- Section for Cell Biology and Physiology, Department of Biology, University of Copenhagen, Copenhagen, Denmark
The remarkable regenerative ability of the skin, governed by complex molecular mechanisms, offers profound insights into the skin repair processes and the pathogenesis of various dermatological conditions. This understanding, derived from studies in human skin and various model systems, has not only deepened our knowledge of skin regeneration but also facilitated the development of skin substitutes in clinical practice. Recent research highlights the crucial role of lymphatic vessels in skin regeneration. Traditionally associated with fluid dynamics and immune modulation, these vessels are now recognized for interacting with skin stem cells and coordinating regeneration. This Mini Review provides an overview of recent advancements in basic and translational research related to skin regeneration, focusing on the dynamic interplay between lymphatic vessels and skin biology. Key highlights include the critical role of stem cell-lymphatic vessel crosstalk in orchestrating skin regeneration, emerging translational approaches, and their implications for skin diseases. Additionally, the review identifies research gaps and proposes potential future directions, underscoring the significance of this rapidly evolving research arena.
Introduction
The skin, a dynamic and multifaceted organ, is widely recognized for its remarkable regenerative capabilities. Its ability to regenerate serves as a foundational knowledge base for stem cell research and is crucial in advancing studies related to wound repair and skin transplantation techniques. Exploring skin regeneration in different model systems and human skin has yielded insights into the complex orchestration of stem cell mechanisms and regenerative processes, shedding light on similar pathways in other tissues. The resilience of skin can be largely attributed to an intricate interplay of biological processes, which encompass immune defense, contraction of fibers and matrix, dynamic fluid transport, and regenerative signals that interact closely with stem cells. This Mini review aims to provide an overview of the recent developments in dermal lymphatics within the context of skin regeneration. It particularly focuses on the newly recognized interactions between lymphatic vessels and hair follicle stem cells. Given the individual significance of hair follicle stem cells and lymphatics in wound repair, this Mini review also outlines potential future research directions, exploring their synergy in wound repair and skin bioengineering. This emerging and evolving field promises to open new avenues in regenerative medicine.
Skin: a layered complexity
The skin comprises the epidermis, dermis, and hypodermis, serving as a crucial interface between the body’s internal and external environments. Its intricate, multilayered structure endows it with a plethora of functions. As a protective barrier, it shields against pathogenic, mechanical, solar, and toxic threats. Its multilayered structure provides protective, sensory, thermoregulatory, and metabolic functions and immunological defense (Hsu and Fuchs, 2022).
The outermost layer, the epidermis, is primarily composed of epidermal keratinocytes and is interspersed with sweat glands and hair follicles, each exhibiting regenerative capabilities attributable to distinct populations of stem cells (Watt, 2014; Hsu and Fuchs, 2022). The dermis, rich in collagen, elastin fibers, proteoglycans, and hyaluronic acid, provides structural integrity, and harbors fibroblasts, blood and lymphatic vessels, nerves, and immune cells supporting the diversity of skin’s functions (Smith et al., 1982; Skobe and Detmar, 2000; Kupper and Fuhlbrigge, 2004; Prost-Squarcioni et al., 2008; Owens and Lumpkin, 2014; Glatte et al., 2019; Kobayashi et al., 2019; Theocharidis and Connelly, 2019; Plikus et al., 2021; Kam et al., 2023). Situated beneath the dermis, the hypodermis mainly comprises adipocytes, collagen, and blood and lymphatic vessels. It is crucial for structural support, thermoregulation and acts as an energy store, playing endocrine and regenerative roles (Driskell et al., 2014). All layers contribute to skin regeneration and repair, pivotal in research and clinical practice. Lymphatic capillaries and vessels across these layers are essential for fluid and immune transport, with emerging roles in regeneration.
Skin lymphatic vasculature
The skin possesses a complex network of blood and lymphatic vasculature, each playing distinct yet complementary roles in skin physiology and pathology. The blood vascular system, known for providing oxygen and nutrients and facilitating immune cell traffic, influences the behavior and functionality of epidermal and hair follicle stem cells, impacting wound repair and skin engineering (Tonnesen et al., 2000; Johnson and Wilgus, 2014; Sada et al., 2016; Li et al., 2019; Oualla-Bachiri et al., 2020; Li and Tumbar, 2021; Moreira and Marques, 2022; Wasko et al., 2022; Kam et al., 2023; Li et al., 2023).
Dermal lymphatic vessels are organized into two plexuses: a superficial plexus and a deeper subcutaneous plexus. The lymphatic capillary network in the upper dermis, also termed initial afferent lymphatics, connects to larger collecting vessels in the lower dermis and the subcutaneous tissue, facilitating fluid and immune transport (Skobe and Detmar, 2000). The initial afferent lymphatic capillaries also interconnect neighboring hair follicle stem cells across the skin, draining into larger collecting vessels (Figure 1A) (Gur-Cohen et al., 2019; Pena-Jimenez et al., 2019; Yoon et al., 2019). Traditionally viewed as passive conduits for fluid, immune cells, and pathogens, lymphatics also exhibit diverse origins and functions (Oliver et al., 2020; Petrova and Koh, 2020). Recent studies using mouse genetically modified models have highlighted their role in coordinating hair follicle regeneration and growth (Gur-Cohen et al., 2019; Pena-Jimenez et al., 2019; Yoon et al., 2019; Yoon and Detmar, 2022), opening potential avenues for translational research.
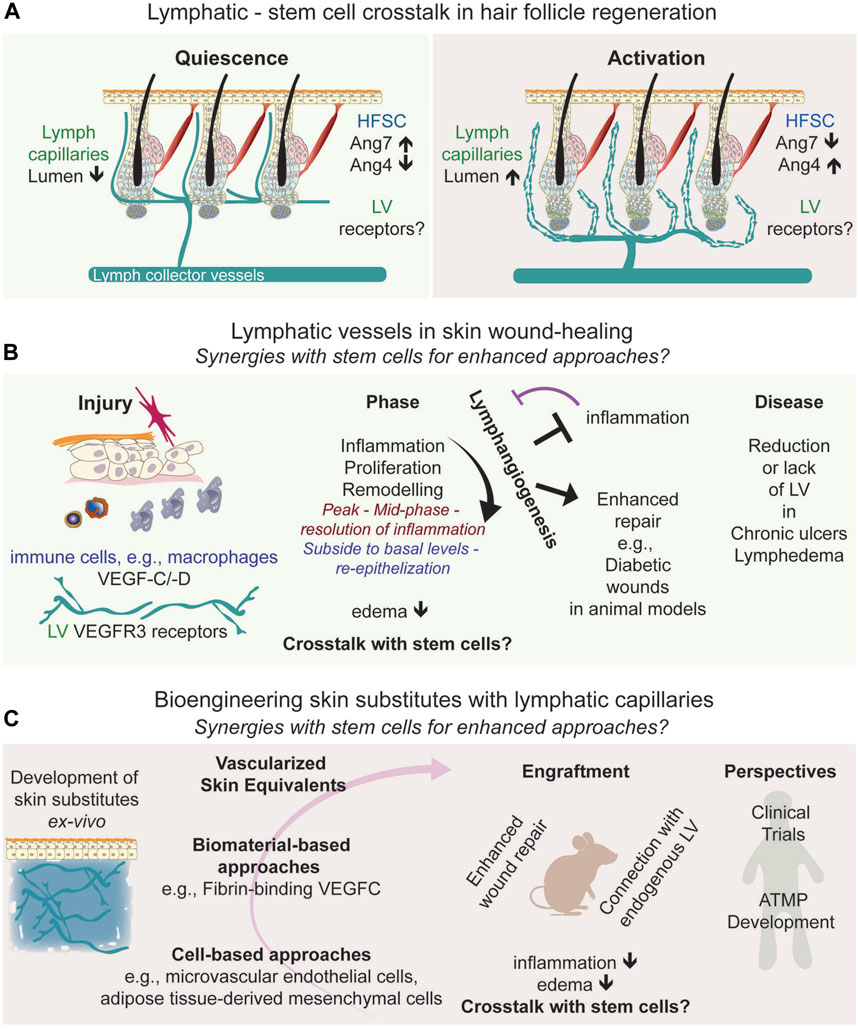
FIGURE 1. Frontiers in Skin Regeneration: Lymphatic Vessels and Stem Cell Interactions. (A) Connections of lymphatic vessels with hair follicle stem cells relevant for skin regeneration, depicting the interactions with bulge stem cells, and the signaling molecules associated to the synergic interactions. (B) Lymphangiogenesis in wound healing, (C) represents advanced skin grafting techniques, symbolizing the progression into translational approaches, and the integration of lymphatics in regenerative medicine, emphasizing their growing importance in therapeutic approaches and forecasts the future potential of these developments in treating chronic wounds, pointing towards new frontiers in medical research and treatment. Abbreviations: HFSC, Hair follicle stem cell; LV, lymphatic vessels; Ang, angiopoietin; VEGFC/D; Vascular endothelial growth factor C/D; ATMP; Advanced Therapeutic Medical Products.
Stem cell and lymphatic vessels crosstalk in hair follicle cycle control
The epidermis, interspersed with sweat glands and hair follicles, contains distinct stem cell populations identified in both mouse and human skin (Lu and Fuchs, 2014; Watt, 2014; Reynolds et al., 2021; Negri and Watt, 2022; Almet et al., 2023; Negri et al., 2023). Hair follicle stem cells, particularly in the bulge region near the sebaceous gland, are well-studied for their regenerative properties, defined in mouse (Cotsarelis et al., 1990; Blanpain et al., 2004; Tumbar et al., 2004) and human skin (Purba et al., 2014; Reynolds et al., 2021; Negri and Watt, 2022; Almet et al., 2023). These multipotent stem cells play a crucial role in the hair follicle cycle, comprising phases of rest (telogen), growth (anagen), and decay (catagen) (Muller-Rover et al., 2001). Studies in mice have revealed that bulge stem cells and progenitor hair germ cells (Greco et al., 2009) have significant regenerative potential, interacting with the dermal papilla (Lei et al., 2017). Their activation initiates the transition from telogen to anagen, leading to the differentiation of transit-amplifying cells into various lineages. Understanding and harnessing the regenerative capacities of bulge stem cells are crucial for promoting endogenous regeneration and advancing stem cell-based therapies. Hair follicle regeneration involves a complex interplay of intrinsic and extrinsic mechanisms (Li and Tumbar, 2021; Hsu and Fuchs, 2022; Zhang and Chen, 2023). The hair follicle’s microenvironment, including its vasculature, significantly influences bulge stem cells (Li and Tumbar, 2021; Yue et al., 2022; Zhang and Chen, 2023). The association of the upper bulge with a perivascular niche is instrumental in sustaining hair follicle stem cells (Xiao et al., 2013), and a population of multipotent stem cells in the bulge can even differentiate into blood vessels (Amoh et al., 2004). However, recent studies have illuminated the role of lymphatic vessels in regulating mouse hair follicle regeneration, expanding their role beyond traditional functions (Gur-Cohen et al., 2019; Pena-Jimenez et al., 2019; Yoon et al., 2019; Yoon and Detmar, 2022). This includes lymphatic vessels responding to Wnt secretion to associate with the stem cell niche and the stem cell secretion of angiopoietin molecules, affecting lymphatic tissue drainage linked to hair follicle regeneration (Figure 1A). Identifying the receptors on lymphatic vessels that lead to changes in tissue drainage will provide further insight into their direct or indirect signaling crosstalk in the skin. The genetic ablation of lymphatic vessels perturbs the hair follicle cycle, potentially due to impairing the concentration gradient of regulatory factors across the skin, and the influx and outflow of immune cells, such as macrophages (Castellana et al., 2014; Wang et al., 2019) and T regulatory cells (Ali et al., 2017; Liu et al., 2022), known to regulate hair follicle regeneration.
Overall, the skin’s microenvironment, heavily influenced by its blood and lymphatic vasculature, is critical in regulating and maintaining hair follicle stem cells. Recent sequencing studies have identified unique factors for lymph vessels compared to blood vessels (Chovatiya et al., 2023). This emerging knowledge holds the potential for exploring the distinct roles of lymphatics-stem cell crosstalk in skin regeneration and their implications in dermatological diseases, including skin cancer (Cazzola et al., 2023) and aging (Kataru et al., 2022), opening new avenues for potential translational studies in skin regeneration.
Blood vasculature and epidermal stem cells: paving the way for understanding lymphatic involvement?
In the stratified epidermis, maintaining the balance between cell proliferation and terminal differentiation is crucial for the continuous renewal of skin cells and enhancing regenerative capabilities. Basal progenitor cells can undergo symmetric divisions to preserve the skin surface area or differentiate into the suprabasal spinous, granular, and corneal layers (Jones et al., 2007; Blanpain and Fuchs, 2009; Simpson et al., 2011; Gandarillas et al., 2018; Cockburn et al., 2022; Prado-Mantilla and Lechler, 2023).
Interestingly, the blood vasculature influences epidermal stem cell behavior, while the role of lymphathic vessels remains poorly understood. Some insights gained from the blood vasculature regarding sustaining skin homeostasis could potentially pave the way for exploring the complementary roles of lymphatic vessels in epidermal stem cell proliferation.
In mouse skin, two distinct basal progenitor stem cell territories exist (Mascre et al., 2012; Gomez et al., 2013; Roy et al., 2016; Sada et al., 2016), with conserved expression patterns with human skin (Ghuwalewala et al., 2022). The mouse inter-scales, equivalent to human inter-ridges, express the Sox6 gene, forming UV-responsive proliferating clusters. Conversely, mouse scales, with non-label retaining properties, equivalent to human rete ridges are enriched with specific gene signatures like Slc1a3, showing resilience to UV stress (Ghuwalewala et al., 2022). Interestingly, mouse scales, analogous to human rete ridges, are located near dense blood vessel arrays, suggesting significant stem cell-blood vessel interactions (Sada et al., 2016).
Mechanical stretch has been shown to induce skin stem cell renewal (Aragona et al., 2020), and blood vessels in abdominal skin promote the formation of epidermal proliferating clusters, which are enhanced by mechanical stretch (Ichijo et al., 2021). In contrast, blood vessel atrophy during aging leads to dermal stiffening and differentiation of basal progenitor stem cells (Ichijo et al., 2022).
These findings underscore the coordinated interaction between epidermal stem cells and blood vasculature in maintaining skin homeostasis and responding to stresses like UV exposure and mechanical stretch.
The role of lymphatic vessels in these processes remains unexplored. The distinct LV territorial distribution in humans and other species (Suami et al., 2007; Soto-Miranda et al., 2013; Suami et al., 2013; Ito and Suami, 2015; Suami, 2017; Suami and Scaglioni, 2017) raises the plausible speculation that lymphatic vessels, like their blood vessel counterparts, may exert similar influences on different basal progenitor cell territories and play a significant role in epidermal regeneration. Such a hypothesis presents a compelling direction for future research to explore the influence of local microenvironments (Lawlor and Kaur, 2015; Roy et al., 2016; Cheng et al., 2018) and different stem cell population responses to mechanical and environmental stress, including mechanical stretch and wound healing (Aragona et al., 2017; Park et al., 2017; Aragona et al., 2020; Gola and Fuchs, 2021; Ghuwalewala et al., 2022).
Lymphatic vessels in skin wound healing: new avenues to explore the synergies with stem cells?
In the multifaceted process of skin wound healing, the re-epithelialized wound epidermis consists of progeny from both epidermal and hair follicle stem cells, as well as de-differentiated cells (Blanpain et al., 2004; Tumbar et al., 2004; Donati et al., 2017; Sun et al., 2023) that repopulate the tissue to achieve wound repair.
During wound healing, lymphangiogenesis, the formation of new lymphatic vessels, is also vital for restoring skin and vascular functionality. Lymphatic vessels are crucial in reducing interstitial pressure and edema by facilitating fluid drainage. They also play a significant role in regulating immune cell transport and responses, positioning them as key therapeutic targets in skin regeneration (Reno and Sabbatini, 2023). Investigating the potential functional relationship between lymphatic vessels and skin stem cells during wound healing could yield further insights into lymphatic-mediated wound repair mechanisms. It is established that lymphangiogenesis and angiogenesis concurrently increase during wound repair (Tonnesen et al., 2000; Cho et al., 2006; Johnson and Wilgus, 2014; Wasko et al., 2022). In the case of lymphangiogenesis, wound-induced inflammation or infections trigger cytokine secretion and the recruitment of innate immune cells, and the secretion of cytokines and the lymphangiogenic factors VEGF-C/-D that bind to VEGF-R3 receptors on lymphatic endothelial cells (Paavonen et al., 2000; Kataru et al., 2009) (Figure 1B). Lymphangiogenesis peaks in the mid-phase to the resolution of inflammation and subsides to basal levels upon completion of re-epithelization (Martinez-Corral et al., 2012) (Figure 1B). Yet, the specific contributions of lymphatic vessels and their molecular signals in each phase of wound healing remain to be fully elucidated.
Recent findings highlight a strong correlation between the inflammatory response and lymphangiogenesis. Anti-inflammatory treatments block lymphangiogenesis (Martinez-Corral et al., 2012) while promoting lymphangiogenesis counteracts inflammation (Huggenberger et al., 2010; Huggenberger et al., 2011). Additionally, the loss of lymphatic vessels can induce a proinflammatory environment, delaying wound closure (Brunner et al., 2023). This suggests a dynamic and reciprocal relationship between lymphatic function and the inflammatory process in wound healing (Figure 1B).
Examining the intricacies of lymphatic crosstalk with other microenvironmental cells, such as macrophages, could be particularly revealing to provide insight into the complex interactions in skin repair connecting stem cells. Macrophages are crucial in wound-induced de novo hair follicle stem cell regeneration (Chen et al., 2015; Wang et al., 2017; Rahmani et al., 2018) and are key regulators of lymphangiogenesis during wound healing (Paavonen et al., 2000; Kataru et al., 2009; Wiig et al., 2013; Hadrian et al., 2021). Understanding the spatio-temporal dynamics between these populations could deepen our knowledge in the context of wound-induced hair regeneration and offer translational implications.
While much of the research mentioned above is based on studies with mice and genetically modified mouse models, these have been crucial in uncovering the role of lymphatic vessels in wound healing. This research opens new possibilities for treating chronic wounds, where normal healing processes are often disrupted. In human skin, chronic ulcers lack lymphatic vessels (Brunner et al., 2023). Mathematical modeling of lymphangiogenesis in diabetic human wounds suggests potential applications for treating such conditions (Bianchi et al., 2015). Additionally, single-cell transcriptomic analyses of human diabetic foot wounds have revealed significant alterations in various signaling pathways, including those related to lymphatic vessels (Sandoval-Schaefer et al., 2023), underscoring the importance of exploring the mechanisms regulating lymphangiogenesis for the treatment of diabetic wounds. In mouse models, a clear link has been established between impaired diabetic wound healing and hindered lymphatic vessel formation due to reduced macrophage activity (Maruyama et al., 2007). Conversely, diabetic wound healing improves when lymphangiogenesis is stimulated (Figure 1B), either through pharmacological means or by using lymphangiogenic factors (Reno and Sabbatini, 2023), such as COMP-Angiopoietin1 (Cho et al., 2006) and VEGF-C (Saaristo et al., 2006; Guc et al., 2017; Lim et al., 2019; Brunner et al., 2023).
In conclusion, the role of lymphatic vessels in skin wound healing is multifaceted and crucial. They are involved in fluid regulation, waste removal, and the modulation of immune responses. While our understanding of their functions in human wound healing is still developing, investigating the spatiotemporal roles of lymphatics in various stages of wound repair, along with their interactions with bulge stem cells, represents a promising and complex area in regenerative medicine to enhance wound repair. This is particularly relevant for chronic wounds and vascular diseases like lymphedema. As research progresses, these insights have the potential to lead to innovative therapeutic strategies, enhancing wound healing and tissue regeneration.
Merging skin grafts and bioengineering perspectives with lymphatic insights: facilitating synergies with stem cells for enhanced approaches?
Skin transplantation and bioengineering approaches have evolved significantly, transitioning from traditional grafting techniques to sophisticated bioengineering methods for developing skin substitutes to treat extensive skin defects. This shift, which began with the clinical use of lab-grown keratinocytes in the 1980s (Watt, 2014), has led to remarkable progress in cellular and tissue-engineered therapies for skin regeneration (De Rosa and De Luca, 2022).
The integration of advanced materials and technologies, including in situ 3D printing, portable bioprinters, and electrosprayers, into cellular therapies is revolutionizing wound healing and skin regeneration (Chouhan et al., 2019). Despite these advancements, the challenge remains to create a skin substitute that fully replicates all the biological characteristics of native skin.
In light of the lymphatic insights discussed earlier, it is evident that fostering synergies with stem cells represents a promising avenue for enhancing approaches in skin grafts and bioengineering perspectives. Recent tissue engineering strategies are increasingly focusing on vascularized skin equivalents, and efforts to promote neotissue vascularization during tissue regeneration are employing innovative biomaterial-based and cell-based approaches (Oualla-Bachiri et al., 2020; Moreira and Marques, 2022).
Due to the significant roles of lymphatic vasculature in skin repairs, their potential to enhance the success of skin grafts and dermal substitutes has recently garnered essential interest (Figure 1C). Engineered fibrin-binding VEGF-C, for instance, has been shown to promote wound healing by increasing immune cell trafficking and matrix remodeling (Guc et al., 2017). A pivotal advancement in skin bioengineering was incorporating microvascular endothelial cells, encompassing both blood and lymphatic endothelial cells, into grafts. This innovative approach created dermo-epidermal skin grafts with integrated lymphatic vessels, which successfully vascularized ex vivo. When transplanted onto wounds in nude rats, these grafts connected with existing lymphatic capillaries, significantly enhancing fluid drainage (Marino et al., 2014). This breakthrough represents a major advancement in developing grafts that closely replicate the natural functionality of the skin. The technique led to the creation of full-thickness skin analogs that mimic the physiological, structural, and functional properties of native skin. These developments address challenges like rapid graft acceptance and effective nutrient and oxygen delivery through pre-established capillary networks.
Furthermore, additional approaches involving adipose tissue-derived isolates has been shown to enhance lymphatic drainage and immune cell trafficking in dermal substitutes. This method stimulates the integration of implants onto mouse wounds, thereby enhancing microvascular network formation and skin regeneration (Frueh et al., 2017; Asano et al., 2023).
Discussion
The facilitation of lymphangiogenesis in skin wound healing presents a multifaceted approach to medical intervention, particularly in reducing edema and promoting debris removal. However, the broader implications of lymphatic vessels, especially their contribution to stem cell activation during the repair process, warrant further exploration. This exploration becomes even more compelling when considering the dynamic interaction of lymphatic vessels with immune cells in the transplanted wound microenvironment. Macrophages, for instance, could significantly enhance the integration process of transplanted tissues, thereby improving the outcomes of skin transplantation and wound repair.
The integration of fundamental knowledge from skin cell and molecular biology, combined with insights into stem cell and lymphatic vessel physiology, is notably advancing the field of regenerative medicine. This synergy is expanding the scope of therapeutic possibilities and offering novel solutions for chronic wounds and skin diseases. Looking ahead, the potential of lab-made grafts and Advanced Therapeutic Medical Products, currently in clinical trials, could be further augmented by incorporating lymphatic vessels. This strategic inclusion aims to replicate the native skin’s functionality more accurately and could improve the efficacy of skin transplantation and regenerative therapies.
As this field evolves, a deeper understanding of the interplay between lymphatic networks, stem cells, and immune responses in wound healing will be crucial. Such knowledge could pave the way for innovative treatments tailored to individual healing processes and specific wound types, especially in chronic wounds where traditional approaches have limited efficacy.
Author contributions
YJ: Writing–original draft, Writing–review and editing. MP-M: Funding acquisition, Writing–original draft, Writing–review and editing, Conceptualization.
Funding
The authors declare financial support was received for the research, authorship, and/or publication of this article. The Perez-Moreno lab received funding by Kræftens Bekæmpelse (A13956); Carlsbergfondet (CF21-0576); Novo Nordisk Fonden (NNF17OC0028028); Candys Foundation (2019-334-14024-1), Toyota-Fonden (KJ/BG-9867F), NEYE-Fonden, and Tømmerhandler Vilhelm Bangs-Fonden.
Acknowledgments
We thank past and present team members of the Perez-Moreno lab and the scientific community for their contributions to this exciting field.
Conflict of interest
The authors declare that the research was conducted in the absence of any commercial or financial relationships that could be construed as a potential conflict of interest.
Publisher’s note
All claims expressed in this article are solely those of the authors and do not necessarily represent those of their affiliated organizations, or those of the publisher, the editors and the reviewers. Any product that may be evaluated in this article, or claim that may be made by its manufacturer, is not guaranteed or endorsed by the publisher.
References
Ali N., Zirak B., Rodriguez R. S., Pauli M. L., Truong H. A., Lai K., et al. (2017). Regulatory T cells in skin facilitate epithelial stem cell differentiation. Cell 169, 1119–1129. doi:10.1016/j.cell.2017.05.002
Almet A. A., Yuan H., Annusver K., Ramos R., Liu Y., Wiedemann J., et al. (2023). A roadmap for a consensus human skin cell atlas and single-cell data standardization. J. Invest. Dermatol 143, 1667–1677. doi:10.1016/j.jid.2023.03.1679
Amoh Y., Li L., Yang M., Moossa A. R., Katsuoka K., Penman S., et al. (2004). Nascent blood vessels in the skin arise from nestin-expressing hair-follicle cells. Proc. Natl. Acad. Sci. U. S. A. 101, 13291–13295. doi:10.1073/pnas.0405250101
Aragona M., Dekoninck S., Rulands S., Lenglez S., Mascre G., Simons B. D., et al. (2017). Defining stem cell dynamics and migration during wound healing in mouse skin epidermis. Nat. Commun. 8, 14684. doi:10.1038/ncomms14684
Aragona M., Sifrim A., Malfait M., Song Y., Van Herck J., Dekoninck S., et al. (2020). Mechanisms of stretch-mediated skin expansion at single-cell resolution. Nature 584, 268–273. doi:10.1038/s41586-020-2555-7
Asano Y., Shimoda H., Okano D., Matsusaki M., Akashi M. (2023). Lymphatic drainage-promoting effects by engraftment of artificial lymphatic vascular tissue based on human adipose tissue-derived mesenchymal stromal cells in mice. J. Tissue Eng. Regen. Med. 2023, 1–15. doi:10.1155/2023/7626767
Bianchi A., Painter K. J., Sherratt J. A. (2015). A mathematical model for lymphangiogenesis in normal and diabetic wounds. J. Theor. Biol. 383, 61–86. doi:10.1016/j.jtbi.2015.07.023
Blanpain C., Fuchs E. (2009). Epidermal homeostasis: a balancing act of stem cells in the skin. Nat. Rev. Mol. Cell Biol. 10, 207–217. doi:10.1038/nrm2636
Blanpain C., Lowry W. E., Geoghegan A., Polak L., Fuchs E. (2004). Self-renewal, multipotency, and the existence of two cell populations within an epithelial stem cell niche. Cell 118, 635–648. doi:10.1016/j.cell.2004.08.012
Brunner L. M., He Y., Cousin N., Scholl J., Albin L. K., Schmucki B., et al. (2023). Promotion of lymphangiogenesis by targeted delivery of VEGF-C improves diabetic wound healing. Cells 12, 472. doi:10.3390/cells12030472
Castellana D., Paus R., Perez-Moreno M. (2014). Macrophages contribute to the cyclic activation of adult hair follicle stem cells. PLoS Biol. 12, e1002002. doi:10.1371/journal.pbio.1002002
Cazzola A., Calzon Lozano D., Menne D. H., Davila Pedrera R., Liu J., Pena-Jimenez D., et al. (2023). Lymph vessels associate with cancer stem cells from initiation to malignant stages of squamous cell carcinoma. Int. J. Mol. Sci. 24, 13615. doi:10.3390/ijms241713615
Chen C. C., Wang L., Plikus M. V., Jiang T. X., Murray P. J., Ramos R., et al. (2015). Organ-level quorum sensing directs regeneration in hair stem cell populations. Cell 161, 277–290. doi:10.1016/j.cell.2015.02.016
Cheng J. B., Sedgewick A. J., Finnegan A. I., Harirchian P., Lee J., Kwon S., et al. (2018). Transcriptional programming of normal and inflamed human epidermis at single-cell resolution. Cell Rep. 25, 871–883. doi:10.1016/j.celrep.2018.09.006
Cho C. H., Sung H. K., Kim K. T., Cheon H. G., Oh G. T., Hong H. J., et al. (2006). COMP-angiopoietin-1 promotes wound healing through enhanced angiogenesis, lymphangiogenesis, and blood flow in a diabetic mouse model. Proc. Natl. Acad. Sci. U. S. A. 103, 4946–4951. doi:10.1073/pnas.0506352103
Chouhan D., Dey N., Bhardwaj N., Mandal B. B. (2019). Emerging and innovative approaches for wound healing and skin regeneration: current status and advances. Biomaterials 216, 119267. doi:10.1016/j.biomaterials.2019.119267
Chovatiya G., Li K. N., Ghuwalewala S., Tumbar T. (2023). Single-cell transcriptomics of adult skin VE-cadherin expressing lineages during hair cycle, bioRxiv, 2023.03.22.533784. doi:10.1101/2023.03.22.533784
Cockburn K., Annusver K., Gonzalez D. G., Ganesan S., May D. P., Mesa K. R., et al. (2022). Gradual differentiation uncoupled from cell cycle exit generates heterogeneity in the epidermal stem cell layer. Nat. Cell Biol. 24, 1692–1700. doi:10.1038/s41556-022-01021-8
Cotsarelis G., Sun T. T., Lavker R. M. (1990). Label-retaining cells reside in the bulge area of pilosebaceous unit: implications for follicular stem cells, hair cycle, and skin carcinogenesis. Cell 61, 1329–1337. doi:10.1016/0092-8674(90)90696-c
De Rosa L., De Luca M. (2022). The joint battle to tackle epidermolysis bullosa through gene therapy. Trends Mol. Med. 28, 533–535. doi:10.1016/j.molmed.2022.05.001
Donati G., Rognoni E., Hiratsuka T., Liakath-Ali K., Hoste E., Kar G., et al. (2017). Wounding induces dedifferentiation of epidermal Gata6(+) cells and acquisition of stem cell properties. Nat. Cell Biol. 19, 603–613. doi:10.1038/ncb3532
Driskell R. R., Jahoda C. A., Chuong C. M., Watt F. M., Horsley V. (2014). Defining dermal adipose tissue. Exp. Dermatol 23, 629–631. doi:10.1111/exd.12450
Frueh F. S., Spater T., Lindenblatt N., Calcagni M., Giovanoli P., Scheuer C., et al. (2017). Adipose tissue-derived microvascular fragments improve vascularization, lymphangiogenesis, and integration of dermal skin substitutes. J. Invest. Dermatol 137, 217–227. doi:10.1016/j.jid.2016.08.010
Gandarillas A., Molinuevo R., Sanz-Gomez N. (2018). Mammalian endoreplication emerges to reveal a potential developmental timer. Cell Death Differ. 25, 471–476. doi:10.1038/s41418-017-0040-0
Ghuwalewala S., Lee S. A., Jiang K., Baidya J., Chovatiya G., Kaur P., et al. (2022). Binary organization of epidermal basal domains highlights robustness to environmental exposure. EMBO J. 41, e110488. doi:10.15252/embj.2021110488
Glatte P., Buchmann S. J., Hijazi M. M., Illigens B. M., Siepmann T. (2019). Architecture of the cutaneous autonomic nervous system. Front. Neurol. 10, 970. doi:10.3389/fneur.2019.00970
Gola A., Fuchs E. (2021). Environmental control of lineage plasticity and stem cell memory. Curr. Opin. Cell Biol. 69, 88–95. doi:10.1016/j.ceb.2020.12.015
Gomez C., Chua W., Miremadi A., Quist S., Headon D. J., Watt F. M. (2013). The interfollicular epidermis of adult mouse tail comprises two distinct cell lineages that are differentially regulated by Wnt, Edaradd, and Lrig1. Stem Cell Rep. 1, 19–27. doi:10.1016/j.stemcr.2013.04.001
Greco V., Chen T., Rendl M., Schober M., Pasolli H. A., Stokes N., et al. (2009). A two-step mechanism for stem cell activation during hair regeneration. Cell Stem Cell 4, 155–169. doi:10.1016/j.stem.2008.12.009
Guc E., Briquez P. S., Foretay D., Fankhauser M. A., Hubbell J. A., Kilarski W. W., et al. (2017). Local induction of lymphangiogenesis with engineered fibrin-binding VEGF-C promotes wound healing by increasing immune cell trafficking and matrix remodeling. Biomaterials 131, 160–175. doi:10.1016/j.biomaterials.2017.03.033
Gur-Cohen S., Yang H., Baksh S. C., Miao Y., Levorse J., Kataru R. P., et al. (2019). Stem cell-driven lymphatic remodeling coordinates tissue regeneration. Science 366, 1218–1225. doi:10.1126/science.aay4509
Hadrian K., Willenborg S., Bock F., Cursiefen C., Eming S. A., Hos D. (2021). Macrophage-mediated tissue vascularization: similarities and differences between cornea and skin. Front. Immunol. 12, 667830. doi:10.3389/fimmu.2021.667830
Hsu Y. C., Fuchs E. (2022). Building and maintaining the skin. Cold Spring Harb. Perspect. Biol. 14, a040840. doi:10.1101/cshperspect.a040840
Huggenberger R., Siddiqui S. S., Brander D., Ullmann S., Zimmermann K., Antsiferova M., et al. (2011). An important role of lymphatic vessel activation in limiting acute inflammation. Blood 117, 4667–4678. doi:10.1182/blood-2010-10-316356
Huggenberger R., Ullmann S., Proulx S. T., Pytowski B., Alitalo K., Detmar M. (2010). Stimulation of lymphangiogenesis via VEGFR-3 inhibits chronic skin inflammation. J. Exp. Med. 207, 2255–2269. doi:10.1084/jem.20100559
Ichijo R., Kabata M., Kidoya H., Muramatsu F., Ishibashi R., Abe K., et al. (2021). Vasculature-driven stem cell population coordinates tissue scaling in dynamic organs. Sci. Adv. 7, eabd2575. doi:10.1126/sciadv.abd2575
Ichijo R., Maki K., Kabata M., Murata T., Nagasaka A., Ishihara S., et al. (2022). Vasculature atrophy causes a stiffened microenvironment that augments epidermal stem cell differentiation in aged skin. Nat. Aging 2, 592–600. doi:10.1038/s43587-022-00244-6
Ito R., Suami H. (2015). Lymphatic territories (lymphosomes) in swine: an animal model for future lymphatic research. Plast. Reconstr. Surg. 136, 297–304. doi:10.1097/PRS.0000000000001460
Johnson K. E., Wilgus T. A. (2014). Vascular endothelial growth factor and angiogenesis in the regulation of cutaneous wound repair. Adv. Wound Care (New Rochelle) 3, 647–661. doi:10.1089/wound.2013.0517
Jones P. H., Simons B. D., Watt F. M. (2007). Sic transit gloria: farewell to the epidermal transit amplifying cell? Cell Stem Cell 1, 371–381. doi:10.1016/j.stem.2007.09.014
Kam C. Y., Singh I. D., Gonzalez D. G., Matte-Martone C., Sola P., Solanas G., et al. (2023). Mechanisms of skin vascular maturation and maintenance captured by longitudinal imaging of live mice. Cell 186, 2345–2360. doi:10.1016/j.cell.2023.04.017
Kataru R. P., Jung K., Jang C., Yang H., Schwendener R. A., Baik J. E., et al. (2009). Critical role of CD11b+ macrophages and VEGF in inflammatory lymphangiogenesis, antigen clearance, and inflammation resolution. Blood 113, 5650–5659. doi:10.1182/blood-2008-09-176776
Kataru R. P., Park H. J., Shin J., Baik J. E., Sarker A., Brown S., et al. (2022). Structural and functional changes in aged skin lymphatic vessels. Front. Aging 3, 864860. doi:10.3389/fragi.2022.864860
Kobayashi T., Naik S., Nagao K. (2019). Choreographing immunity in the skin epithelial barrier. Immunity 50, 552–565. doi:10.1016/j.immuni.2019.02.023
Kupper T. S., Fuhlbrigge R. C. (2004). Immune surveillance in the skin: mechanisms and clinical consequences. Nat. Rev. Immunol. 4, 211–222. doi:10.1038/nri1310
Lawlor K. T., Kaur P. (2015). Dermal contributions to human interfollicular epidermal architecture and self-renewal. Int. J. Mol. Sci. 16, 28098–28107. doi:10.3390/ijms161226078
Lei M., Yang L., Chuong C. M. (2017). Getting to the core of the dermal papilla. J. Invest. Dermatol 137, 2250–2253. doi:10.1016/j.jid.2017.07.824
Li K. N., Chovatiya G., Ko D. Y., Sureshbabu S., Tumbar T. (2023). Blood endothelial ALK1-BMP4 signaling axis regulates adult hair follicle stem cell activation. EMBO J. 42, e112196. doi:10.15252/embj.2022112196
Li K. N., Jain P., He C. H., Eun F. C., Kang S., Tumbar T. (2019). Skin vasculature and hair follicle cross-talking associated with stem cell activation and tissue homeostasis. Elife 8, e45977. doi:10.7554/eLife.45977
Li K. N., Tumbar T. (2021). Hair follicle stem cells as a skin-organizing signaling center during adult homeostasis. EMBO J. 40, e107135. doi:10.15252/embj.2020107135
Lim L., Bui H., Farrelly O., Yang J., Li L., Enis D., et al. (2019). Hemostasis stimulates lymphangiogenesis through release and activation of VEGFC. Blood 134, 1764–1775. doi:10.1182/blood.2019001736
Liu Z., Hu X., Liang Y., Yu J., Li H., Shokhirev M. N., et al. (2022). Glucocorticoid signaling and regulatory T cells cooperate to maintain the hair-follicle stem-cell niche. Nat. Immunol. 23, 1086–1097. doi:10.1038/s41590-022-01244-9
Lu C., Fuchs E. (2014). Sweat gland progenitors in development, homeostasis, and wound repair. Cold Spring Harb. Perspect. Med. 4, a015222. doi:10.1101/cshperspect.a015222
Marino D., Luginbuhl J., Scola S., Meuli M., Reichmann E. (2014). Bioengineering dermo-epidermal skin grafts with blood and lymphatic capillaries. Sci. Transl. Med. 6, 221ra14. doi:10.1126/scitranslmed.3006894
Martinez-Corral I., Olmeda D., Dieguez-Hurtado R., Tammela T., Alitalo K., Ortega S. (2012). In vivo imaging of lymphatic vessels in development, wound healing, inflammation, and tumor metastasis. Proc. Natl. Acad. Sci. U. S. A. 109, 6223–6228. doi:10.1073/pnas.1115542109
Maruyama K., Asai J., Ii M., Thorne T., Losordo D. W., D'Amore P. A. (2007). Decreased macrophage number and activation lead to reduced lymphatic vessel formation and contribute to impaired diabetic wound healing. Am. J. Pathol. 170, 1178–1191. doi:10.2353/ajpath.2007.060018
Mascre G., Dekoninck S., Drogat B., Youssef K. K., Brohee S., Sotiropoulou P. A., et al. (2012). Distinct contribution of stem and progenitor cells to epidermal maintenance. Nature 489, 257–262. doi:10.1038/nature11393
Moreira H. R., Marques A. P. (2022). Vascularization in skin wound healing: where do we stand and where do we go? Curr. Opin. Biotechnol. 73, 253–262. doi:10.1016/j.copbio.2021.08.019
Muller-Rover S., Handjiski B., van der Veen C., Eichmuller S., Foitzik K., McKay I. A., et al. (2001). A comprehensive guide for the accurate classification of murine hair follicles in distinct hair cycle stages. J. Invest. Dermatol 117, 3–15. doi:10.1046/j.0022-202x.2001.01377.x
Negri V. A., Louis B., Zijl S., Ganier C., Philippeos C., Ali S., et al. (2023). Single-cell RNA sequencing of human epidermis identifies Lunatic fringe as a novel regulator of the stem cell compartment. Stem Cell Rep. 18, 2047–2055. doi:10.1016/j.stemcr.2023.09.007
Negri V. A., Watt F. M. (2022). Understanding human epidermal stem cells at single-cell resolution. J. Invest. Dermatol 142, 2061–2067. doi:10.1016/j.jid.2022.04.003
Oliver G., Kipnis J., Randolph G. J., Harvey N. L. (2020). The lymphatic vasculature in the 21(st) century: novel functional roles in homeostasis and disease. Cell 182, 270–296. doi:10.1016/j.cell.2020.06.039
Oualla-Bachiri W., Fernandez-Gonzalez A., Quinones-Vico M. I., Arias-Santiago S. (2020). From grafts to human bioengineered vascularized skin substitutes. Int. J. Mol. Sci. 21, 8197. doi:10.3390/ijms21218197
Owens D. M., Lumpkin E. A. (2014). Diversification and specialization of touch receptors in skin. Cold Spring Harb. Perspect. Med. 4, a013656. doi:10.1101/cshperspect.a013656
Paavonen K., Puolakkainen P., Jussila L., Jahkola T., Alitalo K. (2000). Vascular endothelial growth factor receptor-3 in lymphangiogenesis in wound healing. Am. J. Pathol. 156, 1499–1504. doi:10.1016/S0002-9440(10)65021-3
Park S., Gonzalez D. G., Guirao B., Boucher J. D., Cockburn K., Marsh E. D., et al. (2017). Tissue-scale coordination of cellular behaviour promotes epidermal wound repair in live mice. Nat. Cell Biol. 19, 155–163. doi:10.1038/ncb3472
Pena-Jimenez D., Fontenete S., Megias D., Fustero-Torre C., Grana-Castro O., Castellana D., et al. (2019). Lymphatic vessels interact dynamically with the hair follicle stem cell niche during skin regeneration in vivo. EMBO J. 38, e101688. doi:10.15252/embj.2019101688
Petrova T. V., Koh G. Y. (2020). Biological functions of lymphatic vessels. Science 369, eaax4063. doi:10.1126/science.aax4063
Plikus M. V., Wang X., Sinha S., Forte E., Thompson S. M., Herzog E. L., et al. (2021). Fibroblasts: origins, definitions, and functions in health and disease. Cell 184, 3852–3872. doi:10.1016/j.cell.2021.06.024
Prado-Mantilla A., Lechler T. (2023). Polarity in skin development and cancer. Curr. Top. Dev. Biol. 154, 317–336. doi:10.1016/bs.ctdb.2023.02.003
Prost-Squarcioni C., Fraitag S., Heller M., Boehm N. (2008). Functional histology of dermis. Ann. Dermatol Venereol. 135, 1S5–20. doi:10.1016/S0151-9638(08)70206-0
Purba T. S., Haslam I. S., Poblet E., Jimenez F., Gandarillas A., Izeta A., et al. (2014). Human epithelial hair follicle stem cells and their progeny: current state of knowledge, the widening gap in translational research and future challenges. Bioessays 36, 513–525. doi:10.1002/bies.201300166
Rahmani W., Liu Y., Rosin N. L., Kline A., Raharjo E., Yoon J., et al. (2018). Macrophages promote wound-induced hair follicle regeneration in a CX3CR1-and TGF-β1-dependent manner. J. Invest. Dermatol 138, 2111–2122. doi:10.1016/j.jid.2018.04.010
Reno F., Sabbatini M. (2023). Breaking a vicious circle: lymphangiogenesis as a new therapeutic target in wound healing. Biomedicines 11, 656. doi:10.3390/biomedicines11030656
Reynolds G., Vegh P., Fletcher J., Poyner E. F. M., Stephenson E., Goh I., et al. (2021). Developmental cell programs are co-opted in inflammatory skin disease. Science 371, eaba6500. doi:10.1126/science.aba6500
Roy E., Neufeld Z., Cerone L., Wong H. Y., Hodgson S., Livet J., et al. (2016). Bimodal behaviour of interfollicular epidermal progenitors regulated by hair follicle position and cycling. EMBO J. 35, 2658–2670. doi:10.15252/embj.201693806
Saaristo A., Tammela T., Farkkila A., Karkkainen M., Suominen E., Yla-Herttuala S., et al. (2006). Vascular endothelial growth factor-C accelerates diabetic wound healing. Am. J. Pathol. 169, 1080–1087. doi:10.2353/ajpath.2006.051251
Sada A., Jacob F., Leung E., Wang S., White B. S., Shalloway D., et al. (2016). Defining the cellular lineage hierarchy in the interfollicular epidermis of adult skin. Nat. Cell Biol. 18, 619–631. doi:10.1038/ncb3359
Sandoval-Schaefer T., Phan Q., Dash B. C., Prassinos A. J., Duan K., Gazes M. I., et al. (2023). Transcriptional heterogeneity in human diabetic foot wounds. bioRxiv, 2023.02.16.528839. doi:10.1101/2023.02.16.528839
Simpson C. L., Patel D. M., Green K. J. (2011). Deconstructing the skin: cytoarchitectural determinants of epidermal morphogenesis. Nat. Rev. Mol. Cell Biol. 12, 565–580. doi:10.1038/nrm3175
Skobe M., Detmar M. (2000). Structure, function, and molecular control of the skin lymphatic system. J. Investig. Dermatol Symp. Proc. 5, 14–19. doi:10.1046/j.1087-0024.2000.00001.x
Smith L. T., Holbrook K. A., Byers P. H. (1982). Structure of the dermal matrix during development and in the adult. J. Invest. Dermatol 79 (1), 93s–104s. doi:10.1111/1523-1747.ep12545877
Soto-Miranda M. A., Suami H., Chang D. W. (2013). Mapping superficial lymphatic territories in the rabbit. Anat. Rec. Hob. 296, 965–970. doi:10.1002/ar.22699
Suami H. (2017). Lymphosome concept: anatomical study of the lymphatic system. J. Surg. Oncol. 115, 13–17. doi:10.1002/jso.24332
Suami H., Scaglioni M. F. (2017). Lymphatic territories (lymphosomes) in the rat: an anatomical study for future lymphatic research. Plast. Reconstr. Surg. 140, 945–951. doi:10.1097/PRS.0000000000003776
Suami H., Taylor G. I., Pan W. R. (2007). The lymphatic territories of the upper limb: anatomical study and clinical implications. Plast. Reconstr. Surg. 119, 1813–1822. doi:10.1097/01.prs.0000246516.64780.61
Suami H., Yamashita S., Soto-Miranda M. A., Chang D. W. (2013). Lymphatic territories (lymphosomes) in a canine: an animal model for investigation of postoperative lymphatic alterations. PLoS One 8, e69222. doi:10.1371/journal.pone.0069222
Sun X., Joost S., Kasper M. (2023). Plasticity of epithelial cells during skin wound healing. Cold Spring Harb. Perspect. Biol. 15, a041232. doi:10.1101/cshperspect.a041232
Theocharidis G., Connelly J. T. (2019). Minor collagens of the skin with not so minor functions. J. Anat. 235, 418–429. doi:10.1111/joa.12584
Tonnesen M. G., Feng X., Clark R. A. (2000). Angiogenesis in wound healing. J. Investig. Dermatol Symp. Proc. 5, 40–46. doi:10.1046/j.1087-0024.2000.00014.x
Tumbar T., Guasch G., Greco V., Blanpain C., Lowry W. E., Rendl M., et al. (2004). Defining the epithelial stem cell niche in skin. Science 303, 359–363. doi:10.1126/science.1092436
Wang E. C. E., Dai Z., Ferrante A. W., Drake C. G., Christiano A. M. (2019). A subset of TREM2(+) dermal macrophages secretes oncostatin M to maintain hair follicle stem cell quiescence and inhibit hair growth. Cell Stem Cell 24, 654–669. doi:10.1016/j.stem.2019.01.011
Wang X., Chen H., Tian R., Zhang Y., Drutskaya M. S., Wang C., et al. (2017). Macrophages induce AKT/β-catenin-dependent Lgr5+ stem cell activation and hair follicle regeneration through TNF. Nat. Commun. 8, 14091. doi:10.1038/ncomms14091
Wasko R., Bridges K., Pannone R., Sidhu I., Xing Y., Naik S., et al. (2022). Langerhans cells are essential components of the angiogenic niche during murine skin repair. Dev. Cell 57, 2699–2713.e5. doi:10.1016/j.devcel.2022.11.012
Watt F. M. (2014). Mammalian skin cell biology: at the interface between laboratory and clinic. Science 346, 937–940. doi:10.1126/science.1253734
Wiig H., Schroder A., Neuhofer W., Jantsch J., Kopp C., Karlsen T. V., et al. (2013). Immune cells control skin lymphatic electrolyte homeostasis and blood pressure. J. Clin. Invest. 123, 2803–2815. doi:10.1172/JCI60113
Xiao Y., Woo W. M., Nagao K., Li W., Terunuma A., Mukouyama Y. S., et al. (2013). Perivascular hair follicle stem cells associate with a venule annulus. J. Invest. Dermatol 133, 2324–2331. doi:10.1038/jid.2013.167
Yoon S. Y., Detmar M. (2022). Sostdc1 secreted from cutaneous lymphatic vessels acts as a paracrine factor for hair follicle growth. Curr. Issues Mol. Biol. 44, 2167–2174. doi:10.3390/cimb44050146
Yoon S. Y., Dieterich L. C., Karaman S., Proulx S. T., Bachmann S. B., Sciaroni C., et al. (2019). An important role of cutaneous lymphatic vessels in coordinating and promoting anagen hair follicle growth. PLoS One 14, e0220341. doi:10.1371/journal.pone.0220341
Yue Z., Yang F., Zhang J., Li J., Chuong C. M. (2022). Regulation and dysregulation of hair regeneration: aiming for clinical application. Cell Regen. 11, 22. doi:10.1186/s13619-022-00122-x
Keywords: skin, hair follicle, stem cells, regeneration, microenvironment, lymphatics, engineered skin, skin graft
Citation: Jiang Y and Perez-Moreno M (2024) Translational frontiers: insight from lymphatics in skin regeneration. Front. Physiol. 15:1347558. doi: 10.3389/fphys.2024.1347558
Received: 01 December 2023; Accepted: 01 February 2024;
Published: 29 February 2024.
Edited by:
Alexandra P. Marques, University of Minho, PortugalReviewed by:
Sangbum Park, Michigan State University, United StatesAlex Wong, City of Hope National Medical Center, United States
Copyright © 2024 Jiang and Perez-Moreno. This is an open-access article distributed under the terms of the Creative Commons Attribution License (CC BY). The use, distribution or reproduction in other forums is permitted, provided the original author(s) and the copyright owner(s) are credited and that the original publication in this journal is cited, in accordance with accepted academic practice. No use, distribution or reproduction is permitted which does not comply with these terms.
*Correspondence: Mirna Perez-Moreno, mirna.pmoreno@bio.ku.dk