- 1General Practice Medical Center, West China Hospital, Sichuan University, Chengdu, China
- 2Department of Rehabilitation Medicine, West China Hospital, Sichuan University, Chengdu, China
Glucose metabolism is of critical importance for cell growth and proliferation, the disorders of which have been widely implicated in cancer progression. Glucose uptake is achieved differently by normal cells and cancer cells. Even in an aerobic environment, cancer cells tend to undergo metabolism through glycolysis rather than the oxidative phosphorylation pathway. Disordered metabolic syndrome is characterized by elevated levels of metabolites that can cause changes in the tumor microenvironment, thereby promoting tumor recurrence and metastasis. The activation of glycolysis-related proteins and transcription factors is involved in the regulation of cellular glucose metabolism. Changes in glucose metabolism activity are closely related to activation of protein kinase B (PKB/AKT). This review discusses recent findings on the regulation of glucose metabolism by AKT in tumors. Furthermore, the review summarizes the potential importance of AKT in the regulation of each process throughout glucose metabolism to provide a theoretical basis for AKT as a target for cancers.
1 Introduction
Glucose is the main energy source for most cells and is also an important substrate for biochemical reactions in cells. Glucose metabolism plays a key role in cell growth and proliferation of cells (Palm and Thompson, 2017; Shao et al., 2018). Intracellular glucose metabolism mainly includes the following steps: glucose uptake, glycogenesis and activation of the tricarboxylic acid cycle (TCA) (Han et al., 2016; Ye et al., 2022). A disorder in glucose metabolism can lead to metabolic diseases, such as obesity and type II diabetes (Lu et al., 2021), and cardiovascular disease (Stemmer et al., 2019; Poznyak et al., 2022). Furthermore, in an aerobic environment, cancer cells tend to undergo metabolism through glycolysis rather than the oxidative phosphorylation pathway (Abdel-Wahab et al., 2019). The phenomenon is called the Warburg effect (Wolf et al., 2010; Koppenol et al., 2011; Liberti and Locasale, 2016). Cancer cells can achieve this change in energy metabolism via increased activity of glucose transporters located on cancer cell membranes and enhanced activity of glycolytic enzymes (Zhou et al., 2008; Demasi et al., 2010). Thus, research evaluating mechanisms of glucose metabolism is helpful to identify feasible and effective therapy for the treatment of cancers.
AKT was originally identified and cloned from an AKT8 transforming retrovirus (Staal, 1987). Furthermore, AKT is also known as protein kinase B (PKB), considering its similarity with PKA and PKC (Coffer and Woodgett, 1991). Three subtypes of AKT are expressed has in mammals, namely AKT1, AKT2, and AKT3 (Vanhaesebroeck and Alessi, 2000; Siddika et al., 2022). AKT1 is activated and hyperphosphorylated in most human cancers by at two key regulatory sites (Thr308 and Ser473) (Xing et al., 2019). AKT1 is located in the cytoplasm and is essential for normal systemic growth (Cho et al., 2001). AKT2 is co-localized with mitochondria and is a major essential subtype involved in glucose metabolism (Toulany and Rodemann, 2015). AKT3 is located in the nucleus and nuclear membrane, and is crucial to achieve normal brain size (Easton et al., 2005). Phosphorylation of AKT can regulate the activity of downstream target proteins, which play an important role in controlling various important cellular functions such as cell apoptosis, glucose metabolism (Hua et al., 2021).
To date, it has been suggested that the AKT kinase can be modified though post-translational modifications (PTMs), including phosphorylation, ubiquitination, acetylation, methylation and hydroxylation (Guo et al., 2019). Phosphorylation has been extensively studied. A recent study extended the EPL technique to introduce a wider range of PTMs into AKT, an found that O-GlcNAcylation at Ser473 and phosphorylation at Tyr474 can activate the AKT activity toward peptide and protein substrates (Salguero et al., 2022). Ubiquitination, is a type of PTMs, the changed biological processes in tumor metabolism. The ubiquitination of AKT significantly regulates the activity of the mTORC1, AMPK and PTEN signaling pathways (Deng et al., 2020). In addition, the research determined that CHIP, MULAN, and TTC3 can ubiquitinate AKT and mediate its degradation (Guo, et al., 2019). The Lysine Demethylase 4B (KDM4B) could interact with TRAF6 and promote ubiquitination of AKT for activation, thereby promoting glucose metabolism in colorectal cancer cell (Li et al., 2020). DNA methylation is one of the most extensively studied and fully characterized epigenetic modifications. Emerging evidence demonstrated that protein arginine methyltransferases 5(PRMT5) directly methylates AKT1-R391 to promote AKT kinase activity (Yin et al., 2021). In addition, SETDB1-mediated methylation of AKT1 at K140 in cells, which promotes cell growth and glycolysis through AKT methylation (Figure Figure1). We should in-depth explore of the PTM functions of the AKT to develop novel strategies prevention of cancers.
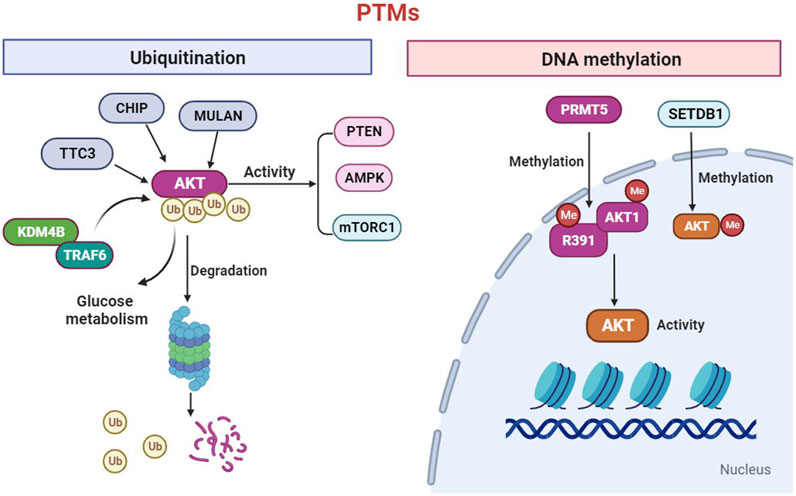
FIGURE 1. AKT can be modified through post-translational modifications (PTMs) including ubiquitination and methylation. The ubiquitination of AKT significantly regulates the activity of the mTORC1, AMPK and PTEN signaling. In addition, the CHIP, MULAN, Lysine Demethylase 4B (KDM4B) and TTC3 can ubiquitinate AKT and mediate its degradation. Furthermore, protein arginine methyltransferases 5(PRMT5) directly methylates AKT1-R391 to promote AKT activity. In addition, SETDB1-mediated methylation of AKT1 which promotes cell growth and glycolysis.
Therefore, AKT is considered an effective target for cancer treatment. In this paper, we review the current knowledge about AKT signaling. We also discuss our current understanding of how AKT regulates glucose metabolism, including uptake, storage, and catabolism. This overview may shed new light on how to treat cancer by exploiting AKT as a molecular target for glucose metabolism.
2 AKT and glucose uptake
Glucose is the main form in which dietary sugars are made available to cells of the human body, and its breakdown is a major source of energy for almost all cells (Palm and Thompson, 2017). Glucose utilization begins with its uptake by cells through a glucose transporter. Glucose molecules are polar and bulky and cannot easily diffuse through the lipid membrane of cells (Navale and Paranjape, 2016). Glucose transporters comprise a wide range of membrane proteins that promote glucose transport across cell membranes (Han et al., 2018). Because glucose is an important source of energy for all life, these transporters are present in almost all cells. It mainly includes two types of transporters: sodium glucose linked transporters (SGLT) and facilitated diffusion glucose transporters (GLUTs) (Duelli and Kuschinsky, 2001; Hsu and Dzhagalov, 2019; Wang L. et al., 2022). GLUTs are integral membrane proteins containing 12 transmembrane helices with amino and carboxy termini exposed to the cytoplasmic side of the plasma membrane (Oka et al., 1990; Hruz and Mueckler, 2001; Chen and Russo, 2012). According to a model of alternative conformation, the GLUT protein transports glucose, expose an individual substrate binding site outside or inside of the cell. The binding of glucose to a site triggers conformational changes related to transport and glucose is released to the other side of the membrane (Hebert and Carruthers, 1992; Cloherty et al., 1995; Chen and Russo, 2012). Three subclasses of facilitative transporters have been identified (Bell et al., 1990; Thorens and Mueckler, 2010; Navale and Paranjape, 2016): Class I facilitative glucose transporters (GLUT1 to GLUT4) (Garvey et al., 1998; Godoy et al., 2006), Class Ⅱ facilitative glucose transporters (GLUT5, GLUT7, GLUT9, and GLUT11) (Li et al., 2004; Godoy, et al., 2006), and Class Ⅲ facilitative glucose transporters (GLUT6, GLUT8, GLUT10, GLUT12, and GLUT13) (Schmidt et al., 2009; Waller et al., 2013). The expression levels of GLUTs are regulated by PKB/AKT (Kupriyanova and Kandror, 1999; Kilic et al., 2007). After ingesting sugary substances, the blood glucose level is significantly increased, correspondingly, and insulin is secreted into the blood by the islets, which reduces the blood glucose level. In a physiological state, the GLUTs are sequestered into intracellular vesicles resulting in a low level of GLUTs at the cell surface (Bryant et al., 2002; Watson et al., 2004; Ramm and James, 2005). When blood sugar levels increase, insulin induces translocation of glucose transporter (e.g., GLUT1 and GLUT4) to the cell membrane, which activates glucose transport across the cell membrane, AKT is involved in this process (Guo et al., 2021). Knockdown of AKT or inhibition of AKT activation by LY294002 can negatively regulate the expression of GLUT1 on the plasma membrane (Lou et al., 2022). In contract AKT activation, induced by arsenic trioxide can promote cell proliferation by increasing GLUT1 expression that improves glucose uptake (Lou, et al., 2022). Furthermore, Zhang et al. found that activation of AKT/mTORC1/NF-κB signaling upregulated of GLUT1 transcription in nasopharyngeal carcinoma cells (Zhang et al., 2017). In contrast, inhibition of AKT activity by LY294002, a PI3 kinase (PI3K) inhibitor, abolishes insulin-induced induction of GLUT1 mRNA in Hepa1c1c7 cells (Barthel et al., 1999). Yu et al. determined that pretreatment with MK2206 (an AKT inhibitor) significantly reduced the effect of NRG-1β on glucose uptake and translocation of GLUT4 to the plasma membrane (Yu M. et al., 2022). In addition, hypoxia inducible factor-1α (HIF-1α), as a downstream target of AKT/mTORC1, has been reported to confer resistance to apoptosis under hypoxic conditions in rhabdomyosarcoma and Ewing sarcoma cells by increasing the expression of the glucose transporter GLUT1 (Fulda and Debatin, 2007; Kilic, et al., 2007). Based on these findings, we believe that AKT controls the transcription level of GLUTs by regulating transcription factors. In addition to affecting the transcription level of GLUTs, AKT has also been reported to be involved in translocation of GLUTs to the plasma membrane in response to insulin (DeFronzo et al., 2015). Studies have shown that AKT is associated with GLUT4-containing vesicles in 3T3-L1 adipocytes and rat cardiac ventricular muscle after insulin treatment (Kupriyanova and Kandror, 1999; Kessler et al., 2000; Hajduch et al., 2001), activated AKT phosphorylation is involved in the translocation to the plasma membrane of GLUT4 (Sasaki et al., 2009; Tan et al., 2012). The phosphorylation of the 160 kDa AKT substrate (AS160) can promote the transport of insulin-induced glucose transporter GLUT4 to the plasma membrane of adipocytes (Tan, et al., 2012). The phosphorylation of AS160 reverses the non-phosphorylated AS160 and GLUT4 vesicles, and promotes GLUT4 translocation (Tan, et al., 2012).
As a substrate for AKT, AS160 is phosphorylated by AKT at Ser-588 and Thr-642 sites in adipocytes (Kane et al., 2002). The role of AS160 in insulin-induced GLUT4 trafficking could be summarized as follows. Firstly, most GLUT4 is in intracellular storage vesicles (Bryant, et al., 2002; Watson, et al., 2004; Ramm and James, 2005). AS160 promotes hydrolysis of Rabs GTP to GDP on the GLUT4 storage vesicles so that Rabs in their inactive GDP-bound state inhibit GLUT4 exocytosis (Ishikura et al., 2007; Sano et al., 2007). Second, after phosphorylation under insulin stimulation, AS160 will bind to 14-3-3 proteins, which interact with phosphoserine or phosphothreonine residues in a variety of proteins to regulate several functions including subcellular redistribution, altered protein conformation, and impaired interaction with other proteins. AS160 dissociates from GLUT4 storage vesicles (Peck et al., 2006; Ramm et al., 2006; Geraghty et al., 2007). Thus, Rabs are loaded with GTP and promote the translocation of GLUT4 to the cell membrane, thus mediating the influx of glucose. Furthermore, TBC1D1 (tre-2/USP6, BUB2), phosphodylinositols (PI) and TXNIP (thioredoxin-interacting protein) also participate in AKT-regulated translocation of GLUT4 to the cell membrane (Taylor et al., 2008; Tan, et al., 2012). TBC1D1 is a member of the TBC1 Rab-GTPase family and may be involved in the regulation of GLUT4 translocation in skeletal muscle (An et al., 2010; Middelbeek et al., 2013; de Wendt et al., 2021). TBC1D1 and AS160 share 47% similarity, and have some structural features in common (Roach et al., 2007). TBC1D1 is also phosphorylated by AKT at the Thr-590 site and the role of TBC1D1 in insulin-induced GLUT4 translocation is similar to AS160 (Middelbeek, et al., 2013). PI3K is a relatively transient class of membrane phospholipids, which play a key role in the regulation of cell and organ functions, including signal transduction, ion channel gating, and vesicle movement (Tewari et al., 2022). PI3K is embedded in the lipid layer to regulate the formation, translocation, and anchoring of the GLUT4 vesicle (Chen et al., 2019; Liu et al., 2020). Phosphatidylinositol 3-phosphate 5-kinase (PIKfyve) is mainly responsible for catalyzing the phosphorylation of PI(3)P to PI(5)P and PI(3,5)P2 (Shisheva, 2008b; Rodgers et al., 2022). PI(3,5)P2 is present in GLUT4 vesicles and may regulate the transport of GLUT4 vesicles (Shisheva, 2008a). The study by Berwick et al. showed that serine 318 phosphorylation of PIKfyve (the substrate of AKT) promotes the trafficking of GLUT4 vesicles (Berwick et al., 2004). TXNIP is a type of α-arrestin protein that plays a role in the regulation of glucose and lipid metabolism (Bodnar et al., 2002; Sheth et al., 2005; Waldhart et al., 2017). Insulin stimulation can force TXNIP to separate from GLUT4 and inhibit the endocytosis of GLUT4, and ultimately improve glucose uptake (Liao et al., 2022). Waldhart et al. found that TXNIP acted as a substrate of AKT, activated AKT phosphorylation of TXNIP at Ser-308, and forced TXNIP to dissociate from the transporters (Waldhart, et al., 2017). Furthermore, TXNIP may inhibit AKT activity through a direct interaction with the PH domain of AKT under glucose stress (Huy et al., 2018). Therefore, insulin stimulation may induce glucose uptake in cells by activating AKT-mediated TXNIP phosphorylation, forcing TXNIP to dissociate from the transporters, thus inhibiting the endocytosis of the glucose transporter GLUT1 (Waldhart, et al., 2017).
In conclusion, AKT could control glucose uptake by regulating the transcription and localization of GLUTs to the cell membrane (Figure 2).
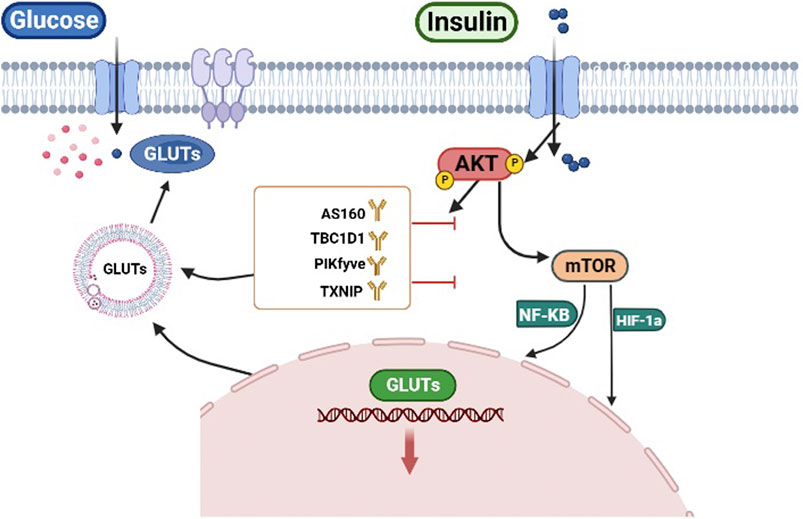
FIGURE 2. AKT regulates glucose uptake. AKT regulates the transcription of GLUTs by HIF-1α and NF-κB. Conversely AKT can directly phosphorylate targets (AS160, TBC1D1, PIKfyve, TXNIP) to regulate GLUTs trafficking.
3 AKT and glycogenesis
Glycogen is a branched chain polymer of glucose, which can be used as the main energy storage of eukaryotes (Tang et al., 2020; Marr et al., 2022). Glycogenesis is a process of glycogen synthesis, in which glucose molecules bind to the glycogen chain for storage (Bandyopadhyay et al., 2022; Semrau et al., 2022). In mammals, glucose is deposited mainly on skeletal muscle and liver, and a little glucose is deposited on other tissues, including the kidneys, heart, fat, and brain (Roach et al., 2012). Glycogen synthase (GS) is a rate limiting enzyme in glycogenesis, and as such its activity is controlled by phosphorylation (inactivation) and dephosphorylation (activity) cycles (Li Q. et al., 2019; Marr, et al., 2022). Glycogen synthase kinase 3 (GSK-3) is an evolutionarily conserved intracellular serine/threonine kinase, which can inhibit GS and regulate glucose metabolism through phosphorylation (Wang N. et al., 2022). Under insulin stimulation, GSK3 is phosphorylated and inactivated by AKT, leading to inhibition of GS phosphorylation (Li T. et al., 2019). However, in primary hepatocytes treated with GlcN, exposure to LY294002 or MK2206 abolished the activity of irisin in promoting GSK3 phosphorylation and reducing GS phosphorylation (Liu H. et al., 2015). Furthermore, many cell signaling pathways have been shown to be related to signal transduction, such as the PI3K/Akt, Wnt, MAPK, and TGF-β signaling pathways (McCubrey et al., 2014; Ngeow et al., 2018; Duda et al., 2020; Papadopoli et al., 2021). Two GSK-3 isoforms exist in mammalian cell, GSK-3α and GSK-3β (Beurel et al., 2015; Okada et al., 2004; von Wilamowitz-Moellendorff et al., 2013), and inhibition of GSK-3 activity by insulin may be due to AKT-catalyzed phosphorylation of an N-terminal serine residue (Ser-21 in GSK-3α and Ser-9 in GSK-3β) (Cross et al., 1995; Li et al., 2010). Studies have shown that PI3K/AKT/GSK3β signaling pathways mediate glycogenesis in the liver (Valicherla et al., 2019). Therefore, after insulin activates AKT, AKT may in turn regulate blood glucose levels by promoting glucose uptake by regulating GLUTs, although AKT promotes glycogen synthesis, or by inhibiting GSK3 activity to activate GS activity. In conclusion, AKT could control glycogen synthesis by regulating GSK3 activity.
4 AKT and glycolysis
After glucose is transported into cells, it is further catabolized to pyruvate by glycolysis (Ganapathy-Kanniappan and Geschwind, 2013; Liu et al., 2021). Multiple enzymes are involved in this process, and AKT regulates these enzymes in a variety of ways. AKT could regulate the transcriptions of glycolysis enzymes via the activation of several transcription factors, such as FoxO, c-Myc, and HIF (Du et al., 2014; Daher et al., 2021; Pan et al., 2022).
4.1 FoxOs
The FoxO Forkhead transcription factors are highly conserved, and are regulated and controlled by the insulin signaling pathway (Nakae et al., 2008; Lee and Dong, 2017; Bhardwaj et al., 2021). FoxOs consist of FoxO1, FoxO3, FoxO4, and FoxO6, which regulate a series of cellular processes including proliferation, apoptosis, and cell metabolism (Tzivion et al., 2011; Klotz et al., 2015). FoxOs play an important role in glycogenolysis by insulin signaling and are located downstream of AKT (Sergi et al., 2019). The transcriptional functions of FoxOs are regulated by their nucleocytoplasmic transport. Under insulin stimulation, AKT is activated and phosphorylates FoxOs, which leads to the export of FoxOs to the cytoplasm (Biggs et al., 1999; Brunet et al., 1999; Kops and Burgering, 1999; Kim et al., 2018). Furthermore. PKB/Akt-dependent phosphorylation of FoxOs transcription factors favors their sequestration in complex with 14-3-3 proteins in the cytoplasm (Rena et al., 2001). FoxO1, FoxO3 and FoxO4 contain three AKT phosphorylation sites, respectively, while FoxO6 contains two. For example, the three sites of murine FoxO1 are Thr24, Ser253 and Ser316 (Nakae, et al., 2008). In murine hematopoietic stem cells (HSCs), the conditional deletion of cited2 resulted in decreased glycolysis; however, inhibition of PI3/Akt (LY294002) partially rescued FoxO phosphorylation and promoted glycolysis (Du, et al., 2014). The transcription of hexokinase (HK) and pyruvate kinase (PK) are repressed by FoxOs activation (Shiau et al., 2022), thus AKT promotes transcription of glycolytic enzymes by inhibiting the nuclear localization of FoxOs (Haeusler et al., 2010; Zhang et al., 2012; Knight et al., 2014; Lien et al., 2016).
4.2 c-Myc
c-Myc is a key transcription factor and plays an important role in many physiological processes such as cell growth, apoptosis, and energy metabolism (Cramer and Schmitt, 2016; Allen-Petersen and Sears, 2019). Multiple regulatory mechanisms are mediated by AKT to regulate c-Myc activity (Cramer and Schmitt, 2016). For example, AKT controls the stability of the c-Myc protein by GSK3β, which in turn phosphorylates c-Myc at Thr58 and promotes its subsequent ubiquitination by the SCF ubiquitin ligase (Gregory et al., 2003; Welcker et al., 2004). AKT inactivation could result in down-regulation of the post-translational expression of Pim-1 and c-Myc protein. Post-translational down-regulation of c-Myc has been reported to occur through T58 phosphorylation caused by GSK-3 inactivation of GSK-3β (Scarpa et al., 2021). c-Myc is translocated into the nucleus to upregulate the transcription of glycolysis enzymes, including HK, G-6-PI and phosphofructokinase (Yang et al., 2018; Yu Q. et al., 2022). In hepatocellular carcinoma cells, the AKT inhibitor (LY294002) was found to reduce the expression of c-Myc, HK2 and PKM2, further inhibiting glycolysis (Shin et al., 2021). Thus, AKT promotes the transcription of glycolysis enzymes by c-Myc.
4.3 HIF
The HIF family is activated in response to hypoxia and consists of three members, HIF1, HIF2, and HIF3 (Cramer and Schmitt, 2016; Catrina and Zheng, 2021; Knutson et al., 2021). HIFs direct glucose away from oxidative phosphorylation toward glycolysis and lactate production (Kierans and Taylor, 2021; Knutson, et al., 2021). The PI3K/AKT pathway has been demonstrated to be involved in regulating HIF-1α expression and transcriptional under hypoxic conditions (Xie et al., 2019; Kierans and Taylor, 2021). In esophageal carcinoma cell lines, hypoxic conditions result in a upregulation of HIF-1α protein and glycolytic enzyme expression, whose expression can be reversed using the PI3K/AKT signal inhibitor wortmannin (Zeng et al., 2016; Kierans and Taylor, 2021). Although the above research indicates that the PI3K/AKT signaling pathway can affect the stability of HIF-1 to regulate glucose metabolism, further research is needed to determine the extent to which the PI3K/AKT signaling pathway is involved in HIF-dependent glucose metabolism.
4.4 Glycolytic enzymes
In addition to regulating the transcription of glycolytic enzymes, AKT is involved in the regulation of the phosphorylation status of multiple glycolytic enzymes and their activity. HK catalyzes the first step of glycolysis by phosphorylating glucose to form glucose 6-phosphate (G-6-P) (Rathmell et al., 2003; Deshmukh et al., 2016). AKT promotes the association of HK1 and HK2 with mitochondria to increase HK activity and directs G6P toward glycolysis (Gottlob et al., 2001; John et al., 2011). AKT directly phosphorylates HK2 at Thr473 to promote mitochondrial association of HK2 mitochondrial association (Yu et al., 2019). AKT also inhibits GSK-3β and indirectly promotes mitochondrial binding to HK2, while also phosphorylating VDAC and inhibiting its ability to bind to HK2 (Pastorino et al., 2005; Cramer and Schmitt, 2016). In the glycolytic pathway, phosphofructokinase 1 (PFK1) catalyzes a rate-limiting step of glycolysis by converting fructose 6-phosphate to fructose 1,6-bisphosphate (Li et al., 2017; Feng et al., 2020). The platelet isoform PFK1 (PFKP) is the predominant isoform of PFK1 (Lee et al., 2017). Lee et al. showed that AKT phosphorylates PFKP at Ser386, and this phosphorylation inhibits PFKP degradation of PFKP (Lee, et al., 2017). Additionally, AKT can phosphorylate the glycolytic activator (PFK-2/FBPase-2), resulting in increased PFK2 activity (Li, et al., 2017; Simon-Molas et al., 2018). Glyceraldehyde-3-phosphate dehydrogenase (GAPDH) is a glycolytic enzyme found in almost all tissues (Tristan et al., 2011; Zhang et al., 2015). AKT may be involved in the regulation of the enzymatic activity of GAPDH. In the HuCCT1 tumor, GAPDH was demonstrated to be a downstream signaling molecule of the Hic-5/Sec/AKT signaling pathway that participated in HuCCT1 cell migration (Wu WS. et al., 2022). However, the specific mechanism involved in AKT regulation of GAPDH activity needs further study. Phosphate glycerate kinase (PGK) catalyzes the reversible reaction of 1,3-diphosphate glyceride (1,3-BPG) with ADP in the glycolytic pathway, forming 3-phosphate glyceride (3-PG) adenosine triphosphate (ATP) (Wang et al., 2007; Wang et al., 2015). A recent study demonstrated that increased expression of PGK1 promoted glycolysis and enhanced the progression of epithelial mesenchymal transition (EMT) in oral squamous cell carcinoma (OSCC) cells (Zhang et al., 2020; Marcucci and Rumio, 2022). PGK1 activity can be inhibited by acetylation at lysine 220 (Wang, et al., 2015). HDAC3 is responsible for the deacetylation of PGK1 to increase PGK1 activity. The AKT/mTORC1 pathway controls HDAC3 S424 phosphorylation to regulate its activity. Hence, AKT indirectly activates PGK1 by HDAC3-mediated PGK1 deacetylation. Pyruvate kinase (PK) is the enzyme that catalyzes the glycolysis process, and consists of four isozymes in vertebrates: PKL, PKR, PKM1, and PKM2 (Chaneton and Gottlieb, 2012). Pyruvate kinase muscle type 2 (PKM2) has been shown to promote cancer cell metabolism and growth (Li Q. et al, 2019). PKM2 is regulated by phosphorylation, which leads to the translocation from the cytoplasm to nucleus (Luo et al., 2011; Yang et al., 2012). PKM2 activity may be inhibited after glycolysis phosphorylation. For example, Hitosugi et al. demonstrated that the fibroblast growth factor receptor 1 (FGFR1) induced the phosphorylation of PKM2 at Tyr105, leading to a decrease in lactate production (Park et al., 2016). Meanwhile, PKM2 has also been reported to be a substrate of AKT (Hitosugi et al., 2009). Park et al. showed that AKT can directly interact with PKM2 and phosphorylate it at Ser202, which is crucial for nuclear translocation of the PKM2 protein under stimulation by insulin-like growth factor 1 (IGF-1) (Park, et al., 2016). The increase in nuclear localization of PKM2 is consistent with the increased expression of GLUT1, HK2, and HIF-1α and glucose entrapment (Salani et al., 2015). This suggests that after transfer of PKM2 to the nucleus, AKT is involved in the regulation of glucose intake.
In conclusion, AKT could control glycolysis by regulating the activity of multiple glycolytic enzymes (Figure 3).
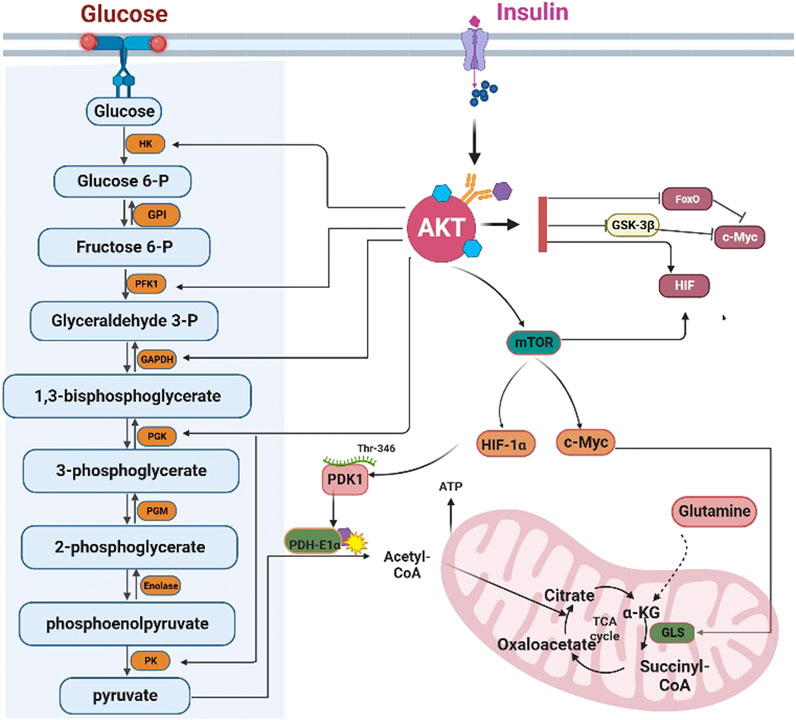
FIGURE 3. AKT regulates glycolysis and the tricarboxylic acid cycle. AKT regulates the transcription of glycolysis enzymes by FoxOs, c-Myc, and HIF-1α. However, AKT can directly phosphorylate the glycolytic enzymes HK, PFK1, GAPDH, PGK, and PK. HK: Hexokinase; GPI: Glucose 6-phosphate isomerase; PFK1 phosphofructokinase 1; TPI triose phosphate isomerase; GAPDH, Glyceraldehyde 3-phosphate dehydrogenase; PGK: phosphoglycerate kinase; PGM: Phosphoglycerate mutase; PK: Pyruvate kinase. AKT can inhibit the influx of acetyl-CoA into the TCA cycle by inhibiting PDH-E1αactivity. Furthermore, AKT can promote the conversion of glutamine into α-ketoglutarate to compensate for the depletion of the intermediates of the TCA cycle. GLS: Glutaminase, α-KG: α-ketoglutarate.
5 AKT and the tricarboxylic acid cycle
The tricarboxylic acid (TCA) cycle is a significant link in glucose metabolism. Pyruvate is transferred into mitochondria and further converted into acetyl-CoA, which is oxidized to ATP and carbon dioxide through TCA cycle (Bowtell et al., 2007; Arnold et al., 2022). Pyruvate from glycolysis enters the TCA cycle through the action of the pyruvate dehydrogenase complex (PDC), which is a compound of three enzymes that converts pyruvate into acetyl-CoA through decarboxylation of pyruvate (Patel et al., 2014; Golias et al., 2019; Lima-Silva et al., 2022). Pyruvate dehydrogenase (PDH) E1α phosphorylation of subunits can inhibit PDC activity (Patel and Korotchkina, 2006; Cerniglia et al., 2015; Sharma et al., 2019). Therefore, phosphorylation of PDH-E1α phosphorylation suppresses the entry of pyruvate into the TCA. Phosphorylation of PDH-E1α is regulated by pyruvate dehydrogenase kinase (PDK) (Sharma, et al., 2019). AKT can regulate PDK activity to further control PDH-E1α activity. As mentioned above, the HIF transcription factors are regulated by AKT/mTORC1 signaling and Kim et al. demonstrated that PDK1 was a direct HIF-1α target gene (Kim et al., 2006); thus, AKT can indirectly regulate the PDH-E1α activity via the HIF-1α/PDK1 axis. Conversely, AKT can phosphorylate PDK1 at Thr-346 during hypoxia, inactivating pyruvate dehydrogenase (Chae et al., 2016). Based on the above, AKT has an impact the influx of acetyl-CoA into the TCA cycle by inhibiting PDC activity.
The TCA cycle is a key metabolic pathway that connects carbohydrate, fat, and protein metabolism. The enzymes in the cyclic reaction can completely oxidize acetyl-CoA into two molecules each of carbon dioxide and water (Liu TY. et al., 2015; Ryan et al., 2021). 3-Methyladenine (3-MA) could lead to inhibition of AKT phosphorylation and inhibition of AKT phosphorylation can reduce the activities of TCA enzymes (Shaerzadeh et al., 2014). Activities of five enzymes in the TCA cycle including citrate synthase, aconitase, fumarase, a-ketoglutarate dehydrogenase, and malate dehydrogenase were reduced in the presence of 3-methyladenine, which inhibits the PI3K/AKT signaling pathway (Shaerzadeh, et al., 2014). However, the specific mechanism of AKT that regulates the activity of TCA enzymes needs further study. The TCA cycle provides intermediates for biosynthetic reactions such as amino acids, nucleotides, and lipids (Cramer and Schmitt, 2016). Due to the metabolic transformation of cancer cells into aerobic glycolysis, cancer cells must coordinate mechanisms to compensate for the consumption of intermediates of the TCA cycle intermediates (Cramer and Schmitt, 2016). In one major pathway, glutamine is converted into α-ketoglutarate (α-KG) and feeds into the TCA cycle (Fan et al., 2013; Hensley et al., 2013). c-Myc is a downstream gene of the AKT/mTORC1 pathway, and can up-regulate the transcription of glutamine transporters ASCT2 and SN2 to stimulate glutamine uptake (Wise et al., 2008; Li and Simon, 2013). The rate-limiting step for glutamine utilization is regulated by the enzyme glutaminase (GLS) (Mukha et al., 2021). GLS transforms glutamine into glutamate to support the TCA together with redox and epigenetic reactions (Johnson et al., 2018). Some studies have shown that GLS is a c-Myc target gene (Wise, et al., 2008; Gao et al., 2009; Li and Simon, 2013). Thus, the AKT/mTORC1 pathway can regulate the TCA cycle by promoting the conversion of glutamine into α-KG.
Overall, AKT controls the TCA cycle by regulating the influx of acetyl-CoA and α-KG into the TCA cycle.
6 Conclusion
In recent decades, there has been renewed interest in explaining how metabolism is altered in metabolic syndromes and cancers based on observations of signal transduction pathway molecules that regulate glucose metabolism (Cramer and Schmitt, 2016). The AKT signaling pathway is involved in the regulation of multiple processes, and researchers have recognized the importance of regulation and function of AKT signaling. Undoubtedly, AKT signaling is closely related to glucose metabolism and its manipulation and activation of its downstream molecules represent promising therapeutic targets for the treatment of metabolic syndromes and cancers. Furthermore, the AKT signaling pathway can be activated due to the expression of certain oncogenes or the omission of certain tumor suppressor genes (Ma et al., 2022). The overexpression of AKT has been demonstrated in many cancers, including ovarian cancer, lung cancer, and pancreatic cancer. At the same time, abnormal activation of AKT is related to increased cancer cell proliferation, survival, and drug resistance (Jacobsen et al., 2017; Ediriweera et al., 2019; Wu Y. et al., 2022; Gu et al., 2022).
Various Akt inhibitors with potential inhibitory functions are being investigated in preclinical studies. AKT inhibitors are classified according to their inhibition mechanisms and chemical scaffolds, and are classified as ATP competitive inhibitors (GSK690693, GDC0068 and AZD5363), allosteric inhibitors (pyranonaphthoquinone lactones), and irreversible inhibitors. Currently, AKT is considered a potential target for cancer treatment and prevention, and some AKT inhibitors have been clinically approved for the treatment of cancer (Chugh et al., 2008). The AKT inhibitor MK-2206 and LY2940002 has been shown to block glucose metabolism. Furthermore, the administration of competitive ATP inhibitors (GSK690693) in mice can significantly inhibit glycogen synthesis and peripheral glucose uptake (Crouthamel et al., 2009). Furthermore, many AKT inhibitors, including patasertib and capivasertib, exert anticancer activity in preclinical studies, and clinical trials are being advanced (Hua, et al., 2021). However, it has not been reported whether all AKT inhibitors are involved in regulating and inhibiting glucose metabolism pathways, except for MK-2206, LY2940002 and GSK690693. Thus, further in-depth research is warranted to investigate the regulatory effects of currently reported AKT inhibitors and to develop new inhibitors. AKT provides a novel target and the molecular mechanisms of AKT regulation in glucose metabolism should be exploited to improve cancer treatment. Small interfering RNA (siRNA) directed against AKT confirmed its impact on glucose metabolism. In HeLa lines, AKT-siRNA enhanced glucose uptake and HK2 expression, suggesting that inhibition of AKT may increase glucose uptake by detaching HK2 from the mitochondria (Neary and Pastorino 2013). Non-etheless, there is great heterogeneity in the energy metabolism of cancer cells, and even the same malignant tumor may exhibit differences in metabolism, which presents challenges to energy metabolism as a target for treatment. To avoid this phenomenon, AKT-specific inhibitors could be used in combination with metabolic target inhibitors, or dual inhibition can be achieved focusing on these targets to achieve better therapeutic properties (Song et al., 2019).
Author contributions
XiL: Writing–original draft, Writing–review and editing, Supervision. SH: Investigation, Supervision, Writing–review and editing. YC: Investigation, Supervision, Writing–review and editing. XuL: Software, Writing–review and editing. JL: Supervision, Writing–original draft, Writing–review and editing. TW: Writing–original draft, Writing–review and editing.
Funding
The author(s) declare that no financial support was received for the research, authorship, and/or publication of this article. This work was supported by grants of Sichuan Provincial Department of Health Project (No. Chuangan Yan 2022-105). This article is supported by the National Clinical Key Specialty Construction Project.
Conflict of interest
The authors declare that the research was conducted in the absence of any commercial or financial relationships that could be construed as a potential conflict of interest.
Publisher’s note
All claims expressed in this article are solely those of the authors and do not necessarily represent those of their affiliated organizations, or those of the publisher, the editors and the reviewers. Any product that may be evaluated in this article, or claim that may be made by its manufacturer, is not guaranteed or endorsed by the publisher.
Abbreviations
PKB/AKT, Protein kinase B; TCA, Tricarboxylic acid cycle; SGLT, Sodium glucose linked transporters; GLUTs, Glucose transporters; GSK-3, Glycogen synthase kinase 3; HK, Hexokinase; PK, Pyruvate kinase; G-6-P, Glucose 6-phosphate; PFK1, Phosphofructokinase 1; PGK, Phosphate glycerate kinase; ATP, Adenosine triphosphate; EMT, Epithelial mesenchymal transition; PDC, Pyruvate dehydrogenase complex; PDK, Pyruvate dehydrogenase kinase; 3-MA, 3-Methyladenine; PGM, phosphoglycerate mutase; PTMS, post-translational modifications; KDM4B, The Lysine Demethylase 4B; PRMTS, Protein arginine methyltransferases 5.
References
Abdel-Wahab A. F., Mahmoud W., Al-Harizy R. M. (2019). Targeting glucose metabolism to suppress cancer progression: prospective of anti-glycolytic cancer therapy. Pharmacol. Res. 150, 104511. Epub 20191031. doi:10.1016/j.phrs.2019.104511
Allen-Petersen B. L., Sears R. C. (2019). Mission possible: advances in MYC therapeutic targeting in cancer. BioDrugs. Oct. 33, 539–553. doi:10.1007/s40259-019-00370-5
An D., Toyoda T., Taylor E. B., Yu H., Fujii N., Hirshman M. F., et al. (2010). TBC1D1 regulates insulin- and contraction-induced glucose transport in mouse skeletal muscle. Diabetes. Jun 59, 1358–1365. Epub 20100318. doi:10.2337/db09-1266
Arnold P. K., Jackson B. T., Paras K. I., Brunner J. S., Hart M. L., Newsom O. J., et al. (2022). A non-canonical tricarboxylic acid cycle underlies cellular identity. Nat. Mar. 603, 477–481. Epub 20220309. doi:10.1038/s41586-022-04475-w
Bandyopadhyay G., Tang K., Webster N. J. G., van den Bogaart G., Mahata S. K. (2022). Catestatin induces glycogenesis by stimulating the phosphoinositide 3-kinase-AKT pathway. Acta Physiol. (Oxf). May 235, e13775. Epub 20220204. doi:10.1111/apha.13775
Barthel A., Okino S. T., Liao J., Nakatani K., Li J., Whitlock J. P., et al. (1999). Regulation of GLUT1 gene transcription by the serine/threonine kinase Akt1. J. Biol. Chem. 274, 20281–20286. doi:10.1074/jbc.274.29.20281
Bell G. I., Kayano T., Buse J. B., Burant C. F., Takeda J., Lin D., et al. (1990). Molecular biology of mammalian glucose transporters. Diabetes care. Mar. 13, 198–208. doi:10.2337/diacare.13.3.198
Berwick D. C., Dell G. C., Welsh G. I., Heesom K. J., Hers I., Fletcher L. M., et al. (2004). Protein kinase B phosphorylation of PIKfyve regulates the trafficking of GLUT4 vesicles. J. Cell Sci. 117, 5985–5993. doi:10.1242/jcs.01517
Beurel E., Grieco S. F., Jope R. S. (2015). Glycogen synthase kinase-3 (GSK3): regulation, actions, and diseases. Pharmacol. Ther. 148, 114–131. doi:10.1016/j.pharmthera.2014.11.016
Bhardwaj G., Penniman C. M., Jena J., Suarez Beltran P. A., Foster C., Poro K., et al. (2021). Insulin and IGF-1 receptors regulate complex I-dependent mitochondrial bioenergetics and supercomplexes via FoxOs in muscle. J. Clin. Invest. 15, 131. doi:10.1172/jci146415
Biggs W. H., Meisenhelder J., Hunter T., Cavenee W. K., Arden K. C. (1999). Protein kinase B/Akt-mediated phosphorylation promotes nuclear exclusion of the winged helix transcription factor FKHR1. Proc. Natl. Acad. Sci. U. S. A. 96, 7421–7426. doi:10.1073/pnas.96.13.7421
Bodnar J. S., Chatterjee A., Castellani L. W., Ross D. A., Ohmen J., Cavalcoli J., et al. (2002). Positional cloning of the combined hyperlipidemia gene Hyplip1. Nat. Genet. Jan. 30, 110–116. doi:10.1038/ng811
Bowtell J. L., Marwood S., Bruce M., Constantin-Teodosiu D., Greenhaff P. L. (2007). Tricarboxylic acid cycle intermediate pool size: functional importance for oxidative metabolism in exercising human skeletal muscle. Sports Med. 37, 1071–1088. doi:10.2165/00007256-200737120-00005
Brunet A., Bonni A., Zigmond M. J., Lin M. Z., Juo P., Hu L. S., et al. (1999). Akt promotes cell survival by phosphorylating and inhibiting a Forkhead transcription factor. Cell. Mar. 19 (96), 857–868. doi:10.1016/s0092-8674(00)80595-4
Bryant N. J., Govers R., James D. E. (2002). Regulated transport of the glucose transporter GLUT4. Nat. Rev. Mol. Cell Biol. 3, 267–277. doi:10.1038/nrm782
Catrina S. B., Zheng X. (2021). Hypoxia and hypoxia-inducible factors in diabetes and its complications. Diabetologia 64, 709–716. Epub 20210126. doi:10.1007/s00125-021-05380-z
Cerniglia G. J., Dey S., Gallagher-Colombo S. M., Daurio N. A., Tuttle S., Busch T. M., et al. (2015). The PI3K/akt pathway regulates oxygen metabolism via pyruvate dehydrogenase (PDH)-E1α phosphorylation. Mol. Cancer Ther. Aug 14, 1928–1938. Epub 20150520. doi:10.1158/1535-7163.MCT-14-0888
Chae Y. C., Vaira V., Caino M. C., Tang H. Y., Seo J. H., Kossenkov A. V., et al. (2016). Mitochondrial akt regulation of hypoxic tumor reprogramming. Cancer Cell 30, 257–272. doi:10.1016/j.ccell.2016.07.004
Chaneton B., Gottlieb E. (2012). Rocking cell metabolism: revised functions of the key glycolytic regulator PKM2 in cancer. Trends Biochem. Sci. 37, 309–316. doi:10.1016/j.tibs.2012.04.003
Chen J. Q., Russo J. (2012). Dysregulation of glucose transport, glycolysis, TCA cycle and glutaminolysis by oncogenes and tumor suppressors in cancer cells. Biochim. Biophys. Acta, 370–384. Epub 20120627. doi:10.1016/j.bbcan.2012.06.004
Chen T., Zhang Y., Liu Y., Zhu D., Yu J., Li G., et al. (2019). MiR-27a promotes insulin resistance and mediates glucose metabolism by targeting PPAR-γ-mediated PI3K/AKT signaling. Aging (Albany NY) 11, 7510–7524. Epub 20190928. doi:10.18632/aging.102263
Cho H., Thorvaldsen J. L., Chu Q., Feng F., Birnbaum M. J. (2001). Akt1/PKBalpha is required for normal growth but dispensable for maintenance of glucose homeostasis in mice. J. Biol. Chem. 276, 38349–38352. Epub 20010831. doi:10.1074/jbc.C100462200
Chugh P., Bradel-Tretheway B., Monteiro-Filho C. M., Planelles V., Maggirwar S. B., Dewhurst S., et al. (2008). Akt inhibitors as an HIV-1 infected macrophage-specific anti-viral therapy. Retrovirology 5 (5), 11. doi:10.1186/1742-4690-5-11
Cloherty E. K., Sultzman L. A., Zottola R. J., Carruthers A. (1995). Net sugar transport is a multistep process. Evidence for cytosolic sugar binding sites in erythrocytes. Biochem. Nov. 28 (34), 15395–15406. doi:10.1021/bi00047a002
Coffer P. J., Woodgett J. R. (1991). Molecular cloning and characterisation of a novel putative protein-serine kinase related to the cAMP-dependent and protein kinase C families. Eur. J. Biochem. 201, 475–481. doi:10.1111/j.1432-1033.1991.tb16305.x
Cramer T., Schmitt C. A. (2016). Metabolism in cancer. Recent results in cancer research Fortschritte der Krebsforschung Progrès dans les recherches sur le cancer.
Cross D. A., Alessi D. R., Cohen P., Andjelkovich M., Hemmings B. A. (1995). Inhibition of glycogen synthase kinase-3 by insulin mediated by protein kinase B. Nature 378, 785–789. doi:10.1038/378785a0
Crouthamel M. C., Kahana J. A., Korenchuk S., Zhang S. Y., Sundaresan G., Eberwein D. J., et al. (2009). Mechanism and management of AKT inhibitor-induced hyperglycemia. Clin. Cancer Res. 1 (15), 217–225. doi:10.1158/1078-0432.CCR-08-1253
Daher M., Basar R., Gokdemir E., Baran N., Uprety N., Nunez Cortes A. K., et al. (2021). Targeting a cytokine checkpoint enhances the fitness of armored cord blood CAR-NK cells. Blood 137, 624–636. doi:10.1182/blood.2020007748
DeFronzo R. A., Ferrannini E., Zimmet P., Alberti G. (2015). International textbook of diabetes mellitus. John Wiley & Sons.
Demasi A. P., Costa A. F., Altemani A., Furuse C., Araujo N. S., Araujo V. C. (2010). Glucose transporter protein 1 expression in mucoepidermoid carcinoma of salivary gland: correlation with grade of malignancy. Int. J. Exp. pathology 91, 107–113. doi:10.1111/j.1365-2613.2009.00702.x
Deng L., Meng T., Chen L., Wei W., Wang P. (2020). The role of ubiquitination in tumorigenesis and targeted drug discovery. Signal Transduct. Target Ther. 5 (5), 11. doi:10.1038/s41392-020-0107-0
Deshmukh A., Deshpande K., Arfuso F., Newsholme P., Dharmarajan A. (2016). Cancer stem cell metabolism: a potential target for cancer therapy. Mol. Cancer. Nov. 8 (15), 69. doi:10.1186/s12943-016-0555-x
de Wendt C., Espelage L., Eickelschulte S., Springer C., Toska L., Scheel A., et al. (2021). Contraction-mediated glucose transport in skeletal muscle is regulated by a framework of AMPK, tbc1d1/4, and Rac1. Diabetes 70, 2796–2809. doi:10.2337/db21-0587
Du J., Li Q., Tang F., Puchowitz M. A., Fujioka H., Dunwoodie S. L., et al. (2014). Cited2 is required for the maintenance of glycolytic metabolism in adult hematopoietic stem cells. Stem Cells Dev. Jan. 15 (23), 83–94. doi:10.1089/scd.2013.0370
Duda P., Akula S. M., Abrams S. L., Steelman L. S., Martelli A. M., Cocco L., et al. (2020). Targeting GSK3 and associated signaling pathways involved in cancer. Cells 9, 1110. doi:10.3390/cells9051110
Duelli R., Kuschinsky W. (2001). Brain glucose transporters: relationship to local energy demand. News physiological Sci. Int. J. physiology Prod. jointly by Int. Union Physiological Sci. Am. Physiological Soc. 16, 71–76. doi:10.1152/physiologyonline.2001.16.2.71
Easton R. M., Cho H., Roovers K., Shineman D. W., Mizrahi M., Forman M. S., et al. (2005). Role for Akt3/protein kinase Bgamma in attainment of normal brain size. Mol. Cell Biol. 25, 1869–1878. doi:10.1128/MCB.25.5.1869-1878.2005
Ediriweera M. K., Tennekoon K. H., Samarakoon S. R. (2019). Role of the PI3K/AKT/mTOR signaling pathway in ovarian cancer: biological and therapeutic significance. Semin. Cancer Biol. 59, 147–160. Epub 20190522. doi:10.1016/j.semcancer.2019.05.012
Fan J., Kamphorst J. J., Mathew R., Chung M. K., White E., Shlomi T., et al. (2013). Glutamine-driven oxidative phosphorylation is a major ATP source in transformed mammalian cells in both normoxia and hypoxia. Mol. Syst. Biol. 9, 712. doi:10.1038/msb.2013.65
Feng J., Li J., Wu L., Yu Q., Ji J., Wu J., et al. (2020). Emerging roles and the regulation of aerobic glycolysis in hepatocellular carcinoma. J. Exp. Clin. Cancer Res. 6 (39), 126. Epub 20200706. doi:10.1186/s13046-020-01629-4
Fulda S., Debatin K. M. (2007). HIF-1-regulated glucose metabolism: a key to apoptosis resistance? Cell Cycle 6 (6), 790–792. Epub 20070427. doi:10.4161/cc.6.7.4084
Ganapathy-Kanniappan S., Geschwind J. F. (2013). Tumor glycolysis as a target for cancer therapy: progress and prospects. Mol. Cancer 12, 152. doi:10.1186/1476-4598-12-152
Gao P., Tchernyshyov I., Chang T. C., Lee Y. S., Kita K., Ochi T., et al. (2009). c-Myc suppression of miR-23a/b enhances mitochondrial glutaminase expression and glutamine metabolism. Nature 458, 762–765. doi:10.1038/nature07823
Garvey W. T., Maianu L., Zhu J. H., Brechtel-Hook G., Wallace P., Baron A. D. (1998). Evidence for defects in the trafficking and translocation of GLUT4 glucose transporters in skeletal muscle as a cause of human insulin resistance. J. Clin. investigation 101, 2377–2386. doi:10.1172/JCI1557
Geraghty K. M., Chen S., Harthill J. E., Ibrahim A. F., Toth R., Morrice N. A., et al. (2007). Regulation of multisite phosphorylation and 14-3-3 binding of AS160 in response to IGF-1, EGF, PMA and AICAR. Biochem. J. 407, 231–241. doi:10.1042/BJ20070649
Godoy A., Ulloa V., Rodriguez F., Reinicke K., Yanez A. J., Garcia Mde L., et al. (2006). Differential subcellular distribution of glucose transporters GLUT1-6 and GLUT9 in human cancer: ultrastructural localization of GLUT1 and GLUT5 in breast tumor tissues. J. Cell. physiology 207, 614–627. doi:10.1002/jcp.20606
Golias T., Kery M., Radenkovic S., Papandreou I. (2019). Microenvironmental control of glucose metabolism in tumors by regulation of pyruvate dehydrogenase. Int. J. Cancer 144, 674–686. Epub 20181004. doi:10.1002/ijc.31812
Gottlob K., Majewski N., Kennedy S., Kandel E., Robey R. B., Hay N. (2001). Inhibition of early apoptotic events by Akt/PKB is dependent on the first committed step of glycolysis and mitochondrial hexokinase. Genes & Dev. 15, 1406–1418. doi:10.1101/gad.889901
Gregory M. A., Qi Y., Hann S. R. (2003). Phosphorylation by glycogen synthase kinase-3 controls c-myc proteolysis and subnuclear localization. J. Biol. Chem. 278, 51606–51612. doi:10.1074/jbc.M310722200
Gu J., Huang W., Wang X., Zhang J., Tao T., Zheng Y., et al. (2022). Hsa-miR-3178/RhoB/PI3K/Akt, a novel signaling pathway regulates ABC transporters to reverse gemcitabine resistance in pancreatic cancer. Mol. Cancer 21, 112. Epub 20220510. doi:10.1186/s12943-022-01587-9
Guo J., Dai X., Laurent B., Zheng N., Gan W., Zhang J., et al. (2019). AKT methylation by SETDB1 promotes AKT kinase activity and oncogenic functions. Nat. Cell Biol. 21, 226–237. Epub 20190128. doi:10.1038/s41556-018-0261-6
Guo X. H., Jiang S. S., Zhang L. L., Hu J., Edelbek D., Feng Y. Q., et al. (2021). Berberine exerts its antineoplastic effects by reversing the Warburg effect via downregulation of the Akt/mTOR/GLUT1 signaling pathway. Oncol. Rep., 46. Epub 20211013. doi:10.3892/or.2021.8204
Haeusler R. A., Kaestner K. H., Accili D. (2010). FoxOs function synergistically to promote glucose production. J. Biol. Chem. 285 (285), 35245–35248. doi:10.1074/jbc.C110.175851
Hajduch E., Litherland G. J., Hundal H. S. (2001). Protein kinase B (PKB/Akt)--a key regulator of glucose transport? FEBS Lett. 16 (492), 199–203. doi:10.1016/s0014-5793(01)02242-6
Han C. Y., Patten D. A., Richardson R. B., Harper M. E., Tsang B. K. (2018). Tumor metabolism regulating chemosensitivity in ovarian cancer. Genes Cancer 9, 155–175. doi:10.18632/genesandcancer.176
Han H. S., Kang G., Kim J. S., Choi B. H., Koo S. H. (2016). Regulation of glucose metabolism from a liver-centric perspective. Exp. Mol. Med. 48, e218. doi:10.1038/emm.2015.122
Hebert D. N., Carruthers A. (1992). Glucose transporter oligomeric structure determines transporter function. Reversible redox-dependent interconversions of tetrameric and dimeric GLUT1. J. Biol. Chem. 267 (267), 23829–23838. doi:10.1016/s0021-9258(18)35912-x
Hensley C. T., Wasti A. T., DeBerardinis R. J. (2013). Glutamine and cancer: cell biology, physiology, and clinical opportunities. J. Clin. investigation 123, 3678–3684. doi:10.1172/JCI69600
Hitosugi T., Kang S., Vander Heiden M. G., Chung T. W., Elf S., Lythgoe K., et al. (2009). Tyrosine phosphorylation inhibits PKM2 to promote the Warburg effect and tumor growth. Sci. Signal. 2 (2), ra73. doi:10.1126/scisignal.2000431
Hruz P. W., Mueckler M. M. (2001). Structural analysis of the GLUT1 facilitative glucose transporter (review). Mol. Membr. Biol. 18, 183–193. doi:10.1080/09687680110072140
Hsu C.-L., Dzhagalov I. L. (2019). Metabolite transporters—the gatekeepers for T cell metabolism. Immunometabolism 1. doi:10.20900/immunometab20190012
Hua H., Zhang H., Chen J., Wang J., Liu J., Jiang Y. (2021). Targeting Akt in cancer for precision therapy. J. Hematol. Oncol. 14, 128. Epub 20210821. doi:10.1186/s13045-021-01137-8
Huy H., Song H. Y., Kim M. J., Kim W. S., Kim D. O., Byun J. E., et al. (2018). TXNIP regulates AKT-mediated cellular senescence by direct interaction under glucose-mediated metabolic stress. Aging Cell 17, e12836. Epub 20180831. doi:10.1111/acel.12836
Ishikura S., Bilan P. J., Klip A. (2007). Rabs 8A and 14 are targets of the insulin-regulated Rab-GAP AS160 regulating GLUT4 traffic in muscle cells. Biochem. biophysical Res. Commun. 353, 1074–1079. doi:10.1016/j.bbrc.2006.12.140
Jacobsen K., Bertran-Alamillo J., Molina M. A., Teixidó C., Karachaliou N., Pedersen M. H., et al. (2017). Convergent Akt activation drives acquired EGFR inhibitor resistance in lung cancer. Nat. Commun. 8 (8), 410. Epub 20170904. doi:10.1038/s41467-017-00450-6
John S., Weiss J. N., Ribalet B. (2011). Subcellular localization of hexokinases I and II directs the metabolic fate of glucose. PloS one 6 (6), e17674. doi:10.1371/journal.pone.0017674
Johnson M. O., Wolf M. M., Madden M. Z., Andrejeva G., Sugiura A., Contreras D. C., et al. (2018). Distinct regulation of Th17 and Th1 cell differentiation by glutaminase-dependent metabolism. Cell 175, 1780–1795. Epub 20181101. doi:10.1016/j.cell.2018.10.001
Kane S., Sano H., Liu S. C., Asara J. M., Lane W. S., Garner C. C., et al. (2002). A method to identify serine kinase substrates. Akt phosphorylates a novel adipocyte protein with a Rab GTPase-activating protein (GAP) domain. J. Biol. Chem. 277, 22115–22118. doi:10.1074/jbc.C200198200
Kessler A., Tomas E., Immler D., Meyer H. E., Zorzano A., Eckel J. (2000). Rab11 is associated with GLUT4-containing vesicles and redistributes in response to insulin. Diabetologia 43, 1518–1527. doi:10.1007/s001250051563
Kierans S. J., Taylor C. T. (2021). Regulation of glycolysis by the hypoxia-inducible factor (HIF): implications for cellular physiology. J. Physiol. 599, 23–37. Epub 20201015. doi:10.1113/JP280572
Kilic M., Kasperczyk H., Fulda S., Debatin K. M. (2007). Role of hypoxia inducible factor-1 alpha in modulation of apoptosis resistance. Oncogene 29 (26), 2027–2038. doi:10.1038/sj.onc.1210008
Kim C. G., Lee H., Gupta N., Ramachandran S., Kaushik I., Srivastava S., et al. (2018). Role of Forkhead Box Class O proteins in cancer progression and metastasis. Semin. Cancer Biol. 50, 142–151. Epub 20170801. doi:10.1016/j.semcancer.2017.07.007
Kim J. W., Tchernyshyov I., Semenza G. L., Dang C. V. (2006). HIF-1-mediated expression of pyruvate dehydrogenase kinase: a metabolic switch required for cellular adaptation to hypoxia. Cell metab. 3, 177–185. doi:10.1016/j.cmet.2006.02.002
Klotz L. O., Sánchez-Ramos C., Prieto-Arroyo I., Urbánek P., Steinbrenner H., Monsalve M. (2015). Redox regulation of FoxO transcription factors. Redox Biol. 6, 51–72. Epub 20150703. doi:10.1016/j.redox.2015.06.019
Knight A. L., Yan X., Hamamichi S., Ajjuri R. R., Mazzulli J. R., Zhang M. W., et al. (2014). The glycolytic enzyme, GPI, is a functionally conserved modifier of dopaminergic neurodegeneration in Parkinson’s models. Cell Metab. 20, 145–157. Epub 20140529. doi:10.1016/j.cmet.2014.04.017
Knutson A. K., Williams A. L., Boisvert W. A., Shohet R. V. (2021). HIF in the heart: development, metabolism, ischemia, and atherosclerosis. J. Clin. Invest. 1, 131. doi:10.1172/jci137557
Koppenol W. H., Bounds P. L., Dang C. V. (2011). Otto Warburg’s contributions to current concepts of cancer metabolism. Nat. Rev. Cancer 11, 325–337. doi:10.1038/nrc3038
Kops G. J., Burgering B. M. (1999). Forkhead transcription factors: new insights into protein kinase B (c-akt) signaling. J. Mol. Med. 77, 656–665. doi:10.1007/s001099900050
Kupriyanova T. A., Kandror K. V. (1999). Akt-2 binds to Glut4-containing vesicles and phosphorylates their component proteins in response to insulin. J. Biol. Chem. 274, 1458–1464. doi:10.1074/jbc.274.3.1458
Lee J. H., Liu R., Li J., Zhang C., Wang Y., Cai Q., et al. (2017). Stabilization of phosphofructokinase 1 platelet isoform by AKT promotes tumorigenesis. Nat. Commun. 8 (8), 949. Epub 20171016. doi:10.1038/s41467-017-00906-9
Lee S., Dong H. H. (2017). FoxO integration of insulin signaling with glucose and lipid metabolism. J. Endocrinol. 233, R67-R79–R79. doi:10.1530/JOE-17-0002
Li B., Simon M. C. (2013). Molecular Pathways: targeting MYC-induced metabolic reprogramming and oncogenic stress in cancer. Clin. cancer Res. official J. Am. Assoc. Cancer Res. 19, 5835–5841. doi:10.1158/1078-0432.CCR-12-3629
Li H., Lan J., Wang G., Guo K., Han C., Li X., et al. (2020). KDM4B facilitates colorectal cancer growth and glucose metabolism by stimulating TRAF6-mediated AKT activation. J. Exp. Clin. Cancer Res. 13, 39. Epub 20200113. doi:10.1186/s13046-020-1522-3
Li L., Eid J. E., Paz A. C., Trent J. C. (2017). Metabolic enzymes in sarcomagenesis: progress toward biology and therapy. BioDrugs 31, 379–392. doi:10.1007/s40259-017-0237-2
Li Q., Manolescu A., Ritzel M., Yao S., Slugoski M., Young J. D., et al. (2004). Cloning and functional characterization of the human GLUT7 isoform SLC2A7 from the small intestine. Am. J. physiology Gastrointest. liver physiology 287, G236–G242. doi:10.1152/ajpgi.00396.2003
Li Q., Zhao Q., Zhang J., Zhou L., Zhang W., Chua B., et al. (2019a). The protein phosphatase 1 complex is a direct target of AKT that links insulin signaling to hepatic glycogen deposition. Cell Rep. 24 (28), 3406–3422. doi:10.1016/j.celrep.2019.08.066
Li T., Han J., Jia L., Hu X., Chen L., Wang Y. (2019b). PKM2 coordinates glycolysis with mitochondrial fusion and oxidative phosphorylation. Protein Cell 10, 583–594. Epub 20190318. doi:10.1007/s13238-019-0618-z
Li Y., Lu H., Huang Y., Xiao R., Cai X., He S., et al. (2010). Glycogen synthase kinases-3beta controls differentiation of malignant glioma cells. Int. J. Cancer 127, 1271–1282. doi:10.1002/ijc.25020
Liao W., Xu N., Zhang H., Liao W., Wang Y., Wang S., et al. (2022). Persistent high glucose induced EPB41L4A-AS1 inhibits glucose uptake via GCN5 mediating crotonylation and acetylation of histones and non-histones. Clin. Transl. Med. 12, e699. doi:10.1002/ctm2.699
Liberti M. V., Locasale J. W. (2016). The Warburg effect: how Does it benefit cancer cells? Trends in biochemical sciences. 41:211–218. doi:10.1016/j.tibs.2015.12.001
Lien E. C., Lyssiotis C. A., Cantley L. C. (2016). Metabolic reprogramming by the PI3K-Akt-mTOR pathway in cancer. Recent results cancer Res. Fortschritte der Krebsforschung Progres dans les recherches sur le cancer 207, 39–72. doi:10.1007/978-3-319-42118-6_3
Lima-Silva L. F., Lee J., Moraes-Vieira P. M. (2022). Soluble carrier transporters and mitochondria in the immunometabolic regulation of macrophages. Antioxid. Redox Signal 36, 906–919. Epub 20220104. doi:10.1089/ars.2021.0181
Liu H., Zhao X., Guo M., Liu H., Zheng Z. (2015a). Growth and metabolism of Beauveria bassiana spores and mycelia. BMC Microbiol. 19 (15), 267. Epub 20151119. doi:10.1186/s12866-015-0592-4
Liu J., Peng Y., Shi L., Wan L., Inuzuka H., Long J., et al. (2021). Skp2 dictates cell cycle-dependent metabolic oscillation between glycolysis and TCA cycle. Cell Res. 31, 80–93. Epub 20200715. doi:10.1038/s41422-020-0372-z
Liu T. Y., Shi C. X., Gao R., Sun H. J., Xiong X. Q., Ding L., et al. (2015b). Irisin inhibits hepatic gluconeogenesis and increases glycogen synthesis via the PI3K/Akt pathway in type 2 diabetic mice and hepatocytes. Clin. Sci. (Lond) 129, 839–850. Epub 20150713. doi:10.1042/CS20150009
Liu X. S., Zeng J., Yang Y. X., Qi C. L., Xiong T., Wu G. Z., et al. (2020). DRD4 mitigates myocardial ischemia/reperfusion injury in association with PI3K/AKT mediated glucose metabolism. Front. Pharmacol. 11, 619426. Epub 20210127. doi:10.3389/fphar.2020.619426
Lou Q., Zhang M., Yang Y., Gao Y. (2022). Low-dose arsenic trioxide enhances membrane-GLUT1 expression and glucose uptake via AKT activation to support L-02 cell aberrant proliferation. Toxicology 475, 153237. Epub 20220614. doi:10.1016/j.tox.2022.153237
Lu Q., Guo P., Liu A., Ares I., Martínez-Larrañaga M. R., Wang X., et al. (2021). The role of long noncoding RNA in lipid, cholesterol, and glucose metabolism and treatment of obesity syndrome. Med. Res. Rev. 41, 1751–1774. Epub 20201224. doi:10.1002/med.21775
Luo W., Hu H., Chang R., Zhong J., Knabel M., O’Meally R., et al. (2011). Pyruvate kinase M2 is a PHD3-stimulated coactivator for hypoxia-inducible factor 1 145:732–744. doi:10.1016/j.cell.2011.03.054
Ma C., Wu J., Wang L., Ji X., Wu Y., Miao L., et al. (2022). Discovery of clinical candidate NTQ1062 as a potent and bioavailable akt inhibitor for the treatment of human tumors. J. Med. Chem. 23 (65), 8144–8168. Epub 20220609. doi:10.1021/acs.jmedchem.2c00527
Marcucci F., Rumio C. (2022). Tumor cell glycolysis-at the crossroad of epithelial-mesenchymal transition and autophagy. Cells, 11. Epub 20220318. doi:10.3390/cells11061041
Marr L., Biswas D., Daly L. A., Browning C., Vial S. C. M., Maskell D. P., et al. (2022). Mechanism of glycogen synthase inactivation and interaction with glycogenin. Nat. Commun. 13 (13), 3372. Epub 20220611. doi:10.1038/s41467-022-31109-6
McCubrey J. A., Steelman L. S., Bertrand F. E., Davis N. M., Abrams S. L., Montalto G., et al. (2014). Multifaceted roles of GSK-3 and Wnt/β-catenin in hematopoiesis and leukemogenesis: opportunities for therapeutic intervention. Leukemia 28, 15–33. Epub 20130619. doi:10.1038/leu.2013.184
Middelbeek R. J., Chambers M. A., Tantiwong P., Treebak J. T., An D., Hirshman M. F., et al. (2013). Insulin stimulation regulates AS160 and TBC1D1 phosphorylation sites in human skeletal muscle. Nutr. diabetes 3, e74. doi:10.1038/nutd.2013.13
Mukha A., Kahya U., Linge A., Chen O., Löck S., Lukiyanchuk V., et al. (2021). GLS-driven glutamine catabolism contributes to prostate cancer radiosensitivity by regulating the redox state, stemness and ATG5-mediated autophagy. Theranostics 11, 7844–7868. Epub 20210626. doi:10.7150/thno.58655
Nakae J., Oki M., Cao Y. (2008). The FoxO transcription factors and metabolic regulation. FEBS Lett. 9 (582), 54–67. Epub 20071120. doi:10.1016/j.febslet.2007.11.025
Navale A. M., Paranjape A. N. (2016). Glucose transporters: physiological and pathological roles. Biophys. Rev. 8, 5–9. doi:10.1007/s12551-015-0186-2
Ngeow K. C., Friedrichsen H. J., Li L., Zeng Z., Andrews S., Volpon L., et al. (2018). BRAF/MAPK and GSK3 signaling converges to control MITF nuclear export. Proc. Natl. Acad. Sci. U. S. A. 11, E8668–e8677. Epub 20180827. doi:10.1073/pnas.1810498115
Oka Y., Asano T., Shibasaki Y., Lin J. L., Tsukuda K., Katagiri H., et al. (1990). C-terminal truncated glucose transporter is locked into an inward-facing form without transport activity. Nature 345, 550–553. doi:10.1038/345550a0
Okada S., Crosson S., Mori M., Saltiel A. R., Pessin J. E. (2004). Insulin action, post-receptor mechanisms. Encyclopedia of endocrine diseases, 14–22.
Palm W., Thompson C. B. (2017). Nutrient acquisition strategies of mammalian cells. Nature 546 (546), 234–242. doi:10.1038/nature22379
Pan T., Sun S., Chen Y., Tian R., Chen E., Tan R., et al. (2022). Immune effects of PI3K/Akt/HIF-1α-regulated glycolysis in polymorphonuclear neutrophils during sepsis. Crit. Care 26 (26), 29. Epub 20220128. doi:10.1186/s13054-022-03893-6
Papadopoli D., Pollak M., Topisirovic I. (2021). The role of GSK3 in metabolic pathway perturbations in cancer. Biochim. Biophys. Acta Mol. Cell Res. 1868, 119059. Epub 20210512. doi:10.1016/j.bbamcr.2021.119059
Park Y. S., Kim D. J., Koo H., Jang S. H., You Y. M., Cho J. H., et al. (2016). AKT-induced PKM2 phosphorylation signals for IGF-1-stimulated cancer cell growth. Oncotarget 26 (7), 48155–48167. doi:10.18632/oncotarget.10179
Pastorino J. G., Hoek J. B., Shulga N. (2005). Activation of glycogen synthase kinase 3beta disrupts the binding of hexokinase II to mitochondria by phosphorylating voltage-dependent anion channel and potentiates chemotherapy-induced cytotoxicity. Cancer Res. 65 (65), 10545–10554. doi:10.1158/0008-5472.CAN-05-1925
Patel M. S., Korotchkina L. G. (2006). Regulation of the pyruvate dehydrogenase complex. Biochem. Soc. Trans. 34, 217–222. doi:10.1042/BST20060217
Patel M. S., Nemeria N. S., Furey W., Jordan F. (2014). The pyruvate dehydrogenase complexes: structure-based function and regulation. J. Biol. Chem. 289, 16615–16623. doi:10.1074/jbc.R114.563148
Peck G. R., Ye S., Pham V., Fernando R. N., Macaulay S. L., Chai S. Y., et al. (2006). Interaction of the Akt substrate, AS160, with the glucose transporter 4 vesicle marker protein, insulin-regulated aminopeptidase. Mol. Endocrinol. 20, 2576–2583. doi:10.1210/me.2005-0476
Poznyak A. V., Litvinova L., Poggio P., Sukhorukov V. N., Orekhov A. N. (2022). Effect of glucose levels on cardiovascular risk. Cells, 11. Epub 20220928. doi:10.3390/cells11193034
Ramm G., James D. E. (2005). GLUT4 trafficking in a test tube. Cell metab. 2, 150–152. doi:10.1016/j.cmet.2005.08.008
Ramm G., Larance M., Guilhaus M., James D. E. (2006). A role for 14-3-3 in insulin-stimulated GLUT4 translocation through its interaction with the RabGAP AS160. J. Biol. Chem. 281, 29174–29180. doi:10.1074/jbc.M603274200
Rathmell J. C., Fox C. J., Plas D. R., Hammerman P. S., Cinalli R. M., Thompson C. B. (2003). Akt-directed glucose metabolism can prevent Bax conformation change and promote growth factor-independent survival. Mol. Cell. Biol. 23, 7315–7328. doi:10.1128/mcb.23.20.7315-7328.2003
Rena G., Prescott A. R., Guo S., Cohen P., Unterman T. G. (2001). Roles of the forkhead in rhabdomyosarcoma (FKHR) phosphorylation sites in regulating 14-3-3 binding, transactivation and nuclear targetting. Biochem. J. 354, 605–612. doi:10.1042/0264-6021:3540605
Roach P. J., Depaoli-Roach A. A., Hurley T. D., Tagliabracci V. S. (2012). Glycogen and its metabolism: some new developments and old themes. Biochem. J. 1 (441), 763–787. doi:10.1042/BJ20111416
Roach W. G., Chavez J. A., Miinea C. P., Lienhard G. E. (2007). Substrate specificity and effect on GLUT4 translocation of the Rab GTPase-activating protein Tbc1d1. Biochem. J. 403 (403), 353–358. doi:10.1042/BJ20061798
Rodgers S. J., Jones E. I., Arumugam S., Hamila S. A., Danne J., Gurung R., et al. (2022). Endosome maturation links PI3Kα signaling to lysosome repopulation during basal autophagy. Embo J. 4, 41, e110398. Epub 20220815.
Ryan D. G., Yang M., Prag H. A., Blanco G. R., Nikitopoulou E., Segarra-Mondejar M., et al. (2021). Disruption of the TCA cycle reveals an ATF4-dependent integration of redox and amino acid metabolism. Elife 10, 10. Epub 20211223. doi:10.7554/elife.72593
Salani B., Ravera S., Amaro A., Salis A., Passalacqua M., Millo E., et al. (2015). IGF1 regulates PKM2 function through Akt phosphorylation. Cell Cycle 14, 1559–1567. doi:10.1080/15384101.2015.1026490
Salguero A. L., Chen M., Balana A. T., Chu N., Jiang H., Palanski B. A., et al. (2022). Multifaceted regulation of akt by diverse C-terminal post-translational modifications. ACS Chem. Biol. 21 (17), 68–76. Epub 20211223. doi:10.1021/acschembio.1c00632
Sano H., Eguez L., Teruel M. N., Fukuda M., Chuang T. D., Chavez J. A., et al. (2007). Rab10, a target of the AS160 Rab GAP, is required for insulin-stimulated translocation of GLUT4 to the adipocyte plasma membrane. Cell metab. 5, 293–303. doi:10.1016/j.cmet.2007.03.001
Sasaki T., Takasuga S., Sasaki J., Kofuji S., Eguchi S., Yamazaki M., et al. (2009). Mammalian phosphoinositide kinases and phosphatases. Prog. Lipid Res. 48, 307–343. Epub 20090704. doi:10.1016/j.plipres.2009.06.001
Scarpa M., Singh P., Bailey C. M., Lee J. K., Kapoor S., Lapidus R. G., et al. (2021). PP2A-activating drugs enhance FLT3 inhibitor efficacy through AKT inhibition-dependent GSK-3β-mediated c-myc and pim-1 proteasomal degradation. Mol. Cancer Ther. 20, 676–690. Epub 20210210. doi:10.1158/1535-7163.MCT-20-0663
Schmidt S., Joost H. G., Schurmann A. (2009). GLUT8, the enigmatic intracellular hexose transporter. Am. J. physiology Endocrinol. metabolism 296, E614–E618. doi:10.1152/ajpendo.91019.2008
Semrau M. S., Giachin G., Covaceuszach S., Cassetta A., Demitri N., Storici P., et al. (2022). Molecular architecture of the glycogen-committed PP1/PTG holoenzyme. Nat. Commun. 13, 6199. Epub 20221019. doi:10.1038/s41467-022-33693-z
Sergi C., Shen F., Liu S. M. (2019). Insulin/IGF-1R, SIRT1, and FOXOs pathways-an intriguing interaction platform for bone and osteosarcoma. Front. Endocrinol. (Lausanne) 10, 93. Epub 20190301. doi:10.3389/fendo.2019.00093
Shaerzadeh F., Motamedi F., Khodagholi F. (2014). Inhibition of akt phosphorylation diminishes mitochondrial biogenesis regulators, tricarboxylic acid cycle activity and exacerbates recognition memory deficit in rat model of Alzheimer’s disease. Cell Mol. Neurobiol. 34, 1223–1233. Epub 20140819. doi:10.1007/s10571-014-0099-9
Shao D., Villet O., Zhang Z., Choi S. W., Yan J., Ritterhoff J., et al. (2018). Glucose promotes cell growth by suppressing branched-chain amino acid degradation. Nat. Commun. 9 (9), 2935. doi:10.1038/s41467-018-05362-7
Sharma G., Wu C. Y., Wynn R. M., Gui W., Malloy C. R., Sherry A. D., et al. (2019). Real-time hyperpolarized (13)C magnetic resonance detects increased pyruvate oxidation in pyruvate dehydrogenase kinase 2/4-double knockout mouse livers. Sci. Rep. 11 (9), 16480. Epub 20191111. doi:10.1038/s41598-019-52952-6
Sheth S. S., Castellani L. W., Chari S., Wagg C., Thipphavong C. K., Bodnar J. S., et al. (2005). Thioredoxin-interacting protein deficiency disrupts the fasting-feeding metabolic transition. J. lipid Res. 46, 123–134. doi:10.1194/jlr.M400341-JLR200
Shiau J. P., Chuang Y. T., Cheng Y. B., Tang J. Y., Hou M. F., Yen C. Y., et al. (2022). Impacts of oxidative stress and PI3K/AKT/mTOR on metabolism and the future direction of investigating fucoidan-modulated metabolism. Antioxidants (Basel), 11. Epub 20220506. doi:10.3390/antiox11050911
Shin N., Lee H. J., Sim D. Y., Im E., Park J. E., Park W. Y., et al. (2021). Apoptotic effect of compound K in hepatocellular carcinoma cells via inhibition of glycolysis and Akt/mTOR/c-Myc signaling. Phytother. Res. 35, 3812–3820. Epub 20210415. doi:10.1002/ptr.7087
Shisheva A. (2008a). Phosphoinositides in insulin action on GLUT4 dynamics: not just PtdIns(3,4,5)P3. Am. J. physiology Endocrinol. metabolism 295, E536–E544. doi:10.1152/ajpendo.90353.2008
Shisheva A. (2008b). PIKfyve: partners, significance, debates and paradoxes. Cell Biol. Int. 32, 591–604. doi:10.1016/j.cellbi.2008.01.006
Siddika T., Balasuriya N., Frederick M. I., Rozik P., Heinemann I. U., O’Donoghue P. (2022). Delivery of active AKT1 to human cells. Cells 29, 11. Epub 20221129. doi:10.3390/cells11233834
Simon-Molas H., Arnedo-Pac C., Fontova P., Vidal-Alabró A., Castaño E., Rodríguez-García A., et al. (2018). PI3K-Akt signaling controls PFKFB3 expression during human T-lymphocyte activation. Mol. Cell Biochem. 448, 187–197. Epub 20180212. doi:10.1007/s11010-018-3325-9
Song M., Bode A. M., Dong Z., Lee M. H. (2019). AKT as a therapeutic target for cancer. Cancer Res. 15 (79), 1019–1031. Epub 20190226. doi:10.1158/0008-5472.CAN-18-2738
Staal S. P. (1987). Molecular cloning of the akt oncogene and its human homologues AKT1 and AKT2: amplification of AKT1 in a primary human gastric adenocarcinoma. Proc. Natl. Acad. Sci. U. S. A. 84, 5034–5037. doi:10.1073/pnas.84.14.5034
Stemmer K., Muller T. D., DiMarchi R. D., Pfluger P. T., Tschop M. H. (2019). CNS-targeting pharmacological interventions for the metabolic syndrome. J. Clin. investigation, 130. doi:10.1172/JCI129195
Tan S. X., Ng Y., Burchfield J. G., Ramm G., Lambright D. G., Stöckli J., et al. (2012). The Rab GTPase-activating protein TBC1D4/AS160 contains an atypical phosphotyrosine-binding domain that interacts with plasma membrane phospholipids to facilitate GLUT4 trafficking in adipocytes. Mol. Cell Biol. 32, 4946–4959. Epub 20121008. doi:10.1128/MCB.00761-12
Tang B., Frasinyuk M. S., Chikwana V. M., Mahalingan K. K., Morgan C. A., Segvich D. M., et al. (2020). Discovery and development of small-molecule inhibitors of glycogen synthase. J. Med. Chem. 9 (63), 3538–3551. Epub 20200323. doi:10.1021/acs.jmedchem.9b01851
Taylor E. B., An D., Kramer H. F., Yu H., Fujii N. L., Roeckl K. S., et al. (2008). Discovery of TBC1D1 as an insulin-AICAR-and contraction-stimulated signaling nexus in mouse skeletal muscle. J. Biol. Chem. 283, 9787–9796. doi:10.1074/jbc.M708839200
Tewari D., Patni P., Bishayee A., Sah A. N., Bishayee A. (2022). Natural products targeting the PI3K-Akt-mTOR signaling pathway in cancer: a novel therapeutic strategy. Semin. Cancer Biol. May 80, 1–17. Epub 20191219. doi:10.1016/j.semcancer.2019.12.008
Thorens B., Mueckler M. (2010). Glucose transporters in the 21st century. Am. J. physiology Endocrinol. metabolism 298, E141–E145. doi:10.1152/ajpendo.00712.2009
Toulany M., Rodemann H. P. (2015). Phosphatidylinositol 3-kinase/Akt signaling as a key mediator of tumor cell responsiveness to radiation. Semin. Cancer Biol. 35, 180–190. Epub 20150717. doi:10.1016/j.semcancer.2015.07.003
Tristan C., Shahani N., Sedlak T. W., Sawa A. (2011). The diverse functions of GAPDH: views from different subcellular compartments. Cell. Signal. Feb 23, 317–323. doi:10.1016/j.cellsig.2010.08.003
Tzivion G., Dobson M., Ramakrishnan G. (2011). FoxO transcription factors; Regulation by AKT and 14-3-3 proteins. Biochimica biophysica acta. Nov 1813, 1938–1945. doi:10.1016/j.bbamcr.2011.06.002
Valicherla G. R., Gupta A. P., Hossain Z., Riyazuddin M., Syed A. A., Husain A., et al. (2019). Pancreastatin inhibitor, PSTi8 ameliorates metabolic health by modulating AKT/GSK-3β and PKCλ/ζ/SREBP1c pathways in high fat diet induced insulin resistance in peri-/post-menopausal rats. Peptides 120, 170147. Epub 20190829. doi:10.1016/j.peptides.2019.170147
Vanhaesebroeck B., Alessi D. R. (2000). The PI3K-PDK1 connection: more than just a road to PKB. Biochem. J. 346 Pt 3 (3), 561–576. doi:10.1042/bj3460561
von Wilamowitz-Moellendorff A., Hunter R. W., García-Rocha M., Kang L., López-Soldado I., Lantier L., et al. (2013). Glucose-6-phosphate-mediated activation of liver glycogen synthase plays a key role in hepatic glycogen synthesis. Diabetes 62, 4070–4082. Epub 20130829. doi:10.2337/db13-0880
Waldhart A. N., Dykstra H., Peck A. S., Boguslawski E. A., Madaj Z. B., Wen J., et al. (2017). Phosphorylation of TXNIP by AKT mediates acute influx of glucose in response to insulin. Cell Rep. 19, 2005–2013. doi:10.1016/j.celrep.2017.05.041
Waller A. P., George M., Kalyanasundaram A., Kang C., Periasamy M., Hu K., et al. (2013). GLUT12 functions as a basal and insulin-independent glucose transporter in the heart. Biochimica biophysica acta, 121–127. doi:10.1016/j.bbadis.2012.09.013
Wang J., Wang J., Dai J., Jung Y., Wei C. L., Wang Y., et al. (2007). A glycolytic mechanism regulating an angiogenic switch in prostate cancer. Cancer Res. 67, 149–159. doi:10.1158/0008-5472.CAN-06-2971
Wang L., Li J., Di L. J. (2022a). Glycogen synthesis and beyond, a comprehensive review of GSK3 as a key regulator of metabolic pathways and a therapeutic target for treating metabolic diseases. Med. Res. Rev. Mar. 42, 946–982. Epub 20211103. doi:10.1002/med.21867
Wang N., Zhang S., Yuan Y., Xu H., Defossa E., Matter H., et al. (2022b). Molecular basis for inhibiting human glucose transporters by exofacial inhibitors. Nat. Commun. 13, 2632. Epub 20220512. doi:10.1038/s41467-022-30326-3
Wang S., Jiang B., Zhang T., Liu L., Wang Y., Wang Y., et al. (2015). Insulin and mTOR pathway regulate HDAC3-mediated deacetylation and activation of PGK1. PLoS Biol. 13, e1002243. Epub 20150910. doi:10.1371/journal.pbio.1002243
Watson R. T., Kanzaki M., Pessin J. E. (2004). Regulated membrane trafficking of the insulin-responsive glucose transporter 4 in adipocytes. Endocr. Rev. Apr 25, 177–204. doi:10.1210/er.2003-0011
Welcker M., Orian A., Jin J., Grim J. E., Harper J. W., Eisenman R. N., et al. (2004). The Fbw7 tumor suppressor regulates glycogen synthase kinase 3 phosphorylation-dependent c-Myc protein degradation. Proc. Natl. Acad. Sci. U. S. A. 101, 9085–9090. doi:10.1073/pnas.0402770101
Wise D. R., DeBerardinis R. J., Mancuso A., Sayed N., Zhang X. Y., Pfeiffer H. K., et al. (2008). Myc regulates a transcriptional program that stimulates mitochondrial glutaminolysis and leads to glutamine addiction. Proc. Natl. Acad. Sci. U. S. A. 105, 18782–18787. doi:10.1073/pnas.0810199105
Wolf A., Agnihotri S., Guha A. (2010). Targeting metabolic remodeling in glioblastoma multiforme. Oncotarget. Nov. 1, 552–562. doi:10.18632/oncotarget.190
Wu W. S., Chen R. F., Cheng C. C., Wei J. L., Lin C. F., You R. I., et al. (2022a). Suppressing of src-hic-5-JNK-AKT signaling reduced GAPDH expression for preventing the progression of HuCCT1 cholangiocarcinoma. Pharmaceutics, 14. Epub 20221202. doi:10.3390/pharmaceutics14122698
Wu Y., Niu D., Deng S., Lei X., Xie Z., Yang X. (2022b). Tumor-derived or non-tumor-derived exosomal noncodingRNAs and signaling pathways in tumor microenvironment. Int. Immunopharmacol. May 106, 108626. Epub 20220218. doi:10.1016/j.intimp.2022.108626
Xie Y., Shi X., Sheng K., Han G., Li W., Zhao Q., et al. (2019). PI3K/Akt signaling transduction pathway, erythropoiesis and glycolysis in hypoxia (Review). Mol. Med. Rep. Feb 19, 783–791. Epub 20181203. doi:10.3892/mmr.2018.9713
Xing Y., Lin N. U., Maurer M. A., Chen H., Mahvash A., Sahin A., et al. (2019). Phase II trial of AKT inhibitor MK-2206 in patients with advanced breast cancer who have tumors with PIK3CA or AKT mutations, and/or PTEN loss/PTEN mutation. Breast Cancer Res. 21, 78. Epub 20190705. doi:10.1186/s13058-019-1154-8
Yang W., Zheng Y., Xia Y., Ji H., Chen X., Guo F., et al. (2012). ERK1/2-dependent phosphorylation and nuclear translocation of PKM2 promotes the Warburg effect. Nat. Cell Biol. 14, 1295–1304. doi:10.1038/ncb2629
Yang X., Ye H., He M., Zhou X., Sun N., Guo W., et al. (2018). LncRNA PDIA3P interacts with c-Myc to regulate cell proliferation via induction of pentose phosphate pathway in multiple myeloma. Biochem. Biophys. Res. Commun. Mar. 25 (498), 207–213. Epub 20180301. doi:10.1016/j.bbrc.2018.02.211
Ye L., Jiang Y., Zhang M. (2022). Crosstalk between glucose metabolism, lactate production and immune response modulation. Cytokine Growth Factor Rev. 68, 81–92. Epub 20221107. doi:10.1016/j.cytogfr.2022.11.001
Yin S., Liu L., Brobbey C., Palanisamy V., Ball L. E., Olsen S. K., et al. (2021). PRMT5-mediated arginine methylation activates AKT kinase to govern tumorigenesis. Nat. Commun. 12 (12), 3444. Epub 20210608. doi:10.1038/s41467-021-23833-2
Yu M., Wu S., Gong C., Chen L. (2022a). Neuregulin-1β increases glucose uptake and promotes GLUT4 translocation in palmitate-treated C2C12 myotubes by activating PI3K/AKT signaling pathway. Front. Pharmacol. 13, 1066279. Epub 20230110. doi:10.3389/fphar.2022.1066279
Yu Q., Dai W., Ji J., Wu L., Feng J., Li J., et al. (2022b). Sodium butyrate inhibits aerobic glycolysis of hepatocellular carcinoma cells via the c-myc/hexokinase 2 pathway. J. Cell Mol. Med. May 26, 3031–3045. Epub 20220416. doi:10.1111/jcmm.17322
Yu X., Wang R., Zhang Y., Zhou L., Wang W., Liu H., et al. (2019). Skp2-mediated ubiquitination and mitochondrial localization of Akt drive tumor growth and chemoresistance to cisplatin. Oncogene 38, 7457–7472. Epub 20190821. doi:10.1038/s41388-019-0955-7
Zeng L., Zhou H. Y., Tang N. N., Zhang W. F., He G. J., Hao B., et al. (2016). Wortmannin influences hypoxia-inducible factor-1 alpha expression and glycolysis in esophageal carcinoma cells. World J. Gastroenterol. 22 (22), 4868–4880. doi:10.3748/wjg.v22.i20.4868
Zhang J., Jia L., Lin W., Yip Y. L., Lo K. W., Lau V. M. Y., et al. (2017). Epstein-barr virus-encoded latent membrane protein 1 upregulates glucose transporter 1 transcription via the mTORC1/NF-kappaB signaling pathways. J. virology. Mar 15, 91. doi:10.1128/JVI.02168-16
Zhang J. Y., Zhang F., Hong C. Q., Giuliano A. E., Cui X. J., Zhou G. J., et al. (2015). Critical protein GAPDH and its regulatory mechanisms in cancer cells. Mar 12, 10–22. doi:10.7497/j.issn.2095-3941.2014.0019
Zhang K., Li L., Qi Y., Zhu X., Gan B., DePinho R. A., et al. (2012). Hepatic suppression of Foxo1 and Foxo3 causes hypoglycemia and hyperlipidemia in mice. Endocrinol. Feb 153, 631–646. doi:10.1210/en.2011-1527
Zhang Y., Cai H., Liao Y., Zhu Y., Wang F., Hou J. (2020). Activation of PGK1 under hypoxic conditions promotes glycolysis and increases stem cell-like properties and the epithelial-mesenchymal transition in oral squamous cell carcinoma cells via the AKT signalling pathway. Int. J. Oncol. Sep. 57, 743–755. Epub 20200616. doi:10.3892/ijo.2020.5083
Keywords: AKT, glucose uptake, glucose glycolysis, cancer, tricarboxylic acid cycle
Citation: Li X, Hu S, Cai Y, Liu X, Luo J and Wu T (2024) Revving the engine: PKB/AKT as a key regulator of cellular glucose metabolism. Front. Physiol. 14:1320964. doi: 10.3389/fphys.2023.1320964
Received: 13 October 2023; Accepted: 12 December 2023;
Published: 08 January 2024.
Edited by:
Dale Tang, Albany Medical College, United StatesReviewed by:
Keith R. Laderoute, Consultant, United StatesMithlesh Kumar Temre, National Institute on Aging (NIH), United States
Vasudevarao Penugurti, Duke University, United States
Copyright © 2024 Li, Hu, Cai, Liu, Luo and Wu. This is an open-access article distributed under the terms of the Creative Commons Attribution License (CC BY). The use, distribution or reproduction in other forums is permitted, provided the original author(s) and the copyright owner(s) are credited and that the original publication in this journal is cited, in accordance with accepted academic practice. No use, distribution or reproduction is permitted which does not comply with these terms.
*Correspondence: Tao Wu, V3RkamYwNjA4MDlAMTYzLmNvbQ==; Xia Li, MTg5ODA2MDY5NzFAMTYzLmNvbQ==