknowledge funding from New Frontiers in Research Fund (NFRF) Exploration Grant (ZL and MJPG) # 50383-10011 and Natural Sciences and Engineering Research Council (NSERC) Grants #RGPIN-2019-05642 and RGPAS-2019-00008 (RED), support from the Quantum Brain Network led by M. Fisher (UCSB) and from IONIS pharmaceutical #52504-10001. IB acknowledges the Waterloo Institute for a Nanotechnology (WIN) Nanofellowship. We acknowledge a WIN visiting fellowship to EP. MJPG and BK are supported by the Canada Research Chairs (CRC) program and ZL by the NSERC program. We thank K. De Silva in Duncan’s lab for their help with mitochondria preparation. We acknowledge the help of J. D. Livingstone with sample preparation for ICP-MS measurements and B. Lee for making the schematic in Figure 1. We appreciate stimulating and useful discussions with M. Fisher and all members of the Waterloo Quantum Biology team.
The authors declare that the research was conducted in the absence of any commercial or financial relationships that could be construed as a potential conflict of interest.
All claims expressed in this article are solely those of the authors and do not necessarily represent those of their affiliated organizations, or those of the publisher, the editors and the reviewers. Any product that may be evaluated in this article, or claim that may be made by its manufacturer, is not guaranteed or endorsed by the publisher.
The Supplementary Material for this article can be found online at: https://www.frontiersin.org/articles/10.3389/fphys.2024.1354091/full#supplementary-material
Abbott D., Davies P., Pati A. K. (2008). Quantum aspects of life. Covent Garden, London, United Kingdom: Imperial College Press. doi:10.1142/P581
Alexander G. J., Lieberman K. W., Okamoto M., Stokes P. E., Triana E. (1982). Lithium toxicology: effect of isotopic composition on lethality and behavior. Pharmacol. Biochem. Behav. 16 (5), 801–804. doi:10.1016/0091-3057(82)90238-6
Berridge M. J., Downes C. P., Hanley M. R. (1989). Neural and developmental actions of lithium: a unifying hypothesis. Cell 59 (3), 411–419. doi:10.1016/0092-8674(89)90026-3
Bosche B., Schäfer M., Graf R., Härtel F. V., Schäfer U., Noll T. (2013). Lithium prevents early cytosolic calcium increase and secondary injurious calcium overload in glycolytically inhibited endothelial cells. Biochem. Biophysical Res. Commun. 434 (2), 268–272. doi:10.1016/j.bbrc.2013.03.047
Boyman L., Williams G. S. B., Khananshvili D., Sekler I., Lederer W. J. (2013). NCLX: the mitochondrial sodium calcium exchanger. J. Mol. Cell. Cardiol. 59, 205–213. doi:10.1016/j.yjmcc.2013.03.012
Brini M., Calì T., Ottolini D., Carafoli E. (2014). Neuronal calcium signaling: function and dysfunction. Cell. Mol. Life Sci. CMLS 71 (15), 2787–2814. doi:10.1007/s00018-013-1550-7
Calvo-Rodriguez M., Hou S. S., Snyder A. C., Kharitonova E. K., Russ A. N., Das S., et al. (2020). Increased mitochondrial calcium levels associated with neuronal death in a mouse model of alzheimer’s disease. Nat. Commun. 11 (1), 2146. doi:10.1038/s41467-020-16074-2
Cascella R., Cecchi C. (2021). Calcium dyshomeostasis in alzheimer’s disease pathogenesis. Int. J. Mol. Sci. 22 (9), 4914. doi:10.3390/ijms22094914
Deline M. L., Straub J., Patel M., Subba P., Grashei M., Fritsvan Heijster H. A., et al. (2023). Lithium isotopes differentially modify mitochondrial amorphous calcium phosphate cluster size distribution and calcium capacity. Front. Physiology 14, 1200119. doi:10.3389/fphys.2023.1200119
Di Martino R. M., Maxwell B. D., Pirali T. (2023). Deuterium in drug discovery: progress, opportunities and challenges. Nat. Rev. Drug Discov. 22 (7), 562–584. doi:10.1038/s41573-023-00703-8
Dubovsky S. L., Franks R. D. (1983). Intracellular calcium ions in affective disorders: a review and an hypothesis. Biol. Psychiatry 18 (7), 781–797.
Elustondo P. A., Alexander N., Kane L., Kane D. A., Pavlov E. V. (2015). Spermine selectively inhibits high-conductance, but not low-conductance calcium-induced permeability transition pore. Biochimica Biophysica Acta (BBA) - Bioenergetics 1847 (2), 231–240. doi:10.1016/j.bbabio.2014.10.007
Ettenberg A., Ayala K., Krug J. T., Collins L., Mayes M. S., Fisher M. P. A. (2020). Differential effects of lithium isotopes in a ketamine-induced hyperactivity model of mania. Pharmacol. Biochem. Behav. 190, 172875. doi:10.1016/j.pbb.2020.172875
Fisher M. P. A. (2015). Quantum cognition: the possibility of processing with nuclear spins in the brain. Ann. Phys. 362, 593–602. doi:10.1016/j.aop.2015.08.020
Giladi M., Mitra S., Simhaev L., Hiller R., Refaeli B., Strauss T., et al. (2022). Exploring the Li+ transporting mutant of NCX_mj for assigning ion binding sites of mitochondrial NCLX. Cell Calcium 107, 102651. doi:10.1016/j.ceca.2022.102651
Glen A. I. (1985). Lithium prophylaxis of recurrent affective disorders. J. Affect. Disord. 8 (3), 259–265. doi:10.1016/0165-0327(85)90024-2
Harrison P. J., Hall N., Mould A., Al-Juffali N., Tunbridge E. M. (2021). Cellular calcium in bipolar disorder: systematic review and meta-analysis. Mol. Psychiatry 26 (8), 4106–4116. doi:10.1038/s41380-019-0622-y
He Z., Zhou Y., Wang Q., Li J., Zheng Z., Chen J., et al. (2017). Inhibiting endoplasmic reticulum stress by lithium chloride contributes to the integrity of blood-spinal cord barrier and functional recovery after spinal cord injury. Am. J. Transl. Res. 9 (3), 1012–1024.
Helmeste D. M., Tang S. W. (1998). The role of calcium in the etiology of the affective disorders. Jpn. J. Pharmacol. 77 (2), 107–116. doi:10.1254/jjp.77.107
Hughes M. S., Birch N. J. (1992). Isotopic differences in the lithium transport rate in human erythrocytes during simultaneous incubations with the stable isotopes 6Li and 7Li. Comptes Rendus l’Academie Sci. Ser. III, Sci. La Vie 314 (4), 153–158.
Kerr F., Bjedov I., Sofola-Adesakin O. (2018). Molecular mechanisms of lithium action: switching the light on multiple targets for dementia using animal models. Front. Mol. Neurosci. 11, 297. doi:10.3389/fnmol.2018.00297
Khacho M., Harris R., Slack R. S. (2019). Mitochondria as central regulators of neural stem cell fate and cognitive function. Nat. Rev. Neurosci. 20 (1), 34–48. doi:10.1038/s41583-018-0091-3
Kuo C. C., Hess P. (1993). Characterization of the high-affinity Ca2+ binding sites in the L-type Ca2+ channel pore in rat phaeochromocytoma cells. J. Physiology 466, 657–682. doi:10.1113/jphysiol.1993.sp019739
Li N., Lu D., Yang L., Tao H., Xu Y., Wang C., et al. (2018). Nuclear spin attenuates the anesthetic potency of xenon isotopes in mice: implications for the mechanisms of anesthesia and consciousness. Anesthesiology 129 (2), 271–277. doi:10.1097/ALN.0000000000002226
Lieberman K. W., Alexander G. J., Stokes P. (1979). Dissimilar effects of lithium isotopes on motility in rats. Pharmacol. Biochem. Behav. 10 (6), 933–935. doi:10.1016/0091-3057(79)90070-4
Livingstone J. D., Gingras M. J. P., Leonenko Z., Beazely M. A. (2023). Search for lithium isotope effects in neuronal HT22 cells. Biochem. Biophysics Rep. 34, 101461. doi:10.1016/j.bbrep.2023.101461
Marais A., Adams B., Ringsmuth A. K., Ferretti M., Michael Gruber J., Hendrikx R., et al. (2018). The future of quantum biology. J. R. Soc. Interface 15 (148), 20180640. doi:10.1098/rsif.2018.0640
Maurer I. C., Schippel P., Volz H.-P. (2009). Lithium-induced enhancement of mitochondrial oxidative phosphorylation in human brain tissue. Bipolar Disord. 11 (5), 515–522. doi:10.1111/j.1399-5618.2009.00729.x
McCarthy M. J., Le Roux M. J., Wei H., Stephen B., Kelsoe J. R., Welsh D. K. (2016). Calcium Channel genes associated with bipolar disorder modulate lithium’s amplification of circadian rhythms. Neuropharmacology 101, 439–448. doi:10.1016/j.neuropharm.2015.10.017
M. Mohseni, Y. Omar, G. Engel, and M. B. Plenio (2014). Quantum effects in biology (Cambridge: Cambridge University Press).
Numata M., Kevin P., Lake N., Orlowski J. (1998). Identification of a mitochondrial Na+/H+Exchanger *. J. Biol. Chem. 273 (12), 6951–6959. doi:10.1074/jbc.273.12.6951
Parthasarathy R., Eisenberg F. (1984). Lack of differential lethality of lithium isotopes in mice. Ann. N. Y. Acad. Sci. 435 (1), 463–465. doi:10.1111/j.1749-6632.1984.tb13853.x
Parthasarathy R., Parthasarathy L., Ramesh T. G., Shyamala Devi C. S., Vadnal R. E. (1992). The effects of lithium isotopes on the myo-inositol 1-phosphatase reaction in rat brain, liver, and testes. Life Sci. 50 (19), 1445–1450. doi:10.1016/0024-3205(92)90263-O
Pasquali L., Busceti C. L., Fulceri F., Paparelli A., Fornai F. (2010). Intracellular pathways underlying the effects of lithium. Behav. Pharmacol. 21 (5–6), 473–492. doi:10.1097/FBP.0b013e32833da5da
Poet M., Vigier N., Bouret Y., Jarretou G., Gautier R., Bendahhou S., et al. (2023). Biological fractionation of lithium isotopes by cellular Na+/H+ exchangers unravels fundamental transport mechanisms. iScience 26 (6), 106887. doi:10.1016/j.isci.2023.106887
Richelson E. (1977). Lithium ion entry through the sodium channel of cultured mouse neuroblastoma cells: a biochemical study. Sci. (New York, N.Y.) 196 (4293), 1001–1002. doi:10.1126/science.860126
Rossi A., Pizzo P., Filadi R. (2019). Calcium, mitochondria and cell metabolism: a functional triangle in bioenergetics. Biochimica Biophysica Acta. Mol. Cell Res. 1866 (7), 1068–1078. doi:10.1016/j.bbamcr.2018.10.016
Ruiz A., Alberdi E., Matute C. (2014). CGP37157, an inhibitor of the mitochondrial Na+/Ca2+ exchanger, protects neurons from excitotoxicity by blocking voltage-gated Ca2+ channels. Cell Death Dis. 5 (4), e1156. doi:10.1038/cddis.2014.134
Rysted J. E., Lin Z., Walters G. C., Maria Noterman A. J. R., Usachev Y. M., Liu G., et al. (2021). Distinct properties of Ca2+ efflux from brain, heart and liver mitochondria: the effects of Na+, Li+ and the mitochondrial Na+/Ca2+ exchange inhibitor CGP37157. Cell Calcium 96, 102382. doi:10.1016/j.ceca.2021.102382
Sechzer J. A., Lieberman K. W., Alexander G. J., Weidman D., Stokes P. E. (1986). Aberrant parenting and delayed offspring development in rats exposed to lithium. Biol. Psychiatry 21 (13), 1258–1266. doi:10.1016/0006-3223(86)90308-2
Sherman W. R., Munsell L. Y., Wong Y. H. (1984). Differential uptake of lithium isotopes by rat cerebral cortex and its effect on inositol phosphate metabolism. J. Neurochem. 42 (3), 880–882. doi:10.1111/j.1471-4159.1984.tb02765.x
Shorter E. (2009). The history of lithium therapy. Bipolar Disord. 11 (2), 4–9. doi:10.1111/j.1399-5618.2009.00706.x
Thompson A. N., Kim I., Panosian T. D., Iverson T. M., Allen T. W., Nimigean C. M. (2009). Mechanism of potassium-channel selectivity revealed by Na(+) and Li(+) binding sites within the KcsA pore. Nat. Struct. Mol. Biol. 16 (12), 1317–1324. doi:10.1038/nsmb.1703
Thomsen K., Shirley D. G. (2006). A hypothesis linking sodium and lithium reabsorption in the distal nephron. Nephrol. Dial. Transplant. 21 (4), 869–880. Official Publication of the European Dialysis and Transplant Association - European Renal Association. doi:10.1093/ndt/gfk029
Vardi O., Maroudas-Sklare N., Kolodny Y., Volosniev A., Saragovi A., Galili N., et al. (2023). Nuclear spin effects in biological processes. Proc. Natl. Acad. Sci. 120 (32), e2300828120. doi:10.1073/pnas.2300828120
Walters G. C., Usachev Y. M. (2023). Mitochondrial calcium cycling in neuronal function and neurodegeneration. Front. Cell Dev. Biol. 11, 1094356. doi:10.3389/fcell.2023.1094356
Weingarten C. P., Murali Doraiswamy P., Fisher M. P. A. (2016). A new spin on neural processing: quantum cognition. Front. Hum. Neurosci. 10, 541. doi:10.3389/fnhum.2016.00541
Zadeh-Haghighi H., Simon C. (2021). Entangled radicals may explain lithium effects on hyperactivity. Sci. Rep. 11 (1), 12121. doi:10.1038/s41598-021-91388-9
Keywords: lithium isotopes fractionation, mitochondria, NCLX, Na/Ca/Li exchange, fluorescence, ICP-MS
Citation: Bukhteeva I, Rahman FA, Kendall B, Duncan RE, Quadrilatero J, Pavlov EV, Gingras MJP and Leonenko Z (2024) Effects of lithium isotopes on sodium/lithium co-transport and calcium efflux through the sodium/calcium/lithium exchanger in mitochondria. Front. Physiol. 15:1354091. doi: 10.3389/fphys.2024.1354091
Received: 11 December 2023; Accepted: 06 March 2024;
Published: 09 April 2024.
Edited by:
Youngchan Kim, University of Surrey, United KingdomReviewed by:
Rebecca Lewis, University of Surrey, United KingdomCopyright © 2024 Bukhteeva, Rahman, Kendall, Duncan, Quadrilatero, Pavlov, Gingras and Leonenko. This is an open-access article distributed under the terms of the Creative Commons Attribution License (CC BY). The use, distribution or reproduction in other forums is permitted, provided the original author(s) and the copyright owner(s) are credited and that the original publication in this journal is cited, in accordance with accepted academic practice. No use, distribution or reproduction is permitted which does not comply with these terms.
*Correspondence: Zoya Leonenko, emxlb25lbmtAdXdhdGVybG9vLmNh
Disclaimer: All claims expressed in this article are solely those of the authors and do not necessarily represent those of their affiliated organizations, or those of the publisher, the editors and the reviewers. Any product that may be evaluated in this article or claim that may be made by its manufacturer is not guaranteed or endorsed by the publisher.
Research integrity at Frontiers
Learn more about the work of our research integrity team to safeguard the quality of each article we publish.