- 1Department of Medicine, Faculty of Medicine and Health Sciences, Université de Sherbrooke, Sherbrooke, Canada
- 2Centre D’imagerie Moléculaire de Sherbrooke, Sherbrooke, Canada
- 3Centre de Recherche Du CHUS, Sherbrooke, Canada
- 4Nestlé Health Science, Lausanne, Switzerland
- 5Centre de Recherche sur le Vieillissement, Sherbrooke, Canada
Ketones are alternative energy substrates for the heart and kidney but no studies have investigated their metabolism simultaneously in both organs in humans. The present double tracer positron emission tomography (PET) study evaluated the organ distribution and basal kinetic rates of the radiolabeled ketone, 11C-acetoacetate (11C-AcAc), in the heart and kidney compared to 11C-acetate (11C-Ac), which is a well-validated metabolic radiotracer. Both tracers were highly metabolized by the left ventricle and the renal cortex. In the heart, kinetic rates were similar for both tracers. But in the renal cortex, uptake of 11C-Ac was higher compared to 11C-AcAc, while the reverse was observed for the clearance. Interestingly, infusion of 11C-AcAc led to a significantly delayed release of radioactivity in the renal medulla and pelvis, a phenomenon not observed with 11C-Ac. This suggests an equilibrium of 11C-AcAc with the other ketone, 11C-D-beta-hydroxybutyrate, and a different clearance profile. Overall, this suggests that in the kidney, the absorption and metabolism of 11C-AcAc is different compared to 11C-Ac. This dual tracer PET protocol provides the opportunity to explore the relative importance of ketone metabolism in cardiac and renal diseases, and to improve our mechanistic understanding of new metabolic interventions targeting these two organs.
1 Introduction
Positron emission tomography (PET) is a sensitive technique to study physiology, metabolism, and molecular pathways in living humans using labeled endogenous and natural exogenous compounds (Tersalvi et al., 2023; Toyama et al., 2021; Amoabeng et al., 2022). The ketone radiotracer 11C-acetoacetate (11C-AcAc) was developed to assess brain ketone metabolism in conditions such as mild cognitive impairment and Alzheimer’s disease (Croteau et al., 2018), which are characterized by regional brain glucose hypometabolism but with preserved brain ketone metabolism (Cunnane et al., 2020). Once injected, AcAc equilibrates rapidly with D-BHB (Figure 1) and is transformed into AcAc-CoA and acetyl-CoA in the mitochondria of key organs such as the brain (Matsuura et al., 2023). Acetyl-CoA enters the Krebs’s (Tricarboxylic Acid [TCA]) cycle as a key substrate leading to the production of energy and ATP via the oxidation-phosphorylation pathway. PET with 11C-AcAc also allows increased brain metabolism of ketones from an exogenous ketone supplement to be measured (Fortier et al., 2019). Recently, a pilot PET study with 11C-AcAc confirmed that the heart and kidney actively consume exogenous D-beta-hydroxybutyrate (D-BHB), with uptake significantly greater than that of the brain (Cuenoud et al., 2020).
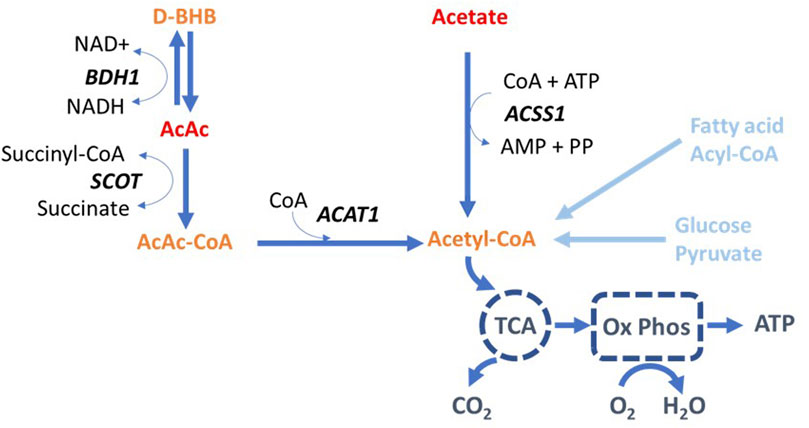
FIGURE 1. Metabolism of acetoacetate and acetate leading to the production of mitochondrial acetyl-CoA. Fatty acids and glucose are the main precursors of acetyl-CoA via acyl-CoA and pyruvate, respectively. AcAc: acetoacetate; ACAT: acetyl-CoA acetyltransferase; ACSS: acyl-CoA short-chain synthetase; AMP: Adenosine monophosphate; ATP: Adenosine triphosphate; BDH: 3-hydroxybutyrate dehydrogenase; CoA: Coenzyme A; D-BHB: D-beta-hydroxybutyrate; NAD: Nicotinamide adenine dinucleotide; PP: pyrophosphate; Ox Phos: oxidative phosphorylation; SCOT: succinyl-CoA:3-ketoacid CoA transferase; TCA: tricarboxylic acid cycle (or Krebs’s cycle).
The PET tracer, 11C-acetate (11C-Ac), has been used extensively to measure oxidative metabolism and substrate perfusion, both in the heart and the kidney (Klein et al., 2001; Normand et al., 2019). In the heart, 11C-Ac is rapidly taken up by the left ventricle reflecting the TCA cycle flux well (Figure 1). It provides a good estimate of both myocardial oxygen consumption (MVO2) using the tracer clearance rate, and myocardial blood flow (MBF) determined from the tracer uptake rate (Klein et al., 2001). Both values are important to understand various pathophysiological mechanisms and are directly related to cardiac function. In the kidney, rapid uptake of 11C-Ac is observed exclusively in the cortex, with minimal medullary uptake or pelvic urinary excretion, and with a clearance rate similar to its myocardial clearance relative to the oxygen consumption index (Juillard et al., 2007; Normand et al., 2019). In the kidney with impaired function, both 11C-Ac uptake and clearance are reportedly decreased (Shreve et al., 1995).
In the present study, we performed a head-to-head comparison of 11C-AcAc and 11C-Ac using dynamic imaging on a high-resolution PET scanner with a large-field of view in which the human heart and kidney could be viewed simultaneously. We determined organ distribution and basal kinetic rates of absorption and metabolism for both tracers.
2 Materials and methods
2.1 Study approval
The study protocol was approved by the Human Ethics Committee of the CIUSSS-Estrie-CHUS. All participants provided written informed consent in accordance with the Declaration of Helsinki. This study was registered at ClinicalTrials.gov (NCT05238805) under the name Double Pet Project: A comparison between 11C-acetate and 11C-acetoacetate heart and kidney uptake.
2.2 Experimental design
2.2.1 Participant preparation and imaging
Ten healthy participants (26.3 ± 3.7 years old, BMI 24.1 ± 4.3 kg/m2, see Supplementary material for full characterization) were asked to come for a single session during which the 11C-AcAc and 11C-Ac PET scans were performed consecutively within 2 h at to ensure that all participants were imaged under similar conditions, they were asked to refrain from taking alcohol or performing intense physical activity 24 h beforehand. To standardize the cohort and evaluate the two radiotracers at baseline without elevated blood ketones, they were asked to be fasted for 4 h and to drink 500 mL of water 1 h before imaging. A blood sample for clinical chemistry was taken immediately before starting the first scan. Each cardiac-gated, list-mode scan started at the time of intravenous injection of the radiotracer (319 ± 53 MBq) and lasted 30 min. There was a 30-min wash-out period between the two tracers, which were administered in random order. Both radiotracers were labelled at carbon position one, with >98% of purity, and specific activity >18.4 GBq/µmol for 11C-Ac and >158.4 GBq/µmol for 11C-AcAc. Blood pressure and heart rate were monitored at baseline and throughout each scan.
2.2.2 Imaging protocol and image reconstruction
All PET scans were performed on a Biograph Vision 600 (Siemens, Erlangen, Germany) scanner with a 26 cm axial field of view, a matrix of 220 × 220 voxels and a voxel size of 1.65 mm3. A 30-min list-mode PET acquisition was performed along with electrocardiogram cardiac gating. Two image reconstructions (dynamic and gated) were performed with an iterative algorithm using point spread function and time of flight modeling. For the dynamic reconstruction, time frames were reconstructed as follows: 12 × 10 s, 6 × 30 s, 6 × 150 s, 2 × 300 s. For the cardiac-gated reconstruction, the sum of the 5–30 min post-injection images was reconstructed into 16 gates.
2.2.3 Image analysis
The pharmacokinetic and left ventricle heart function analyses were performed with PMOD software (PMOD Technologies LLC., Zurich, Switzerland). Right ventricle heart function analyses were performed using Cedars-Sinai Medical Center Cardiac suite software (QPET; Cedars-Sinai, CA, United States). Only the first 15 min of each dynamic scan were used for pharmacokinetic modeling due to the rapid decay of the 11C signal. For analysis of heart function with the gated images, the last 25 min of the scan were used to avoid contamination of the images by the tracer in blood.
For cardiac pharmacokinetic analysis, the PMOD cardiac module was used which provides a semi-automatic segmentation of the myocardial regions and ventricles. The same module was used for pharmacokinetic modeling with a heart-specific, one-tissue, two-compartment model with built-in corrections for partial-volume and blood metabolites. The arterial input function was derived from the left ventricle region. For 11C-Ac, plasma metabolite correction was performed using values from the literature and built into the PMOD cardiac module (van den Hoff et al., 2001). For 11C-AcAc, plasma 11C-CO2 concentration was measured in a separate cohort of healthy participants and a linear model correction was applied with respect to the timeline acquisition ([11C-CO2] = 1.323*t) (Supplementary Figure S1). The pharmacokinetic parameters provided K1 (mL/g/min), representing the radiotracer uptake by the tissue (perfusion), and k2 (min-1) representing tracer rapid clearance or metabolism into 11CO2. MBF and MVO2 were calculated based on the 11C-Ac pharmacokinetic parameters (van den Hoff et al., 2001). The relationship between K1 of 11C-AcAc and the MBF derived from 11C-Ac was investigated using a generalized Renkin-Crone model (van den Hoff et al., 2001). To account for fluctuations of the heart rate and blood pressure between scans, the K1 was scaled (K1s) using the rate-pressure product (RPP) with the following formula in which HR is the heart rate, and SBP is the systolic blood pressure:
Gated cardiac imaging was analyzed from automatic segmentation to obtain the left and right ventricle ejection fraction, end-diastolic volume, and end-systolic volume (Labbé et al., 2012). For the kidney pharmacokinetic analysis, the cortex was segmented manually on a summed image of the radiotracer washout period (2–30 min). Great care was taken to avoid large blood vessels or any contamination of the signal from urine and renal pelvis. We did not attempt to segment the renal medulla because of the high 11C signal in the pelvis region following administration of 11C-AcAc which was not observed with 11C-Ac. A one-tissue, two-compartment model was used for both radiotracers. Additionally, an irreversible two-tissue, three-compartment model was assessed for 11C-AcAc. The two-tissue model had a third parameter, k3 representing the local accumulation of 11C-AcAc or its metabolites. The one and two tissue models were compared visually and with the Akaike information criterion and were found to be equivalent. The two-tissue model for 11C-Ac was not assessed because previous studies by our group showed that the one-tissue model was superior to assess perfusion and oxygen consumption (Bjornstad et al., 2023). The blood input function provided to the model was the same as for the cardiac analysis (left ventricle) with identical correction for the presence of metabolites. Quantification of blood flow or oxygen consumption by the kidney using 11C-Ac is not yet fully validated, so only K1 and k2 were calculated for both radiotracers (Normand et al., 2019).
2.2.4 Statistical analysis
Statistical analyses were performed using PRISM 9.5.1 (GraphPad Software Inc., CA, United States). All data are expressed as the mean (standard deviation) or median [interquartile range] based on visual inspection of histograms for distribution. Pharmacokinetic data were normally distributed, so statistical differences were investigated using either a paired Student’s t-test or a repeated measures one-way ANOVA with Tukey correction for multiple comparisons. Pearson correlation coefficients were also calculated. Heart function parameters following a normal distribution were compared using a paired Student’s t-tests, other parameters were compared using a Wilcoxon signed-rank test. A p-value ≤0.05 was considered significant.
3 Results
Figure 2 shows typical images obtained with the two tracers with the heart and kidneys scanned simultaneously. Dynamic time frames after tracer administration superimposed on a low-dose computed tomography (Figure 2A) illustrate the time dependent uptake and clearance of the tracers in the left ventricle and kidney cortex. The heart 11C-AcAc and 11C-Ac images (Figure 2B) were very similar in appearance with most of the intensity of both tracers located in the left ventricle. The time-activity curves for the two radiotracers were very similar for the left ventricle (Figure 3A), with a very fast uptake, and a similar maximum standardized uptake value (SUVmax; Table 1). However, for the kidney, the images for the two tracers differed significantly: 11C-Ac was distributed mainly in the cortex, while 11C-AcAc was also detected in the medulla, renal pelvis and ureter, where it had a hyperintense signal (Figure 2C). In the renal cortex, 11C-AcAc was taken up faster than 11C-Ac but reached a lower SUVmax (Figure 3A). In the renal pelvis, a small initial signal appeared rapidly with both tracers (Figure 3A). A later and larger signal with a Tmax of 7.1 min appeared only with 11C-AcAc (Table 1).
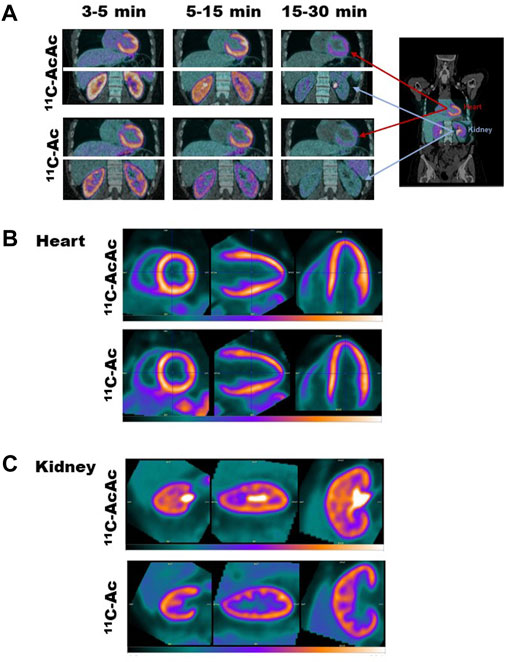
FIGURE 2. Typical PET images. (A) Dynamic time frames after tracer administration for the heart and kidney within the 26-cm axial field of view of the PET scanner superimposed on a low-dose computerized tomography. Summed images for the heart (5–30 min) (B) and kidney (5–15 min) (C) in the short, vertical, and horizontal long axis for the two radiotracers, 11C-AcAc (acetoacetate) and 11C-Ac (acetate).
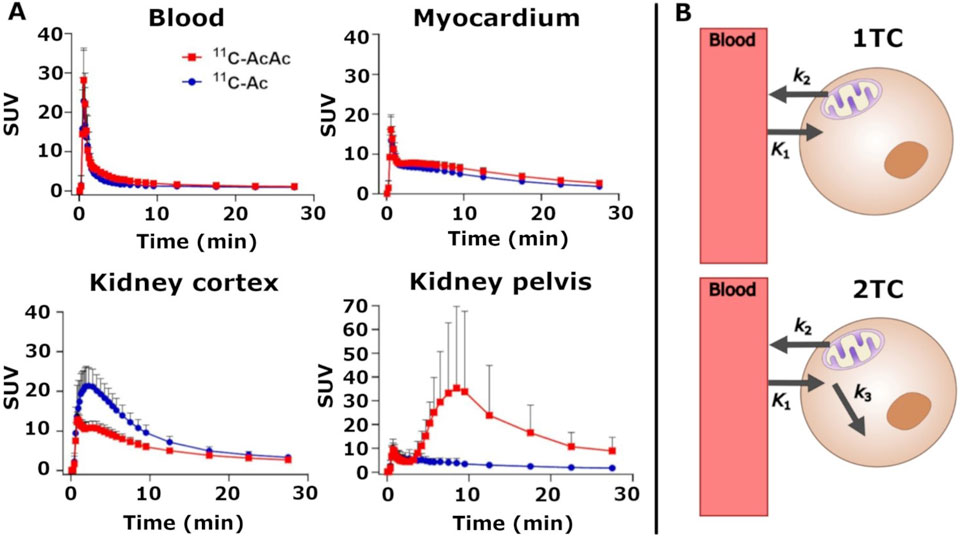
FIGURE 3. (A) 11C-AcAc (acetoacetate) and 11C-Ac (acetate) time activity curves. Each point is the average ± standard deviation (n = 10). (B) Compartmental models were used to derive the pharmacokinetic parameters. 1TC: one-tissue compartment model; 2 TC: two-tissue compartment model; SUV—standardized uptake value.
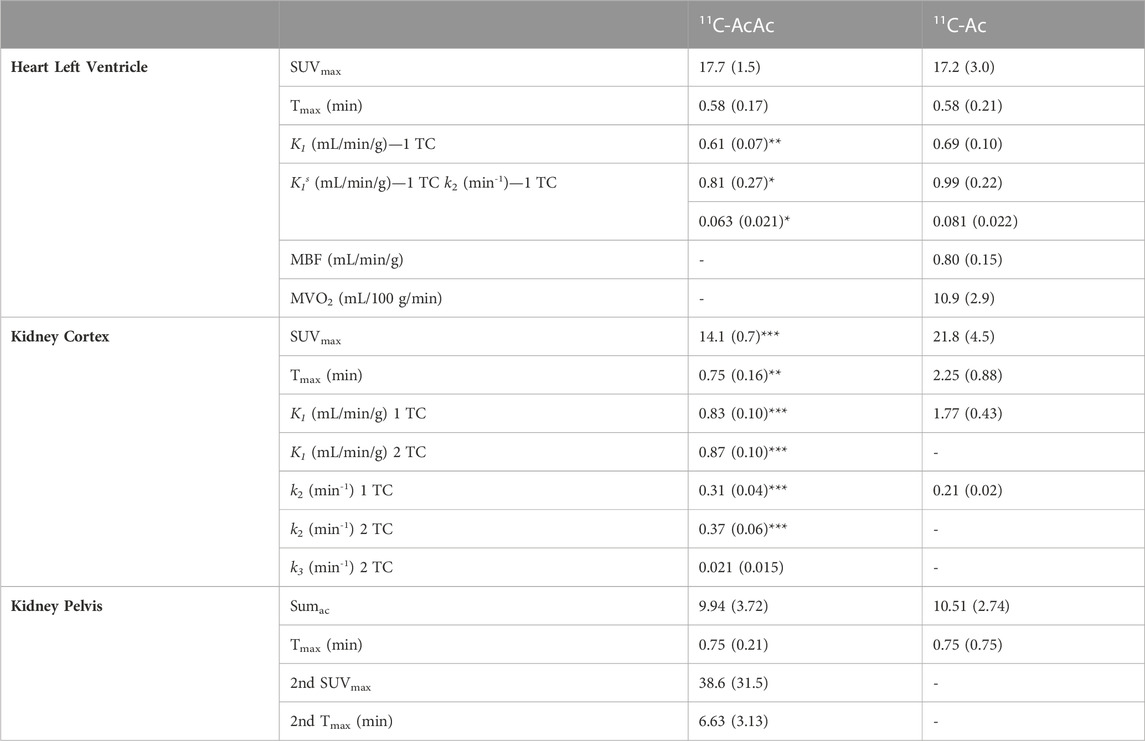
TABLE 1. Kinetic analysis of 11C-AcAc (acetoacetate) and 11C-Ac (acetate) in the heart and kidney. Values are presented as mean (standard deviation). 11C-AcAc: 11C-acetoacetate; 11C-Ac: 11C-acetate; SUVmax: maximum standardized uptake value; Tmax: time to reach SUVmax; K1: kinetic rate for tracer transport into the organ; K1s: kinetic rate for tracer transport into the organ normalized with the rate pressure product; k2: rate constant for tracer clearance from the organ; MBF: myocardial blood flow; MVO2: myocardial oxygen consumption, calculated by the product 1.35 x k2 -9.6 × 10−3; 1 TC: one-tissue compartment model; 2 TC: two-tissue compartment model. *p ≤ 0.05; **p ≤ 0.01; ***p ≤ 0.001 compared to 11C-Ac.
3.1 Pharmacokinetic analysis of the heart
Table 1 shows the cardiac pharmacokinetic parameters derived for 11C-AcAc and 11C-Ac as well as MBF and MVO2 for 11C-Ac. The values for K1 and scaled K1 using RPP (K1s) were similar for both tracers. Figure 4A shows individual K1 values for the left ventricular myocardium (LV) of each participant. The myocardial K1 values for the two radiotracers were significantly positively correlated (Figure 4B) prompting us to investigate the possible relationship between MBF derived from 11C-Ac and the K1 of 11C-AcAc. Although we found a statistically significant positive correlation between these two parameters (Figure 4C), the narrow range of blood flow values did not allow us to fit a Renkin-Crone model. Scaling the K1 to the RPP increased the correlation between the two tracers (r2 = 0.46 without scaling vs. r2 = 0.71 with scaling; Figure 4B), most likely because it accounted for changes in cardiac workload between scans. The cardiac clearance k2 was higher for 11C-Ac compared to 11C-AcAc (22%, p = 0.021, Figure 5A and Table 1). The k2s for 11C-AcAc and 11C-Ac were not significantly correlated (Figure 5B). Heart function parameters and vital signs for both radiotracers are shown in Supplementary Table S2. Vital signs were within normal range and similar during both scans.
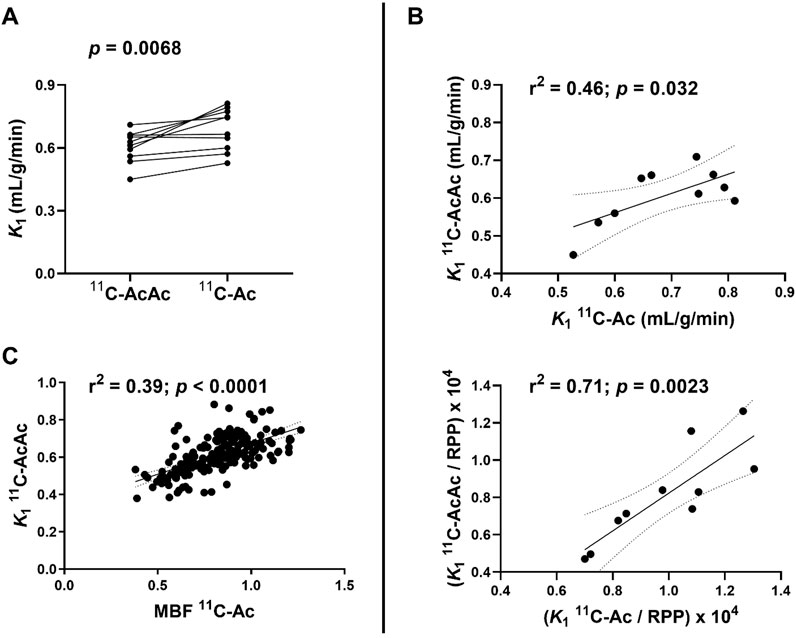
FIGURE 4. Uptake rate (K1) of 11C-AcAc (acetoacetate) and 11C-Ac (acetate) in the myocardium (n = 10). (A) Individual K1 values. Points are paired for both tracers for each participant. (B) Correlation of 11C-AcAc and 11C-Ac K1 without (upper panel) and with (lower panel) scaling with the individual rate-pressure product (RPP). (C) Correlation between 11C-Ac derived myocardial blood flow (MBF) and 11C-AcAc K1 for the 17 myocardial segments in all 10 participants. Paired two-tailed Student’s t-test for 11C-AcAc and 11C-Ac. r2: coefficient of determination.
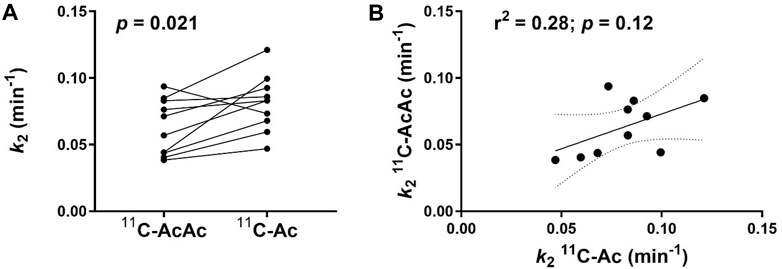
FIGURE 5. Clearance rate (k2) of 11C-AcAc (acetoacetate) and 11C-Ac (acetate) in the myocardium (n = 10). (A) Paired data for individual k2 of 11C-AcAc and 11C-Ac in each participant. (B) Correlation of 11C-AcAc and 11C-Ac k2 in each participant. Paired two-tailed Student’s t-test for 11C-AcAc and 11C-Ac. r2: coefficient of determination.
3.2 Pharmacokinetic analysis of the renal cortex
Table 1 shows the pharmacokinetic parameters obtained for the renal cortex. There was no significant difference between the Akaike information criterion for the one-tissue and irreversible two-tissue model of 11C-AcAc. Statistical analyses produced similar results for both models;, accordingly, we present here only results comparing the one-tissue model for 11C-AcAc with the one-tissue model for 11C-Ac.
Renal cortical uptake of 11C-Ac (K1) was 53% higher than for 11C-AcAc (p < 0.0001) (Figure 6A). In contrast to the myocardium, K1 for 11C-AcAc and 11C-Ac were not correlated in the renal cortex (Figure 6B). Renal blood flow was not calculated for 11C-Ac because it is not yet a fully validated method.
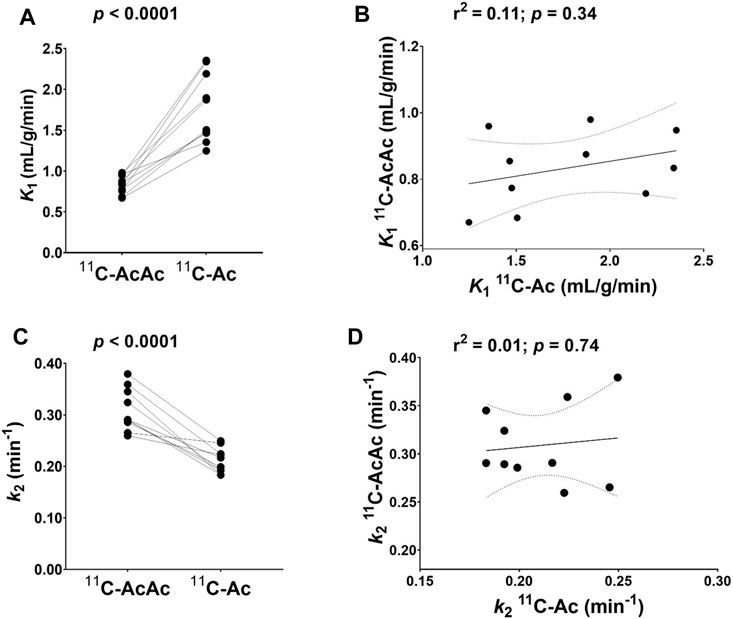
FIGURE 6. Uptake rate (K1) and clearance rate (k2) of 11C-AcAc (acetoacetate) and 11C-Ac (acetate) in the renal cortex (n = 10) using a one-tissue model. (A) Individual K1 values for 11C-AcAc and 11C-Ac. Points are paired across both tracers for each participant. (B) Correlation of 11C-AcAc and 11C-Ac K1 values with one point for each participant. (C) Individual k2 values for 11C-AcAc and 11C-Ac. Points are paired across both tracers for each participant. (D) Correlation of 11C-AcAc and 11C-Ac k2 values with one point for each participant. Paired two-tailed Student’s t-test for 11C-AcAc and 11C-Ac. r2: coefficient of determination.
Unlike in the heart, the renal cortex metabolized 11C-AcAc at a significantly higher rate than 11C-Ac (46%; p < 0.0001) (Figure 7C). Similar to K1, renal k2 of 11C-AcAc and 11C-Ac were not significantly correlated (Figure 7D).
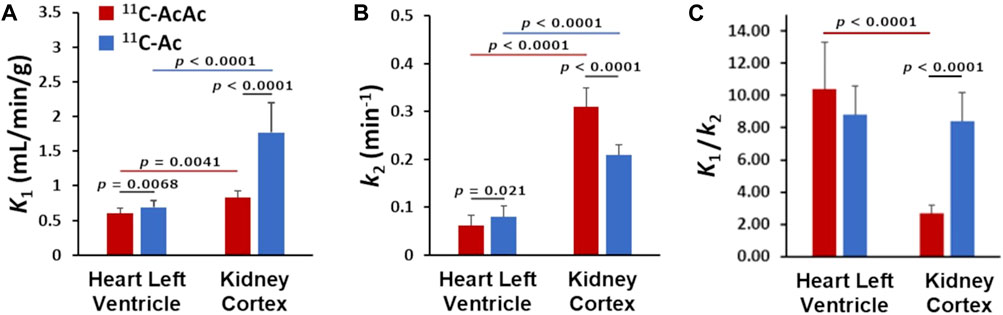
FIGURE 7. Comparison of 11C-AcAc (acetoacetate) and 11C-Ac (acetate) kinetic rates in the heart and kidney cortex (n = 10): (A) Uptake rate (K1), (B) Clearance rate (k2), (C) Kinetic ratio K1/k2.
3.3 Comparison between the kinetic rates of the tracers in the heart versus renal cortex
For both 11C-AcAc and 11C-Ac, K1 was higher in the renal cortex than in the myocardium (Table 1; Figures 7A, B). Versus the myocardium, K1 for 11C-AcAc was 1.4 ± 0.3-fold higher in the renal cortex (p = 0.0009) while for 11C-Ac, K1 was 2.6 ± 0.8-fold higher in the renal cortex (p < 0.0001). Similarly, k2 for 11C-AcAc was 5.3 ± 1.6-fold higher (p < 0.0001), and for 11C-Ac was 2.8 ± 1.0-fold higher (p < 0.0001) in renal cortex vs. myocardium.
The K1/k2 ratio provides further information about the relationship between tracer uptake and clearance (Figure 7C). This ratio was similar in both organs for 11C-Ac, but significantly lower for 11C-AcAc in the renal cortex, suggesting the utilization of different kinetic pathways in the kidney.
4 Discussion
This study compared the metabolism of the two radiotracers11C-AcAc and 11C-Ac in the heart and kidney. Similar regional uptake of these tracers was observed with preferential uptake in the left ventricle and in the renal cortex. Overall, the uptake/clearance kinetic ratio (K1/k2) of 11C-Ac was similar in the heart and renal cortex, and for 11C-AcAc in the heart. However, the kinetic ratio was significantly lower for 11C-AcAc the renal cortex (Figure 7C). This suggests that ketones might have similar kinetics as 11C-Ac in the heart, but different uptake and clearance in the kidney. Strikingly, 11C-AcAc led to a slowly appearing hyperintense signal in the renal pelvis which was not detected with 11C-Ac, suggesting additional metabolic pathways possibly involving hepatic 11C-AcAc conversion to the other ketone 11C-D-BHB. Heart function parameters calculated for each radiotracer provided similar values.
4.1 11C-AcAc cardiac PET reveals similar kinetic and functional values compared to 11C-Ac
Cardiac PET with 11C-Ac is a well-established and validated method to assess MBF and MVO2, and our results agree with those already published (Shreve et al., 1995; Normand et al., 2019; Hansen et al., 2022). We compared 11C-Ac kinetics to those of 11C-AcAc as a first step towards understanding whether the uptake (K1) or clearance (k2) parameters of 11C-AcAc were related to blood flow and oxidative metabolism. This information is crucial to correctly interpret dynamic 11C-AcAc PET scans assessing cardiac ketone metabolism. Both radiotracers showed relatively high uptake in the myocardium and provide good quality images, with semi-automatic segmentation facilitating the kinetic analysis. Using one compartmental pharmacokinetic modeling, kinetic parameters of 11C-AcAc were similar but statistically lower than for 11C-Ac. K1swere significantly positively correlated between the two radiotracers; this positive correlation was more evident using a scaled K1 (K1s; derived via RPP) which took into account the differences in heart rate and blood pressure between participants.
Overall, these results show that, like for 11C-Ac, MBF is a determinant of 11C-AcAc uptake in the myocardium and that oxidative metabolism is a determinant of its rapid clearance. Comparison between 11C-AcAc and 11C-Ac in the healthy rat heart also indicates similar kinetics, with those derived from 11C-AcAc being 10%–30% lower than with 11C-Ac (Croteau et al., 2014), an effect similar what we observed here. The lower myocardial clearance rate of 11C-AcAc versus 11C-Ac may be attributable to the additional metabolic step required to convert AcAc to acetyl-CoA, and to the equilibrium between AcAc and D-BHB (Figure 1). For heart studies, it is reasonable to assume that the K1 and k2 parameters of the one-tissue, two-compartment 11C-AcAc model are good indices of tissue perfusion and the rate of ketone metabolism, respectively. However, the exact relationship between K1 of 11C-AcAc and MBF requires further investigation in conditions under which MBF is increased (e.g., stress test) or when the ketone transport rate could be different (e.g., increased ketosis, disease states).
4.2 Renal 11C-AcAc kinetics are different when compared to 11C-Ac
In the kidney, we were able to semi-automatically segment the cortex. Plots of calculated SUV as time activity curves allowed for the kinetic rates of perfusion (K1) and metabolism (k2) of both tracers to be assessed in the renal cortex using a one-tissue two-compartment model and a two-tissue three-compartment model for 11C-AcAc. Similar K1 and k2 values were obtained using either one or two-tissue compartment modeling with 11C-AcAc. The relatively small k3 value derived from the two-compartment value suggests a low accumulation of 11C-AcAc or its metabolite 11C-D-BHB in the renal cortex. Therefore, we used the simpler one-tissue model for further comparisons between 11C-Ac and 11C-AcAc tissue kinetics. However, by neglecting metabolite recirculation and urinary excretion, this model could induce a bias in the calculated ketone consumption rate. Validation of the renal excretion pathway and the metabolic fate of acetoacetate would lead to a more appropriate interpretation of the kinetic analysis results. Moreover, the one-tissue compartment model does not take into account the possibility that circulating 11C- CO2 could be taken by the tissue. Considering that 11C-Ac produces more 11C-CO2 over the scan period than 11C-AcAc, this could explain some of the differences between the two radiotracers. However, most of the uptake and metabolism occur early after radiotracer injection, when blood 11C-CO2 is low. Also, previous studies (Ng et al., 1994) have described the fate of the 11C metabolites in 6 compartments to validate 11C-Ac perfusion and oxygen consumption measures.
The use of 11C-Ac to assess energy metabolism in the kidney is less well known than for the heart. Direct comparison with 15O-H2O has shown that uptake of 11C-Ac is related to kidney perfusion (Normand et al., 2019). Impaired renal clearance of 11C-Ac is a good marker of diabetic nephropathy as well as renal stenosis and is positively correlated to renal oxygen consumption over a wide range of values (Shreve et al., 1995; Juillard et al., 2007). Both uptake and clearance of 11C-Ac are higher in the kidney than in the myocardium although the ratio of uptake to clearance (K1/k2) remained the same (Figure 7-C).
The kinetics of 11C-AcAc in the kidney were quite different to that of 11C-Ac. Firstly, 11C-AcAc K1 was significantly lower than 11C-Ac K1 and only marginally higher than in the myocardium (Table 1; Figures 7–A). This was despite a 3-fold higher blood flow in the renal cortex compared to the myocardium (Normand et al., 2019), which suggests that renal 11C-AcAc K1 underestimates renal perfusion to a greater extent than myocardial perfusion. Blood filtrate transit, excretion, and reabsorption in the tubule add a layer of complexity to kidney metabolism that is not present in the myocardium. This could impact measures of renal uptake and metabolism for the two radiotracers and may be sensitive to changes in transport between the red blood cells and plasma, further investigation is needed. Secondly, the clearance k2 of 11C-AcAc was significantly higher in the renal cortex compared to the myocardium or to the 11C-Ac k2 value. This was striking given the relatively lower 11C-AcAc K1 in the renal cortex and suggests longer retention of the 11C-Ac in the renal cortex. Overall, these results are consistent with the hypothesis that uptake of 11C-AcAc depends more on active transport into kidney cells, with significant irreversible trapping and/or metabolism of 11C-AcAc, whereas the kinetic of 11C-Ac in the renal cortex, like in the heart, is more dependent on blood flow and better reflects oxygen consumption.
4.3 11C-AcAc hyperintensity in the renal pelvis might be derived from 11C-D-BHB
A significant part of the signal for 11C-AcAc in the kidney was observed in the pelvis and to some degree in the medulla, which was not the case for 11C-Ac (Figure 2B). With 11C-AcAc, an initial small and rapid increase in the 11C-AcAc signal occurred in the renal pelvis was followed by a slower higher intensity signal (Figure 3A). We speculate that this larger second signal was derived from the slower systemic formation and efflux of 11C-D-BHB. First, upon intra peritoneal injection of AcAc in mice, a relatively rapid but delayed increase in plasma D-BHB occurs within the first 15–30 min via hepatic BDH-1 metabolism and other BDH-1 expressing tissues (Stagg et al., 2021), suggesting that part of an i.v. dose of 11C-AcAc would be rapidly converted to 11C-D-BHB. Second, only one rapid 11C-AcAc peak was observed in the renal cortex (Figure 3B). No other peak was detected later, either with 11C-AcAc or 11C-Ac, and the increase in plasma 11C-CO2 over time was linear over 30 min (see Supplementary Figure S1). This suggests that the renal pelvic signal could not have originated from a delayed release of 11C-AcAc, that it was specific to 11C-AcAc and that it was not a downstream metabolite of Ac-CoA. Third, while ketones and Ac are efficiently reabsorbed from the urine by several monocarboxylate transporters localized along the nephron (Becker et al., 2010), subtle differences in transport efficiency between D-BHB and AcAc have been observed and might explain some of the difference in their regional renal reabsorption (Plata et al., 2007). Fourth, in a very recent human PET tracer study (Luong et al., 2023), 11C-D-BHB was qualitatively distributed evenly across the entire kidney and was not preferentially retained in the renal cortex, with a rapid constant elimination curve without a second peak. We interpret this to imply that 11C-AcAc injected i.v. is probably converted in part to 11C-D-BHB and that BHB and AcAc have slightly different regional renal reabsorption and metabolism along the nephron.
4.4 Limitations
The current study was performed in healthy participants after a 4-h fast without ketone supplementation, and cardiac PET was performed at rest only. Further studies will be required to evaluate the effect of cardiac stress and circulating energy substrates, especially ketones, on the kinetics of 11C-AcAc in diseases such as cardiorenal syndromes and chronic kidney disease. Findings with 11C-AcAc in the kidney and the possible role of 11C-D-BHB require additional experiments to better understand their regional renal uptake and metabolism. The availability of 11C-D-BHB as a new ketone tracer should make these investigations possible.
5 Conclusion
This study demonstrates the feasibility of simultaneously measuring heart and kidney ketone metabolism with 11C-AcAc in comparison with the metabolic tracer, 11C-Ac. Compared to 11C-Ac, the most significant difference was detected in the renal cortex, where a slower uptake and faster clearance of 11C-AcAc was observed, as well as a slowly appearing hyperintense signal in the renal pelvis. Overall, this suggests that in the kidney, the absorption and metabolism of 11C-AcAc is different to that of 11C-Ac, in part due to its conversion to 11C-D-BHB. Our dual tracer PET protocol provides an opportunity to explore the relative importance of ketone metabolism in cardiac and renal diseases, and to improve our mechanistic understanding of new metabolic interventions.
Data availability statement
The original contributions presented in the study are included in the article/Supplementary Material, further inquiries can be directed to the corresponding author.
Ethics statement
The studies involving humans were approved by Human Ethics Committee of the CIUSSS-Estrie-CHUS. The studies were conducted in accordance with the local legislation and institutional requirements. The participants provided their written informed consent to participate in this study.
Author contributions
BC: Conceptualization, Formal Analysis, Funding acquisition, Supervision, Writing–original draft, Writing–review and editing. EC: Conceptualization, Data curation, Formal Analysis, Methodology, Software, Supervision, Writing–original draft, Writing–review and editing. VS-P: Conceptualization, Data curation, Formal Analysis, Investigation, Methodology, Visualization, Writing–review and editing. GR: Conceptualization, Data curation, Formal Analysis, Investigation, Methodology, Software, Visualization, Writing–original draft, Writing–review and editing. MF: Data curation, Investigation, Project administration, Supervision, Writing–review and editing. CV: Data curation, Investigation, Project administration, Supervision, Writing–review and editing. AC: Conceptualization, Funding acquisition, Methodology, Resources, Supervision, Writing–review and editing. SC: Conceptualization, Formal Analysis, Funding acquisition, Investigation, Methodology, Resources, Supervision, Writing–review and editing.
Funding
The author(s) declare financial support was received for the research, authorship, and/or publication of this article. This study was supported by Nestlé Health Science.
Conflict of interest
Author BC was employed by Nestlé Health Science.
The remaining authors declare that the research was conducted in the absence of any commercial or financial relationships that could be construed as a potential conflict of interest.
Publisher’s note
All claims expressed in this article are solely those of the authors and do not necessarily represent those of their affiliated organizations, or those of the publisher, the editors and the reviewers. Any product that may be evaluated in this article, or claim that may be made by its manufacturer, is not guaranteed or endorsed by the publisher.
Supplementary material
The Supplementary Material for this article can be found online at: https://www.frontiersin.org/articles/10.3389/fphys.2023.1280191/full#supplementary-material
References
Amoabeng K. A., Laurila S., Juárez-Orozco L. E., Marthinsen A. B. L., Moczulski D., Rebelos E., et al. (2022). The utilization of positron emission tomography in the evaluation of renal health and disease. Clin. Transl. Imaging 10, 59–69. doi:10.1007/s40336-021-00469-2
Becker H. M., Mohebbi N., Perna A., Ganapathy V., Capasso G., Wagner C. A. (2010). Localization of members of MCT monocarboxylate transporter family Slc16 in the kidney and regulation during metabolic acidosis. Am. J. Physiol. Ren. Physiol. 299 (1), F141–F154. doi:10.1152/ajprenal.00488.2009
Bjornstad P., Richard G., Choi Y. J., Nowak K. L., Steele C., Chonchol M., et al. (2023). Perturbed kidney energetics and cyst burden in autosomal dominant polycystic kidney disease.submitted. American Journal of Kidney Diseases.
Croteau E., Castellano C. A., Fortier M., Bocti C., Fulop T., Paquet N., et al. (2018). A cross-sectional comparison of brain glucose and ketone metabolism in cognitively healthy older adults, mild cognitive impairment and early Alzheimer's disease. Exp. Gerontol. 107, 18–26. doi:10.1016/j.exger.2017.07.004
Croteau E., Tremblay S., Gascon S., Dumulon-Perreault V., Labbé S. M., Rousseau J. A., et al. (2014). [(11)C]-Acetoacetate PET imaging: A potential early marker for cardiac heart failure. Nucl. Med. Biol. 41 (10), 863–870. doi:10.1016/j.nucmedbio.2014.08.006
Cuenoud B., Hartweg M., Godin J. P., Croteau E., Maltais M., Castellano C. A., et al. (2020). Metabolism of exogenous D-beta-hydroxybutyrate, an energy substrate avidly consumed by the heart and kidney. Front. Nutr. 7, 13. doi:10.3389/fnut.2020.00013
Cunnane S. C., Trushina E., Morland C., Prigione A., Casadesus G., Andrews Z. B., et al. (2020). Brain energy rescue: an emerging therapeutic concept for neurodegenerative disorders of ageing. Nat. Rev. Drug Discov. 19 (9), 609–633. doi:10.1038/s41573-020-0072-x
Fortier M., Castellano C. A., Croteau E., Langlois F., Bocti C., St-Pierre V., et al. (2019). A ketogenic drink improves brain energy and some measures of cognition in mild cognitive impairment. Alzheimers Dement. 15 (5), 625–634. doi:10.1016/j.jalz.2018.12.017
Hansen K. B., Sörensen J., Hansson N. H., Nielsen R., Larsen A. H., Frøkiær J., et al. (2022). Myocardial efficiency in patients with different aetiologies and stages of heart failure. Eur. Heart J. Cardiovasc Imaging 23 (3), 328–337. doi:10.1093/ehjci/jeab227
Juillard L., Lemoine S., Janier M. F., Barthez P. Y., Bonnefoi F., Laville M. (2007). Validation of renal oxidative metabolism measurement by positron-emission tomography. Hypertension 50 (1), 242–247. doi:10.1161/HYPERTENSIONAHA.107.089607
Klein L. J., Visser F. C., Knaapen P., Peters J. H., Teule G. J., Visser C. A., et al. (2001). Carbon-11 acetate as a tracer of myocardial oxygen consumption. Eur. J. Nucl. Med. 28 (5), 651–668. doi:10.1007/s002590000472
Labbé S. M., Grenier-Larouche T., Noll C., Phoenix S., Guérin B., Turcotte E. E., et al. (2012). Increased myocardial uptake of dietary fatty acids linked to cardiac dysfunction in glucose-intolerant humans. Diabetes 61 (11), 2701–2710. doi:10.2337/db11-1805
Luong T. V., Nielsen E. N., Falborg L., Kjærulff M. L. G., Tolbod L. P., Søndergaard E., et al. (2023). Intravenous and oral whole body ketone dosimetry, biodistribution, metabolite correction and kinetics studied by (R)-[1-11C]β-hydroxybutyrate ([11C]OHB) PET in healthy humans. EJNMMI Radiopharm. Chem. 8 (1), 12. doi:10.1186/s41181-023-00198-z
Matsuura T. R., Puchalska P., Crawford P. A., Kelly D. P. (2023). Ketones and the heart: metabolic principles and therapeutic implications. Circ. Res. 132 (7), 882–898. doi:10.1161/CIRCRESAHA.123.321872
Ng C. K., Huang S. C., Schelbert H. R., Buxton D. B. (1994). Validation of a model for [1-11C]acetate as a tracer of cardiac oxidative metabolism. Am. J. Physiol. 266 (4), H1304–H1315. doi:10.1152/ajpheart.1994.266.4.H1304Pt 2
Normand G., Lemoine S., Le Bars D., Merida I., Irace Z., Troalen T., et al. (2019). PET [11C]acetate is also a perfusion tracer for kidney evaluation purposes. Nucl. Med. Biol. 76-77, 10–14. doi:10.1016/j.nucmedbio.2019.08.004
Plata C., Sussman C. R., Sindic A., Liang J. O., Mount D. B., Josephs Z. M., et al. (2007). Zebrafish Slc5a12 encodes an electroneutral sodium monocarboxylate transporter (SMCTn). A comparison with the electrogenic SMCT (SMCTe/Slc5a8). J. Biol. Chem. 282 (16), 11996–12009. doi:10.1074/jbc.M609313200
Shreve P., Chiao P. C., Humes H. D., Schwaiger M., Gross M. D. (1995). Carbon-11-acetate PET imaging in renal disease. J. Nucl. Med. 36 (9), 1595–1601.
Stagg D. B., Gillingham J. R., Nelson A. B., Lengfeld J. E., d'Avignon D. A., Puchalska P., et al. (2021). Diminished ketone interconversion, hepatic TCA cycle flux, and glucose production in D-β-hydroxybutyrate dehydrogenase hepatocyte-deficient mice. Mol. Metab. 53, 101269. doi:10.1016/j.molmet.2021.101269
Tersalvi G., Beltrani V., Grübler M. R., Molteni A., Cristoforetti Y., Pedrazzini G., et al. (2023). Positron emission tomography in heart failure: from Pathophysiology to clinical application. J. Cardiovasc Dev. Dis. 10 (5), 220. doi:10.3390/jcdd10050220
Toyama Y., Werner R. A., Ruiz-Bedoya C. A., Ordonez A. A., Takase K., Lapa C., et al. (2021). Current and future perspectives on functional molecular imaging in nephro-urology: theranostics on the horizon. Theranostics 11 (12), 6105–6119. doi:10.7150/thno.58682
Keywords: heart, kidney, ketone, 11C-acetoacetate, 11C-acetate, cardiorenal, metabolism, beta-hydroxybutyrate
Citation: Cuenoud B, Croteau E, St-Pierre V, Richard G, Fortier M, Vandenberghe C, Carpentier AC and Cunnane SC (2023) Cardiorenal ketone metabolism: a positron emission tomography study in healthy humans. Front. Physiol. 14:1280191. doi: 10.3389/fphys.2023.1280191
Received: 19 August 2023; Accepted: 26 September 2023;
Published: 06 October 2023.
Edited by:
Takahiko Nakagawa, Shiga University of Medical Science, JapanReviewed by:
Shinji Kume, Shiga University of Medical Science, JapanVesa Juhani Oikonen, Turku PET Centre, Finland
Copyright © 2023 Cuenoud, Croteau, St-Pierre, Richard, Fortier, Vandenberghe, Carpentier and Cunnane. This is an open-access article distributed under the terms of the Creative Commons Attribution License (CC BY). The use, distribution or reproduction in other forums is permitted, provided the original author(s) and the copyright owner(s) are credited and that the original publication in this journal is cited, in accordance with accepted academic practice. No use, distribution or reproduction is permitted which does not comply with these terms.
*Correspondence: Bernard Cuenoud, YmVybmFyZC5jdWVub3VkQG5lc3RsZS5jb20=