- 1Institute of Life Sciences & Biomedical Collaborative Innovation Center of Zhejiang Province, Wenzhou University, Wenzhou, China
- 2Zhuji People’s Hospital of Zhejiang Province, Zhuji, China
- 3Key Laboratory for Biorheological Science and Technology of Ministry of Education, State and Local Joint Engineering Laboratory for Vascular Implants, Bioengineering College of Chongqing University, Chongqing, China
- 4Department of Science and Education, The Third People’s Hospital Health Care Group of Cixi, Ningbo, China
Skin pigmentation abnormalities, ranging from aesthetic concerns to severe hyperpigmentation disease, have profound implications for individuals’ psychological and economic wellbeing. The intricate etiology of hyperpigmentation and our evolving comprehension of its underlying mechanisms underscore the need for robust animal models. Zebrafish, renowned for their transparent embryos and genetic parallels to humans, have been spotlighted as a pivotal model for skin pigmentation studies. This review offers a concise overview of zebrafish skin attributes, highlighting the shared melanin production pathways with humans. We systematically dissect the diverse strategies to craft zebrafish models of abnormal skin pigmentation, spanning physical, chemical, and genetic interventions, while critically appraising the merits and constraints of each approach. Additionally, we elucidate the metrics employed to gauge the efficacy of these models. Concluding, we cast a visionary gaze on prospective breakthroughs in the domain, aiming to steer forthcoming efforts in refined zebrafish models for skin pigmentation research.
1 Introduction
Skin pigmentation serves as a crucial protective mechanism against various external threats. It acts as a shield against ultraviolet rays, plays an integral role in immune function, skin cell metabolism, and stratification, and offers protection against external pollutants (Raveendra et al., 2020). Despite its protective functions, various factors can lead to abnormal skin pigmentation (Cortés et al., 2022). Such abnormalities, whether stemming from diseases or external stimuli, manifest as conditions such as skin heterochromia, melasma, and post-inflammatory hyperpigmentation. These conditions can be categorized as either genetic or acquired diseases (Abolfotouh et al., 2012). Annually, tens or hundreds of millions of individuals globally grapple with diverse pigmentation disorders (Kutlubay et al., 2019; Ekore and Ekore, 2021; Albaghdadi et al., 2022). Beyond the evident cosmetic concerns, these disorders can severely impact individuals’ psychological wellbeing, leading to feelings of embarrassment, sadness, low self-esteem, and even severe mental disorders (Lee, 2021) like anxiety and depression (Dabas et al., 2020). Such conditions can significantly degrade the quality of life of affected individuals (Danescu et al., 2020), and the associated treatment processes often entail substantial medical and economic burdens.
The primary triggers for skin pigmentation disorders include inflammation (Ding et al., 2020), Sun exposure (Solano, 2020; Yardman-Frank and Fisher, 2021), chloasma (Lee, 2015; Mobasher et al., 2020), and a combination of specific diseases and medications (Giménez García and Carrasco Molina, 2019). For instance, injuries like cuts or conditions like eczema can incite inflammation, in which mediators such as arachidonic acid, prostaglandins, and interleukins can activate epidermal melanocytes (Morelli et al., 1989; Gledhill et al., 2010; Upadhyay et al., 2021). This activation enhances melanin production, leading to pigmentation (Silpa-Archa et al., 2017). Similarly, excessive exposure to ultraviolet radiation (UVR) (Guo et al., 2023) can induce melanin production (Yardman-Frank and Fisher, 2021), resulting in conditions like Sun spots (Solano, 2020). Hormonal fluctuations, especially in women during conditions like pregnancy, can lead to the development of melasma (Jang et al., 2010; Schmidt et al., 2016; Filoni et al., 2019). However, factors like Sun exposure, genetic predispositions, and hormonal changes can also cause melasma in men (Bolanca et al., 2008; Sarkar et al., 2010; Filoni et al., 2019). For example, the circulating luteinizing hormone is significantly higher and testosterone is markedly low (Sialy et al., 2000). Additionally, certain medications (Nahhas et al., 2019) and medical conditions, such as Nelson’s syndrome (Daniel et al., 2018) and Addison’s disease (Agrawala et al., 2013), can trigger skin pigmentation disorders. There are also tuberculosis patients who take pyrazinamide can cause brown pigmentation on the face (Denti et al., 2022; Gils et al., 2022) and so on.
In the field of biomedical research, the generation and utilization of animal models are of paramount importance. These models offer insights into the onset and progression of human diseases, paving the way for the development of preventive and therapeutic strategies. Over the past decades, numerous cell and animal models have been established to study pigmentation diseases, facilitating the discovery of therapeutic drugs and methodologies. Traditional animal modeling methods, along with their merits and demerits, are summarized in Table 1.
Despite their effectiveness in representing pigmentation diseases, traditional models often fall short due to their time-consuming generation process, intricate operations, and high costs. As a result, researchers have turned to explore alternative models, such as the zebrafish (Danio rerio). The zebrafish, with its unique skin structure and melanin production mechanism, offers several advantages as a model animal (Ceol et al., 2008; Lin et al., 2016; Rosa et al., 2022). This review delves into the skin structure of zebrafish, the intricacies of melanin production, and the benefits of using zebrafish as a model. It also provides a comprehensive overview of the application of physical, chemical and genetic methods to establish abnormal zebrafish skin pigmentation models. The challenges, potential improvement strategies, and future trends associated with this model are also discussed.
To ensure a comprehensive review, we conducted systematic searches in databases including PubMed, Web of Science, and Scopus. Our search strategy employed combinations of keywords such as “zebrafish”, “skin pigmentation”, “melanin production”, and “abnormal pigmentation”. We restricted our search to articles published between 2000 and 2023. Inclusion criteria: Articles written in English; Experimental studies focusing on zebrafish models for skin pigmentation. Exclusion criteria: Articles not directly related to the theme; Non-peer-reviewed articles; Studies focusing on other animal models.
2 Skin characteristics of zebrafish
2.1 Skin structure of zebrafish
Zebrafish, named for their distinctive back and abdominal stripes reminiscent of a zebra’s pattern (Patterson and Parichy, 2019), have a skin structure that can be broadly categorized into four layers (Figure 1A): epidermis, dermis, subcutaneous tissue, and muscle.
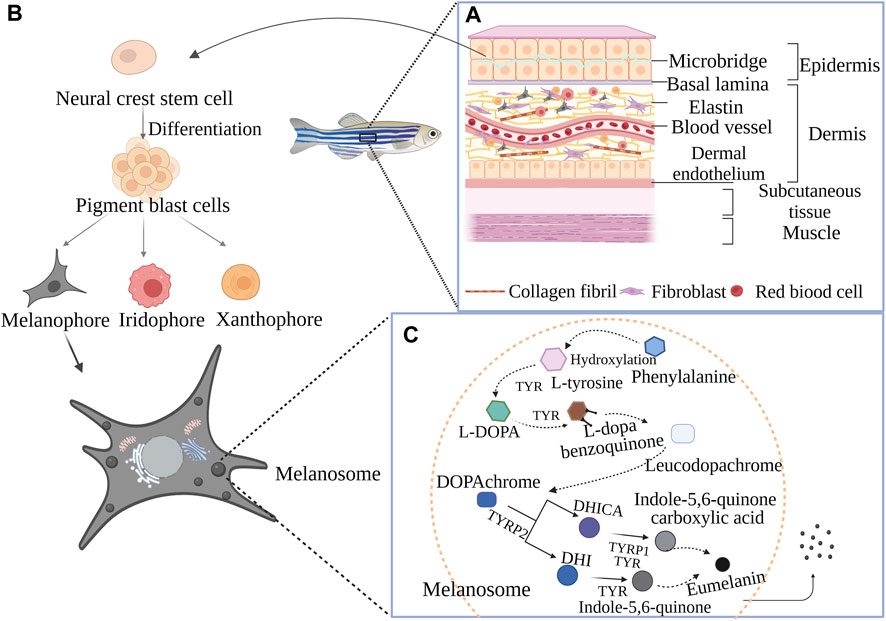
FIGURE 1. The composition of pigment cells, skin structure, and the process of eumelanin production in zebrafish: (A) Detailed structure of the zebrafish skin; (B) Species of zebrafish pigment cells; (C) The process of eumelanin production in melanosomes.
Epidermis: The outermost layer acts as a protective barrier between the zebrafish and its environment, playing a pivotal role in interaction and communication with external elements. Comprising two layers of flat epithelial cells, the epidermis is densely populated with various cell types, including melanocytes, squamous cells, squamous epithelial cells, and color cells (Patterson and Parichy, 2013). Among them, melanocytes synthesize melanin and regulate fish color. Squamous cells secrete keratinous substances to enhance skin defense. Squamous epithelial cells and color cells secrete mucus and color pigments, which play a role in protecting the skin and attracting partners. Microridges are also distributed in the center of the epidermis keratinocyte surface. Scales are attached to fish skin as they mature for protection (Sire and Akimenko, 2004). Similar to the epidermis of terrestrial vertebrates such as guinea pigs or humans, the fish epidermis is a multilayer tissue that is separated from the dermis by a layer of filamentous proteins, the basal lamina (Sonawane et al., 2005).
Dermis: Situated beneath the epidermis, the dermis is rich in collagen fibers, elastic fibers, and fibroblasts (Le Guellec et al., 2004). The dermis is composed of a relaxed layer and a dense layer and, in marked contrast to mammalian skin, is separated from the subcutaneous adipogenic tissue by another inner layer, called the dermal endothelium. The thin relaxed layer of the fish dermis consists of loosely arranged connective tissue and is complemented by blood vessels and nerve fibers. Dermal cells are mainly fibroblasts interspersed with different pigment cells (Frohnhöfer et al., 2013). It provides structural support to the skin and houses numerous capillaries and nerve endings, essential for nutrient supply and sensory transmission.
Subcutaneous tissue: The subcutaneous tissue layer lies beneath the dermis and is mainly composed of fat and connective tissue. The main functions of the subcutaneous tissue layer are to provide support, insulation, and energy storage for the skin. The muscle layer (Goody et al., 2017) is the innermost layer of the skin and is composed of muscle tissue.
Muscle Layer: The primary function of the muscle layer is to control movement. The skin of embryonic zebrafish is composed of an outer epidermal cell layer and an inner basal epidermal cell layer, and the latter is bound to the basement membrane.
Interestingly, the skin structure of adult zebrafish mirrors that of human skin, encompassing layers such as the basal, spinous, granular, hyaline, and epidermal keratinocyte layer (Li et al., 2011).
2.2 Melanin production in zebrafish
The melanin synthesis mechanism in zebrafish closely aligns with that in humans. Originating from embryonic neural crest stem cells, adult zebrafish chromatophores play a crucial role in pigment formation (Figure 1B) (Quigley et al., 2004; Budi et al., 2008; Robinson and Fisher, 2009). These stem cells differentiate into pigment blast cells, which further evolve into melanophores, iridophores, and xanthophores (Kelsh et al., 2009; Kondo and Shirota, 2009; Patterson and Parichy, 2019), directly influencing the number and composition of pigment cells. Melanophores and iridophores make up the horizontal black stripes, and xanthophores and iridophores (Hirata et al., 2003) make up the light-colored stripes (Maderspacher and Nüsslein-Volhard, 2003).
In addition to cartilaginous, neuronal, and glial derivatives common to mammals and birds, the zebrafish neural crest produces three different types of pigment cells. Melanophores first appear in the embryo and laterally form on both sides of the head about 24 h after fertilization, then continue to extend downwards to produce melanin. In the production of the embryonic/early larval pigment pattern at approximately 4 days after fertilization (Haffter et al., 1996), clusters of xanthophores are scattered on the side, melaophores and iridophores grow along the dorsal and ventral edges of the body and on the vitelline, and some melanocytes appear in the transverse striae along the horizontal muscle wall. Over the next few days, a small number of melanophores will appear and grow into side lines (Hultman and Johnson, 2010). Xanthophores initially appear on the dorsal side of the head and then gradually extend caudally. Finally, iridophores appear in the tail of the eyes and in the choroid, slowly developing patches on the yolk. However, after the eighth day, the yellow pigment cell mass of the embryonic/early larval stage gradually disappeared and began to form the pigmented streak pattern of the adult fish (McMenamin et al., 2014). The spatial distribution of pigment cells in the subcutaneous, muscle and epidermis of the zebrafish adult skin results in the formation of dark and light stripes (Le Guellec et al., 2004; Hirata et al., 2005). Zebrafish scales also have more superficial layers of pigment cells that make up the black lines on the back. In addition, there are stripes and light-colored patches on the middle fin. In summary, a zebrafish with “zebra stripes” is formed.
The melanin closely related to skin color in zebrafish is eumelanin. The three key enzymes encoding melanin biosynthesis in zebrafish are tyrosinase (TYR) (Zhu et al., 2015), tyrosinase-related protein 1 (TYRP-1) and tyrosinase-related protein 2 (TYRP-2) (Jung et al., 2016). The synthesis of eumelanin (Sugumaran et al., 2020; Zhou et al., 2021) in zebrafish is highly similar to that in mammals and mainly occurs in melanosomes in melanocytes. The specific process is as follows (Figure 1C) (Sugumaran et al., 2020; Zhou et al., 2021; Liu et al., 2022): Firstly, phenylalanine is hydroxylated to L-tyrosine or directly initiated by L-tyrosine, and L-3, 4-dihydroxyphenylalanine (L-DOPA) is formed by tyrosinase catalysis. L-DOPA is oxidized to L-dopa benzoquinone under the action of TYR. L-dopa benzoquinone undergoes a series of oxidation and polymerization reactions to produce colorless leucodopachrome. Because it is colorless and extremely unstable, it can be rapidly oxidized to dopachrochrome (DOPA chrome), which can be hydroxylated to 5, 6-dihydroxyindole-2-carboxylic acid (DHICA) or decarboxylated to 5, 6-dihydroxyindoles (DHI) under the action of dopachrochrome tautomerase (DCT) (it also known as TYRP-2). DHICA forms indole-5, 6-quinone carboxylic acid under the action of TYRP-1 and TYR. After decarboxylation, DHI is rapidly oxidized by TYR, resulting in the formation of indole-5, 6-quinone by polymerization reaction. Indole-5, 6-quinone carboxylic acid and indole-5, 6-quinone are both eumelanins that primarily determine the skin color of zebrafish. The factors that regulate melanin production are microphthalmia-associated transcription factor (MITF) in melanocytes. Many cytokines activate signaling pathways by interacting with receptors on melanin membranes, including the phosphatidylinositol 3-kinase (PI3K)/Akt pathway, cyclic adenosine monophosphate (cAMP)/protein kinase A (PKA) pathway, mitogen-activated protein kinase (MAPK) pathway, endothelin-1 mediated signaling cascade pathway and Wnt pathway (Liu et al., 2022). The regulation of all signaling pathways involves MITF (Zhou et al., 2021), which not only regulates the proliferation and survival of melanocytes but also regulates the expression of TYR, TYRP-1, and TYRP-2.
Zebrafish serve as an exemplary model (Lajis, 2018) for studying melanin development (Ziegler, 2003) and pigmentation disorder (Figure 2). Remarkably, about 87% of zebrafish genes share a high similarity with human genes, and the pathways associated with human melanin production are well-conserved (Du et al., 2016; Lei et al., 2023). The transparent nature of zebrafish embryos and larvae facilitates in vivo imaging, offering a holistic view of melanocyte changes. Pigmentation is the result of the production and transfer of melanin in the body, which involves the regulation of PI3K, cAMP, PKA, MAPK and other signaling pathways. Therefore, zebrafish as a model animal provides a reliable carrier for the study of pigmentation diseases. In addition, zebrafish have a smaller experimental area than other animals, and its genetic ease and other advantages make zebrafish models (MacRae and Peterson, 2015) provide better help for disease research. In addition, zebrafish has many advantages (Lin et al., 2023) over other model animals such as mice, such as in vitro insemination, in vitro development, rapid sexual maturation, high fecundity and low feeding cost (Chakraborty et al., 2009). Finally, zebrafish embryonic development takes place in vitro and the early embryonic body is transparent (Yalcin et al., 2017; Gore et al., 2018), making it suitable for holistic imaging and real-time in vivo observation (Lin F.-J. et al., 2022).
3 Models establishment of abnormal skin pigmentation in zebrafish
Zebrafish have emerged as a pivotal model organism in the realm of melanin biology and associated diseases. Their diminutive size, transparent physiology, and significant physiological parallels with mammals make them invaluable for biochemical research. The presence of pigmented bands and melanin accumulation on their body surfaces further simplifies the study of pigmentation. Figure 3 encapsulates three modeling methods.
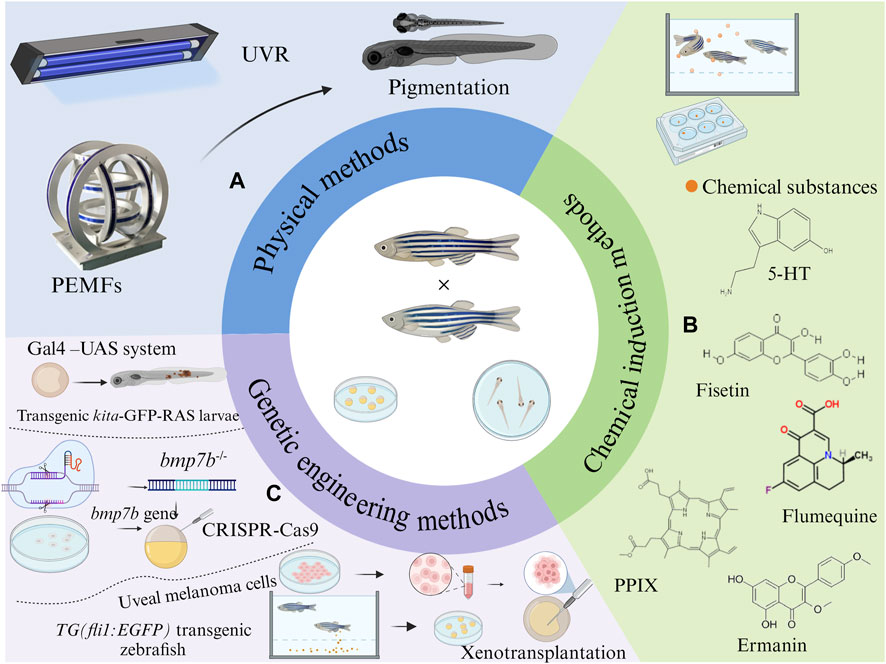
FIGURE 3. Strategies to establish zebrafish models with abnormal pigmentation: (A) Physical methods, (B) chemical induction methods, and (C) genetic engineering methods.
3.1 Physical methods
3.1.1 UVR method
UVR has been identified as a potent factor that can intensify melanin pigmentation (Hu et al., 2019), leading to hyperpigmentation disorders. Several studies have employed UVR to simulate animal models of these diseases (Figure 3A). Post-hatching, zebrafish larvae are subjected to varying UVB energy levels for distinct durations daily (Chen et al., 2019; Hu et al., 2019; Chen et al., 2022). For instance, Hu et al. (2019) exposed one-day-old larvae to UVB at 300 mJ/cm2 daily for five consecutive days. Another study by Chen et al. (2022) Collected embryos of AB wild-type zebrafish at 2 days post-fertilization and exposed them to UVB five times with 30 min intervals, delivering 8,100 mJ/cm2 (9 mW/cm2, 15 min) of energy each time, for 5 days. Chen et al. (2019) subjected larvae, 72 h post-hatching to 302 nm UVR, administering 100 mJ/cm2 of energy five times daily at 30 min intervals for 5 days. Such UVR exposure darkened the zebrafish skin color (Yang et al., 2021), elevated their melanin content, and triggered the secretion of α-melanocyte stimulating hormone and adrenocorticotropic hormone by their melanocytes, keratinocytes and fibroblasts (Shin et al., 2015). These hormones elevate intracellular cAMP content in melanocytes through autocrine or paracrine stimulation, subsequently activating PKA. The activated PKA then phosphorylates the cAMP-response element-binding protein (CREB), amplifying the expression of microphthalmia-related transcription factors, which in turn promote melanin pigmentation. Thus, UVB-induced melanogenesis, by activating PKA signaling pathway, is instrumental in constructing a zebrafish model for skin pigmentation diseases (Chiaverini et al., 2008).
3.1.2 Pulsed electromagnetic fields (PEMFs)
Beyond the traditional use of UVR for disease models establishment, recent studies have unveiled the potential of PEMFs in modulating pigmentation (Figure 3A) (Kim et al., 2018). PEMFs were generated using a Helmholtz coil, with a frequency of 60 Hz and intensities of 2G, 4G and 20G, respectively. Zebrafish embryos or larvae were exposed to these fields at 28.5°C for durations ranging from 5 to 15 days (Kim et al., 2018). Following PEMFs stimulation, a notable darkening of the zebrafish skin was observed, indicative of enhanced melanin production. This was further corroborated by an increase in the number of melanin granules and the upregulation of melanogenesis-related genes such as DCT, TYRP-1, TYRP-2, and melanocortin 1 receptor (MC1R) (Kim Y.-M. et al., 2016; Cho et al., 2016; Kim et al., 2017).
Intriguingly, MITF were significantly activated, leading to an increased expression of TYRP-1 and DCT. This was accompanied by a marked decrease in the phosphorylation of extracellular signal-regulated kinase (ERK) and a significant increase in p38 phosphorylation, both of which are key signaling pathways implicated in melanin production. Therefore, PEMFs exposure appears to enhance melanin in production and transport by modulating the phosphorylation states of ERK and p38 (Kim et al., 2018). This induces MITF and DCT, promoting pigmentation in zebrafish and facilitating the establishment of a pigmentation disease model via the p-ERK/p-p38 pathway (Jin and Thibaudeau, 1999; Richardson et al., 2008; Ye et al., 2011; Liu et al., 2013; Wang et al., 2018).
3.2 Chemical induction methods
In recent years, many chemicals have been found to promote the overproduction of melanin in zebrafish, which is favored by researchers due to its easy operation and variety. Therefore, it is possible to induce the abnormal pigmentation model of zebra fish by chemical substances. In Table 2, the methods and related mechanisms of building zebrafish pigmentation anomaly model induced by chemical substances were summarized. The chemical induction methods and the structure of the chemical substance are shown in Figure 3B.
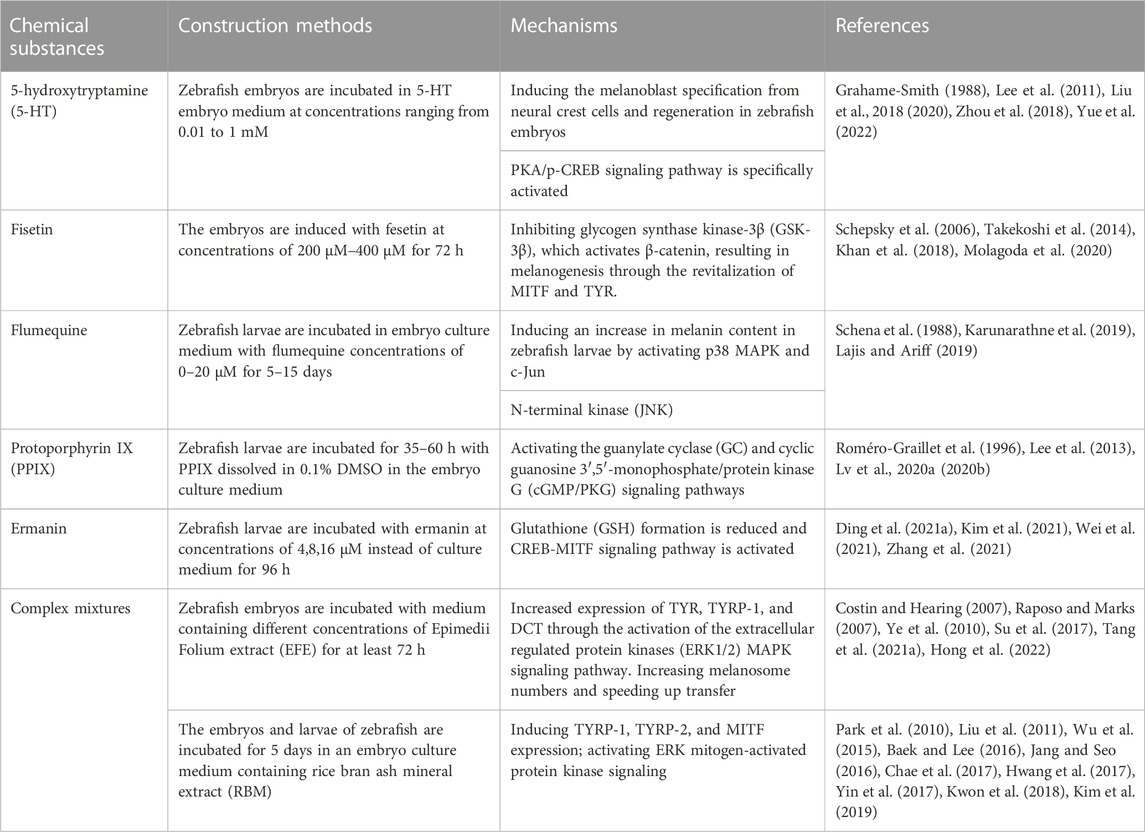
TABLE 2. Chemical methods and related physiological mechanisms for constructing a zebrafish model of abnormal pigmentation.
3.2.1 5-HT
Serotonin, also known as 5-HT, is a biogenic amine synthesized from tryptophan and a neurotransmitter that has been identified as a key player in melanogenesis (van Hooft and Vijverberg, 2000). It is synthesized from the essential amino acid tryptophan. Tryptophan is decomposed by enzyme tryptophan hydroxylase to produce the precursor 5-hydroxytryptophan, which is then transformed into 5-HT by amino acid decarboxylase. Recent studies have demonstrated that 5-HT can stimulate melanin production in zebrafish embryos, leading to an increase in melanin content and a darkening of the skin stripes (Liu et al., 2020; Tang et al., 2023). Zebrafish embryos were incubated in a culture medium with varying concentrations of 5-HT, ranging from 0.01 to 1 mM. The results showed that a dosage-dependent increase in melanin content and melanocytes count. Furthermore, 5-HT was found to induce melanoblast specification from neural crest cells and promote the regeneration of zebrafish embryos.
The mechanism behind this involves the upregulation of key proteins MITF, TYR, TYRP-1, and TYRP-2, which are crucial for melanin synthesis (Hushcha et al., 2021). Additionally, 5-HT was found to increase the expression of regeneration-associated genes, including kita, mitfa, and DCT34. These suggest that 5-HT may specifically activate the PKA/p-CREB signaling pathway, thereby up-regulating the expression of MITF and TYR to promote synthesis of melanin (Liu et al., 2020). Recent research has further elucidated the role of the 5-HT receptor in melanogenesis, showing that it enhances the pigmentation response to environmental stressors through the cAMP-PKA-MAPK, Rab27a/RhoA, and PI3K/AKT signaling pathways (Tang et al., 2023). This highlights the evolutionary significance of the 5-HT system in pigmentation biology and its potential as a therapeutic target for pigmentation disorders.
3.2.2 Fisetin
Fisetin, a naturally occurring dietary flavonoid, is primarily found in the lacquer family tree and wood wax (Khan et al., 2018). It (3,3′,4′,7-tetrahydroxyflavone) contains a typical flavonol backbone with three additional hydroxyl functional groups (Molagoda et al., 2020). Recent research has highlighted its potential in promoting melanogenesis. Specifically, fisetin has been shown to enhance melanin production and elevate the activity of TYR (Takekoshi et al., 2014). In experimental setup, zebrafish embryos were exposed to fisetin concentrations ranging between 200 and 400 µM over a period of 72 h. The outcome of this exposure was evident in the zebrafish larvae, which exhibited a noticeably darker skin hue compared to their untreated counterparts. Furthermore, there was a marked upregulation in the expression of MITF and TYR (Molagoda et al., 2020).
The underlying mechanism through which fisetin exerts its effects on pigmentation appears to involve the modulation of the GSK-3β pathway. Fisetin is believed to bind to GSK-3β, inhibiting its activity. This inhibition prevents the proteasomal degradation of β-catenin, leading to an accumulation of free β-catenin (Molagoda et al., 2020). Once liberated, β-catenin translocates to the cell nucleus where it binds to specific promoter region of the MITF gene. This binding event activates MITF expression, which in turn stimulates TYR-mediated melanin synthesis. The end result is hyperpigmentation in zebrafish (Schepsky et al., 2006).
3.2.3 Flumequine
Flumequine, a derivative fluoroquinolone class of antibiotics, is traditionally employed in veterinary medicine to address intestinal infections (Schena et al., 1988). It has a structure of pyridine quinoline and 3-oxo monocarboxylic acid, and is also an organic fluorine compound (Tarushi et al., 2013). Beyond its antibacterial properties, recent investigations have unveiled its potential in modulating melanin synthesis. In a controlled study, zebrafish embryos were exposed to varying concentrations of flumequine, ranging from 0 to 20 μM, over a span of 5–15 days. The aftermath of this exposure was evident in zebrafish larvae, which manifested enhanced skin hyperpigmentation. Concurrently, there was a significant surge in melanin production, accompanied by an upregulation in the expression of TYR and MITF.
The underlying mechanism through which flumequine influences pigmentation appears to be multifaceted. Notably, flumequine was found to promote the phosphorylation of p38, MAPK and JNK (Karunarathne et al., 2019). This phosphorylation cascade is believed to induce an elevation in the activity of MITF and TYR, pivotal regulators of melanin synthesis (Niu et al., 2018). Beside, it can also promote melanin production and accumulation by inducing the elevation of MITF and TYR activity through the activation of p38 MAPK and JNK (Lajis and Ariff, 2019), but not its direct binding to TYR. Additionally, flumequine have no toxic effect on zebrafish larvae (Karunarathne et al., 2019). Therefore, the concentration gradient of flumequine can be used to construct different zebrafish skin pigmentation models.
3.2.4 PPIX
PPIX, is a heterocyclic organic compound, which consists of four pyrrole rings, and is the final intermediate in the heme biosynthetic pathway. Its tetrapyrrole structure enables it to chelate transition metals to form metalloporphyrins, which perform a variety of biologic functions (Sachar et al., 2016). Beyond its role in heme synthesis, PPIX has been recognized for its diverse biological functions, including promoting cell respiration, enhancing protein and glucose metabolism, and inhibiting complement binding (Thapar and Bonkovsky, 2008; Dailey and Meissner, 2013). Recent studies have illuminated its potential in melanogenesis, particularly in melanoma cells, where it acts as a stimulator of melanogenesis (Kim et al., 2006; Kim et al., 2016 B.-H.; Alesiani et al., 2009).
In experimental setups involving zebrafish, larvae were exposed to PPIX dissolved in 0.1% DMSO in the embryo culture medium for durations ranging from 35 to 60 h. The outcome of this exposure was evident in the zebrafish larvae, which exhibited increased body pigmentation (Lv et al., 2020a). Concurrently, there was a significant rise in TYR activity. Mechanistically, PPIX is believed to elevate cGMP levels and PKG activity by stimulating GC. This activation cascade subsequently promotes melanogenesis, primarily through the CREB signaling pathway (Lv et al., 2020a). Furthermore, PPIX activates the GC/cGMP/PKG signaling pathway, leading to the upregulation of several key proteins and genesm including TYR, MITF, myosin Va, melanophilin, Rab27a, and Cdc42 (Roméro-Graillet et al., 1996; Lee et al., 2013; Lv et al., 2020a; Lv et al., 2020b). These proteins and genes play pivotal roles in melanin synthesis and melanosome transport.
3.2.5 Ermanin
Ermanin, a flavonoid compound extracted from bee glue having methoxyl functional groups, has been traditionally employed in Chinese medicine as a remedy for vitiligo and as an agent to stimulate melanin production (Ding Q. et al., 2021). In a controlled experimental setup, fertilized zebrafish embryos were incubated in a medium enriched with ermanin (Ding Q. et al., 2021). The outcome of this exposure was evident in the zebrafish larval, which manifested enhanced melanin production and a deepening of skin pigmentation. Notably, the absence of mortality or deformities in the young fish underscores the non-toxic nature of ermanin.
Mechanistically, ermanin appears to influence melanin synthesis through multiple pathways. It has been observed to augment melanin biosynthesis by activating the CREB-MITF pathway and subsequently upregulating its downstream proteins, including TYR, TYRP-1, and DCT (Kim et al., 2021; Zhang et al., 2021). Additionally, ermanin has been linked to alterations in GSH redox homeostasis, and the accumulation of reactive oxygen species (ROS). This shift in redox balance is believed to promote melanin production (Wei et al., 2021).
3.2.6 Complex mixtures
While individual compounds have been extensively studied for their melanogenic properties, complex mixtures also hold significant potential in advancing our understanding of melanogenesis and in constructing zebrafish skin hyperpigmentation models.
Epimedium brevicornum Maxim., a staple in traditional Chinese medicine, is renowned for its therapeutic effects on sexual dysfunction and osteoporosis (Hong et al., 2022). Recent research has unveiled the melanogenic properties of Epimedium flavonoids. Icariin, the principal bioactive component of this herb, not only stimulates keratinocyte proliferation, promoting hair growth, but also augments melanogenic activity (Ye et al., 2010; Su et al., 2017). When zebrafish embryos are exposed to varying concentrations of EFE for a duration of 72 h, there was a marked increase in TYR activity and melanin particle count, leading to pronounced skin pigmentation (Hong et al., 2022). Mechanistically, EFE is believed to enhance TYR activity through the MAPK/ERK1/2 signaling pathway, upregulating the expression of TYRP-1, TYRP-2 and DCT (Raposo and Marks, 2007). Furthermore, EFE plays a povotal role in melanosome biosynthesis and transport, accelerating melanosomes maturation in melanocytes, promoting dendritic growth, and facilitating melanosome transfer from melanocytes to keratinocytes (Tang H. et al., 2021).
Rice bran ash (RBA) is an unconventional agent in melanogenesis, a byproduct of rice bran incineration. Rich in silicon and supplemented with minerals like potassium, carbon, and phosphorus (Liu et al., 2011; Kwon et al., 2018). RBM has been shown to enhance melanogenesis through the phosphorylation of CREB (Jang and Seo, 2016; Kwon et al., 2018; Kim et al., 2019). In experiments where zebrafish embryos and larvae were exposed to RBM for 5 days, there was a noticeable increase in melanin content, melanin particle count, and the expression of pigment-associated genes (Yin et al., 2017; Kim et al., 2019). This melanogenic effect of RBM is attributed to the downregulation of ERK phosphorylation (Park et al., 2010; Baek and Lee, 2016; Chae et al., 2017) and the activation of the ERK mitogen-activated protein kinase signaling pathway (Wu et al., 2015; Hwang et al., 2017), culminating in the upregulation of MITF and enhanced melanin production.
3.3 Genetic engineering methods
The main operational elements are described in the genetic engineering methods in Figure 3C.
3.3.1 Oncogenic HRAS expression driven by kita promoter/enhancer sequences leads to hyperproliferation of embryonic melanocytes
The expression of oncogenic HRAS is known to play a pivotal role in the excessive proliferation of melanocytes, thereby establishing zebrafish as a model for studying abnormal skin pigmentation patterns (Santoriello et al., 2010; del Ama et al., 2016). In a novel approach, researchers crossed zebrafish from Et (kita:GalTA4,UAS:mCherry) hzm1 line (Distel et al., 2009) with those from the Tg(UAS:eGFP-H-RAS_GV12) io6 line (Anelli et al., 2009). The offspring from this cross, referred to as kita-GFP-RAS zebrafish (Figure 3C), displayed distinct phenotypic changes. Specially, by the third day post-fertilization (DPF), these larvae exhibited altered pigment patterns and heightened oxidation levels. As they matured, between 2 and 4 weeks, a surge in transformed melanocytes was observed, predominantly in the tail stems.
The expression of the HRAS oncogene not only stimulates melanocyte growth but also sustains their proliferative state (Santoriello et al., 2010). The hyperpigmentation observed in kita-GFP-RAS larvae was not a result of ERK1/2 phosphorylation. Instead, it was found to be contingent on copper uptake, similar to control melanocytes (Hawkins et al., 2008). Copper ion is an important cogroup of TYR, which is closely related to TYR activity and promotes normal nutrition and metabolism of melanocytes. A significant revelation from this model was the tumor formation induced by oncogenic HRAS in the presence of transcriptionally active p53. Furthermore, melanoblasts expressing kita were more susceptible to transformed by the HRAS oncogene when active p53 was present. This led to the development of melanomas with a higher efficiency and reduced latency compared to melanoblasts and melanocytes expressing mitfa (Santoriello et al., 2010).
3.3.2 Xenograft human melanoma cells
The xenograft technique has emerged as a pivotal tool in the realm of melanoma research, particularly for the in-depth study of skin pigmentation disorders. By leveraging this technique, zebrafish can be effectively utilized as a rapid and sensitive in vivo vertebrate model for melanoma (van der Ent et al., 2014; Perez et al., 2018).
Central to the pathogenesis of ovarian melanoma are mutations in the G-proteins GNA11 and GNAQ. These mutations act as the driving force, leading to the activation of the MAPK pathway, among others significant pathways (Van Raamsdonk et al., 2009; Van Raamsdonk et al., 2010). Approximately 80% of Uveal Melanomas (UM) possess somatic activating mutations in GNA111 or GNAQ (Yu et al., 2014). The aberrant activation of this pathway results in excessive melanin deposition in the eyes and skin, culminating in the development of melanoma (van der Ent et al., 2014).
Researchers utilized the TG (fli1:EGFP) transgenic zebrafish (Lawson and Weinstein, 2002), characterized by EGFP expression in endothelial cells against a wild-type background. Following mating, embryos were harvested and incubated at 28°C in embryo culture medium base. Subsequent stages involved molting of the embryos. Human uveal melanoma cells, upon reaching 60%–90% confluency, were trypsinized with a 0.25% trypsin/0.53 mM EDTA. After centrifugation and washing, the cells were suspended in 2% polyvinylpyrrolidone-40. Using precision glass capillary needles, about 400–500 cells were injected into the yolk sac of 2-day post-fertilization embryos. Following a 6-day incubation at 34°C in a zebrafish embryo culture medium, the zebrafish model was effectively established (van der Ent et al., 2014).
3.3.3 Knockout of bmp7b gene
Bone morphogenetic protein 7 (BMP7) is an integral member of the transforming growth factor-β (TGF-β) superfamily, a 35 kDa homodimeric protein (Ozkaynak et al., 1990; Ducy and Karsenty, 2000), is renowned not just for its role in cartilage and bone formation but also for its influence on mammalian eye development, melanin synthesis, and melanoma genesis (Rothhammer et al., 2005; Vitic et al., 2021). In zebrafish, BMP7 diversifies into two distinct subtypes: bmp7a and bmp7b (Shawi and Serluca, 2008). Of these, bmp7b is particularly associated with pigment production.
Dong et al. Utilized zebrafish transgenic with green fluorescent protein for osteoblast-specific transcription factor (Dong et al., 2022). They harnessed the CRISPR-Cas9 technology to construct bmp7b mutant zebrafish (Figure 3C). The mutation in Bmp7b led to a pronounced increase in retinal melanin in the eyes, augmented melanin distribution in the skin, and a surge in melanin content throughout the zebrafish body. This increase in melanin might be attributed to the altered expression of genes such as wnt7ba, gna14, and erbb3b, especially in the iris of eyes.
The deletion of the Bmp7b gene results in a significant rise in melanin content in both the skin and eyes of the zebrafish. This suggests that while bmp7b naturally inhibited melanin production in zebrafish, its knockout counteracts this inhibitory effect, leading to enhanced melanin synthesis and a consequent darkening of the zebrafish body color. The underlying mechanisms for these observations could be associated with various melanogenic signaling pathways, including MAPK, Wnt, and TGF-β (Jin et al., 2001).
4 The evaluation method of the effect
Zebrafish, due to their transparent skin during the larval stage, have emerged as an invaluable model for studying skin pigmentation. Upon the successful establishment of the zebrafish skin pigmentation model, researchers have primarily employed two distinct methodologies to assess the whitening effect (Figure 4).
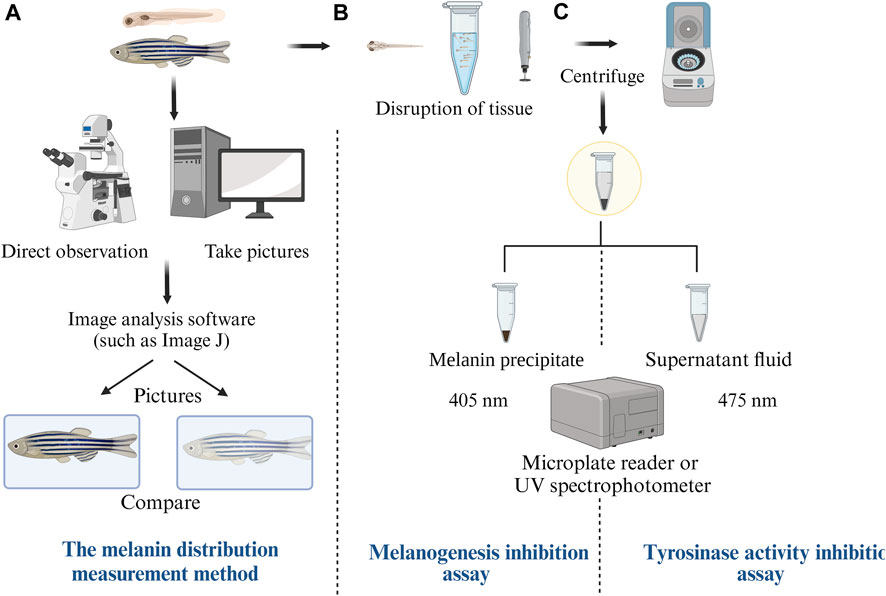
FIGURE 4. Comprehensive Evaluation Techniques for Zebrafish Skin Pigmentation Effects. (A) Melanin distribution measurement method: compare the level of melanin in the pictures, (B) Melanogenesis inhibition assay: show the cellular mechanisms of melanin production and how to measure inhibition, (C)Tyrosinase activity inhibition assay: Show the role of enzymes in melanin synthesis and how to measure their activity.
4.1 Direct visualization and quantification of melanin distribution
In the larval phase, zebrafish exhibit transparent skin, allowing for the clear observation of melanin production on their body surface. Advanced imaging such as light microscope, inverted microscope, or fluorescence microscope, facilitate the immediate visualization of these melanin changes (Logan et al., 2006; Zhang et al., 2018). Image analysis software, notably ImageJ, can be utilized to compute the melanin content. By contrasting the melanin alterations on the fish’s surfaces, one can ascertain the inhibitory effect of melanin (Park et al., 2019). The melanin distribution measurement technique, illustrated in Figure 4A, offers a direct and rapid insight into the whitening effect.
4.2 Biochemical assessment of melanin production and tyrosinase activity
This approach encompasses two primary assays (Pillaiyar et al., 2017; Wu et al., 2018): the melanogenesis inhibition assay (Figure 4B) and the tyrosinase activity inhibition assay (Figure 4C). To use this methododology, both zebrafish embryos and adult fish undergo fragmentation, centrifugation, and stratification. The melanogenesis inhibition assay involves extracting the melanin precipitate, measuring its absorbance value at a wavelength of 405 nm, and subsequently calculating the melanin content and its production inhibition rate. Conversely, the TYR activity inhibition assay requires the supernatant from the previous process, measuring its absorbance at a wavelength of 475 nm, and then determining the TYR activity inhibition rate (Ding Y. et al., 2021). These assays provide evaluations of the whitening effect from the perspectives of melanin production inhibition and TYR activity inhibition, yielding data that is both intuitive and reliable.
5 Conclusion and perspectives
Skin pigmentation disorders not only present a significant physical challenge but also exert profound psychological and economic impacts on affected individuals. Therefore, a series of products or clinical trials related to skin pigmentation have been developed in recent years. The related main functions, innovations and achievements are shown in Table 3. The quest for efficacious therapeutic agents and a deep understanding of these disorders necessitates the deployment of suitable preclinical research models. The establishment of animal models of skin pigmentation diseases using zebrafish as a model organism provides researchers with an important tool to study the pathogenesis of diseases and develop new treatments. We have made some important findings by studying the zebrafish model, and there are many potential opportunities and challenges for future research. The rapid development and high reproduction rate of zebrafish make it an ideal choice for the rapid establishment of various disease models, which also promotes its wide application in practical research. For example, zebrafish has a transparent torso when it is young and has unique advantages in global imaging and real-time in vivo observation (MacRae and Peterson, 2015). In addition, the zebrafish disease model can be used for high-throughput drug screening and plays an important role in the verification of treatment strategies (Takamiya et al., 2016). Using gene knockout and editing techniques, researchers can more accurately reveal the key molecular and cellular mechanisms related to abnormal skin pigmentation diseases, in order to further understand the role of different genes in skin pigmentation diseases. To provide a theoretical basis for treatment. Moreover, the zebrafish’s propensity for rapid chemical-induced disease modeling offers a distinct advantage over traditional animal models like guinea pigs and mice, which often present challenges in terms of time and efficacy (Patterson and Parichy, 2019).
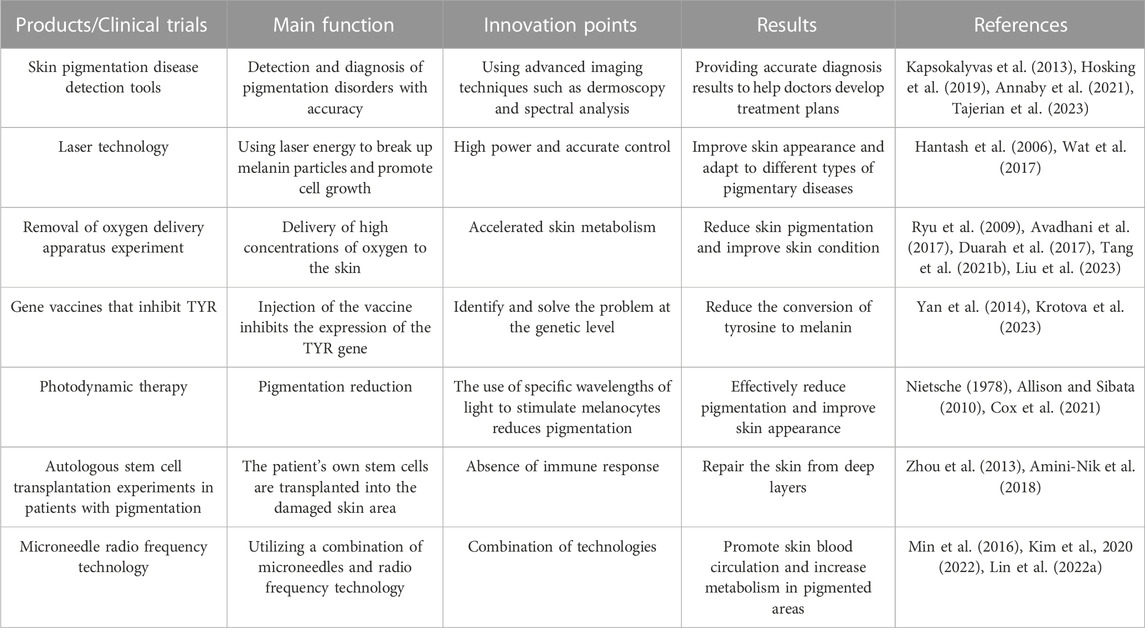
TABLE 3. Products or clinical trials related to skin pigmentation developed in recent years, main functions, innovations and results.
However, it is crucial to address certain challenges. For instance (Figure 5), while UV irradiation is a primary method for inducing abnormal pigmentation in zebrafish, concerns about self-healing, immunity, and impacts on overall development persist (de Gruijl, 2017; Del Bino et al., 2018). Some researchers have explored pulsed electromagnetic waves as an alternative, which might mitigate issues associated with UV exposure (Kim et al., 2018). Yet, the quest for the optimal physical modeling method continues, with investigations into varying electromagnetic wave intensities and frequencies showing promise.
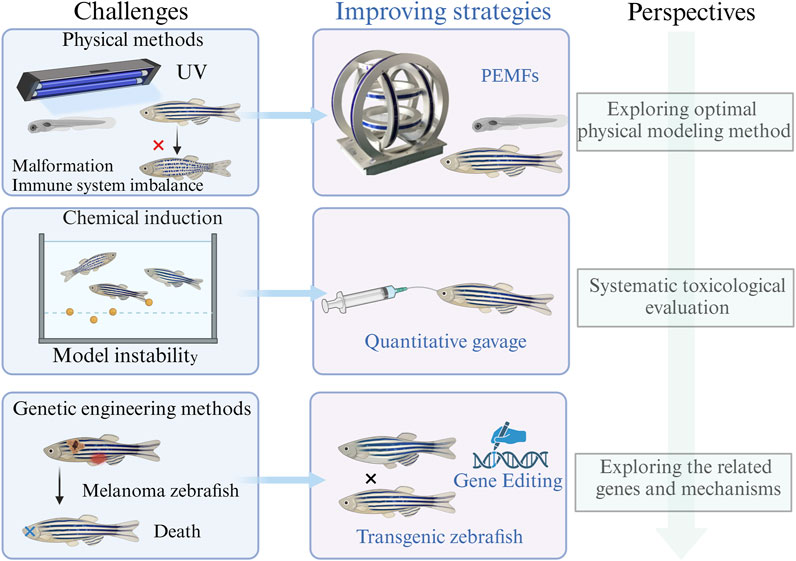
FIGURE 5. The challenge, improving strategies and perspectives of zebrafish models of abnormal skin pigmentation.
Furthermore (Figure 5), while many chemical inducers of pigmentation in zebrafish appear non-toxic in the short term (Karunarathne et al., 2019; Lv et al., 2020a; Liu et al., 2020; Molagoda et al., 2020; Ding Q. et al., 2021; Hong et al., 2022), comprehensive evaluations of long-term and acute toxicities remain scarce. Such assessments are paramount for the zebrafish model’s broader application in therapeutic evaluations. Future research might consider inducing polarity in zebrafish through extended exposure or increased concentrations, followed by systematic toxicological evaluation.
On the genetic front, while rapid and stable modeling is achievable, inducing melanoma (van der Ent et al., 2014) as the primary approach differs from the pathogenesis of straightforward skin pigmentation disorders (Rigopoulos et al., 2007; Wang et al., 2023). Efforts to address this (Figure 5), such as the bmp7b gene knockout in zebrafish (Dong et al., 2022), have encountered challenges, including development inhibition. Moreover, genetic variations alone cannot encapsulate the entirety of hyperpigmentation disorders pathogenesis (Videira et al., 2013; D’Mello et al., 2016; Wakamatsu et al., 2021). Future endeavors should focus on pinpointing key genes implicated in the pathogenesis of abnormal pigmentation diseases. A holistic regulation of these genes might pave the way for a more scientifically robust zebrafish model.
In addition, due to the complex and diverse etiology of hyperpigmentation diseases, individualized treatment strategies may be the focus of future research. Using the zebrafish model, researchers can study the effects of different etiologies and treatments on individuals, thereby providing customized treatment options for patients. In recent years, the development of gene editing technology has provided new tools for the study of pigmentation diseases. Future studies could use gene editing techniques to simulate gene mutations in human pigmentation disorders in zebrafish models and investigate the impact of these mutations on disease development. The zebrafish model can also be used to screen and evaluate potential drug targets. Future research should focus on the discovery and validation of new drug targets to provide new directions for the treatment of pigmentation disorders.
In summation, as scientific advancements continue and our understanding of zebrafish deepens, the establishment of animal models of skin pigmentation diseases using zebrafish as a model organism provides researchers with an important tool for studying disease pathogenesis and developing new treatments. Future research should continue to deeply explore the pathogenesis of pigmentation diseases, develop more effective treatments, and explore new therapeutic targets using gene editing technology and drug screening technology. These efforts will help to improve the quality of life of patients and bring new breakthroughs in the treatment of hyperpigmentation diseases.
Author contributions
JQ: Data curation, Investigation, Writing–original draft. MY: Data curation, Investigation, Writing–original draft. YF: Data curation, Investigation, Writing–original draft. JZ: Software, Supervision, Validation, Writing–original draft. TX: Software, Supervision, Validation, Writing–original draft. FL: Software, Supervision, Validation, Writing–original draft. KZ: Conceptualization, Writing–review and editing. LH: Conceptualization, Writing–review and editing. LJ: Conceptualization, Writing–review and editing. DS: Conceptualization, Writing–review and editing.
Funding
The author(s) declare financial support was received for the research, authorship, and/or publication of this article. This work was supported by the Graduate Scientific Research Foundation of Wenzhou University (3162023003048, 3162023003049, 3162023003051 and 3162023004076).
Conflict of interest
The authors declare that the research was conducted in the absence of any commercial or financial relationships that could be construed as a potential conflict of interest.
Publisher’s note
All claims expressed in this article are solely those of the authors and do not necessarily represent those of their affiliated organizations, or those of the publisher, the editors and the reviewers. Any product that may be evaluated in this article, or claim that may be made by its manufacturer, is not guaranteed or endorsed by the publisher.
References
Abolfotouh M. A., Al-Khowailed M. S., Suliman W. E., Al-Turaif D. A., Al-Bluwi E., Al-Kahtani H. S. (2012). Quality of life in patients with skin diseases in central Saudi Arabia. Int. J. Gen. Med. 5, 633–642. doi:10.2147/IJGM.S33276
Ackermann J., Frutschi M., Kaloulis K., McKee T., Trumpp A., Beermann F. (2005). Metastasizing melanoma formation caused by expression of activated N-RasQ61K on an INK4a-deficient background. Cancer Res. 65, 4005–4011. doi:10.1158/0008-5472.CAN-04-2970
Agrawala R. K., Sahoo S. K., Choudhury A. K., Mohanty B. K., Baliarsinha A. K. (2013). Pigmentation in vitamin B12 deficiency masquerading Addison’s pigmentation: a rare presentation. Indian J. Endocrinol. Metab. 17, S254–S256. doi:10.4103/2230-8210.119591
Ahn H.-Y., Choo Y.-M., Cho Y.-S. (2018). Anti-pigmentation effects of eight phellinus linteus-fermented traditional crude herbal extracts on Brown Guinea pigs of ultraviolet B-induced hyperpigmentation. J. Microbiol. Biotechnol. 28, 375–380. doi:10.4014/jmb.1711.11043
Albaghdadi M., Thibodeau M. L., Lara-Corrales I. (2022). Updated Approach to Patients with Multiple Café au Lait Macules. Dermatol. Clin. 40, 9–23. doi:10.1016/j.det.2021.08.002
Alesiani D., Cicconi R., Mattei M., Bei R., Canini A. (2009). Inhibition of Mek 1/2 kinase activity and stimulation of melanogenesis by 5,7-dimethoxycoumarin treatment of melanoma cells. Int. J. Oncol. 34, 1727–1735. doi:10.3892/ijo_00000303
Allison R. R., Sibata C. H. (2010). Photodynamic therapy: mechanism of action and role in the treatment of skin disease. G. Ital. Dermatol Venereol. 145, 491–507.
Amini-Nik S., Dolp R., Eylert G., Datu A.-K., Parousis A., Blakeley C., et al. (2018). Stem cells derived from burned skin - the future of burn care. EBioMedicine 37, 509–520. doi:10.1016/j.ebiom.2018.10.014
Anelli V., Santoriello C., Distel M., Köster R. W., Ciccarelli F. D., Mione M. (2009). Global repression of cancer gene expression in a zebrafish model of melanoma is linked to epigenetic regulation. Zebrafish 6, 417–424. doi:10.1089/zeb.2009.0612
Annaby M. H., Elwer A. M., Rushdi M. A., Rasmy M. E. M. (2021). Melanoma detection using spatial and spectral analysis on superpixel graphs. J. Digit. Imaging 34, 162–181. doi:10.1007/s10278-020-00401-6
Avadhani K. S., Manikkath J., Tiwari M., Chandrasekhar M., Godavarthi A., Vidya S. M., et al. (2017). Skin delivery of epigallocatechin-3-gallate (EGCG) and hyaluronic acid loaded nano-transfersomes for antioxidant and anti-aging effects in UV radiation induced skin damage. Drug Deliv. 24, 61–74. doi:10.1080/10717544.2016.1228718
Baek S., Lee S.-H. (2016). Omeprazole inhibits melanin biosynthesis in melan-a cells and zebrafish. Exp. Dermatol 25, 239–241. doi:10.1111/exd.12915
Bardeesy N., Bastian B. C., Hezel A., Pinkel D., DePinho R. A., Chin L. (2001). Dual inactivation of RB and p53 pathways in RAS-induced melanomas. Mol. Cell. Biol. 21, 2144–2153. doi:10.1128/MCB.21.6.2144-2153.2001
Blumberg P. M. (1988). Protein kinase C as the receptor for the phorbol ester tumor promoters: sixth Rhoads memorial award lecture. Cancer Res. 48, 1–8. Available at: https://pubmed.ncbi.nlm.nih.gov/3275491/(Accessed April 3, 2023).
Bolanca I., Bolanca Z., Kuna K., Vuković A., Tuckar N., Herman R., et al. (2008). Chloasma--the mask of pregnancy. Coll. Antropol. 32 (Suppl. 2), 139–141.
Budi E. H., Patterson L. B., Parichy D. M. (2008). Embryonic requirements for ErbB signaling in neural crest development and adult pigment pattern formation. Development 135, 2603–2614. doi:10.1242/dev.019299
Ceol C. J., Houvras Y., White R. M., Zon L. I. (2008). Melanoma biology and the promise of zebrafish. Zebrafish 5, 247–255. doi:10.1089/zeb.2008.0544
Chae J. K., Subedi L., Jeong M., Park Y. U., Kim C. Y., Kim H., et al. (2017). Gomisin N inhibits melanogenesis through regulating the PI3K/akt and MAPK/ERK signaling pathways in melanocytes. Int. J. Mol. Sci. 18, 471. doi:10.3390/ijms18020471
Chakraborty C., Hsu C. H., Wen Z. H., Lin C. S., Agoramoorthy G. (2009). Zebrafish: a complete animal model for in vivo drug discovery and development. Curr. Drug Metab. 10, 116–124. doi:10.2174/138920009787522197
Chakravarti S., Magnuson T., Lass J. H., Jepsen K. J., LaMantia C., Carroll H. (1998). Lumican regulates collagen fibril assembly: skin fragility and corneal opacity in the absence of lumican. J. Cell Biol. 141, 1277–1286. doi:10.1083/jcb.141.5.1277
Chen B., Chen H., Qu H., Qiao K., Xu M., Wu J., et al. (2022). Photoprotective effects of Sargassum thunbergii on ultraviolet B-induced mouse L929 fibroblasts and zebrafish. BMC Complement. Med. Ther. 22, 144. doi:10.1186/s12906-022-03609-x
Chen Y.-H., Chen Y.-J., Chou C.-Y., Wen C.-C., Cheng C.-C. (2019). UV-protective activities of pineapple leaf extract in zebrafish embryos. Res. Chem. Intermed. 45, 65–75. doi:10.1007/s11164-018-3632-5
Chiaverini C., Beuret L., Flori E., Busca R., Abbe P., Bille K., et al. (2008). Microphthalmia-associated transcription factor regulates RAB27A gene expression and controls melanosome transport. J. Biol. Chem. 283, 12635–12642. doi:10.1074/jbc.M800130200
Cho S.-E., Kim Y.-M., Kang K.-H., Kim S.-C., Park J.-K., Seo Y.-K. (2016). Pigmentation effect of electromagnetic fields at various intensities to melanocytes. Tissue Eng. Regen. Med. 13, 560–567. doi:10.1007/s13770-016-0090-2
Cortés H., Rojas-Márquez M., Del Prado-Audelo M. L., Reyes-Hernández O. D., González-Del Carmen M., Leyva-Gómez G. (2022). Alterations in mental health and quality of life in patients with skin disorders: a narrative review. Int. J. Dermatol 61, 783–791. doi:10.1111/ijd.15852
Costin G.-E., Hearing V. J. (2007). Human skin pigmentation: melanocytes modulate skin color in response to stress. FASEB J. 21, 976–994. doi:10.1096/fj.06-6649rev
Cox S. V., Zachary C. B., Cohen J. L. (2021). Skin preparation for photodynamic therapy, chemexfoliation, and ablative laser resurfacing: a systematic literature review. Dermatol Surg. 47, 938–941. doi:10.1097/DSS.0000000000003026
Dabas G., Vinay K., Parsad D., Kumar A., Kumaran M. S. (2020). Psychological disturbances in patients with pigmentary disorders: a cross-sectional study. J. Eur. Acad. Dermatol Venereol. 34, 392–399. doi:10.1111/jdv.15987
Dailey H. A., Meissner P. N. (2013). Erythroid heme biosynthesis and its disorders. Cold Spring Harb. Perspect. Med. 3, a011676. doi:10.1101/cshperspect.a011676
Danescu S., Salavastru C., Tiplica G. S., Fritz K. (2020). Treatment of pigmentation disorders in association with systemic diseases. Hautarzt 71, 932–943. doi:10.1007/s00105-020-04715-y
Daniel E., Debono M., Caunt S., Girio-Fragkoulakis C., Walters S. J., Akker S. A., et al. (2018). A prospective longitudinal study of Pasireotide in Nelson’s syndrome. Pituitary 21, 247–255. doi:10.1007/s11102-017-0853-3
de Gruijl F. R. (2017). UV adaptation: pigmentation and protection against overexposure. Exp. Dermatol 26, 557–562. doi:10.1111/exd.13332
del Ama L. F., Jones M., Walker P., Chapman A., Braun J. A., Mohr J., et al. (2016). Reprofiling using a zebrafish melanoma model reveals drugs cooperating with targeted therapeutics. Oncotarget 7, 40348–40361. doi:10.18632/oncotarget.9613
Del Bino S., Duval C., Bernerd F. (2018). Clinical and biological characterization of skin pigmentation diversity and its consequences on UV impact. Int. J. Mol. Sci. 19, 2668. doi:10.3390/ijms19092668
Denti P., Wasmann R. E., van Rie A., Winckler J., Bekker A., Rabie H., et al. (2022). Optimizing dosing and fixed-dose combinations of rifampicin, isoniazid, and pyrazinamide in pediatric patients with tuberculosis: a prospective population pharmacokinetic study. Clin. Infect. Dis. 75, 141–151. doi:10.1093/cid/ciab908
Dhomen N., Reis-Filho J. S., da Rocha Dias S., Hayward R., Savage K., Delmas V., et al. (2009). Oncogenic Braf induces melanocyte senescence and melanoma in mice. Cancer Cell 15, 294–303. doi:10.1016/j.ccr.2009.02.022
Ding Q., Luo L., Yu L., Huang S.-L., Wang X.-Q., Zhang B. (2021a). The critical role of glutathione redox homeostasis towards oxidation in ermanin-induced melanogenesis. Free Radic. Biol. Med. 176, 392–405. doi:10.1016/j.freeradbiomed.2021.09.017
Ding X.-J., Zhang Z.-Y., Jin J., Han J.-X., Wang Y., Yang K., et al. (2020). Salidroside can target both P4HB-mediated inflammation and melanogenesis of the skin. Theranostics 10, 11110–11126. doi:10.7150/thno.47413
Ding Y., Jiang Y., Im S. T., Myung S., Kim H.-S., Lee S.-H. (2021b). Diphlorethohydroxycarmalol inhibits melanogenesis via protein kinase A/cAMP response element-binding protein and extracellular signal-regulated kinase-mediated microphthalmia-associated transcription factor downregulation in α-melanocyte stimulating hormone-stimulated B16F10 melanoma cells and zebrafish. Cell Biochem. Funct. 39, 546–554. doi:10.1002/cbf.3620
Distel M., Wullimann M. F., Köster R. W. (2009). Optimized Gal4 genetics for permanent gene expression mapping in zebrafish. Proc. Natl. Acad. Sci. U. S. A. 106, 13365–13370. doi:10.1073/pnas.0903060106
D’Mello S. A. N., Finlay G. J., Baguley B. C., Askarian-Amiri M. E. (2016). Signaling pathways in melanogenesis. Int. J. Mol. Sci. 17, 1144. doi:10.3390/ijms17071144
Dong X.-R., Wan S.-M., Zhou J.-J., Nie C.-H., Chen Y.-L., Diao J.-H., et al. (2022). Functional differentiation of BMP7 genes in zebrafish: bmp7a for dorsal-ventral pattern and bmp7b for melanin synthesis and eye development. Front. Cell Dev. Biol. 10, 838721. doi:10.3389/fcell.2022.838721
Du Y., Guo Q., Shan M., Wu Y., Huang S., Zhao H., et al. (2016). Spatial and temporal distribution of dopaminergic neurons during development in zebrafish. Front. Neuroanat. 10, 115. doi:10.3389/fnana.2016.00115
Duarah S., Durai R. D., Narayanan V. B. (2017). Nanoparticle-in-gel system for delivery of vitamin C for topical application. Drug Deliv. Transl. Res. 7, 750–760. doi:10.1007/s13346-017-0398-z
Ducy P., Karsenty G. (2000). The family of bone morphogenetic proteins. Kidney Int. 57, 2207–2214. doi:10.1046/j.1523-1755.2000.00081.x
Ekore R. I., Ekore J. O. (2021). Excoriation (skin-picking) disorder among adolescents and young adults with acne-induced postinflammatory hyperpigmentation and scars. Int. J. Dermatol 60, 1488–1493. doi:10.1111/ijd.15587
Elmets C. A., Yusuf N., Hamza S., Iranikakh N., Smith J., Volk A. L., et al. (2004). Topical application of dimethylbenz[a]anthracene results in the generation of multiple melanocytic nevi in C3H/HeN mice. Toxicol. Appl. Pharmacol. 195, 355–360. doi:10.1016/j.taap.2003.09.029
Filoni A., Mariano M., Cameli N. (2019). Melasma: how hormones can modulate skin pigmentation. J. Cosmet. Dermatol 18, 458–463. doi:10.1111/jocd.12877
Frohnhöfer H. G., Krauss J., Maischein H.-M., Nüsslein-Volhard C. (2013). Iridophores and their interactions with other chromatophores are required for stripe formation in zebrafish. Development 140, 2997–3007. doi:10.1242/dev.096719
Galivo F., Diaz R. M., Wongthida P., Thompson J., Kottke T., Barber G., et al. (2010). Single-cycle viral gene expression, rather than progressive replication and oncolysis, is required for VSV therapy of B16 melanoma. Gene Ther. 17, 158–170. doi:10.1038/gt.2009.161
Gils T., Lynen L., de Jong B. C., Van Deun A., Decroo T. (2022). Pretomanid for tuberculosis: a systematic review. Clin. Microbiol. Infect. 28, 31–42. doi:10.1016/j.cmi.2021.08.007
Giménez García R. M., Carrasco Molina S. (2019). Drug-induced hyperpigmentation: review and case series. J. Am. Board Fam. Med. 32, 628–638. doi:10.3122/jabfm.2019.04.180212
Gledhill K., Rhodes L. E., Brownrigg M., Haylett A. K., Masoodi M., Thody A. J., et al. (2010). Prostaglandin-E2 is produced by adult human epidermal melanocytes in response to UVB in a melanogenesis-independent manner. Pigment. Cell Melanoma Res. 23, 394–403. doi:10.1111/j.1755-148X.2010.00696.x
Goody M. F., Carter E. V., Kilroy E. A., Maves L., Henry C. A. (2017). “Muscling” throughout life: integrating studies of muscle development, homeostasis, and disease in zebrafish. Curr. Top. Dev. Biol. 124, 197–234. doi:10.1016/bs.ctdb.2016.11.002
Gore A. V., Pillay L. M., Venero Galanternik M., Weinstein B. M. (2018). The zebrafish: a fintastic model for hematopoietic development and disease. Wiley Interdiscip. Rev. Dev. Biol. 7, e312. doi:10.1002/wdev.312
Guo H., Zeng H., Hu Y., Jiang L., Lei L., Hung J., et al. (2023). UVB promotes melanogenesis by regulating METTL3. J. Cell Physiol. 238, 2161–2171. doi:10.1002/jcp.31077
Haffter P., Granato M., Brand M., Mullins M. C., Hammerschmidt M., Kane D. A., et al. (1996). The identification of genes with unique and essential functions in the development of the zebrafish, Danio rerio. Development 123, 1–36. doi:10.1242/dev.123.1.1
Hantash B. M., Bedi V. P., Sudireddy V., Struck S. K., Herron G. S., Chan K. F. (2006). Laser-induced transepidermal elimination of dermal content by fractional photothermolysis. J. Biomed. Opt. 11, 041115. doi:10.1117/1.2241745
Hawkins T. A., Cavodeassi F., Erdélyi F., Szabó G., Lele Z. (2008). The small molecule Mek1/2 inhibitor U0126 disrupts the chordamesoderm to notochord transition in zebrafish. BMC Dev. Biol. 8, 42. doi:10.1186/1471-213X-8-42
Hirata M., Nakamura K., Kanemaru T., Shibata Y., Kondo S. (2003). Pigment cell organization in the hypodermis of zebrafish. Dev. Dyn. 227, 497–503. doi:10.1002/dvdy.10334
Hirata M., Nakamura K.-I., Kondo S. (2005). Pigment cell distributions in different tissues of the zebrafish, with special reference to the striped pigment pattern. Dev. Dyn. 234, 293–300. doi:10.1002/dvdy.20513
Hong C., Yang L., Zhang Y., Li Y., Wu H. (2022). Epimedium brevicornum Maxim. Extract exhibits pigmentation by melanin biosynthesis and melanosome biogenesis/transfer. Front. Pharmacol. 13, 963160. doi:10.3389/fphar.2022.963160
Hosking A.-M., Coakley B. J., Chang D., Talebi-Liasi F., Lish S., Lee S. W., et al. (2019). Hyperspectral imaging in automated digital dermoscopy screening for melanoma. Lasers Surg. Med. 51, 214–222. doi:10.1002/lsm.23055
Hu S., Huang J., Pei S., Ouyang Y., Ding Y., Jiang L., et al. (2019). Ganoderma lucidum polysaccharide inhibits UVB-induced melanogenesis by antagonizing cAMP/PKA and ROS/MAPK signaling pathways. J. Cell Physiol. 234, 7330–7340. doi:10.1002/jcp.27492
Hubmann R., Sieghart W., Schnabl S., Araghi M., Hilgarth M., Reiter M., et al. (2017). Gliotoxin targets nuclear NOTCH2 in human solid tumor derived cell lines in vitro and inhibits melanoma growth in xenograft mouse model. Front. Pharmacol. 8, 319. doi:10.3389/fphar.2017.00319
Hultman K. A., Johnson S. L. (2010). Differential contribution of direct-developing and stem cell-derived melanocytes to the zebrafish larval pigment pattern. Dev. Biol. 337, 425–431. doi:10.1016/j.ydbio.2009.11.019
Hushcha Y., Blo I., Oton-Gonzalez L., Mauro G. D., Martini F., Tognon M., et al. (2021). microRNAs in the regulation of melanogenesis. Int. J. Mol. Sci. 22, 6104. doi:10.3390/ijms22116104
Hwang Y. S., Kim Y.-J., Kim M. O., Kang M., Oh S. W., Nho Y. H., et al. (2017). Cannabidiol upregulates melanogenesis through CB1 dependent pathway by activating p38 MAPK and p42/44 MAPK. Chem. Biol. Interact. 273, 107–114. doi:10.1016/j.cbi.2017.06.005
Jang H.-J., Seo Y.-K. (2016). Pigmentation effect of rice bran extracted minerals comprising soluble silicic acids. Evid. Based Complement. Altern. Med. 2016, 3137486. doi:10.1155/2016/3137486
Jang Y. H., Lee J. Y., Kang H. Y., Lee E.-S., Kim Y. C. (2010). Oestrogen and progesterone receptor expression in melasma: an immunohistochemical analysis. J. Eur. Acad. Dermatol Venereol. 24, 1312–1316. doi:10.1111/j.1468-3083.2010.03638.x
Jin E. J., Erickson C. A., Takada S., Burrus L. W. (2001). Wnt and BMP signaling govern lineage segregation of melanocytes in the avian embryo. Dev. Biol. 233, 22–37. doi:10.1006/dbio.2001.0222
Jin E. J., Thibaudeau G. (1999). Effects of lithium on pigmentation in the embryonic zebrafish (Brachydanio rerio). Biochim. Biophys. Acta 1449, 93–99. doi:10.1016/s0167-4889(98)00176-1
Jung E., Kim J. H., Kim M. O., Jang S., Kang M., Oh S. W., et al. (2016). Afzelin positively regulates melanogenesis through the p38 MAPK pathway. Chem. Biol. Interact. 254, 167–172. doi:10.1016/j.cbi.2016.06.010
Kapsokalyvas D., Bruscino N., Alfieri D., de Giorgi V., Cannarozzo G., Cicchi R., et al. (2013). Spectral morphological analysis of skin lesions with a polarization multispectral dermoscope. Opt. Express 21, 4826–4840. doi:10.1364/OE.21.004826
Karunarathne W. A. H. M., Molagoda I. M. N., Kim M. S., Choi Y. H., Oren M., Park E. K., et al. (2019). Flumequine-mediated upregulation of p38 MAPK and JNK results in melanogenesis in B16F10 cells and zebrafish larvae. Biomolecules 9, 596. doi:10.3390/biom9100596
Kelsh R. N., Harris M. L., Colanesi S., Erickson C. A. (2009). Stripes and belly-spots -- a review of pigment cell morphogenesis in vertebrates. Semin. Cell Dev. Biol. 20, 90–104. doi:10.1016/j.semcdb.2008.10.001
Khan H., Marya null, Amin S., Kamal M. A., Patel S. (2018). Flavonoids as acetylcholinesterase inhibitors: current therapeutic standing and future prospects. Biomed. Pharmacother. 101, 860–870. doi:10.1016/j.biopha.2018.03.007
Kim B.-H., Park K. C., Park J. H., Lee C. G., Ye S.-K., Park J. Y. (2016a). Inhibition of tyrosinase activity and melanin production by the chalcone derivative 1-(2-cyclohexylmethoxy-6-hydroxy-phenyl)-3-(4-hydroxymethyl-phenyl)-propenone. Biochem. Biophys. Res. Commun. 480, 648–654. doi:10.1016/j.bbrc.2016.10.110
Kim H. M., Oh S., Byun K.-A., Yang J. Y., Sun H. J., Kang D., et al. (2022). Radiofrequency irradiation mitigated UV-B-induced skin pigmentation by increasing lymphangiogenesis. Molecules 27, 454. doi:10.3390/molecules27020454
Kim J., Lee Y. I., Kim J., Jung J. Y., Lee W. J., Lee J. H. (2020). Safety of combined fractional microneedle radiofrequency and CO2 as an early intervention for inflammatory acne and scarring treated with concomitant isotretinoin. Dermatol Surg. 46, e71–e77. doi:10.1097/DSS.0000000000002364
Kim K.-S., Kim J. A., Eom S.-Y., Lee S. H., Min K. R., Kim Y. (2006). Inhibitory effect of piperlonguminine on melanin production in melanoma B16 cell line by downregulation of tyrosinase expression. Pigment. Cell Res. 19, 90–98. doi:10.1111/j.1600-0749.2005.00281.x
Kim T.-H., Kim J.-Y., Bae J., Kim Y.-M., Won M.-H., Ha K.-S., et al. (2021). Korean Red ginseng prevents endothelial senescence by downregulating the HO-1/NF-κB/miRNA-155-5p/eNOS pathway. J. Ginseng Res. 45, 344–353. doi:10.1016/j.jgr.2020.08.002
Kim Y.-M., Cho S.-E., Kim S.-C., Jang H.-J., Seo Y.-K. (2017). Effects of extremely low frequency electromagnetic fields on melanogenesis through p-ERK and p-SAPK/JNK pathways in human melanocytes. Int. J. Mol. Sci. 18, 2120. doi:10.3390/ijms18102120
Kim Y.-M., Cho S.-E., Seo Y.-K. (2016b). The activation of melanogenesis by p-CREB and MITF signaling with extremely low-frequency electromagnetic fields on B16F10 melanoma. Life Sci. 162, 25–32. doi:10.1016/j.lfs.2016.08.015
Kim Y.-M., Lee E.-C., Lim H.-M., Seo Y.-K. (2019). Rice bran ash mineral extract increases pigmentation through the p-ERK pathway in zebrafish (Danio rerio). Int. J. Mol. Sci. 20, 2172. doi:10.3390/ijms20092172
Kim Y.-M., Lim H.-M., Ro H.-S., Ki G.-E., Seo Y.-K. (2018). Pulsed electromagnetic fields increase pigmentation through the p-ERK/p-p38 pathway in zebrafish (Danio rerio). Int. J. Mol. Sci. 19, 3211. doi:10.3390/ijms19103211
Kondo S., Shirota H. (2009). Theoretical analysis of mechanisms that generate the pigmentation pattern of animals. Semin. Cell Dev. Biol. 20, 82–89. doi:10.1016/j.semcdb.2008.10.008
Krotova K., Kuoch Yoshitomi H., Caine C., Aslanidi G. (2023). Tumor antigen-loaded AAV vaccine drives protective immunity in a melanoma animal model. Mol. Ther. Methods Clin. Dev. 28, 301–311. doi:10.1016/j.omtm.2023.01.006
Kutlubay Z., Cesur S. K., Aşkın Ö., Tüzün Y. (2019). The color of skin: brown diseases of the skin, nails, and mucosa. Clin. Dermatology 37, 487–506. doi:10.1016/j.clindermatol.2019.07.007
Kuzu O. F., Nguyen F. D., Noory M. A., Sharma A. (2015). Current state of animal (mouse) modeling in melanoma research. Cancer Growth Metastasis 8, 81–94. doi:10.4137/CGM.S21214
Kwon S.-J., Kim Y.-M., Jang H.-J., Seo Y.-K. (2018). Synergistic effect of rice bran extract and extremely low-frequency electromagnetic fields on dermal papilla/melanocytes in melanogenesis. Bioelectromagnetics 39, 595–603. doi:10.1002/bem.22151
Lajis A. F. B. (2018). A zebrafish embryo as an animal model for the treatment of hyperpigmentation in cosmetic Dermatology medicine. Medicina 54, 35. doi:10.3390/medicina54030035
Lajis A. F. B., Ariff A. B. (2019). Discovery of new depigmenting compounds and their efficacy to treat hyperpigmentation: evidence from in vitro study. J. Cosmet. Dermatol 18, 703–727. doi:10.1111/jocd.12900
Lawson N. D., Weinstein B. M. (2002). In vivo imaging of embryonic vascular development using transgenic zebrafish. Dev. Biol. 248, 307–318. doi:10.1006/dbio.2002.0711
Lee A.-Y. (2015). Recent progress in melasma pathogenesis. Pigment. Cell Melanoma Res. 28, 648–660. doi:10.1111/pcmr.12404
Lee A.-Y. (2021). Skin pigmentation abnormalities and their possible relationship with skin aging. Int. J. Mol. Sci. 22, 3727. doi:10.3390/ijms22073727
Lee C. S., Park M., Han J., Lee J.-H., Bae I.-H., Choi H., et al. (2013). Liver X receptor activation inhibits melanogenesis through the acceleration of ERK-mediated MITF degradation. J. Invest. Dermatol 133, 1063–1071. doi:10.1038/jid.2012.409
Lee H. J., Park M. K., Kim S. Y., Park Choo H. Y., Lee A. Y., Lee C. H. (2011). Serotonin induces melanogenesis via serotonin receptor 2A. Br. J. Dermatol 165, 1344–1348. doi:10.1111/j.1365-2133.2011.10490.x
Le Guellec D., Morvan-Dubois G., Sire J.-Y. (2004). Skin development in bony fish with particular emphasis on collagen deposition in the dermis of the zebrafish (Danio rerio). Int. J. Dev. Biol. 48, 217–231. doi:10.1387/ijdb.15272388
Lei P., Zhang W., Ma J., Xia Y., Yu H., Du J., et al. (2023). Advances in the utilization of zebrafish for assessing and understanding the mechanisms of nano-/microparticles toxicity in water. Toxics 11, 380. doi:10.3390/toxics11040380
Li Q., Frank M., Thisse C. I., Thisse B. V., Uitto J. (2011). Zebrafish: a model system to study heritable skin diseases. J. Invest. Dermatol 131, 565–571. doi:10.1038/jid.2010.388
Liang G., Liu M., Wang Q., Shen Y., Mei H., Li D., et al. (2017). Itraconazole exerts its anti-melanoma effect by suppressing Hedgehog, Wnt, and PI3K/mTOR signaling pathways. Oncotarget 8, 28510–28525. doi:10.18632/oncotarget.15324
Lin B., Ma J., Fang Y., Lei P., Wang L., Qu L., et al. (2023). Advances in zebrafish for diabetes mellitus with wound model. Bioeng. (Basel) 10, 330. doi:10.3390/bioengineering10030330
Lin C.-Y., Chiang C.-Y., Tsai H.-J. (2016). Zebrafish and Medaka: new model organisms for modern biomedical research. J. Biomed. Sci. 23, 19. doi:10.1186/s12929-016-0236-5
Lin F., Song J., Huang Y., Zhai Y., Yao X., Yang B. (2022a). Successful treatment of microneedle fractional radiofrequency for refractory depressed scars in buttocks: a case report. J. Cosmet. Dermatol 21, 3629–3630. doi:10.1111/jocd.14979
Lin F.-J., Li H., Wu D.-T., Zhuang Q.-G., Li H.-B., Geng F., et al. (2022b). Recent development in zebrafish model for bioactivity and safety evaluation of natural products. Crit. Rev. Food Sci. Nutr. 62, 8646–8674. doi:10.1080/10408398.2021.1931023
Liu F., Qu L., Li H., He J., Wang L., Fang Y., et al. (2022). Advances in biomedical functions of natural whitening substances in the treatment of skin pigmentation diseases. Pharmaceutics 14, 2308. doi:10.3390/pharmaceutics14112308
Liu L., Fu M., Pei S., Zhou L., Shang J. (2018). R-fluoxetine increases melanin synthesis through a 5-HT1A/2A receptor and p38 MAPK signaling pathways. Int. J. Mol. Sci. 20, 80. doi:10.3390/ijms20010080
Liu L., Zhong M., Dong J., Chen M., Shang J., Yue Y. (2020). 5-Hydroxytryptamine (5-HT) positively regulates pigmentation via inducing melanoblast specification and melanin synthesis in zebrafish embryos. Biomolecules 10, 1344. doi:10.3390/biom10091344
Liu W.-S., Kuan Y.-D., Chiu K.-H., Wang W.-K., Chang F.-H., Liu C.-H., et al. (2013). The extract of Rhodobacter sphaeroides inhibits melanogenesis through the MEK/ERK signaling pathway. Mar. Drugs 11, 1899–1908. doi:10.3390/md11061899
Liu Y., Guo Y., Zhu Y., An D., Gao W., Wang Z., et al. (2011). A sustainable route for the preparation of activated carbon and silica from rice husk ash. J. Hazard Mater 186, 1314–1319. doi:10.1016/j.jhazmat.2010.12.007
Liu Z., Tang W., Liu J., Han Y., Yan Q., Dong Y., et al. (2023). A novel sprayable thermosensitive hydrogel coupled with zinc modified metformin promotes the healing of skin wound. Bioact. Mater 20, 610–626. doi:10.1016/j.bioactmat.2022.06.008
Logan D. W., Burn S. F., Jackson I. J. (2006). Regulation of pigmentation in zebrafish melanophores. Pigment. Cell Res. 19, 206–213. doi:10.1111/j.1600-0749.2006.00307.x
Lv J., An X., Jiang S., Yang Y., Song G., Gao R. (2020a). Protoporphyrin IX stimulates melanogenesis, melanocyte dendricity, and melanosome transport through the cGMP/PKG pathway. Front. Pharmacol. 11, 569368. doi:10.3389/fphar.2020.569368
Lv J., Fu Y., Cao Y., Jiang S., Yang Y., Song G., et al. (2020b). Isoliquiritigenin inhibits melanogenesis, melanocyte dendricity and melanosome transport by regulating ERK-mediated MITF degradation. Exp. Dermatol 29, 149–157. doi:10.1111/exd.14066
MacRae C. A., Peterson R. T. (2015). Zebrafish as tools for drug discovery. Nat. Rev. Drug Discov. 14, 721–731. doi:10.1038/nrd4627
Maderspacher F., Nüsslein-Volhard C. (2003). Formation of the adult pigment pattern in zebrafish requires leopard and obelix dependent cell interactions. Development 130, 3447–3457. doi:10.1242/dev.00519
McMenamin S. K., Bain E. J., McCann A. E., Patterson L. B., Eom D. S., Waller Z. P., et al. (2014). Thyroid hormone-dependent adult pigment cell lineage and pattern in zebrafish. Science 345, 1358–1361. doi:10.1126/science.1256251
Min S., Park S. Y., Yoon J. Y., Kwon H. H., Suh D. H. (2016). Fractional microneedling radiofrequency treatment for acne-related post-inflammatory erythema. Acta Derm. Venereol. 96, 87–91. doi:10.2340/00015555-2164
Mobasher P., Foulad D. P., Raffi J., Zachary C., Fackler N., Zohuri N., et al. (2020). Catamenial hyperpigmentation: a review. J. Clin. Aesthet. Dermatol 13, 18–21.
Molagoda I. M. N., Karunarathne W. A. H. M., Park S. R., Choi Y. H., Park E. K., Jin C.-Y., et al. (2020). GSK-3β-Targeting fisetin promotes melanogenesis in B16F10 melanoma cells and zebrafish larvae through β-catenin activation. Int. J. Mol. Sci. 21, 312. doi:10.3390/ijms21010312
Morelli J. G., Yohn J. J., Lyons M. B., Murphy R. C., Norris D. A. (1989). Leukotrienes C4 and D4 as potent mitogens for cultured human neonatal melanocytes. J. Invest. Dermatol 93, 719–722. doi:10.1111/1523-1747.ep12284392
Nahhas A. F., Braunberger T. L., Hamzavi I. H. (2019). An update on drug-induced pigmentation. Am. J. Clin. Dermatol 20, 75–96. doi:10.1007/s40257-018-0393-2
Nietsche U. B. (1978). Photochemotherapy of psoriasis. Int. J. Dermatol 17, 149–157. doi:10.1111/j.1365-4362.1978.tb06127.x
Nishizawa A., Maruta Y., Fukuda M. (2022). Rab32/38-Dependent and -independent transport of tyrosinase to melanosomes in B16-F1 melanoma cells. Int. J. Mol. Sci. 23, 14144. doi:10.3390/ijms232214144
Niu C., Yin L., Aisa H. A. (2018). Novel furocoumarin derivatives stimulate melanogenesis in B16 melanoma cells by up-regulation of MITF and TYR family via akt/gsk3β/β-catenin signaling pathways. Int. J. Mol. Sci. 19, 746. doi:10.3390/ijms19030746
Noonan F. P., Recio J. A., Takayama H., Duray P., Anver M. R., Rush W. L., et al. (2001). Neonatal sunburn and melanoma in mice. Nature 413, 271–272. doi:10.1038/35095108
Ozkaynak E., Rueger D. C., Drier E. A., Corbett C., Ridge R. J., Sampath T. K., et al. (1990). OP-1 cDNA encodes an osteogenic protein in the TGF-beta family. EMBO J. 9, 2085–2093. doi:10.1002/j.1460-2075.1990.tb07376.x
Park J. J., Hwang S. J., Kang Y. S., Jung J., Park S., Hong J. E., et al. (2019). Synthesis of arbutin-gold nanoparticle complexes and their enhanced performance for whitening. Arch. Pharm. Res. 42, 977–989. doi:10.1007/s12272-019-01164-7
Park S. Y., Kim Y. H., Kim Y. H., Park G., Lee S.-J. (2010). Beta-carboline alkaloids harmaline and harmalol induce melanogenesis through p38 mitogen-activated protein kinase in B16F10 mouse melanoma cells. BMB Rep. 43, 824–829. doi:10.5483/BMBRep.2010.43.12.824
Patterson L. B., Parichy D. M. (2013). Interactions with iridophores and the tissue environment required for patterning melanophores and xanthophores during zebrafish adult pigment stripe formation. PLoS Genet. 9, e1003561. doi:10.1371/journal.pgen.1003561
Patterson L. B., Parichy D. M. (2019). Zebrafish pigment pattern formation: insights into the development and evolution of adult form. Annu. Rev. Genet. 53, 505–530. doi:10.1146/annurev-genet-112618-043741
Perez D. E., Henle A. M., Amsterdam A., Hagen H. R., Lees D. J. A. (2018). Uveal melanoma driver mutations in GNAQ/11 yield numerous changes in melanocyte biology. Pigment Cell and melanoma Res. 31, 604–613. doi:10.1111/pcmr.12700
Pillaiyar T., Manickam M., Jung S.-H. (2017). Downregulation of melanogenesis: drug discovery and therapeutic options. Drug Discov. Today 22, 282–298. doi:10.1016/j.drudis.2016.09.016
Quigley I. K., Turner J. M., Nuckels R. J., Manuel J. L., Budi E. H., MacDonald E. L., et al. (2004). Pigment pattern evolution by differential deployment of neural crest and post-embryonic melanophore lineages in Danio fishes. Development 131, 6053–6069. doi:10.1242/dev.01526
Raposo G., Marks M. S. (2007). Melanosomes--dark organelles enlighten endosomal membrane transport. Nat. Rev. Mol. Cell Biol. 8, 786–797. doi:10.1038/nrm2258
Raveendra L., Sidappa H., Shree S. (2020). A study of quality of life in patients with facial melanoses. Indian Dermatol Online J. 11, 154–157. doi:10.4103/idoj.IDOJ_209_19
Richardson J., Lundegaard P. R., Reynolds N. L., Dorin J. R., Porteous D. J., Jackson I. J., et al. (2008). mc1r Pathway regulation of zebrafish melanosome dispersion. Zebrafish 5, 289–295. doi:10.1089/zeb.2008.0541
Rigopoulos D., Gregoriou S., Katsambas A. (2007). Hyperpigmentation and melasma. J. Cosmet. Dermatol 6, 195–202. doi:10.1111/j.1473-2165.2007.00321.x
Robinson K. C., Fisher D. E. (2009). Specification and loss of melanocyte stem cells. Semin. Cell Dev. Biol. 20, 111–116. doi:10.1016/j.semcdb.2008.11.016
Roméro-Graillet C., Aberdam E., Biagoli N., Massabni W., Ortonne J. P., Ballotti R. (1996). Ultraviolet B radiation acts through the nitric oxide and cGMP signal transduction pathway to stimulate melanogenesis in human melanocytes. J. Biol. Chem. 271, 28052–28056. doi:10.1074/jbc.271.45.28052
Rosa J. G. S., Lima C., Lopes-Ferreira M. (2022). Zebrafish larvae behavior models as a tool for drug screenings and pre-clinical trials: a review. Int. J. Mol. Sci. 23, 6647. doi:10.3390/ijms23126647
Rothhammer T., Poser I., Soncin F., Bataille F., Moser M., Bosserhoff A.-K. (2005). Bone morphogenic proteins are overexpressed in malignant melanoma and promote cell invasion and migration. Cancer Res. 65, 448–456. doi:10.1158/0008-5472.448.65.2
Ryu A., Arakane K., Koide C., Arai H., Nagano T. (2009). Squalene as a target molecule in skin hyperpigmentation caused by singlet oxygen. Biol. Pharm. Bull. 32, 1504–1509. doi:10.1248/bpb.32.1504
Sachar M., Anderson K. E., Ma X. (2016). Protoporphyrin IX: the good, the bad, and the ugly. J. Pharmacol. Exp. Ther. 356, 267–275. doi:10.1124/jpet.115.228130
Santoriello C., Gennaro E., Anelli V., Distel M., Kelly A., Hurlstone A., et al. (2010). Kita driven expression of oncogenic HRAS leads to early onset and highly penetrant melanoma in zebrafish. PloS one 5, e15170. doi:10.1371/journal.pone.0015170
Sarkar R., Puri P., Jain R. K., Singh A., Desai A. (2010). Melasma in men: a clinical, aetiological and histological study. J. Eur. Acad. Dermatol Venereol. 24, 768–772. doi:10.1111/j.1468-3083.2009.03524.x
Scatozza F., Moschella F., D’Arcangelo D., Rossi S., Tabolacci C., Giampietri C., et al. (2020). Nicotinamide inhibits melanoma in vitro and in vivo. J. Exp. Clin. Cancer Res. 39, 211. doi:10.1186/s13046-020-01719-3
Schena F. P., Gesualdo L., Caracciolo G. (1988). A multicentre study of flumequine in the treatment of urinary tract infections. J. Antimicrob. Chemother. 21, 101–106. doi:10.1093/jac/21.1.101
Schepsky A., Bruser K., Gunnarsson G. J., Goodall J., Hallsson J. H., Goding C. R., et al. (2006). The microphthalmia-associated transcription factor Mitf interacts with beta-catenin to determine target gene expression. Mol. Cell Biol. 26, 8914–8927. doi:10.1128/MCB.02299-05
Schmidt T. H., Khanijow K., Cedars M. I., Huddleston H., Pasch L., Wang E. T., et al. (2016). Cutaneous findings and systemic associations in women with polycystic ovary syndrome. JAMA Dermatol 152, 391–398. doi:10.1001/jamadermatol.2015.4498
Shawi M., Serluca F. C. (2008). Identification of a BMP7 homolog in zebrafish expressed in developing organ systems. Gene Expr. Patterns 8, 369–375. doi:10.1016/j.gep.2008.05.004
Shin H., Hong S. D., Roh E., Jung S.-H., Cho W.-J., Park S. H., et al. (2015). cAMP-dependent activation of protein kinase A as a therapeutic target of skin hyperpigmentation by diphenylmethylene hydrazinecarbothioamide. Br. J. Pharmacol. 172, 3434–3445. doi:10.1111/bph.13134
Shu P., Yu M., Li Y., Luo Y., Liu H., Zhu H., et al. (2021). Synthesis of cinnamoyl glucoside derivatives and their antiproliferation activities against murine melanoma B16-F10 cell line. Carbohydr. Res. 504, 108332. doi:10.1016/j.carres.2021.108332
Sialy R., Hassan I., Kaur I., Dash R. J. (2000). Melasma in men: a hormonal profile. J. Dermatol 27, 64–65. doi:10.1111/j.1346-8138.2000.tb02122.x
Silpa-Archa N., Kohli I., Chaowattanapanit S., Lim H. W., Hamzavi I. (2017). Postinflammatory hyperpigmentation: a comprehensive overview: epidemiology, pathogenesis, clinical presentation, and noninvasive assessment technique. J. Am. Acad. Dermatol 77, 591–605. doi:10.1016/j.jaad.2017.01.035
Sire J.-Y., Akimenko M.-A. (2004). Scale development in fish: a review, with description of sonic hedgehog (shh) expression in the zebrafish (Danio rerio). Int. J. Dev. Biol. 48, 233–247. doi:10.1387/ijdb.15272389
Soica C., Danciu C., Savoiu-Balint G., Borcan F., Ambrus R., Zupko I., et al. (2014). Betulinic acid in complex with a gamma-cyclodextrin derivative decreases proliferation and in vivo tumor development of non-metastatic and metastatic B164A5 cells. Int. J. Mol. Sci. 15, 8235–8255. doi:10.3390/ijms15058235
Solano F. (2020). Photoprotection and skin pigmentation: melanin-related molecules and some other new agents obtained from natural sources. Molecules 25, 1537. doi:10.3390/molecules25071537
Sonawane M., Carpio Y., Geisler R., Schwarz H., Maischein H.-M., Nuesslein-Volhard C. (2005). Zebrafish penner/lethal giant larvae 2 functions in hemidesmosome formation, maintenance of cellular morphology and growth regulation in the developing basal epidermis. Development 132, 3255–3265. doi:10.1242/dev.01904
Su Y.-S., Fan Z.-X., Xiao S.-E., Lin B.-J., Miao Y., Hu Z.-Q., et al. (2017). Icariin promotes mouse hair follicle growth by increasing insulin-like growth factor 1 expression in dermal papillary cells. Clin. Exp. Dermatol 42, 287–294. doi:10.1111/ced.13043
Sugumaran M., Evans J., Ito S., Wakamatsu K. (2020). Nonenzymatic spontaneous oxidative transformation of 5,6-dihydroxyindole. Int. J. Mol. Sci. 21, 7321. doi:10.3390/ijms21197321
Tajerian A., Kazemian M., Tajerian M., Akhavan Malayeri A. (2023). Design and validation of a new machine-learning-based diagnostic tool for the differentiation of dermatoscopic skin cancer images. PLoS One 18, e0284437. doi:10.1371/journal.pone.0284437
Takamiya M., Xu F., Suhonen H., Gourain V., Yang L., Ho N. Y., et al. (2016). Melanosomes in pigmented epithelia maintain eye lens transparency during zebrafish embryonic development. Sci. Rep. 6, 25046. doi:10.1038/srep25046
Takekoshi S., Nagata H., Kitatani K. (2014). Flavonoids enhance melanogenesis in human melanoma cells. Tokai J. Exp. Clin. Med. 39, 116–121.
Tang H., Yang L., Wu L., Wang H., Chen K., Wu H., et al. (2021a). Kaempferol, the melanogenic component of Sanguisorba officinalis, enhances dendricity and melanosome maturation/transport in melanocytes. J. Pharmacol. Sci. 147, 348–357. doi:10.1016/j.jphs.2021.08.009
Tang H., Zhang Y., Yang L., Hong C., Chen K., Li Y., et al. (2023). Serotonin/5-HT7 receptor provides an adaptive signal to enhance pigmentation response to environmental stressors through cAMP-PKA-MAPK, Rab27a/RhoA, and PI3K/AKT signaling pathways. FASEB J. 37, e22893. doi:10.1096/fj.202201352RR
Tang X., Dong Q., Li J., Li F., Michniak-Kohn B. B., Zhao D., et al. (2021b). Anti-melanogenic mechanism of tetrahydrocurcumin and enhancing its topical delivery efficacy using a lecithin-based nanoemulsion. Pharmaceutics 13, 1185. doi:10.3390/pharmaceutics13081185
Tarushi A., Lafazanis K., Kljun J., Turel I., Pantazaki A. A., Psomas G., et al. (2013). First- and second-generation quinolone antibacterial drugs interacting with zinc(II): structure and biological perspectives. J. Inorg. Biochem. 121, 53–65. doi:10.1016/j.jinorgbio.2012.12.009
Thapar M., Bonkovsky H. L. (2008). The diagnosis and management of erythropoietic protoporphyria. Gastroenterol. Hepatol. (N Y) 4, 561–566.
Upadhyay P. R., Ho T., Abdel-Malek Z. A. (2021). Participation of keratinocyte- and fibroblast-derived factors in melanocyte homeostasis, the response to UV, and pigmentary disorders. Pigment. Cell Melanoma Res. 34, 762–776. doi:10.1111/pcmr.12985
van der Ent W., Burrello C., Teunisse A. F. A. S., Ksander B. R., van der Velden P. A., Jager M. J., et al. (2014). Modeling of human uveal melanoma in zebrafish xenograft embryos. Invest. Ophthalmol. Vis. Sci. 55, 6612–6622. doi:10.1167/iovs.14-15202
van Hooft J. A., Vijverberg H. P. (2000). 5-HT(3) receptors and neurotransmitter release in the CNS: a nerve ending story? Trends Neurosci. 23, 605–610. doi:10.1016/s0166-2236(00)01662-3
Van Raamsdonk C. D., Bezrookove V., Green G., Bauer J., Gaugler L., O’Brien J. M., et al. (2009). Frequent somatic mutations of GNAQ in uveal melanoma and blue naevi. Nature 457, 599–602. doi:10.1038/nature07586
Van Raamsdonk C. D., Griewank K. G., Crosby M. B., Garrido M. C., Vemula S., Wiesner T., et al. (2010). Mutations in GNA11 in uveal melanoma. N. Engl. J. Med. 363, 2191–2199. doi:10.1056/NEJMoa1000584
Videira I. F., Moura D. F. L., Magina S. (2013). Mechanisms regulating melanogenesis. Bras Dermatol 88, 76–83. doi:10.1590/s0365-05962013000100009
Vitic Z., Safory H., Jovanovic V. M., Sarusi Y., Stavsky A., Kahn J., et al. (2021). BMP5/7 protect dopaminergic neurons in an α-synuclein mouse model of Parkinson's disease. Brain 144, e15. doi:10.1093/brain/awaa368
Wakamatsu K., Zippin J. H., Ito S. (2021). Chemical and biochemical control of skin pigmentation with special emphasis on mixed melanogenesis. Pigment. Cell Melanoma Res. 34, 730–747. doi:10.1111/pcmr.12970
Walker G. J., Hayward N. K. (2002). Pathways to melanoma development: lessons from the mouse. J. Invest. Dermatol 119, 783–792. doi:10.1046/j.1523-1747.2002.00217.x
Wang R. F., Ko D., Friedman B. J., Lim H. W., Mohammad T. F. (2023). Disorders of hyperpigmentation. Part I. Pathogenesis and clinical features of common pigmentary disorders. J. Am. Acad. Dermatol 88, 271–288. doi:10.1016/j.jaad.2022.01.051
Wang Y., Zhou X., Zhao B., Ren X., Chen Y., Si J., et al. (2018). Early embryonic exposure of ionizing radiations disrupts zebrafish pigmentation. J. Cell Physiol. 234, 940–949. doi:10.1002/jcp.26922
Wang Z.-J., Xu W., Liang J.-W., Wang C.-S., Kang Y. (2017). Effect of fucoidan on B16 murine melanoma cell melanin formation and apoptosis. Afr. J. Tradit. Complement. Altern. Med. 14, 149–155. doi:10.21010/ajtcam.v14i4.18
Wat H., Wu D. C., Chan H. H. L. (2017). Fractional resurfacing in the Asian patient: current state of the art. Lasers Surg. Med. 49, 45–59. doi:10.1002/lsm.22579
Wei X., Shi J., Lin Q., Ma X., Pang Y., Mao H., et al. (2021). Targeting ACLY attenuates tumor growth and acquired cisplatin resistance in ovarian cancer by inhibiting the PI3K-AKT pathway and activating the AMPK-ROS pathway. Front. Oncol. 11, 642229. doi:10.3389/fonc.2021.642229
Wu P.-Y., You Y.-J., Liu Y.-J., Hou C.-W., Wu C.-S., Wen K.-C., et al. (2018). Sesamol inhibited melanogenesis by regulating melanin-related signal transduction in B16F10 cells. Int. J. Mol. Sci. 19, 1108. doi:10.3390/ijms19041108
Wu S.-Y. S., Wang H.-M. D., Wen Y.-S., Liu W., Li P.-H., Chiu C.-C., et al. (2015). 4-(Phenylsulfanyl)butan-2-One suppresses melanin synthesis and melanosome maturation in vitro and in vivo. Int. J. Mol. Sci. 16, 20240–20257. doi:10.3390/ijms160920240
Yalcin H. C., Amindari A., Butcher J. T., Althani A., Yacoub M. (2017). Heart function and hemodynamic analysis for zebrafish embryos. Dev. Dyn. 246, 868–880. doi:10.1002/dvdy.24497
Yan J., Tingey C., Lyde R., Gorham T. C., Choo D. K., Muthumani A., et al. (2014). Novel and enhanced anti-melanoma DNA vaccine targeting the tyrosinase protein inhibits myeloid-derived suppressor cells and tumor growth in a syngeneic prophylactic and therapeutic murine model. Cancer Gene Ther. 21, 507–517. doi:10.1038/cgt.2014.56
Yang H.-L., Lin C.-P., Vudhya Gowrisankar Y., Huang P.-J., Chang W.-L., Shrestha S., et al. (2021). The anti-melanogenic effects of ellagic acid through induction of autophagy in melanocytes and suppression of UVA-activated α-MSH pathways via Nrf2 activation in keratinocytes. Biochem. Pharmacol. 185, 114454. doi:10.1016/j.bcp.2021.114454
Yardman-Frank J. M., Fisher D. E. (2021). Skin pigmentation and its control: from ultraviolet radiation to stem cells. Exp. Dermatol 30, 560–571. doi:10.1111/exd.14260
Ye Y., Chou G.-X., Wang H., Chu J.-H., Yu Z.-L. (2010). Flavonoids, apigenin and icariin exert potent melanogenic activities in murine B16 melanoma cells. Phytomedicine 18, 32–35. doi:10.1016/j.phymed.2010.06.004
Ye Y., Wang H., Chu J.-H., Chou G.-X., Yu Z.-L. (2011). Activation of p38 MAPK pathway contributes to the melanogenic property of apigenin in B16 cells. Exp. Dermatol 20, 755–757. doi:10.1111/j.1600-0625.2011.01297.x
Yin L., Niu C., Liao L.-X., Dou J., Habasi M., Aisa H. A. (2017). An isoxazole chalcone derivative enhances melanogenesis in B16 melanoma cells via the akt/gsk3β/β-catenin signaling pathways. Molecules 22, 2077. doi:10.3390/molecules22122077
Yu F.-X., Luo J., Mo J.-S., Liu G., Kim Y. C., Meng Z., et al. (2014). Mutant Gq/11 promote uveal melanoma tumorigenesis by activating YAP. Cancer Cell 25, 822–830. doi:10.1016/j.ccr.2014.04.017
Yue Y., Zhong M., An X., Feng Q., Lai Y., Yu M., et al. (2022). Serotonin (5-HT) 2A receptor involvement in melanin synthesis and transfer via activating the PKA/CREB signaling pathway. Int. J. Mol. Sci. 23, 6111. doi:10.3390/ijms23116111
Zhang J., Chambers I., Yun S., Phillips J., Krause M., Hamza I. (2018). Hrg1 promotes heme-iron recycling during hemolysis in the zebrafish kidney. PLoS Genet. 14, e1007665. doi:10.1371/journal.pgen.1007665
Zhang Z.-D., Yang Y.-J., Liu X.-W., Qin Z., Li S.-H., Li J.-Y. (2021). Aspirin eugenol ester ameliorates paraquat-induced oxidative damage through ROS/p38-MAPK-mediated mitochondrial apoptosis pathway. Toxicology 453, 152721. doi:10.1016/j.tox.2021.152721
Zhou L., Cai M., Ren Y., Wu H., Liu M., Chen H., et al. (2018). The different roles of 5-HT1A/2A receptors in fluoxetine ameliorated pigmentation of C57BL/6 mouse skin in response to stress. J. Dermatol Sci. 92, 222–229. doi:10.1016/j.jdermsci.2018.10.002
Zhou M., Zhang Z., Wu J., Lin F., Fu L., Wang S., et al. (2013). Dermal mesenchymal stem cells (DMSCs) inhibit skin-homing CD8+ T cell activity, a determining factor of vitiligo patients’ autologous melanocytes transplantation efficiency. PLoS One 8, e60254. doi:10.1371/journal.pone.0060254
Zhou S., Zeng H., Huang J., Lei L., Tong X., Li S., et al. (2021). Epigenetic regulation of melanogenesis. Ageing Res. Rev. 69, 101349. doi:10.1016/j.arr.2021.101349
Zhu P.-Y., Yin W.-H., Wang M.-R., Dang Y.-Y., Ye X.-Y. (2015). Andrographolide suppresses melanin synthesis through Akt/GSK3β/β-catenin signal pathway. J. Dermatol Sci. 79, 74–83. doi:10.1016/j.jdermsci.2015.03.013
Ziegler I. (2003). The pteridine pathway in zebrafish: regulation and specification during the determination of neural crest cell-fate. Pigment. Cell Res. 16, 172–182. doi:10.1034/j.1600-0749.2003.00044.x
Glossary
Keywords: zebrafish, melanin, pigmentation disorders, skin, models
Citation: Qu J, Yan M, Fang Y, Zhao J, Xu T, Liu F, Zhang K, He L, Jin L and Sun D (2023) Zebrafish in dermatology: a comprehensive review of their role in investigating abnormal skin pigmentation mechanisms. Front. Physiol. 14:1296046. doi: 10.3389/fphys.2023.1296046
Received: 19 September 2023; Accepted: 13 November 2023;
Published: 23 November 2023.
Edited by:
Vikash Kumar, Central Inland Fisheries Research Institute (ICAR), IndiaReviewed by:
Dhananjay Soren, Ravenshaw University, IndiaSoumya Prasad Panda, Central Inland Fisheries Research Institute (ICAR), India
Copyright © 2023 Qu, Yan, Fang, Zhao, Xu, Liu, Zhang, He, Jin and Sun. This is an open-access article distributed under the terms of the Creative Commons Attribution License (CC BY). The use, distribution or reproduction in other forums is permitted, provided the original author(s) and the copyright owner(s) are credited and that the original publication in this journal is cited, in accordance with accepted academic practice. No use, distribution or reproduction is permitted which does not comply with these terms.
*Correspondence: Luqing He, aGVscTYxOUAxNjMuY29t; Libo Jin, MjAxNjAxMjFAd3p1LmVkdS5jbg==; Da Sun, c3VuZGF5QHd6dS5lZHUuY24=
†These authors have contributed equally to this work