- 1Neuroscience Research Department, Research Centre, King Fahad Medical City, Riyadh, Saudi Arabia
- 2BrainExperiments.com, Montreal, QC, Canada
- 3Department of Biomedical and Pharmaceutical Sciences, Chapman University School of Pharmacy, Irvine, CA, United States
Numerous neurodegenerative diseases result from altered ion channel function and mutations. The intracellular redox status can significantly alter the gating characteristics of ion channels. Abundant neurodegenerative diseases associated with oxidative stress have been documented, including Parkinson’s, Alzheimer’s, spinocerebellar ataxia, amyotrophic lateral sclerosis, and Huntington’s disease. Reactive oxygen and nitrogen species compounds trigger posttranslational alterations that target specific sites within the subunits responsible for channel assembly. These alterations include the adjustment of cysteine residues through redox reactions induced by reactive oxygen species (ROS), nitration, and S-nitrosylation assisted by nitric oxide of tyrosine residues through peroxynitrite. Several ion channels have been directly investigated for their functional responses to oxidizing agents and oxidative stress. This review primarily explores the relationship and potential links between oxidative stress and ion channels in neurodegenerative conditions, such as cerebellar ataxias and Parkinson’s disease. The potential correlation between oxidative stress and ion channels could hold promise for developing innovative therapies for common neurodegenerative diseases.
1 Introduction
Reactive oxygen species (ROS) are generated by living organisms as a result of their regular cellular metabolic processes and environmental factors, such as smoking, air pollutants, UV radiation, alcohol consumption, infections, non-steroidal anti-inflammatory drugs (NSAIDs), and inflammation (Valko et al., 2006; Birben et al., 2012). ROS are required in small to moderate amounts for normal cellular functions. However, elevated concentrations induce detrimental alterations to proteins, DNA, and lipids, which hinder cell function (Liguori et al., 2018; Uttara et al., 2009). The lack of antioxidants generates oxidative stress that increases reactive species’ levels (Uttara et al., 2009). Pathological states often result in intracellular oxidative agents overtaking reducing agents, causing redox imbalances and oxidative stress (Simon et al., 2013; Sies et al., 2017). Numerous oxidative stress-related diseases have been reported (Sies et al., 2017; Ramírez et al., 2016), such as neurodegenerative disorders involving Parkinson’s (Henchcliffe and Beal, 2008), Alzheimer’s (Gella and Durany, 2009; Chang et al., 2014), spinocerebellar ataxia (Guevara-García et al., 2012), Huntington’s disease (Browne et al., 1999), and amyotrophic lateral sclerosis (ALS) (Cunha-Oliveira et al., 2020). Several cardiovascular diseases are also linked with oxidative stress, such as hypertension (Griendling et al., 2021), heart failure (Pagan et al., 2022), myocardial ischemia (Kurian et al., 2016), and atherosclerosis (Kattoor et al., 2017). Other pathologies linked to oxidative stress involve obesity (Manna and Jain, 2015), chronic inflammation (Orzechowski et al., 2019), and chronic pain (Kaushik et al., 2020). The literature is rich in presenting compelling evidence of a significant association between neurodegenerative disorders, aging, and oxidative stress (Browne et al., 1999; Gella and Durany, 2009; Uttara et al., 2009; Riverón et al., 2010; Chang et al., 2014; Cunha-Oliveira et al., 2020). Oxidative stress causes neuroinflammation, and mitochondrial dysfunction leads to apoptosis and cell damage that triggers neurodegenerative processes (Figure 1) (Selivanov et al., 2011; Ashok et al., 2022). Several neuroprotective therapies have been developed to combat ROS that damage neurons and cause neurodegenerative disorders (Uttara et al., 2009). The intracellular redox status can significantly alter the gating properties of ion channels (Akbarali, 2014). Indeed, various neurodegenerative diseases result from altered ion channel function and mutations (Li and Lester, 2001).
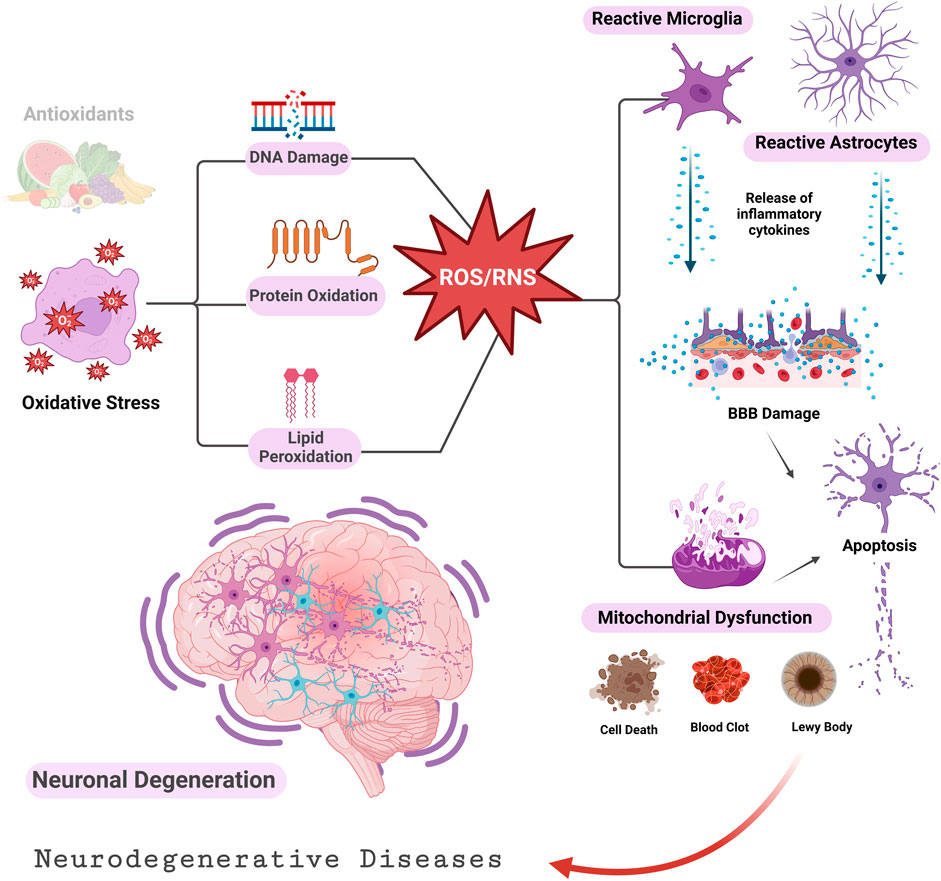
FIGURE 1. Oxidative stress and neurodegenerative diseases (Ashok et al., 2022). An imbalance between ROS/RNS and antioxidants damages lipids, proteins, and DNA. Cellular apoptosis and tissue death are promoted by impaired mitochondrial function and buildup of activated astrocytes and microglia. BBB, Blood Brain Barrier.
This review is an effort to summarize some of the common modifications in ion channel regulations by ROS in some neurodegenerative disease states.
2 Oxidative stress and ion channels
Oxidative stress passively damages proteins, lipids, and DNA but also directly modulates many molecules in the cell signaling network, such as ion channels. The ion channel is a macromolecular pore in cell membranes that selectively conducts Na+, K+, Ca2+, and Cl–ions. These pores are essential in conducting the ions across cell membranes (Litalien et al., 2011). Different stimuli open ion channels and conduct ions into or out of the cells, including changes in membrane potential, chemical stimuli, or mechanical deformation (Li and Lester, 2001). According to the stimulus they respond to, ion channels can be classified into three superfamilies: voltage-gated (Purves et al., 2001a), ligand-gated (Purves et al., 2001b), or mechanosensitive (Martinac, 2012). Ion channel subtypes are differentiated by their primary structure, distribution, and functional properties (Zheng and Trudeau, 2023). In voltage-gated ion channels, the membrane potential changes, and a specific ion is selectively dissolved; these channels can be categorized into different families based on the ion specificity (Purves et al., 2001a). Neurotransmitters or other ligands can trigger ligand-gated ion channels. There are several subtypes of ligand-gated channels, just like voltage-gated channels. Mechanosensitive ion channels respond to alterations in mechanical forces on the cell membrane (Zheng and Trudeau, 2023). Ion channels play a fundamental role in nerve conduction, neural communication, and muscle contraction, in addition to their conical function of transporting ions across the cell membrane to set membrane potential (Rosendo-Pineda et al., 2020) (Table 1) (Figure 2). Detailed information on recent ion channel types can be found in the excellent textbook by Zheng and Trudeau et al. (Zheng and Trudeau, 2023). There are also multiple reviews on specific types of ion channels (Purves et al., 2001b; Shah and Aizenman, 2014; de Lera Ruiz and Kraus, 2015; Nam et al., 2023a).
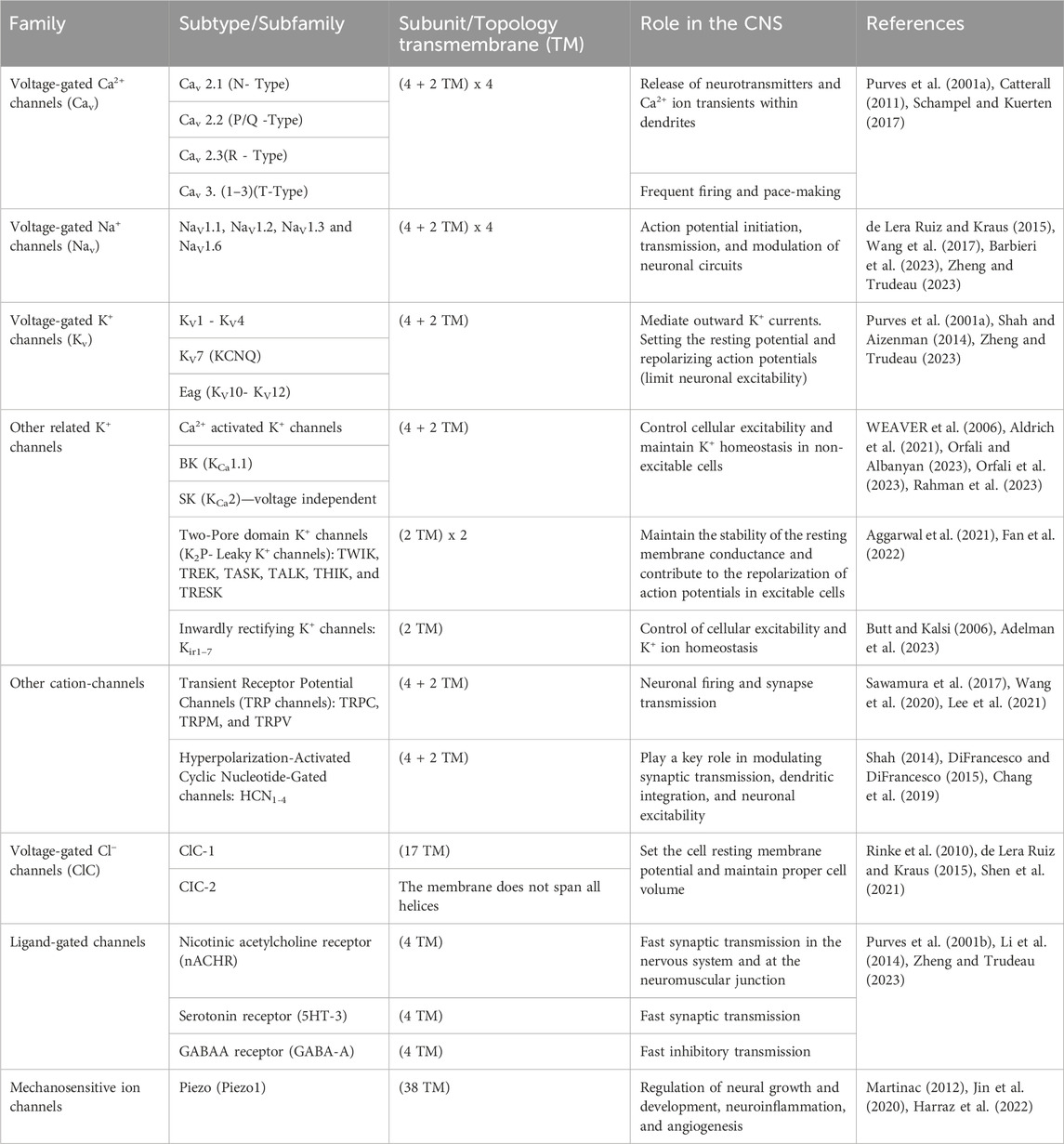
TABLE 1. A Summary of different ion channel types and their role in the CNS (Zheng and Trudeau, 2023).
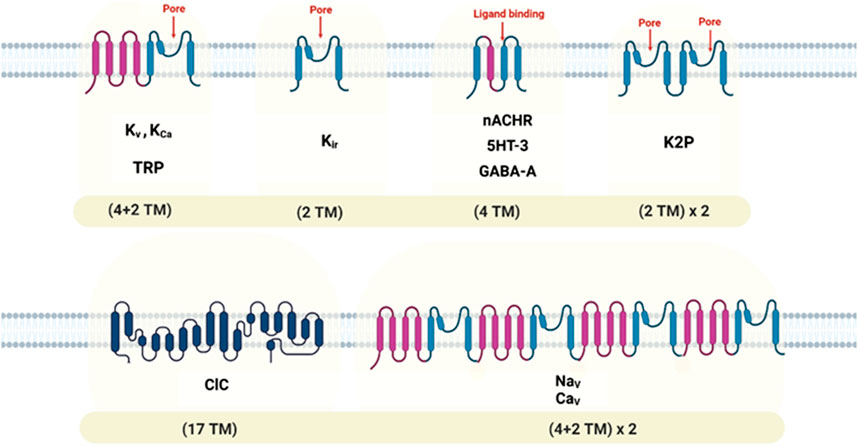
FIGURE 2. Topology diagrams of some ion channel families, showing locations of transmembrane domains and pore-forming segments. Transmembrane, (TM).
Posttranslational oxidative modifications of certain proteins, such as ion channels, can result from imbalances in cellular redox state caused by ROS production, ineffective antioxidant defenses, or environmental oxidative stress. (Bogeski and Niemeyer, 2014; Kiselyov and Muallem, 2016). In ion channels and other proteins, cysteine residues are the most vulnerable to oxidation due to their highly reactive thiol groups. It is possible to oxidize thiols into sulfonic acids and sulfonic based on the amount of oxidant present, the redox potential, the amount of charge, and the temperature. Various oxidative modifications can be applied to cysteines, including processes like glutathionylation and nitrosylation. Elevated levels of ROS can lead to the decomposition of amino acids, such as lysine and arginine, into aldehydes or the conversion of methionine residues into sulfoxides and sulfones (Bogeski and Niemeyer, 2014). Reactive nitrogen species (RNS) and ROS have the potential to directly alter ion channels by nitrosylation, nitration, and oxidation of specific amino acid residues. This can eventually affect the signaling pathways that modulate channel function, modifying gene transcription, turnover, proteasomal degradation, and trafficking (Akbarali, 2014). Sensitivity to alterations in the side chains of the amino acid residues that serve as the targets for ROS/RNS is typically associated with the presence of sulfur atoms in (methionine and cysteine), aromatic rings (tryptophan, histidine, and phenylalanine), or hydroxyl groups (tyrosine), (Annunziato et al., 2002; Akbarali, 2014; Miranda et al., 2023). In biological systems, there is a physiological balance between the generation of ROS and their detoxification through antioxidant scavengers, such as glutathione, catalase, and superoxide dismutase. When there is an imbalance, oxidative stress occurs (Gulcin, 2020).
Many types of ion channels are recognized to be modulated by oxidative stress. This modulation can be beneficial in some, while in others, it leads to pathological states (Figure 3) (Akbarali, 2014). For example, alterations in the gating properties and ion selectivity of voltage-gated ion channels may occur. Oxidative stress can also affect ligand-gated ion channels, thereby altering their signaling pathways and sensitivity (Miranda et al., 2023). Another consequence of oxidative stress on regulating intracellular Ca2+ levels is its ability to alter the function of Ca2+ release channels within the endoplasmic reticulum and Ca2+ uptake channels in the cell’s plasma membrane (Santulli et al., 2017). Ion channels might also function as sensors of redox changes, given that various ion channels are closely linked to oxidative stress. Also, ROS-induced damage can be restored through natural protective mechanisms. Consequently, for therapeutic purposes, understanding how these reactants affect ion channel functionality is essential to understanding how oxidative stress-related diseases are triggered.
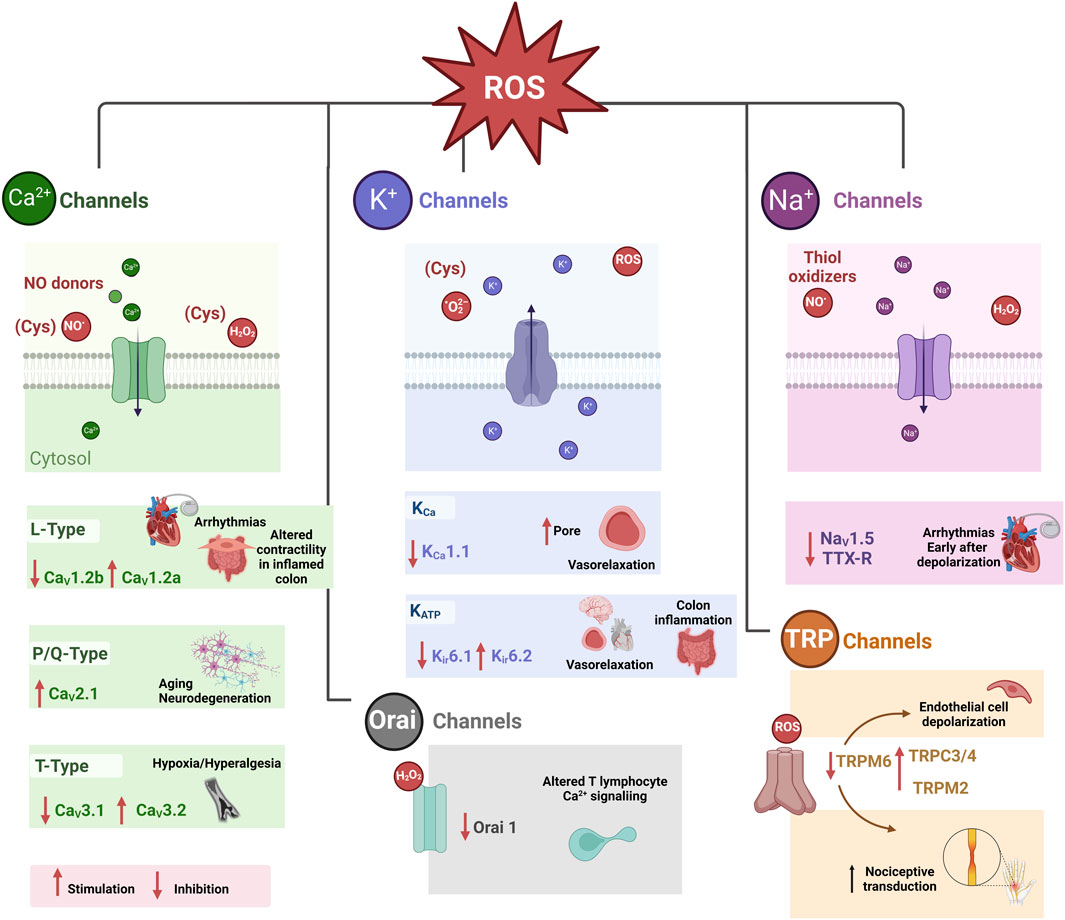
FIGURE 3. Pathophysiological consequences of redox modulation of some ion channels (Akbarali, 2014). Cysteine (CyS), Store-operated Ca2+ release-activated Ca2+ (CRAC) channels or (Orai 1 channels). Tetrodotoxin-resistant (TTX-R) Na+ (NaV1.9) TTX-R.
2.1 Regulation of ion channels by antioxidants
Post-translational modifications (PTMs) are significant mechanisms modulating the functions of ion channels. Protein phosphorylation is a classical PTM, and protein kinases regulate many ion channels throughout phosphorylation (Yang et al., 2014). There are different types of PTMs, such as Ubiquitylation, S-glutathionylation, O-glycosylation, etc. Both normal and abnormal states, including oxidative stress, are linked to post-translational modifications (PTMs) mediated by redox processes targeting cysteine residues’ thiol group. Redox-mediated post-translational modifications (PTMs) constitute a significant set of PTMs that specifically target the thiol group of cysteine residues. These modifications are evident in various physiological and pathological contexts, including situations marked by oxidative stress. Redox-mediated post-translational modifications (PTMs) constitute a significant group of PTMs that specifically affect the thiol group of cysteine residues. These modifications are evident in various physiological and pathological conditions characterized by oxidative stress (Moran et al., 2001; Dalle-Donne et al., 2008; Yang et al., 2014). One prominent mechanism for redox-mediated thiol modulation is S-glutathionylation, which involves adding a glutathione (GSH) group to the protein. The presence of reactive oxygen species (ROS) plays a vital role in facilitating S-glutathionylation. This phenomenon is increasingly observed in various ion channels, including voltage-gated Ca2+ channels and ATP-sensitive K+ channels (KATP) (Tang et al., 2011; Yang et al., 2011).
Reduced GSH is a significant non-enzymatic antioxidant in mammalian cells (Averill-Bates, 2023). GSH is a tripeptide composed of glycine, cysteine, glutamate, and the active thiol group in the cysteine residue that acts as a potent antioxidant. This antioxidant GSH is produced within the cell’s cytoplasm and transported to the mitochondria (Wadey et al., 2009). Hydrogen sulfide (H2S) has long been regarded as toxic, but it is now being found to play an important physiological role (at low concentrations). Nitric oxide (NO) is another well-known gaseous intracellular signal transducer, including H2S. H2S is produced from cysteine by several enzymes and plays a physiological role in cell signaling regulation, homeostasis, and combating oxidative species, such as ROS/RNS, in the body (Olas, 2015; Shefa et al., 2018).
Studies on the modulation of ion channel functions by NO and H2S are summarized in Table 2.
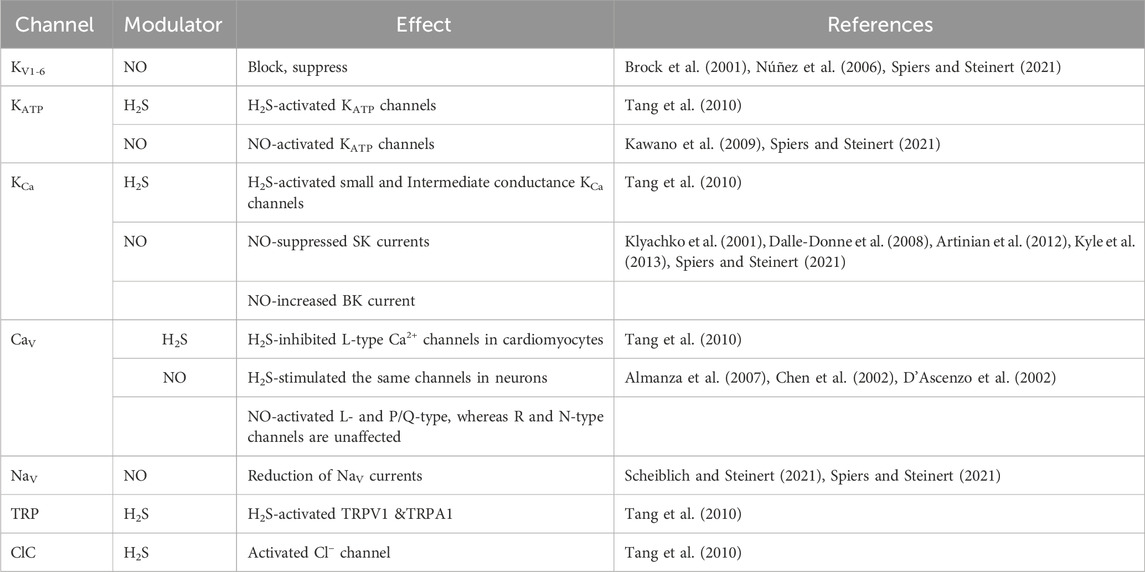
TABLE 2. A summary of studies on NO, H2S modulation affecting ion channels. Nitric oxide (NO), Hydrogen sulfide (H2S).
2.2 Ion channel mutations and oxidative stress
Most known human ion channel diseases or channelopathies are hereditary and investigated through genetic approaches (Li and Lester, 2001). Genetic analysis studies can be challenging because the clinical phenotypes are complex, and significant genetic heterogeneity exists. In other words, mutations in different genes may lead to the same clinical phenotype. Despite these challenges, numerous genes associated with human diseases have been successfully identified, characterized, and localized by applying molecular genetic techniques (Li and Lester, 2001; Nam et al., 2023a). Genome-wide association studies (GWAS) have linked ion channels to oxidative stress-related disorders (Akbarali, 2014; Ramírez et al., 2016; Liguori et al., 2018). The consequences of these ion channel mutations related to oxidative stress are diverse and contribute to the pathogenesis of various diseases, such as neurological disorders, cardiac arrhythmias, and certain types of cancers. Moreover, the aging process is linked with increased oxidative stress and a higher incidence of ion channel dysfunctions (Uttara et al., 2009; Chang et al., 2014; Kurian et al., 2016; Liguori et al., 2018). Understanding the relationship between ion channel mutations and oxidative stress is essential for developing targeted therapeutic strategies. Detailed information on specific potassium channel mutations and oxidative stress-related disorders, such as ataxias, can be found in these excellent manuscripts (Figueroa et al., 2010; Duarri et al., 2012; Duarri et al., 2012; Lee et al., 2012; Nam et al., 2023a). This review will generally cover different ion channel mutations caused by oxidative stress in neurodegenerative diseases.
3 Oxidative stress in neurodegenerative disorders
Neurodegenerative disorders affect millions of people worldwide. Brain atrophy is the hallmark of neurodegenerative diseases due to constant decline in neuronal function. Despite age being a significant risk factor for all neurodegenerative disorders, recent research indicates that genetic makeup and environmental factors greatly influence the risk as well (Chen et al., 2012; Lamptey et al., 2022). Although neurodegenerative disorders have distinct etiologies and develop in different brain sites, recent studies have observed that their effects on cellular and molecular mechanisms are similar (Aborode et al., 2022; Gates et al., 2022; Teleanu et al., 2022; Rehman et al., 2023). The central nervous system (CNS) has a significant oxidative potential because of its elevated oxygen usage. However, the CNS is particularly vulnerable to oxidative stress because of the abundance of readily oxidizable substances, limited levels of primary and secondary antioxidants, elevated iron content in specific brain regions, the generation of ROS by various internal mechanisms, and the presence of non-replicating neurons (Maher, 2006; Adibhatla and Hatcher, 2010; Guevara-García et al., 2012). Figure 1 demonstrates the tendency of neurodegenerative diseases to progress as a result of oxidative stress (Teleanu et al., 2022). Cells malfunction and even undergo apoptosis because the redox balance shifts to oxidative (Lew et al., 2022). Various neurodegenerative disorders are believed to be impacted by oxidative stress (Figure 1). Ion channels’ dysregulation is another common pathophysiologic mechanism that causes degenerative CNS diseases of widely differing genetic etiologies (Huang and Shakkottai, 2023). Furthermore, H2S at low concentrations lowers the level of ROS and thus protects neurons from oxidative stresses (Shefa et al., 2018). The inhaled form of H2S has a neuroprotective role In a Parkinson’s disease mouse model (Kida et al., 2011). It also protects neurons from apoptosis and degeneration (Olas, 2015).
Oxidative stress has been suggested as a factor in the development of various neurodegenerative disorders, including certain types of ataxias. The etiology of the diseases is multifaceted, with genetic and familial investigations underscoring their heterogeneity (Guevara-García et al., 2012). Point mutations often lead to diminished expression of proteins specific to the mutated genes. The connection between neurodegenerative disorders and oxidative stress is dependent on molecular, in vitro, and animal studies findings. Nonetheless, conflicting results emerge from human biomarker studies, indicating the necessity for additional research on the role of redox in neurodegenerative disorders associated with channelopathies (Li and Lester, 2001; Guevara-García et al., 2012).
Listed below are some findings that correlate with the modulation of ion channels and overproduction of ROS with neurodegenerative disorders, such as inherited cerebellar ataxia and Parkinson’s disease.
3.1 Inherited cerebellar ataxia
Inherited Cerebellar Ataxias (ICAs) combine a group of complex and uncommon neurodegenerative conditions that impact the cerebellum, spinal cord, and peripheral nerves (Coarelli et al., 2023). A person with ICA can experience balance, gait, speech, limb movement, eye movement, and cognitive difficulties. A significant correlation exists between ataxia location and cerebellar neuropathology: hemisphere lesions result in limb or appendicular ataxia, while midline lesions result in gait ataxia (Kashyap et al., 2020). Spinocerebellar ataxia (SCA) is a subgroup of hereditary cerebellar ataxia, a progressive, neurodegenerative, heterogeneous, rare disease that affects the cerebellum (Brooker et al., 2021; Bhandari et al., 2023). The pathology of spinocerebellar ataxia is still unknown, but the principal cells involved in degeneration are Purkinje cells (Koeppen, 2005). Purkinje cells regulate fine movement and muscle coordination. Thus, a decline in the normal firing of the Purkinje cells leads to an excessive calcium influx and excitotoxicity (Koeppen, 2005; Hosy et al., 2011). In the CNS, particularly the cerebellum, histopathology shows atrophy and enlargement of the lateral ventricles, loss of myelin in the frontal horn of the spinal cord, and axonal degeneration (Bhandari et al., 2023).
3.1.1 ICA and oxidative stress
There is an association between oxidative stress and several neurological disorders, including hereditary ataxias (Guevara-García et al., 2012; Lew et al., 2022). Numerous investigations have been conducted to prove the therapeutic roles of antioxidants in ICAs (Sarva and Shanker, 2014; Braga Neto et al., 2016; Picher-Martel and Dupre, 2018). Nevertheless, the results indicated that these antioxidants only partially alleviated symptoms of ICAs. This limitation may be because of the emphasis on clinical outcomes rather than a comprehensive understanding of the underlying molecular mechanisms associated with their approach to addressing oxidative stress (Picher-Martel and Dupre, 2018; Lew et al., 2022). The cause of ICAs is diverse (Coarelli et al., 2023). The link between ataxia and oxidative stress depends mainly on molecular, in vitro, and in vivo studies. Recent findings, for example, have indicated that ataxin 2 and others are linked with the redox imbalance in this disease (Guevara-García et al., 2012).
The significance of understanding the influence of oxidative stress on ion channels is crucial in considering ataxias. It is also needed to develop innovative approaches via alternative therapeutic intervention in ICA and related diseases.
3.1.2 Ion channels involved in oxidative stress-related ataxia
A cerebellar cortex includes Purkinje cells that integrate all input into the cerebellum (Hosy et al., 2011; Hirano, 2018; Huang and Shakkottai, 2023). A common feature of cerebellar ataxia is cerebellar atrophy and Purkinje neuron degeneration (Koeppen, 2005; Cocozza et al., 2021). Purkinje neurons are unique in that they spike independently of synaptic stimulation. SCA mouse models demonstrate that disruptions in the firing in Purkinje neurons considerably weaken motor function, indicating that this pacemaking ability of Purkinje neurons plays a critical role in motor coordination (Kurian et al., 2016; Bhandari et al., 2023; Dell’Orco et al., 2015; Jayabal et al., 2016). In resting conditions, Purkinje neurons fire at an average frequency of 40 Hz with unvarying inter-spike interval duration. Ion channels are predominantly responsible for maintaining this regularity (Raman and Bean, 1999; Braga Neto et al., 2016; Huang and Shakkottai, 2023).
In Purkinje neurons, voltage-gated Na+ channels (Nav1.6 and Nav1.1) initiate action potentials when they are activated. Then, the voltage-gated K+ channel activation will mediate the repolarization of the action potential. Ca2+ enters Purkinje neurons via voltage-gated Ca2+ channels, mainly Cav2.1 and Cav3.1, upon depolarization. When Ca2+ binds to a specific type of K+ channel, called Ca2+-activated K+ channels, an outward K+ current is generated, and the Purkinje neuron is hyperpolarized to produce the afterhyperpolarization (AHP) (Cooper and Jan, 1999; Li and Lester, 2001; Burke and Bender, 2019). The main Ca2+-activated K+ channels that generate the AHP in Purkinje neurons are small-conductance Ca2+-activated K+ channels type 2 (KCa2.2) channels (Kasumu et al., 2012; Nam et al., 2017; Nam et al., 2023b) and large-conductance Ca2+-activated K+ (BK) channels (Du et al., 2020) (Table 3).
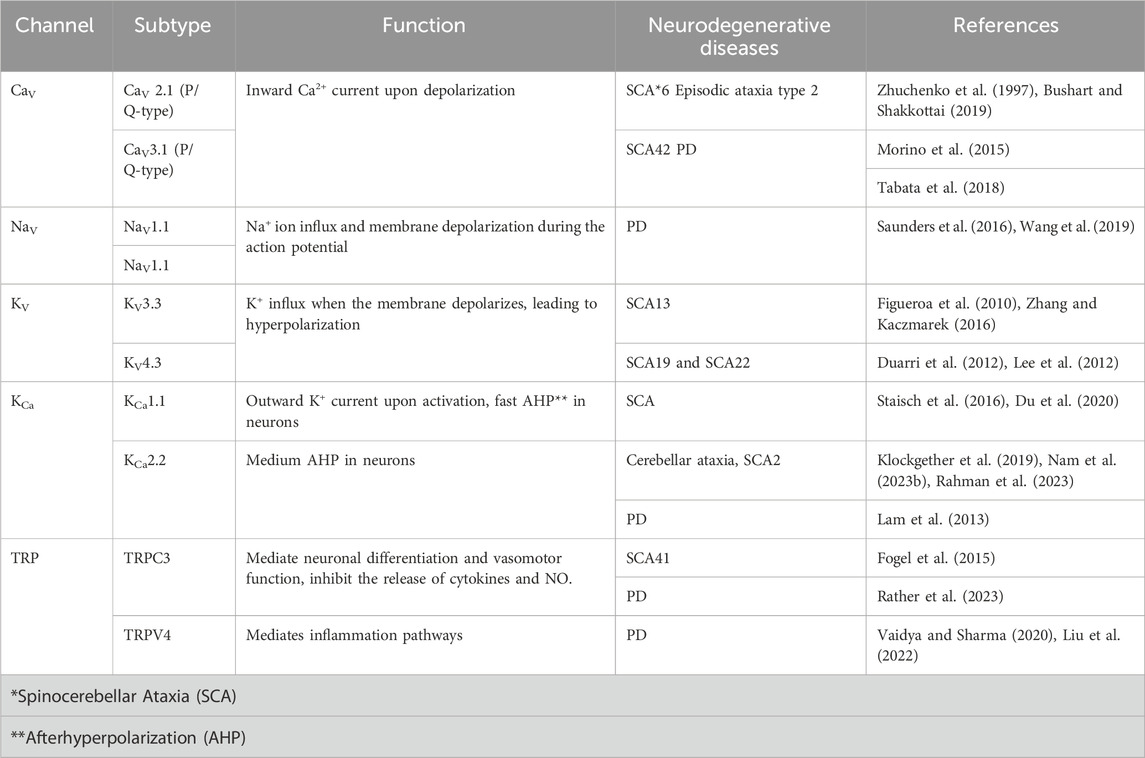
TABLE 3. Summary of some ion channels involved in oxidative stress-related neurodegenerative disorders.
Because ion channels play an important function in Purkinje neuron physiology, maintaining redox equilibrium is crucial to maintaining neurons’ homeostasis due to the alteration of their activity by oxidative stress. Enzymatic antioxidants, such as superoxide dismutase, glutathione peroxidases, and catalase, along with non-enzymatic antioxidants like GSH and vitamins A, C, and E, counteract various types of oxidative stress (Irato and Santovito, 2021). These antioxidants, whether endogenous or exogenous, reduce oxidative stress and scavenge ROS in ICAs, which could pave the way for a new ICA treatment (Pandolfo, 2008; Lew et al., 2022). Activating antioxidative transcription factor NRF2 could be a viable strategy to alleviate oxidative damage in ICAs (Kavian et al., 2018). In response to oxidative stress, NRF2 modulates key antioxidant enzymes, which, either directly or indirectly, regulate redox homeostasis (Liu et al., 2016; Lew et al., 2022). AM-36 is a neuroprotective agent that combines antioxidant and Na+ channel blockade properties (Callaway et al., 2001). Compared to agents possessing only one of these actions, AM-36 inhibited toxicity and apoptosis (mediated by the generation of ROS) (Callaway et al., 2001). Therefore, it is important to understand the link between the strategy targeting specific ion channels and antioxidants in mediating the progression of ICAs.
3.2 Parkinson’s disease
Parkinson’s disease (PD) is a common neurodegenerative condition distinguished by the progressive degeneration of dopaminergic cells in the substantia nigra, a region in the midbrain known for its accumulation of synuclein (Poewe et al., 2017). The dopamine secretion by these neurons is crucial for controlling movement ease and balance. Multiple pathways and mechanisms are involved in the underlying molecular pathogenesis, including synthase proteostasis, oxidative stress, mitochondrial function, neuroinflammation, Ca2+ homeostasis, and axonal transport (Miraglia et al., 2015; Poewe et al., 2017). The etiologies of PD are still questionable. Leucine-rich repeat kinase 2 (LRRK2) Mutations are one of the causative genetic variants that account for several autosomal, dominantly inherited PD (Blauwendraat et al., 2020). It has also been discovered that other genes, including ATP13A2, SNCA, PINK, GIGYF2, HTRA2, and DJ1, can cause familiar and early-onset PD. Among their functions are the degradation of ubiquitin proteins, the response to oxidative stress, apoptosis, cell survival, and mitochondrial function (Maiti et al., 2017).
3.2.1 Parkinson’s diseases and oxidative stress
Oxidative stress significantly promotes the erosion of dopaminergic neurons in PD (Dias et al., 2013). Oxygen is essential for brain function, and a large amount of oxygen is converted into ROS. Overproduction of ROS in the brain raises oxidative stress in people with Parkinson’s disease (Chang and Chen, 2020). Oxidative stress is closely related to other components of the degenerative process, like excitotoxicity, nitric oxide toxicity, and mitochondrial dysfunction (Jenner, 2003; Henchcliffe and Beal, 2008). Several genes associated with familial PD, including parkin, alpha-synuclein, LRRK2, DJ-1, and PINK-1, have been identified, providing important understandings of the molecular pathways underlying the disease pathogenesis, as well as highlighting earlier mysterious mechanisms where oxidative stress plays a role in the disease (Dias et al., 2013). Examination of brain tissue in Parkinson’s disease patients reveals a reduced level of GSH compared to glutathione disulfide (GSSG) compared to healthy brain tissue (Sian et al., 1994; Pearce et al., 1997). As oxidative stress leads to programmed cell death, the mitochondrial condition of GSH has gained recognition as a significant indicator of this occurrence (Chang and Chen, 2020).
3.2.2 Ion channels involved in oxidative stress-related Parkinson’s
Targeting ion channels provides an intriguing mechanistic strategy to address the progression of PD and other neurodegenerative disorders because of their important roles in neuronal activities (Braga Neto et al., 2016). K+ channels are important in neuronal excitability, neurotransmitter release, neuroinflammation, and synaptic transmission in PD pathology. In dopaminergic neurons, voltage-dependent K+ currents mediate repolarizing action potentials and fine-tune pacemaker firing rates (Braga Neto et al., 2016; Picher-Martel and Dupre, 2018). Voltage-gated Na+ channels modulate pacemaker frequency (Chen et al., 2012; Sarva and Shanker, 2014).
Tetrodotoxin inhibits the voltage-gated Na+ channels, revealing slow oscillatory potentials regulated by L-Type voltage-gated Ca2+ channels that regulate the precision, frequency, and robustness of peacemaking (Zaichick et al., 2017) (Table 3). While excessive firing and excess Ca2+ are significantly studied in connection to neurodegeneration, the direct function of the related ion channels in PD pathogenesis is mysterious. A variety of Parkinson’s disease animal models, ranging from toxin-induced to genetically modified mice, exhibit abnormalities in the operation of different ion channels (Daniel et al., 2021). The pathogenesis of Parkinson’s disease encompasses numerous interconnected pathways, including protein aggregation, oxidative stress, mitochondrial impairments, and abnormalities in autophagy. As a result, there have been numerous efforts to address these pathways in order to provide neuroprotection (Daniel et al., 2021). While several of these drugs in preclinical studies have demonstrated positive outcomes, none of these interventions have effectively transitioned into clinical application (Jenner, 2003).
In the field of ion channel drug discovery, a significant challenge is preventing side effects arising from both target and off-target mechanisms. Additionally, subtype selectivity is challenging when various homologous members belong to the same subfamily (Brown et al., 2020; Chen et al., 2023).
4 Concluding remarks
Ion channel malfunction is a common factor in neurological disorders, even when various genes are implicated as the root causes of these diseases. The malfunction of ion channels can result from changes in the intracellular redox environment, which alter how these channels function. Consequently, oxidative stress shows a significant role in the onset and development of neurodegenerative conditions involving ataxias, Parkinson’s, Alzheimer’s, and ALS. Despite recent advancements, the precise mechanisms of reactive oxygen species (ROS)-mediated neurodegenerative diseases remain partially understood. The role of ion channels in neurodegenerative disorders associated with oxidative stress has now been recognized, as they experience functional adjustments in such conditions. However, the significance of targeting ion channels therapeutically varies depending on the disease and the tissues in which these channels are active. Ultimately, neurodegenerative diseases may be effectively treated with a combination of ion channel-modulating therapy and antioxidant medication. More research on the function of ion channels in oxidative stress may provide a platform for exploring new therapeutic approaches for treating many neurodegenerative diseases associated with oxidative stress.
Author contributions
RO: Visualization, Writing–original draft. AA: Writing–review and editing. RSO: Visualization, Writing–original draft. LL: Writing–review and editing. NC: Writing–review and editing. AMA: Writing–review and editing. Y-WN: Supervision, Writing–review and editing. MZ: Conceptualization, Supervision, Writing–review and editing.
Funding
The author(s) declare financial support was received for the research, authorship, and/or publication of this article. The study was supported by a 23AIREA1039423 grant from the American Heart Association and a 4R33NS101182-03 grant from NIH awarded to MZ.
Acknowledgments
We thank King Fahad Medical City Writing Center for revising the manuscript. Thanks to Rahaf and Raghad Alabdulsalam for their technical Support. The figures were created with BioRender and published with permission.
Conflict of interest
Author RSO is a member of BrainExperiments.com.
The remaining authors declare that the research was conducted in the absence of any commercial or financial relationships that could be construed as a potential conflict of interest.
Publisher’s note
All claims expressed in this article are solely those of the authors and do not necessarily represent those of their affiliated organizations, or those of the publisher, the editors and the reviewers. Any product that may be evaluated in this article, or claim that may be made by its manufacturer, is not guaranteed or endorsed by the publisher.
Abbreviations
(ALS), Amyotrophic lateral sclerosis; (AHP), Afterhyperpolarization; (Ca2+), Calcium; (CNS), Central nervous system; (GWAS), Genome-wide association studies; (GSH), Glutathione; (H2S), Hydrogen sulfide; (ICAs), Inherited Cerebellar Ataxias; (BK), Large-conductance Ca+2-activated K+; (LRRK2), Leucine-rich repeat kinase 2; (LTCC), L-type voltage-gated Ca2+channel; (NO), Nitric oxide; (NSAIDs), Non-steroidal anti-inflammatory drugs; (nACHR), Nicotinic acetylcholine receptor; (PD), Parkinson’s disease; (PTMs), Post-translational modifications; (K+), Potassium; (ROS), Reactive oxygen species; (RNS), Reactive nitrogen species; (KCa2.x or SK), Small-conductance Ca2+-activated K+; (TMs), Transmembrane helices; (K2P), Two-Pore domain K+ channels; (TRP), Transient Receptor Potential; (SCA), Spinocerebellar ataxias; (KV), Voltage-gated K+; (NaV), Voltage-gated Na+; (CaV), Voltage-gated Ca2+.
References
Aborode A. T., Pustake M., Awuah W. A., Alwerdani M., Shah P., Yarlagadda R., et al. (2022). Targeting oxidative stress mechanisms to treat alzheimer’s and Parkinson’s disease: a critical review. Oxid. Med. Cell Longev. 2022, 7934442. doi:10.1155/2022/7934442
Adelman J. P., Clapham D. E., Hibino H., Inanobe A., Jan L. Y., Karschin A., et al. (2023). Inwardly rectifying potassium channels (KIR) in GtoPdb v.2023.1. IUPHARBPS Guide Pharmacol. CITE 2023. doi:10.2218/gtopdb/F74/2023.1
Adibhatla R. M., Hatcher J. F. (2010). Lipid oxidation and peroxidation in CNS health and disease: from molecular mechanisms to therapeutic opportunities. Antioxid. Redox Signal 12, 125–169. doi:10.1089/ars.2009.2668
Aggarwal P., Singh S., Ravichandiran V. (2021). Two-pore domain potassium channel in neurological disorders. J. Membr. Biol. 254, 367–380. doi:10.1007/s00232-021-00189-8
Akbarali H. (2014). Oxidative stress and ion channels. Syst. Biol. Free Radic. Antioxid., 355–373. doi:10.1007/978-3-642-30018-9_12
Aldrich R., Chandy K. G., Grissmer S., Gutman G. A., Kaczmarek L. K., Wei A. D., et al. (2021). IUPHAR/BPS guide to pharmacology CITE [English]. Edinburgh University Library. Available at: http://journals.ed.ac.uk/gtopdb-cite.
Almanza A., Navarrete F., Vega R., Soto E. (2007). Modulation of voltage-gated Ca2+ current in vestibular hair cells by nitric oxide. J. Neurophysiol. 97, 1188–1195. doi:10.1152/jn.00849.2006
Annunziato L., Pannaccione A., Cataldi M., Secondo A., Castaldo P., Di Renzo G., et al. (2002). Modulation of ion channels by reactive oxygen and nitrogen species: a pathophysiological role in brain aging? Neurobiol. Aging 23, 819–834. doi:10.1016/s0197-4580(02)00069-6
Artinian L., Zhong L., Yang H., Rehder V. (2012). Nitric oxide as intracellular modulator: internal production of NO increases neuronal excitability via modulation of several ionic conductances. Eur. J. Neurosci. 36, 3333–3343. doi:10.1111/j.1460-9568.2012.08260.x
Ashok A., Andrabi S. S., Mansoor S., Kuang Y., Kwon B. K., Labhasetwar V. (2022). Antioxidant therapy in oxidative stress-induced neurodegenerative diseases: role of nanoparticle-based drug delivery systems in clinical translation. Antioxidants (Basel). 11 (2), 408. doi:10.3390/antiox11020408
Averill-Bates D. A. (2023). The antioxidant glutathione. Vitam. Horm. 121, 109–141. doi:10.1016/bs.vh.2022.09.002
Barbieri R., Nizzari M., Zanardi I., Pusch M., Gavazzo P. (2023). Voltage-gated sodium channel dysfunctions in neurological disorders. Life 13, 1191. doi:10.3390/life13051191
Bhandari J., Thada P. K., Samanta D. (2023). Spinocerebellar ataxia. StatPearls, treasure island (FL). St. Petersburg, Florida: StatPearls Publishing.
Birben E., Sahiner U. M., Sackesen C., Erzurum S., Kalayci O. (2012). Oxidative stress and antioxidant defense. World Allergy Organ J. 5, 9–19. doi:10.1097/WOX.0b013e3182439613
Blauwendraat C., Nalls M. A., Singleton A. B. (2020). The genetic architecture of Parkinson’s disease. Lancet Neurol. 19, 170–178. doi:10.1016/S1474-4422(19)30287-X
Bogeski I., Niemeyer B. A. (2014). Redox regulation of ion channels. Antioxid. Redox Signal 21, 859–862. doi:10.1089/ars.2014.6019
Braga Neto P., Pedroso J. L., Kuo S.-H., Marcondes Junior C. F., Teive H. A. G., Barsottini O. G. P. (2016). Current concepts in the treatment of hereditary ataxias. Arq. Neuropsiquiatr. 74, 244–252. doi:10.1590/0004-282X20160038
Brock M. W., Mathes C., Gilly W. F. (2001). Selective open-channel block of shaker (Kv1) potassium channels by S-nitrosodithiothreitol (sndtt). J. Gen. Physiol. 118, 113–134. doi:10.1085/jgp.118.1.113
Brooker S. M., Edamakanti C. R., Akasha S. M., Kuo S.-H., Opal P. (2021). Spinocerebellar ataxia clinical trials: opportunities and challenges. Ann. Clin. Transl. Neurol. 8, 1543–1556. doi:10.1002/acn3.51370
Brown B. M., Shim H., Christophersen P., Wulff H. (2020). Pharmacology of small- and intermediate-conductance calcium-activated potassium channels. Annu. Rev. Pharmacol. Toxicol. 60, 219–240. doi:10.1146/annurev-pharmtox-010919-023420
Browne S. E., Ferrante R. J., Beal M. F. (1999). Oxidative stress in Huntington’s disease. Brain Pathol. Zurich Switz. 9, 147–163. doi:10.1111/j.1750-3639.1999.tb00216.x
Burke K. J., Bender K. J. (2019). Modulation of ion channels in the axon: mechanisms and function. Front. Cell Neurosci. 13, 221. doi:10.3389/fncel.2019.00221
Bushart D. D., Shakkottai V. G. (2019). Ion channel dysfunction in cerebellar ataxia. Neurosci. Lett. 688, 41–48. doi:10.1016/j.neulet.2018.02.005
Butt A. M., Kalsi A. (2006). Inwardly rectifying potassium channels (Kir) in central nervous system glia: a special role for Kir4.1 in glial functions. J. Cell Mol. Med. 10, 33–44. doi:10.1111/j.1582-4934.2006.tb00289.x
Callaway J. K., Beart P. M., Jarrott B., Giardina S. F. (2001). Incorporation of sodium channel blocking and free radical scavenging activities into a single drug, AM-36, results in profound inhibition of neuronal apoptosis. Br. J. Pharmacol. 132, 1691–1698. doi:10.1038/sj.bjp.0704018
Catterall W. A. (2011). Voltage-gated calcium channels. Cold Spring Harb. Perspect. Biol. 3, a003947. doi:10.1101/cshperspect.a003947
Chang K.-H., Chen C.-M. (2020). The role of oxidative stress in Parkinson’s disease. Antioxidants 9, 597. doi:10.3390/antiox9070597
Chang X., Wang J., Jiang H., Shi L., Xie J. (2019). Hyperpolarization-activated cyclic nucleotide-gated channels: an emerging role in neurodegenerative diseases. Front. Mol. Neurosci. 12, 141. doi:10.3389/fnmol.2019.00141
Chang Y.-T., Chang W.-N., Tsai N.-W., Huang C.-C., Kung C.-T., Su Y.-J., et al. (2014). The roles of biomarkers of oxidative stress and antioxidant in alzheimer’s disease: a systematic review. Biomed. Res. Int. 2014, 182303. doi:10.1155/2014/182303
Chen J., Daggett H., De Waard M., Heinemann S. H., Hoshi T. (2002). Nitric oxide augments voltage-gated P/Q-type Ca(2+) channels constituting a putative positive feedback loop. Free Radic. Biol. Med. 32, 638–649. doi:10.1016/s0891-5849(02)00748-7
Chen X., Feng Y., Quinn R. J., Pountney D. L., Richardson D. R., Mellick G. D., et al. (2023). Potassium channels in Parkinson’s disease: potential roles in its pathogenesis and innovative molecular targets for treatment. Pharmacol. Rev. 75, 758–788. doi:10.1124/pharmrev.122.000743
Chen X., Guo C., Kong J. (2012). Oxidative stress in neurodegenerative diseases. Neural Regen. Res. 7, 376–385. doi:10.3969/j.issn.1673-5374.2012.05.009
Coarelli G., Wirth T., Tranchant C., Koenig M., Durr A., Anheim M. (2023). The inherited cerebellar ataxias: an update. J. Neurol. 270, 208–222. doi:10.1007/s00415-022-11383-6
Cocozza S., Pontillo G., De Michele G., Di Stasi M., Guerriero E., Perillo T., et al. (2021). Conventional MRI findings in hereditary degenerative ataxias: a pictorial review. Neuroradiology 63, 983–999. doi:10.1007/s00234-021-02682-2
Cooper E. C., Jan L. Y. (1999). Ion channel genes and human neurological disease: recent progress, prospects, and challenges. Proc. Natl. Acad. Sci. 96, 4759–4766. doi:10.1073/pnas.96.9.4759
Cunha-Oliveira T., Montezinho L., Mendes C., Firuzi O., Saso L., Oliveira P. J., et al. (2020). Oxidative stress in amyotrophic lateral sclerosis: pathophysiology and opportunities for pharmacological intervention. Oxid. Med. Cell Longev. 2020, 5021694. doi:10.1155/2020/5021694
Dalle-Donne I., Milzani A., Gagliano N., Colombo R., Giustarini D., Rossi R. (2008). Molecular mechanisms and potential clinical significance of S-glutathionylation. Antioxid. Redox Signal 10, 445–473. doi:10.1089/ars.2007.1716
Daniel N. H., Aravind A., Thakur P. (2021). Are ion channels potential therapeutic targets for Parkinson’s disease? NeuroToxicology 87, 243–257. doi:10.1016/j.neuro.2021.10.008
D’Ascenzo M., Martinotti G., Azzena G. B., Grassi C. (2002). cGMP/protein kinase G-dependent inhibition of N-type Ca2+ channels induced by nitric oxide in human neuroblastoma IMR32 cells. J. Neurosci. Off. J. Soc. Neurosci. 22, 7485–7492. doi:10.1523/JNEUROSCI.22-17-07485.2002
de Lera Ruiz M., Kraus R. L. (2015). Voltage-gated sodium channels: structure, function, pharmacology, and clinical indications. J. Med. Chem. 58, 7093–7118. doi:10.1021/jm501981g
Dell’Orco J. M., Wasserman A. H., Chopra R., Ingram M. A. C., Hu Y.-S., Singh V., et al. (2015). Neuronal atrophy early in degenerative ataxia is a compensatory mechanism to regulate membrane excitability. J. Neurosci. Off. J. Soc. Neurosci. 35, 11292–11307. doi:10.1523/JNEUROSCI.1357-15.2015
Dias V., Junn E., Mouradian M. M. (2013). The role of oxidative stress in Parkinson’s disease. J. Park Dis. 3, 461–491. doi:10.3233/JPD-130230
DiFrancesco J. C., DiFrancesco D. (2015). Dysfunctional HCN ion channels in neurological diseases. Front. Cell Neurosci. 6, 174. doi:10.3389/fncel.2015.00071
Du X., Carvalho-de-Souza J. L., Wei C., Carrasquel-Ursulaez W., Lorenzo Y., Gonzalez N., et al. (2020). Loss-of-function BK channel mutation causes impaired mitochondria and progressive cerebellar ataxia. Proc. Natl. Acad. Sci. U. S. A. 117, 6023–6034. doi:10.1073/pnas.1920008117
Duarri A., Jezierska J., Fokkens M., Meijer M., Schelhaas H. J., den Dunnen W. F. A., et al. (2012). Mutations in potassium channel kcnd3 cause spinocerebellar ataxia type 19. Ann. Neurol. 72, 870–880. doi:10.1002/ana.23700
Fan X., Lu Y., Du G., Liu J. (2022). Advances in the understanding of two-pore domain TASK potassium channels and their potential as therapeutic targets. Molecules 27, 8296. doi:10.3390/molecules27238296
Figueroa K. P., Minassian N. A., Stevanin G., Waters M., Garibyan V., Forlani S., et al. (2010). KCNC3: phenotype, mutations, channel biophysics – a study of 260 familial ataxia patients. Hum. Mutat. 31, 191–196. doi:10.1002/humu.21165
Fogel B. L., Hanson S. M., Becker E. B. E. (2015). Do mutations in the murine ataxia gene TRPC3 cause cerebellar ataxia in humans? Mov. Disord. Off. J. Mov. Disord. Soc. 30, 284–286. doi:10.1002/mds.26096
Gates E. J., Bernath A. K., Klegeris A. (2022). Modifying the diet and gut microbiota to prevent and manage neurodegenerative diseases. Rev. Neurosci. 33, 767–787. doi:10.1515/revneuro-2021-0146
Gella A., Durany N. (2009). Oxidative stress in Alzheimer disease. Cell Adhes. Migr. 3, 88–93. doi:10.4161/cam.3.1.7402
Griendling K. K., Camargo L. L., Rios F. J., Alves-Lopes R., Montezano A. C., Touyz R. M. (2021). Oxidative stress and hypertension. Circ. Res. 128, 993–1020. doi:10.1161/CIRCRESAHA.121.318063
Guevara-García M., Gil-del Valle L., Velásquez-Pérez L., García-Rodríguez J. C. (2012). Oxidative stress as a cofactor in spinocerebellar ataxia type 2. Redox Rep. Commun. Free Radic. Res. 17, 84–89. doi:10.1179/1351000212Y.0000000005
Gulcin İ. (2020). Antioxidants and antioxidant methods: an updated overview. Arch. Toxicol. 94, 651–715. doi:10.1007/s00204-020-02689-3
Harraz O. F., Klug N. R., Senatore A. J., Hill-Eubanks D. C., Nelson M. T. (2022). Piezo1 is a mechanosensor channel in central nervous system capillaries. Circ. Res. 130, 1531–1546. doi:10.1161/CIRCRESAHA.122.320827
Henchcliffe C., Beal M. F. (2008). Mitochondrial biology and oxidative stress in Parkinson disease pathogenesis. Nat. Clin. Pract. Neurol. 4, 600–609. doi:10.1038/ncpneuro0924
Hirano T. (2018). Purkinje neurons: development, morphology, and function. Cerebellum Lond Engl. 17, 699–700. doi:10.1007/s12311-018-0985-7
Hosy E., Piochon C., Teuling E., Rinaldo L., Hansel C. (2011). SK2 channel expression and function in cerebellar Purkinje cells. J. Physiol. 589, 3433–3440. doi:10.1113/jphysiol.2011.205823
Huang H., Shakkottai V. G. (2023). Targeting ion channels and Purkinje neuron intrinsic membrane excitability as a therapeutic strategy for cerebellar ataxia. Life 13, 1350. doi:10.3390/life13061350
Irato P., Santovito G. (2021). Enzymatic and non-enzymatic molecules with antioxidant function. Antioxidants 10, 579. doi:10.3390/antiox10040579
Jayabal S., Chang H. H. V., Cullen K. E., Watt A. J. (2016). 4-aminopyridine reverses ataxia and cerebellar firing deficiency in a mouse model of spinocerebellar ataxia type 6. Sci. Rep. 6, 29489. doi:10.1038/srep29489
Jenner P. (2003). Oxidative stress in Parkinson’s disease. Ann. Neurol. 53 (Suppl. 3), S26–S36. doi:10.1002/ana.10483
Jin P., Jan L. Y., Jan Y.-N. (2020). Mechanosensitive ion channels: structural features relevant to mechanotransduction mechanisms. Annu. Rev. Neurosci. 43, 207–229. doi:10.1146/annurev-neuro-070918-050509
Kashyap B., Phan D., Pathirana P. N., Horne M., Power L., Szmulewicz D. (2020). Objective assessment of cerebellar ataxia: a comprehensive and refined approach. Sci. Rep. 10, 9493. doi:10.1038/s41598-020-65303-7
Kasumu A. W., Hougaard C., Rode F., Jacobsen T. A., Sabatier J. M., Eriksen B. L., et al. (2012). Selective positive modulator of calcium-activated potassium channels exerts beneficial effects in a mouse model of spinocerebellar ataxia type 2. Chem. Biol. 19, 1340–1353. doi:10.1016/j.chembiol.2012.07.013
Kattoor A. J., Pothineni N. V. K., Palagiri D., Mehta J. L. (2017). Oxidative stress in atherosclerosis. Curr. Atheroscler. Rep. 19, 42. doi:10.1007/s11883-017-0678-6
Kaushik A. S., Strath L. J., Sorge R. E. (2020). Dietary interventions for treatment of chronic pain: oxidative stress and inflammation. Pain Ther. 9, 487–498. doi:10.1007/s40122-020-00200-5
Kavian N., Mehlal S., Jeljeli M., Saidu N. E. B., Nicco C., Cerles O., et al. (2018). The nrf2-antioxidant response element signaling pathway controls fibrosis and autoimmunity in scleroderma. Front. Immunol. 9, 1896. doi:10.3389/fimmu.2018.01896
Kawano T., Zoga V., Kimura M., Liang M.-Y., Wu H.-E., Gemes G., et al. (2009). Nitric oxide activates ATP-sensitive potassium channels in mammalian sensory neurons: action by direct S-nitrosylation. Mol. Pain 5, 12. doi:10.1186/1744-8069-5-12
Kida K., Yamada M., Tokuda K., Marutani E., Kakinohana M., Kaneki M., et al. (2011). Inhaled hydrogen sulfide prevents neurodegeneration and movement disorder in a mouse model of Parkinson’s disease. Antioxid. Redox Signal 15, 343–352. doi:10.1089/ars.2010.3671
Kiselyov K., Muallem S. (2016). ROS and intracellular ion channels. Cell Calcium 60, 108–114. doi:10.1016/j.ceca.2016.03.004
Klockgether T., Mariotti C., Paulson H. L. (2019). Spinocerebellar ataxia. Nat. Rev. Dis. Primer 5, 24. doi:10.1038/s41572-019-0074-3
Klyachko V. A., Ahern G. P., Jackson M. B. (2001). cGMP-mediated facilitation in nerve terminals by enhancement of the spike afterhyperpolarization. Neuron 31, 1015–1025. doi:10.1016/s0896-6273(01)00449-4
Koeppen A. H. (2005). The pathogenesis of spinocerebellar ataxia. Cerebellum Lond Engl. 4, 62–73. doi:10.1080/14734220510007950
Kurian G. A., Rajagopal R., Vedantham S., Rajesh M. (2016). The role of oxidative stress in myocardial ischemia and reperfusion injury and remodeling: revisited. Oxid. Med. Cell Longev. 2016, 1656450. doi:10.1155/2016/1656450
Kyle B. D., Hurst S., Swayze R. D., Sheng J., Braun A. P. (2013). Specific phosphorylation sites underlie the stimulation of a large conductance, Ca(2+)-activated K(+) channel by cGMP-dependent protein kinase. FASEB J. Off. Publ. Fed. Am. Soc. Exp. Biol. 27, 2027–2038. doi:10.1096/fj.12-223669
Lam J., Coleman N., Garing A. L. A., Wulff H. (2013). The therapeutic potential of small-conductance KCa2 channels in neurodegenerative and psychiatric diseases. Expert Opin. Ther. Targets 17, 1203–1220. doi:10.1517/14728222.2013.823161
Lamptey R. N. L., Chaulagain B., Trivedi R., Gothwal A., Layek B., Singh J. (2022). A review of the common neurodegenerative disorders: current therapeutic approaches and the potential role of nanotherapeutics. Int. J. Mol. Sci. 23, 1851. doi:10.3390/ijms23031851
Lee K., Jo Y. Y., Chung G., Jung J. H., Kim Y. H., Park C.-K. (2021). Functional importance of transient receptor potential (TRP) channels in neurological disorders. Front. Cell Dev. Biol. 9, 611773. doi:10.3389/fcell.2021.611773
Lee Y., Durr A., Majczenko K., Huang Y., Liu Y., Lien C., et al. (2012). Mutations in KCND3 cause spinocerebellar ataxia type 22. Ann. Neurol. 72, 859–869. doi:10.1002/ana.23701
Lew S. Y., Phang M. W. L., Chong P. S., Roy J., Poon C. H., Yu W. S., et al. (2022). Discovery of therapeutics targeting oxidative stress in autosomal recessive cerebellar ataxia: a systematic review. Pharmaceuticals 15, 764. doi:10.3390/ph15060764
Li M., Lester H. A. (2001). Ion Channel diseases of the central nervous system. CNS Drug Rev. 7, 214–240. doi:10.1111/j.1527-3458.2001.tb00196.x
Li S., Wong A. H. C., Liu F. (2014). Ligand-gated ion channel interacting proteins and their role in neuroprotection. Front. Cell Neurosci. 8, 125. doi:10.3389/fncel.2014.00125
Liguori I., Russo G., Curcio F., Bulli G., Aran L., Della-Morte D., et al. (2018). Oxidative stress, aging, and diseases. Clin. Interv. Aging 13, 757–772. doi:10.2147/CIA.S158513
Litalien C., Beaulieu P. (2011). “Chapter 117 - molecular mechanisms of drug actions: from receptors to effectors,” in Pediatr. Crit. Care Editors B. P. Fuhrman, and J. J. Zimmerman Fourth Ed (Saint Louis: Mosby), 1553–1568. doi:10.1016/B978-0-323-07307-3.10117-X
Liu N., Bai L., Lu Z., Gu R., Zhao D., Yan F., et al. (2022). TRPV4 contributes to ER stress and inflammation: implications for Parkinson’s disease. J. Neuroinflammation 19, 26. doi:10.1186/s12974-022-02382-5
Liu X., Ward K., Xavier C., Jann J., Clark A. F., Pang I.-H., et al. (2016). The novel triterpenoid RTA 408 protects human retinal pigment epithelial cells against H2O2-induced cell injury via NF-E2-related factor 2 (Nrf2) activation. Redox Biol. 8, 98–109. doi:10.1016/j.redox.2015.12.005
Maher P. (2006). Redox control of neural function: background, mechanisms, and significance. Antioxid. Redox Signal 8, 1941–1970. doi:10.1089/ars.2006.8.1941
Maiti P., Manna J., Dunbar G. L. (2017). Current understanding of the molecular mechanisms in Parkinson’s disease: targets for potential treatments. Transl. Neurodegener. 6 (28), 28. doi:10.1186/s40035-017-0099-z
Manna P., Jain S. K. (2015). Obesity, oxidative stress, adipose tissue dysfunction, and the associated health risks: causes and therapeutic strategies. Metab. Syndr. Relat. Disord. 13, 423–444. doi:10.1089/met.2015.0095
Martinac B. (2012). Mechanosensitive ion channels: an evolutionary and scientific tour de force in mechanobiology. Channels 6, 211–213. doi:10.4161/chan.22047
Miraglia F., Betti L., Palego L., Giannaccini G. (2015). Parkinson’s disease and alpha-synucleinopathies: from arising pathways to therapeutic challenge. Cent. Nerv. Syst. Agents Med. Chem. 15, 109–116. doi:10.2174/1871524915666150421114338
Miranda M. R., Vestuto V., Moltedo O., Manfra M., Campiglia P., Pepe G. (2023). The ion channels involved in oxidative stress-related gastrointestinal diseases. Oxygen 3, 336–365. doi:10.3390/oxygen3030022
Moran L. K., Gutteridge J. M., Quinlan G. J. (2001). Thiols in cellular redox signalling and control. Curr. Med. Chem. 8, 763–772. doi:10.2174/0929867013372904
Morino H., Matsuda Y., Muguruma K., Miyamoto R., Ohsawa R., Ohtake T., et al. (2015). A mutation in the low voltage-gated calcium channel CACNA1G alters the physiological properties of the channel, causing spinocerebellar ataxia. Mol. Brain 8, 89. doi:10.1186/s13041-015-0180-4
Nam Y.-W., Downey M., Rahman M. A., Cui M., Zhang M. (2023a). Channelopathy of small- and intermediate-conductance Ca2+-activated K+ channels. Acta Pharmacol. Sin. 44, 259–267. doi:10.1038/s41401-022-00935-1
Nam Y.-W., Orfali R., Liu T., Yu K., Cui M., Wulff H., et al. (2017). Structural insights into the potency of SK channel positive modulators. Sci. Rep. 7, 17178. doi:10.1038/s41598-017-16607-8
Nam Y.-W., Rahman M. A., Yang G., Orfali R., Cui M., Zhang M. (2023b). Loss-of-function KCa2.2 mutations abolish channel activity. Am. J. Physiol-Cell Physiol. 324, C658–C664. doi:10.1152/ajpcell.00584.2022
Núñez L., Vaquero M., Gómez R., Caballero R., Mateos-Cáceres P., Macaya C., et al. (2006). Nitric oxide blocks hKv1.5 channels by S-nitrosylation and by a cyclic GMP-dependent mechanism. Cardiovasc Res. 72, 80–89. doi:10.1016/j.cardiores.2006.06.021
Olas B. (2015). Hydrogen sulfide in signaling pathways. Clin. Chim. Acta Int. J. Clin. Chem. 439, 212–218. doi:10.1016/j.cca.2014.10.037
Orfali R., Albanyan N. (2023). Ca2+-Sensitive potassium channels. Molecules 28, 885. doi:10.3390/molecules28020885
Orfali R., AlFaiz A., Rahman M. A., Lau L., Nam Y.-W., Zhang M. (2023). KCa2 and KCa3.1 channels in the airways: a new therapeutic target. Biomedicines 11, 1780. doi:10.3390/biomedicines11071780
Orzechowski A., Cywińska A., Rostagno A. A., Rizzi F. M. (2019). Oxidative stress, chronic inflammation, and amyloidoses. Oxid. Med. Cell Longev. 2019, e6024975. doi:10.1155/2019/6024975
Pagan L. U., Gomes M. J., Martinez P. F., Okoshi M. P. (2022). Oxidative stress and heart failure: mechanisms, signalling pathways, and therapeutics. Oxid. Med. Cell Longev. 2022, e9829505. doi:10.1155/2022/9829505
Pandolfo M. (2008). Drug Insight: antioxidant therapy in inherited ataxias. Nat. Clin. Pract. Neurol. 4, 86–96. doi:10.1038/ncpneuro0704
Pearce R. K., Owen A., Daniel S., Jenner P., Marsden C. D. (1997). Alterations in the distribution of glutathione in the substantia nigra in Parkinson’s disease. J. Neural Transm. (Vienna). 104 (6-7), 661–677. doi:10.1007/BF01291884
Picher-Martel V., Dupre N. (2018). Current and promising therapies in autosomal recessive ataxias. CNS Neurol. Disord. Drug Targets 17, 161–171. doi:10.2174/1871527317666180419115029
Poewe W., Seppi K., Tanner C. M., Halliday G. M., Brundin P., Volkmann J., et al. (2017). Parkinson disease. Nat. Rev. Dis. Primer 3, 17013. doi:10.1038/nrdp.2017.13
Purves D., Augustine G. J., Fitzpatrick D., Katz L. C., LaMantia A.-S., McNamara J. O., et al. (2001a). “Voltage-gated ion channels,” in Neurosci. 2nd Ed. (Sunderland, Massachusetts: Sinauer Associates).
Purves D., Augustine G. J., Fitzpatrick D., Katz L. C., LaMantia A.-S., McNamara J. O., et al. (2001b). “Ligand-gated ion channels,” in Neurosci. 2nd Ed. (Sunderland, Massachusetts: Sinauer Associates).
Rahman M. A., Orfali R., Dave N., Lam E., Naguib N., Nam Y.-W., et al. (2023). KCa2.2 (KCNN2): a physiologically and therapeutically important potassium channel. J. Neurosci. Res. 101, 1699–1710. doi:10.1002/jnr.25233
Raman I. M., Bean B. P. (1999). Ionic currents underlying spontaneous action potentials in isolated cerebellar Purkinje neurons. J. Neurosci. Off. J. Soc. Neurosci. 19, 1663–1674. doi:10.1523/JNEUROSCI.19-05-01663.1999
Ramírez A., Vázquez-Sánchez A. Y., Carrión-Robalino N., Camacho J. (2016). Ion channels and oxidative stress as a potential link for the diagnosis or treatment of liver diseases. Oxid. Med. Cell Longev. 2016, 3928714. doi:10.1155/2016/3928714
Rather M. A., Khan A., Wang L., Jahan S., Rehman M. U., Makeen H. A., et al. (2023). TRP channels: role in neurodegenerative diseases and therapeutic targets. Heliyon 9, e16910. doi:10.1016/j.heliyon.2023.e16910
Rehman M. U., Sehar N., Dar N. J., Khan A., Arafah A., Rashid S., et al. (2023). Mitochondrial dysfunctions, oxidative stress and neuroinflammation as therapeutic targets for neurodegenerative diseases: an update on current advances and impediments. Neurosci. Biobehav Rev. 144, 104961. doi:10.1016/j.neubiorev.2022.104961
Rinke I., Artmann J., Stein V. (2010). ClC-2 voltage-gated channels constitute part of the background conductance and assist chloride extrusion. J. Neurosci. 30, 4776–4786. doi:10.1523/JNEUROSCI.6299-09.2010
Riverón F. G., Martínez B. O., Gutiérrez G. R., Pandolfi B. A., Pupo B. J., Pereira N., et al. (2010). Oxidative damage and antioxidant enzymes in blood of patients with Spinocerebellar Ataxia Type 2. Rev. Cuba Gen. 4 (1), 42–47.
Rosendo-Pineda M. J., Moreno C. M., Vaca L. (2020). Role of ion channels during cell division. Cell Calcium 91, 102258. doi:10.1016/j.ceca.2020.102258
Santulli G., Nakashima R., Yuan Q., Marks A. R. (2017). Intracellular calcium release channels: an update. J. Physiol. 595, 3041–3051. doi:10.1113/JP272781
Sarva H., Shanker V. L. (2014). Treatment options in degenerative cerebellar ataxia: a systematic review. Mov. Disord. Clin. Pract. 1, 291–298. doi:10.1002/mdc3.12057
Saunders A., Huang K. W., Sabatini B. L. (2016). Globus pallidus externus neurons expressing parvalbumin interconnect the subthalamic nucleus and striatal interneurons. PloS One 11, e0149798. doi:10.1371/journal.pone.0149798
Sawamura S., Shirakawa H., Nakagawa T., Mori Y., Kaneko S. (2017). “TRP channels in the brain: what are they there for?,” in Neurobiol. TRP channels. Editor T. L. R. Emir (Boca Raton (FL): CRC Press/Taylor and Francis).
Schampel A., Kuerten S. (2017). Danger: high voltage—the role of voltage-gated calcium channels in central nervous system pathology. Cells 6, 43. doi:10.3390/cells6040043
Scheiblich H., Steinert J. R. (2021). Nitrergic modulation of neuronal excitability in the mouse hippocampus is mediated via regulation of Kv2 and voltage-gated sodium channels. Hippocampus 31, 1020–1038. doi:10.1002/hipo.23366
Selivanov V. A., Votyakova T. V., Pivtoraiko V. N., Zeak J., Sukhomlin T., Trucco M., et al. (2011). Reactive oxygen species production by forward and reverse electron fluxes in the mitochondrial respiratory chain. PLOS Comput. Biol. 7, e1001115. doi:10.1371/journal.pcbi.1001115
Shah M. M. (2014). Cortical HCN channels: function, trafficking and plasticity. J. Physiol. 592, 2711–2719. doi:10.1113/jphysiol.2013.270058
Shah N. H., Aizenman E. (2014). Voltage-gated potassium channels at the crossroads of neuronal function, ischemic tolerance, and neurodegeneration. Transl. Stroke Res. 5, 38–58. doi:10.1007/s12975-013-0297-7
Shefa U., Kim M.-S., Jeong N. Y., Jung J. (2018). Antioxidant and cell-signaling functions of hydrogen sulfide in the central nervous system. Oxid. Med. Cell Longev. 2018, 1873962. doi:10.1155/2018/1873962
Shen K.-F., Yang X.-L., Liu G.-L., Zhu G., Wang Z.-K., Shi X.-J., et al. (2021). The role of voltage-gated chloride channels in the epileptogenesis of temporal lobe epilepsy. eBioMedicine 70, 103537. doi:10.1016/j.ebiom.2021.103537
Sian J., Dexter D. T., Lees A. J., Daniel S., Agid Y., Javoy-Agid F., et al. (1994). Alterations in glutathione levels in Parkinson’s disease and other neurodegenerative disorders affecting basal ganglia. Ann. Neurol. 36, 348–355. doi:10.1002/ana.410360305
Sies H., Berndt C., Jones D. P. (2017). Oxidative stress. Annu. Rev. Biochem. 86, 715–748. doi:10.1146/annurev-biochem-061516-045037
Simon F., Varela D., Cabello-Verrugio C. (2013). Oxidative stress-modulated TRPM ion channels in cell dysfunction and pathological conditions in humans. Cell Signal 25, 1614–1624. doi:10.1016/j.cellsig.2013.03.023
Spiers J. G., Steinert J. R. (2021). Nitrergic modulation of ion channel function in regulating neuronal excitability. Channels 15, 666–679. doi:10.1080/19336950.2021.2002594
Staisch J., Du X., Carvalho-de-Souza J., Kubota T., Bezanilla F., Gomez C. (2016). A mutation causing reduced BK channel activity leads to cognitive inpairment and progressive cerebellar ataxia (P5.394). Neurology 86, 86. doi:10.1212/wnl.86.16_supplement.p5.394
Tabata Y., Imaizumi Y., Sugawara M., Andoh-Noda T., Banno S., Chai M., et al. (2018). T-Type calcium channels determine the vulnerability of dopaminergic neurons to mitochondrial stress in familial Parkinson disease. Stem Cell Rep. 11, 1171–1184. doi:10.1016/j.stemcr.2018.09.006
Tang G., Wu L., Wang R. (2010). Interaction of hydrogen sulfide with ion channels. Clin. Exp. Pharmacol. Physiol. 37, 753–763. doi:10.1111/j.1440-1681.2010.05351.x
Tang H., Viola H. M., Filipovska A., Hool L. C. (2011). Ca(v)1.2 calcium channel is glutathionylated during oxidative stress in Guinea pig and ischemic human heart. Free Radic. Biol. Med. 51, 1501–1511. doi:10.1016/j.freeradbiomed.2011.07.005
Teleanu D. M., Niculescu A.-G., Lungu , Radu C. I., Vladâcenco O., Roza E., et al. (2022). An overview of oxidative stress, neuroinflammation, and neurodegenerative diseases. Int. J. Mol. Sci. 23, 5938. doi:10.3390/ijms23115938
Uttara B., Singh A. V., Zamboni P., Mahajan R. T. (2009). Oxidative stress and neurodegenerative diseases: a review of upstream and downstream antioxidant therapeutic options. Curr. Neuropharmacol. 7, 65–74. doi:10.2174/157015909787602823
Vaidya B., Sharma S. S. (2020). Transient receptor potential channels as an emerging target for the treatment of Parkinson’s disease: an insight into role of pharmacological interventions. Front. Cell Dev. Biol. 8, 584513. doi:10.3389/fcell.2020.584513
Valko M., Rhodes C. J., Moncol J., Izakovic M., Mazur M. (2006). Free radicals, metals and antioxidants in oxidative stress-induced cancer. Chem. Biol. Interact. 160, 1–40. doi:10.1016/j.cbi.2005.12.009
Wadey A. L., Muyderman H., Kwek P. T., Sims N. R. (2009). Mitochondrial glutathione uptake: characterization in isolated brain mitochondria and astrocytes in culture. J. Neurochem. 109 (Suppl. 1), 101–108. doi:10.1111/j.1471-4159.2009.05936.x
Wang J., Ou S.-W., Wang Y.-J. (2017). Distribution and function of voltage-gated sodium channels in the nervous system. Channels Austin Tex 11, 534–554. doi:10.1080/19336950.2017.1380758
Wang R., Tu S., Zhang J., Shao A. (2020). Roles of TRP channels in neurological diseases. Oxid. Med. Cell Longev. 2020, 7289194. doi:10.1155/2020/7289194
Wang Z., Lin Y., Liu W., Kuang P., Lao W., Ji Y., et al. (2019). Voltage-gated sodium channels are involved in cognitive impairments in Parkinson’s disease-like rats. Neuroscience 418, 231–243. doi:10.1016/j.neuroscience.2019.08.024
Weaver A. K., Bomben V. C., Sontheimer H. (2006). Expression and function of calcium-activated potassium channels in human glioma cells. Glia 54, 223–233. doi:10.1002/glia.20364
Yang Y., Jin X., Jiang C. (2014). S-glutathionylation of ion channels: insights into the regulation of channel functions, thiol modification crosstalk, and mechanosensing. Antioxid. Redox Signal 20, 937–951. doi:10.1089/ars.2013.5483
Yang Y., Shi W., Chen X., Cui N., Konduru A. S., Shi Y., et al. (2011). Molecular basis and structural insight of vascular K(ATP) channel gating by S-glutathionylation. J. Biol. Chem. 286, 9298–9307. doi:10.1074/jbc.M110.195123
Zaichick S. V., McGrath K. M., Caraveo G. (2017). The role of Ca2+ signaling in Parkinson’s disease. Dis. Model Mech. 10, 519–535. doi:10.1242/dmm.028738
Zhang Y., Kaczmarek L. K. (2016). Kv3.3 potassium channels and spinocerebellar ataxia. J. Physiol. 594, 4677–4684. doi:10.1113/JP271343
Zheng J., Trudeau M. C. (2023). Textbook of ion channels volume II: properties, function, and pharmacology of the superfamilies. Boca Raton, Florida: CRC Press.
Keywords: antioxidants, calcium channel, neurodegenerative disorders, oxidative stress, potassium channels, reactive oxygen species, sodium channels, glutathione
Citation: Orfali R, Alwatban AZ, Orfali RS, Lau L, Chea N, Alotaibi AM, Nam Y-W and Zhang M (2024) Oxidative stress and ion channels in neurodegenerative diseases. Front. Physiol. 15:1320086. doi: 10.3389/fphys.2024.1320086
Received: 11 October 2023; Accepted: 12 January 2024;
Published: 29 January 2024.
Edited by:
Antonio Gonzalez, University of Extremadura, SpainReviewed by:
Lei Wang, University of Miami, United StatesMurugesan Velayutham, West Virginia University, United States
Copyright © 2024 Orfali, Alwatban, Orfali, Lau, Chea, Alotaibi, Nam and Zhang. This is an open-access article distributed under the terms of the Creative Commons Attribution License (CC BY). The use, distribution or reproduction in other forums is permitted, provided the original author(s) and the copyright owner(s) are credited and that the original publication in this journal is cited, in accordance with accepted academic practice. No use, distribution or reproduction is permitted which does not comply with these terms.
*Correspondence: Young-Woo Nam, eXduYW1AY2hhcG1hbi5lZHU=; Miao Zhang, emhhbmdAY2hhcG1hbi5lZHU=