- 1Graduate School of Sports Medicine, CHA University, Pocheon-si, Gyeonggi-do, Republic of Korea
- 2Department of Medicine, General Graduate School, CHA University, Pocheon-si, Gyeonggi-do, Republic of Korea
Purpose: Currently, there is no interventional approach to increase the intensity of Surya Namaskar a popular hatha yoga sequence used worldwide. Therefore, this study investigated how tempo-based high-intensity interval cardio yoga (HIICY) and traditional interval hatha yoga (TIHY) affects cardiometabolic fitness in active adults.
Methods: Twenty physically active male and female individuals were randomly separated into HIICY (5 males, 5 females, 1.5 s tempo) and TIHY (5 males, 5 females, 3 s tempo) groups. The intervention included twelve exercise sessions for 4 weeks in both groups. Participants conducted a ramp test to determine their maximal oxygen uptake (
Results:
Conclusion: A 4-week period of HIICY improved cardiometabolic fitness, oxidative capacity, and metabolic flexibility compared with TIHY, in physically active adults. Therefore, HIICY is suitable as HY-specific HIIT and time-efficient approach for relatively healthy individuals.
Introduction
The World Health Organization’s (WHO) definition of “physical inactivity” refers to the failure to meet recommended levels of weekly physical activity. This guideline consists of at least 150 min of moderate-intensity or 75 min of high-intensity aerobic activity per week (Santos et al., 2023). It is now well appreciated that physical inactivity is the fourth leading cause of death and has been reported to contribute to multiple chronic diseases including insulin resistance, type 2 diabetes mellitus, obesity, metabolic and endocrine diseases (Balakumar et al., 2016; Kerr and Booth, 2022). The global economic burden of non-communicable diseases associated with lack of physical activity was estimated to be between US$ 53.8 and 67.5 billion as of 2016 (Scheltens et al., 2021; Santos et al., 2023). One increased metabolic equivalent of task (1 MET =
Cardiorespiratory fitness consists of a wide range of parameters between an aerobic base and maximal aerobic exercise capacity, which represents maximal aerobic performance and the functional capacity of numerous bodily systems. These can be determined by maximal oxygen uptake (
In terms of improving
HIIT prescription is used as an alternative physical exercise for those who do not have enough time contemporary people and is also consistently ranked in the top 10 fitness trends of the American College of Sports Medicine (ACSM) (Sabag et al., 2022; Kercher et al., 2023). Previous studies have reported that HIIT is efficient at developing the three energy systems utilized in humans in a time-efficient manner, which includes active or passive recovery following repeated rounds of exercise. HIIT protocols are designed to reach an exercise intensity such as >90% of maximal heart rate (HRmax), the second ventilatory threshold (>VT2), over the second lactate threshold (>4 mmol·L-1; zone 3: high-intensity exercise), and >85% of
In terms of metabolism, metabolic flexibility is defined as the ability to rapidly convert to generate adenosine triphosphate (ATP) from efficient fat and carbohydrate utilization based on physiological demands (Goodpaster and Sparks, 2017; Galgani et al., 2022). Efficient metabolic flexibility is essential to prevent metabolic diseases directly related to physical inactivity-induced mitochondrial dysfunction (San-Millán and Brooks, 2018; Yang et al., 2022a). HIIT increases metabolic flexibility, which indicates a strong relationship with metabolic health parameters such as insulin sensitivity and mitochondrial respiratory capacity (Aparecido et al., 2022).
In this regard, yoga is a mind-body practice consisting of physical postures (Asana), breathing techniques (Pranayama), and meditation (Dhyana) (Patwardhan, 2017). Notably, yoga is one of the most practiced complementary or alternative exercise interventions to achieve optimal physical and mental health (Udhan et al., 2018; Khoshnaw and Ghadge, 2021). Furthermore, hatha yoga (HY) is becoming increasingly popular in the United States and Europe as an alternative form of physical activity that can support individuals to reach globally recommended levels of physical activity (Larson-Meyer, 2016). The main goals of HY are to enable practitioners to improve body, breath, and spirit states and to “prepare a healthy mind and body to immerse oneself in meditation for self-realization” (Schmalzl et al., 2015). A specific set of 19 asanas called Surya Namaskar B (Sun Salutations, SS) includes the vinyasa system and is one of the most basic and representative sequences in many styles of yoga classes worldwide (Papp et al., 2016; Brinsley et al., 2022).
The average metabolic cost during HY focusing on various postures, alignments, and breathing for different times is less than 3 METs and show results similar to walking on a treadmill at 3.2 km·h−1 (Hagins et al., 2007; Larson-Meyer, 2016). However, HY (Surya Namaskar) lasts at least 10 min and can contribute sufficiently intense physical activity to improve an individual’s cardiorespiratory fitness with 4-6 METs, corresponding to ACSM’s moderate-to-high-intensity physical activity guidelines (Hagins et al., 2007; Mody, 2011; Larson-Meyer, 2016; Potiaumpai et al., 2016). A recent study by Lee et al. (2021) reported that HY, which consisted of 1.5 s for each asana and lasted for 10 min, could be used as HIIT in physically active individuals. The results of this study showed %HRpeak of HRmax, %HRmean of HRmax, METs of
Therefore, the aim of the study was to investigate how at least 4-week tempo-based HIICY and traditional interval hatha yoga (TIHY) practices affected cardiometabolic fitness parameters, such as
Materials and methods
Participants
The sample size for the study was calculated using G*Power software version 3.1.9.4 (Heinlich Heine University, Düsseldorf, Germany) which considered: effect size = 0.3, alpha error probability = 0.05, and statistical power = 0.8. The effect size was determined based on previous studies (Astorino et al., 2012; Ormsbee et al., 2015; Lee et al., 2021). The total required sample size was calculated to be twenty participants assuming a 10% dropout rate (n = 20). Participants were randomly separated into two tempo-based HY groups: ten physically active individuals were assigned high-intensity interval cardio yoga (1.5 s tempo HIICY; five males and five females) and another 10 participated in traditional interval hatha yoga (3 s tempo TIHY; five males and five females) (Figure 1). All participants satisfied the minimum physical activity standards per week based on WHO guidelines and had no pre-existing cardiovascular, pulmonary, or metabolic diseases or musculoskeletal disorders (Santos et al., 2023). All participants were yoga beginners. Therefore, before the experiment began, they learned the movement sequences with the yoga instructor and quickly became familiar with the movements and sequences through the qualified yoga instructor’s demonstrations during the intervention. The participants maintained their previous physical activity levels throughout the 4-week intervention period and performed no additional training. Anthropometric measurements of all participants were taken in the fasting state. The data were as follows (mean ± standard deviation; SD): age 30 ± 5 years, height 169.6 ± 7.4 cm, body mass 66.8 ± 12.8, body fat 23.4% ± 7.5%, and BMI of 23.0 ± 3.3 kg·m−2. The anthropometric data of HIICY and TIHY groups were not significantly different (Table 1). Participants were instructed not to change their diet during the intervention and did not take any medication before and during pre- and post-tests and abstained from nicotine and alcohol for 24 h prior to the testing. This study was approved by the Institutional Review Board of CHA University (IRB No. 1044308-202206-HR-031-02). The approved protocols followed the ethical standards in the Declaration of Helsinki. All participants signed informed consent forms.
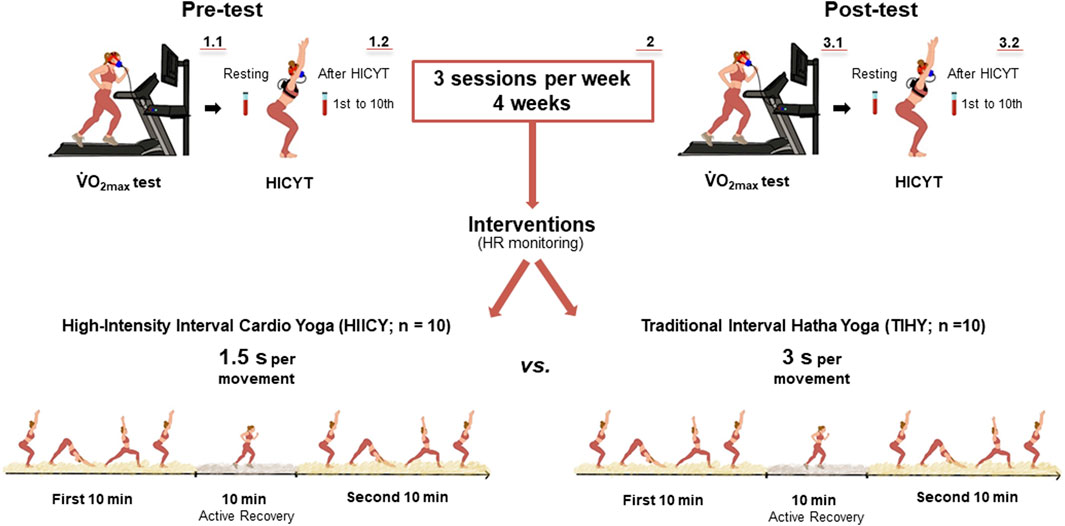
FIGURE 1. Study procedure. The pre-test including
Study procedure
All participants attended two laboratory visits for pre- and post-testing between 4 weeks. Testing was performed at a temperature of 23°C and relative humidity of 50%. Anthropometric data of participants were analyzed using an eight-electrode segmental multi-frequency bioelectrical impedance analyzer (BIA: 20–100 kHz; InBody 270; InBody Co. Ltd., Seoul, Republic of Korea). All participants rested for 2 h after lunchtime and conducted a ramp test for
Maximal oxygen uptake ( O2max) test
The pre- and post-ramp tests were performed via continuous incremental ramp protocols on a treadmill with the breath-by-breath method using a portable gas analyzer (MetaMax 3B; Cortex Biophysik, Leipzig, Germany). The gas analyzer was calibrated before each test with 15% O2 and 5% CO2 (Cortex Biophysik, Leipzig, Germany) and the turbine volume transducer was calibrated using a 3-L syringe (Hans Rudolph, Kansas, United States). An initial warm-up was conducted for 10 min by running at 70% of the estimated HRmax (Helgerud et al., 2022). The ramp protocol was applied for
High-intensity cardio yoga test (HICYT) and 4-week HIICY and TIHY interventions
After
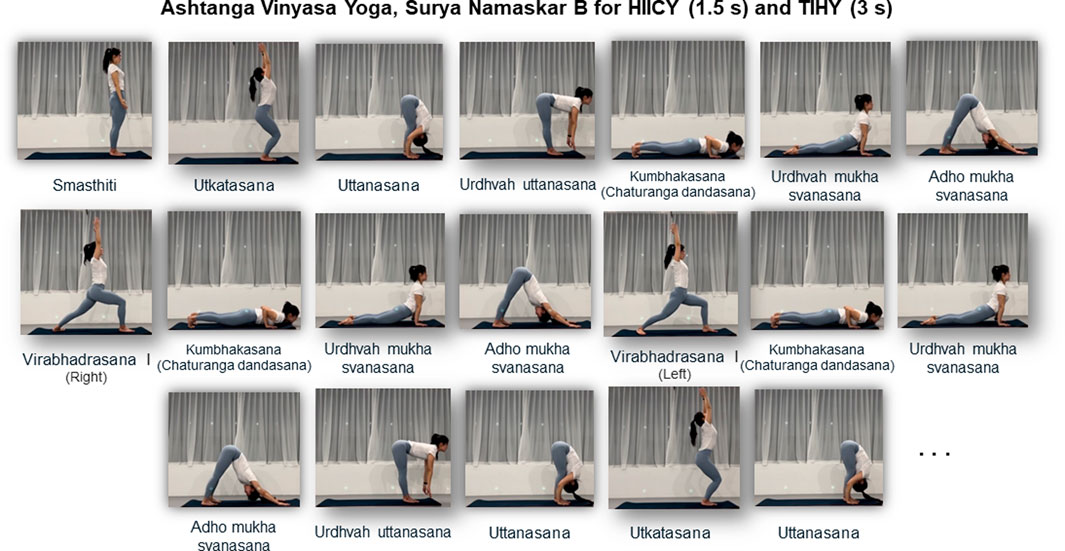
FIGURE 2. Ten-minute Surya Namaskar B sequence. Interval cardio yoga consists of 10-min of active recovery by walking between two sets of 10-min tempo-based Surya Namaskar B; the total duration of HY intervention was 30 min. HIICY; high-intensity interval cardio yoga, TIHY; traditional interval hatha yoga.
Calculations of fat and carbohydrate oxidation rate during HICYT
During HICYT,
Calculations of energy system contributions (PCr-La−-O2 method) during HICYT
The contributions of three energy systems (phosphagen [WPCr], glycolytic [WGly], and oxidative [WOxi] in kJ and %) were calculated by the PCr-La−-O2 method (Julio et al., 2017; Yang et al., 2018; Yang et al., 2022b; Kaufmann et al., 2022; Yang et al., 2023). Oxygen consumption parameters (resting oxygen consumption,
The WOxi was calculated by subtracting
The WGly analysis was performed by La−rest and La−peak values, assuming that an accumulation of 1 mmol·L−1 was equivalent to 3 mL O2·kg−1 of body mass (di Prampero and Ferretti, 1999). Delta La− (∆La−) was calculated as the difference between La−peak after 10-min HICYT and La−rest before (Beneke et al., 2004; Campos et al., 2012; Yang et al., 2018; Yang et al., 2022b).
The WPCr value was calculated using
A caloric quotient of 20.92 kJ was applied in the three energy system calculations (Gastin, 2001). Total energy demand was calculated as the sum of the three energy systems in kJ (Campos et al., 2012). The relative energy system contributions were calculated in % compared to total energy demand.
Statistical analyses
All parameters were analyzed using GraphPad Prism 9.4.1 (GraphPad Prism Software Inc., La Jolla, CA, USA) and data are presented as mean ± standard deviation (SD). Normality of the data was analyzed using the Shapiro−Wilk test. All physiological variables, energetic contributions, and metabolic flexibility during HICYT were analyzed using a two-way (group x time) repeated-measures analysis of variance (ANOVA) with the Greenhouse-Geisser correction for violation of the sphericity assumption. If the main effect was significant, a Bonferroni post-hoc test was performed to compare among different conditions. The significance level was set at p < 0.05. The effect sizes of repeated-measures ANOVA were calculated as partial eta squared [
Results
Two-way repeated-measures ANOVA for absolute, relative
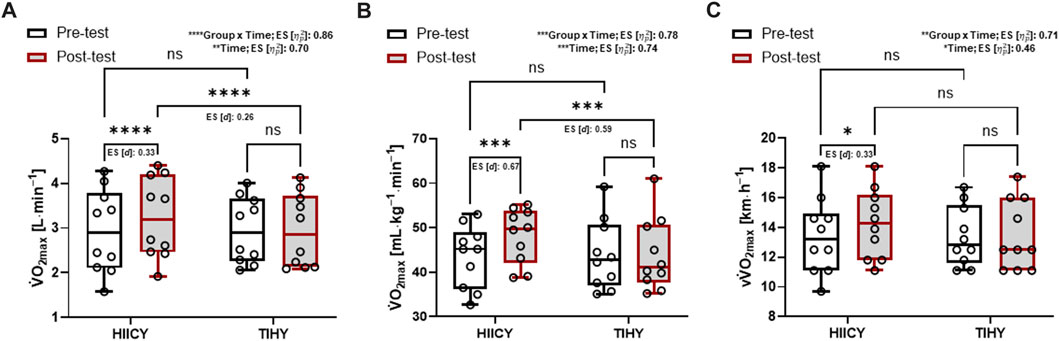
FIGURE 3. Time and group × time interaction effects and comparisons of absolute and relative
Physiological parameters during HICYT
There was no significant difference in anthropometric data between HIICY and TIHY groups. Table 3 shows the physiological parameters during 10-min HICYT pre- and post-tests between different groups. The values of absolute and relative
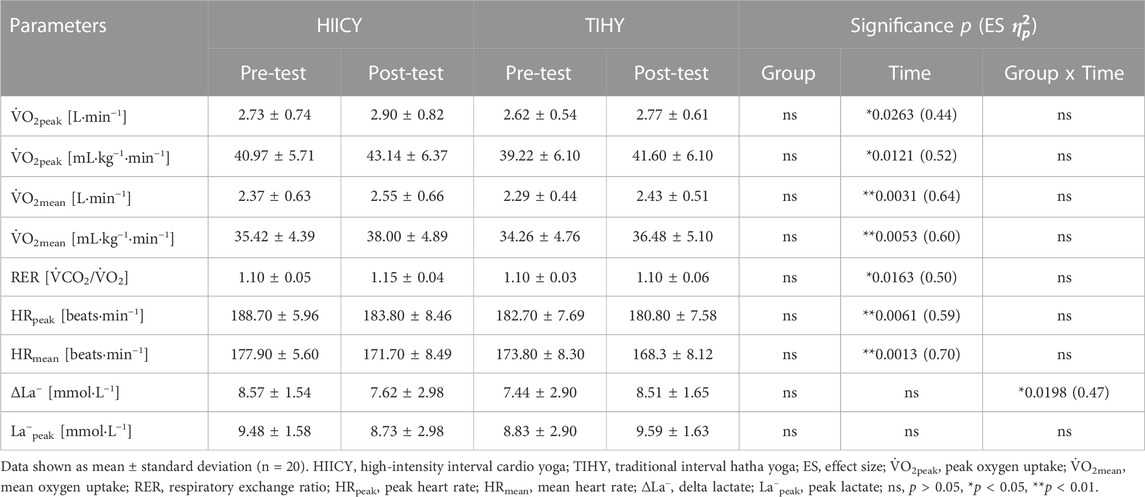
TABLE 3. Physiological parameters during the 10-min high-intensity cardio yoga test between different groups (HIICY; n = 10 and TIHY; n = 10).
Energetic contribution during HICYT
Two-way repeated-measures ANOVA showed significant time effects in absolute (kJ) for WOxi and WTotal, and a group × time interaction effect in absolute WGly (time effects: p < 0.01; [
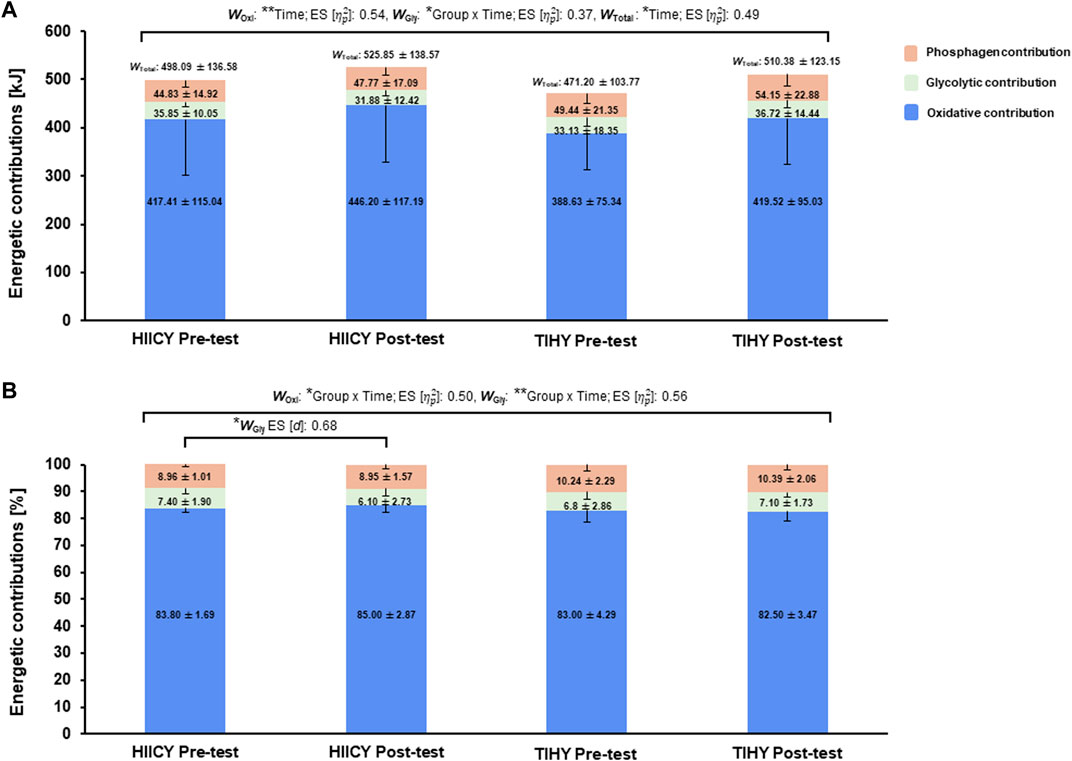
FIGURE 4. Time and group × time interaction effects and comparisons of energetic contributions during the HICYT after 4-week interventions. (A) Comparisons of three energy contributions in kJ between pre- and post-test in HIICY and TIHY groups, respectively and (B) comparisons of three energy contributions in % between pre- and post-testing HIICY and TIHY groups, respectively. ES; effect sizes, WOxi; oxidative energy demand, WGly; glycolytic energy demand, WTotal; total energy demand, HICYT; high-intensity cardio yoga test, HIICY; high-intensity interval cardio yoga, TIHY; traditional interval hatha yoga. *p < 0.05, **p < 0.01.
Metabolic flexibility including FATox and CHOox during HICYT
Two-way repeated-measures ANOVA showed group × time interaction effects at the fourth and time and group × time interaction effects at sixth minute of FATox (p = 0.0312; effect size [
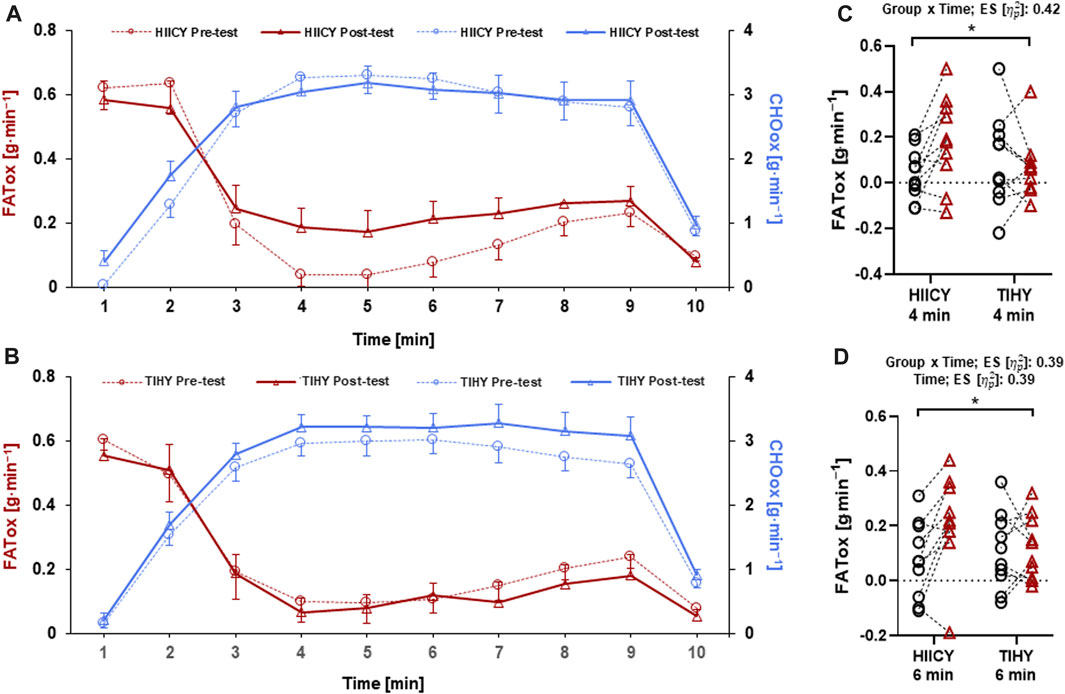
FIGURE 5. FATox and CHOox between HIICY and TIHY during the HICYT. (A) FATox and CHOox in the HIICY group between pre- and post-testing, (B) FATox and CHOox in the TIHY group between pre- and post-testing, (C) group × time interaction effect of FATox at 4 min during the HICYT, and (D) time and group × time interaction effects of FATox at 6 min during the HICYT. FATox; fat oxidation, CHOox; carbohydrate oxidation, HIICY; high-intensity interval cardio yoga, TIHY; traditional interval hatha yoga, HICYT; high-intensity cardio yoga test. *p < 0.05.
Relationships between metabolic flexibility, energetic contributions and physiological variables
High positive correlations were found between WOxi in kJ, and absolute
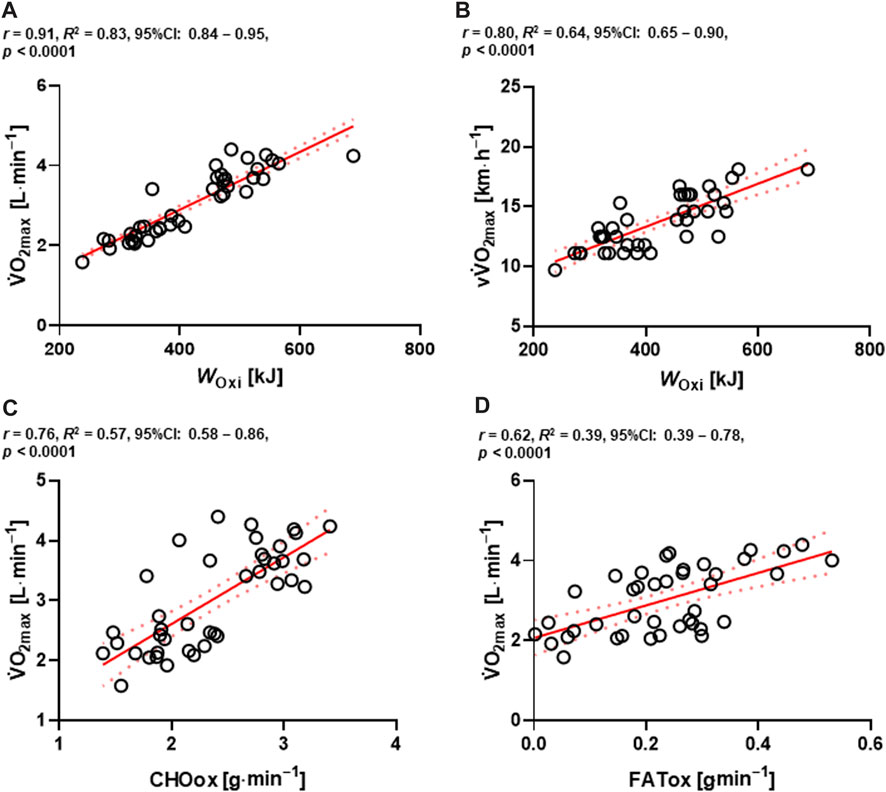
FIGURE 6. Relationships between absolute
Discussion
Training effects of HIIT and HY on health, oxidative capacity, and metabolism have been well reported previously. To date, however, there was no interventional approach to HIICY. It is unclear whether HIICY as HIHY can improve cardiometabolic fitness parameters such as
Regarding maximal aerobic performance, 4-week HIICY improved
HRpeak and HRmean tended to improve post-testing in the HIICY group. Significant increases in pulmonary oxygen uptake and skeletal muscle oxygen demand cause HIIT-induced cardiovascular adaptations during intensive and prolonged HIIT sessions. Exercise-induced improvement in
The reduced value of %WGly with increased
Furthermore, numerous studies have shown an increased density of monocarboxylate transporters (MCT) 1 and 4 after HIIT (Burgomaster et al., 2007; Perry et al., 2008; McGinley and Bishop, 2016). Higher MCT 1 and 4 density increased lactate transport and likely supported a reduction in glycogen breakdown and La− at a given intensity (Perry et al., 2008). Accordingly, group × time interaction effect of energetic contributions was observed and the relative value of WGly post-testing in the HIICY group was decreased (Figure 4B). Because of the aforementioned physiological adaptations, oxidative capacity might be improved, which would in turn increase the lactate elimination rate and increase ATP re-synthesis during high-intensity aerobic exercise (Burgomaster et al., 2008; MacInnis and Gibala, 2017; Brooks, 2018; Hwang et al., 2022; Yang et al., 2023). Indeed, muscle glycogen is likely conserved, delaying the onset of muscle fatigue and improving oxidative exercise performance (Hearris et al., 2018). Also, high positive correlations among WOxi in kJ and absolute
In terms of metabolic flexibility, time and group × time interaction effects of FATox at the fourth and sixth during the HICYT between HIICY and TIHY groups and moderate to high correlations among
In sum, this study has demonstrated increased cardiometabolic fitness, including
This study does have some limitations. First, it only targeted one cohort of physically active adults and the HIICY approach should be investigated in more diverse populations such as in athletes, sedentary people, and clinical populations in further studies. Second, the small sample size might have influenced our results. Therefore, more participants should be recruited in a future study. Third, experimenting with efficient periodization to progressively increase HY intensity from TIHY to HIICY should be considered. Finally, more direct measurements during training using proteomics and metabolomics, and the measurement of fluorescent protein tools should be investigated to determine molecular responses in the future.
Conclusion
Our findings indicate that 4-week of HIICY compared with TIHY improved cardiometabolic fitness, oxidative capacity, and metabolic flexibility in physically active individuals. Therefore, HIICY is a suitable training regimen for HY-specific HIIT, and is appropriate for relatively healthy individuals who may have HY experience but need time-efficient exercise options. Through our study outcomes, it is once again confirmed that exercise intensity during HY is important to improving
Data availability statement
The original contributions presented in the study are included in the article/Supplementary material, further inquiries can be directed to the corresponding author.
Ethics statement
The studies involving humans were approved by the Institutional Review Board of CHA University. The studies were conducted in accordance with the local legislation and institutional requirements. The participants provided their written informed consent to participate in this study. Written informed consent was obtained from the individual(s) for the publication of any potentially identifiable images or data included in this article.
Author contributions
S-YP: Conceptualization, Data curation, Formal Analysis, Investigation, Methodology, Visualization, Writing–original draft, Writing–review and editing. W-HY: Conceptualization, Formal Analysis, Methodology, Project administration, Resources, Supervision, Writing–review and editing.
Funding
The author(s) declare that no financial support was received for the research, authorship, and/or publication of this article.
Acknowledgments
The authors would like to thank all of the participants in this study.
Conflict of interest
The authors declare that the research was conducted in the absence of any commercial or financial relationships that could be construed as a potential conflict of interest.
Publisher’s note
All claims expressed in this article are solely those of the authors and do not necessarily represent those of their affiliated organizations, or those of the publisher, the editors and the reviewers. Any product that may be evaluated in this article, or claim that may be made by its manufacturer, is not guaranteed or endorsed by the publisher.
References
Acevedo E. O., Goldfarb A. H. (1989). Increased training intensity effects on plasma lactate, ventilatory threshold, and endurance. Med. Sci. Sports Exerc 21(5), 563–568. doi:10.1249/00005768-198910000-00011
Aparecido J. M. L., Marquezi M. L., Couto H. L. d. O., Santos T. M. d. S., Cruz A. F. C., Lopes N. B., et al. (2022). Six HIT sessions improve cardiorespiratory fitness and metabolic flexibility in insulin resistant and insulin sensitive adolescents with obesity. Int. J. Environ. Res. Public Health 19 (17), 10568. doi:10.3390/ijerph191710568
Astorino T. A., Allen R. P., Roberson D. W., Jurancich M. (2012). Effect of high-intensity interval training on cardiovascular function, VO2max, and muscular force. J. Strength Cond. Res. 26 (1), 138–145. doi:10.1519/JSC.0b013e318218dd77
Astorino T. A., Schubert M. M. (2018). Changes in fat oxidation in response to various regimes of high intensity interval training (HIIT). Eur. J. Appl. Physiol. 118, 51–63. doi:10.1007/s00421-017-3756-0
Balakumar P., Maung-U K., Jagadeesh G. (2016). Prevalence and prevention of cardiovascular disease and diabetes mellitus. Pharmacol. Res. 113, 600–609. doi:10.1016/j.phrs.2016.09.040
Batacan R. B., Duncan M. J., Dalbo V. J., Tucker P. S., Fenning A. S. (2017). Effects of high-intensity interval training on cardiometabolic health: A systematic review and meta-analysis of intervention studies. Br. J. Sports Med. 51 (6), 494–503. doi:10.1136/bjsports-2015-095841
Beneke R., Beyer T., Jachner C., Erasmus J., Hütler M. (2004). Energetics of karate kumite. Eur. J. Appl. Physiol. 92 (4-5), 518–523. doi:10.1007/s00421-004-1073-x
Bersiner K., Park S. Y., Schaaf K., Yang W. H., Theis C., Jacko D., et al. (2023). Resistance exercise: A mighty tool that adapts, destroys, rebuilds and modulates the molecular and structural environment of skeletal muscle. Phys. Act. Nutr. 27 (2), 78–95. doi:10.20463/pan.2023.0021
Billat L. (2001). Interval training for performance: a scientific and empirical practice. Special recommendations for middle- and long-distance running. Part I: aerobic interval training. Sports Med. 31 (1), 13–31. doi:10.2165/00007256-200131010-00002
Billat V. L., Hill D. W., Pinoteau J., Petit B., Koralsztein J. P. (1996). Effect of protocol on determination of velocity at VO2 max and on its time to exhaustion. Arch. Physiol. Biochem. 104 (3), 313–321. doi:10.1076/apab.104.3.313.12908
Bosquet L., Léger L., Legros P. (2002). Methods to determine aerobic endurance. Sports Med. 32 (11), 675–700. doi:10.2165/00007256-200232110-00002
Brinsley J., Smout M., Girard D., Davison K. (2022). Acute mood and cardiovascular responses to moderate intensity vinyasa yoga, static yin yoga and aerobic exercise in people with depression and/or anxiety disorders: A 5-arm randomised controlled trial. Ment. Health Phys. Act. 22, 100450. doi:10.1016/j.mhpa.2022.100450
Brooks G. A. (2018). The science and translation of lactate shuttle theory. Cell. Metab. 27 (4), 757–785. doi:10.1016/j.cmet.2018.03.008
Buchheit M., Laursen P. B. (2013a). High-intensity interval training, solutions to the programming puzzle: part I: cardiopulmonary emphasis. Sports Med. 43 (5), 313–338. doi:10.1007/s40279-013-0029-x
Buchheit M., Laursen P. B. (2013b). High-intensity interval training, solutions to the programming puzzle: part II: anaerobic energy, neuromuscular load and practical applications. Sports Med. 43 (10), 927–954. doi:10.1007/s40279-013-0066-5
Burgomaster K. A., Cermak N. M., Phillips S. M., Benton C. R., Bonen A., Gibala M. J. (2007). Divergent response of metabolite transport proteins in human skeletal muscle after sprint interval training and detraining. Am. J. Physiol. Regul. Integr. Comp. Physiol. 292 (5), R1970–R1976. doi:10.1152/ajpregu.00503.2006
Burgomaster K. A., Howarth K. R., Phillips S. M., Rakobowchuk M., MacDonald M. J., McGee S. L., et al. (2008). Similar metabolic adaptations during exercise after low volume sprint interval and traditional endurance training in humans. J. Physiol. 586 (1), 151–160. doi:10.1113/jphysiol.2007.142109
Campos F. A., Bertuzzi R., Dourado A. C., Santos V. G., Franchini E. (2012). Energy demands in taekwondo athletes during combat simulation. Eur. J. Appl. Physiol. 112 (4), 1221–1228. doi:10.1007/s00421-011-2071-4
Chrøis K. M., Dohlmann T. L., Søgaard D., Hansen C. V., Dela F., Helge J. W., et al. (2020). Mitochondrial adaptations to high intensity interval training in older females and males. Eur. J. Sport Sci. 20 (1), 135–145. doi:10.1080/17461391.2019.1615556
Coffey V. G., Hawley J. A. (2007). The molecular bases of training adaptation. Sports Med. 37, 737–763. doi:10.2165/00007256-200737090-00001
Costigan S. A., Eather N., Plotnikoff R. C., Taaffe D. R., Lubans D. R. (2015). High-intensity interval training for improving health-related fitness in adolescents: a systematic review and meta-analysis. Br. J. Sports Med. 49 (19), 1253–1261. doi:10.1136/bjsports-2014-094490
de Campos Mello F., de Moraes Bertuzzi R. C., Grangeiro P. M., Franchini E. (2009). Energy systems contributions in 2,000 m race simulation: A comparison among rowing ergometers and water. Eur. J. Appl. Physiol. 107 (5), 615–619. doi:10.1007/s00421-009-1172-9
Di Donato D. M., West D. W., Churchward-Venne T. A., Breen L., Baker S. K., Phillips S. M. (2014). Influence of aerobic exercise intensity on myofibrillar and mitochondrial protein synthesis in young men during early and late postexercise recovery. Am. J. Physiol. Endocrinol. Metab. 306(9), E1025–E1032. doi:10.1152/ajpendo.00487.2013
di Prampero P. E., Ferretti G. (1999). The energetics of anaerobic muscle metabolism: A reappraisal of older and recent concepts. Respir. Physiol. 118(2-3), 103–115. doi:10.1016/s0034-5687(99)00083-3
Egan B., Carson B. P., Garcia-Roves P. M., Chibalin A. V., Sarsfield F. M., Barron N., et al. (2010). Exercise intensity-dependent regulation of peroxisome proliferator-activated receptor coactivator-1 mRNA abundance is associated with differential activation of upstream signalling kinases in human skeletal muscle. J. Physiol. 588 (10), 1779–1790. doi:10.1113/jphysiol.2010.188011
Egan B., Zierath J. R. (2013). Exercise metabolism and the molecular regulation of skeletal muscle adaptation. Cell. Metab. 17 (2), 162–184. doi:10.1016/j.cmet.2012.12.012
Franchini E., Cormack S., Takito M. Y. (2019). Effects of high-intensity interval training on olympic combat sports athletes' performance and physiological adaptation: A systematic review. J. Strength Cond. Res. 33 (1), 242–252. doi:10.1519/JSC.0000000000002957
Fritz C. O., Morris P. E., Richler J. J. (2012). Effect size estimates: current use, calculations, and interpretation. J. Exp. Psychol. Gen. 141 (1), 2–18. doi:10.1037/a0024338
Galgani J. E., Bergouignan A., Rieusset J., Moro C., Nazare J.-A. (2022). Editorial: metabolic flexibility. Front. Nutr. 1254, 946300. doi:10.3389/fnut.2022.946300
Gaskill S. E., Serfass R. C., Bacharach D. W., Kelly J. M. (1999). Responses to training in cross-country skiers. Med. Sci. Sports Exerc 31, 1211–1217. doi:10.1097/00005768-199908000-00020
Gastin P. B. (2001). Energy system interaction and relative contribution during maximal exercise. Sports Med. 31 (10), 725–741. doi:10.2165/00007256-200131100-00003
Gibala M. J., Little J. P., MacDonald M. J., Hawley J. A. (2012). Physiological adaptations to low-volume, high-intensity interval training in health and disease. J. Physiol. 590 (5), 1077–1084. doi:10.1113/jphysiol.2011.224725
Gibala M. J., McGee S. L., Garnham A. P., Howlett K. F., Snow R. J., Hargreaves M. (2009). Brief intense interval exercise activates AMPK and p38 MAPK signaling and increases the expression of PGC-1alpha in human skeletal muscle. J. Appl. Physiol. 106 (3), 929–934. doi:10.1152/japplphysiol.90880.2008
Goodpaster B. H., Sparks L. M. (2017). Metabolic flexibility in health and disease. Cell. Metab. 25 (5), 1027–1036. doi:10.1016/j.cmet.2017.04.015
Hagins M., Moore W., Rundle A. (2007). Does practicing hatha yoga satisfy recommendations for intensity of physical activity which improves and maintains health and cardiovascular fitness? BMC Complement. Altern. Med. 7 (1), 40–49. doi:10.1186/1472-6882-7-40
Hearris M. A., Hammond K. M., Fell J. M., Morton J. P. (2018). Regulation of muscle glycogen metabolism during exercise: implications for endurance performance and training adaptations. Nutrients 10 (3), 298. doi:10.3390/nu10030298
Helgerud J., Haglo H., Hoff J. (2022). Prediction of vo2max from submaximal exercise using the smartphone application myworkout go: validation study of a digital health method. JMIR Cardio 6 (2), e38570. doi:10.2196/38570
Hickson R. C., Rosenkoetter M. A. (1981). Reduced training frequencies and maintenance of increased aerobic power. Med. Sci. Sports Exerc 13(1), 13–16. doi:10.1249/00005768-198101000-00011
Howlett R. A., Parolin M. L., Dyck D. J., Hultman E., Jones N. L., Heigenhauser G. J., et al. (1998). Regulation of skeletal muscle glycogen phosphorylase and PDH at varying exercise power outputs. Am. J. Physiol. 275 (2), R418–R425. doi:10.1152/ajpregu.1998.275.2.R418
Hsu C.-C., Fu T.-C., Yuan S.-S., Wang C.-H., Liu M.-H., Shyu Y.-C., et al. (2019). High-intensity interval training is associated with improved long-term survival in heart failure patients. J. Clin. Med. 8 (3), 409. doi:10.3390/jcm8030409
Hwang J., Moon N.-R., Heine O., Yang W.-H. (2022). The ability of energy recovery in professional soccer players is increased by individualized low-intensity exercise. PLoS One 17 (6), e0270484. doi:10.1371/journal.pone.0270484
Jacob N., So I., Sharma B., Marzolini S., Tartaglia M. C., Oh P., et al. (2023). Effects of high-intensity interval training protocols on blood lactate levels and cognition in healthy adults: systematic review and meta-regression. Sports Med. 53 (5), 977–991. doi:10.1007/s40279-023-01815-2
Jamnick N. A., Pettitt R. W., Granata C., Pyne D. B., Bishop D. J. (2020). An examination and critique of current methods to determine exercise intensity. Sports Med. 50 (10), 1729–1756. doi:10.1007/s40279-020-01322-8
Jeukendrup A., Wallis G. (2005). Measurement of substrate oxidation during exercise by means of gas exchange measurements. Int. J. Sports Med. 26 (S 1), S28–S37. doi:10.1055/s-2004-830512
Julio U. F., Panissa V. L. G., Esteves J. V., Cury R. L., Agostinho M. F., Franchini E. (2017). Energy-system contributions to simulated judo matches. Int. J. Sports Physiol. Perform. 12 (5), 676–683. doi:10.1123/ijspp.2015-0750
Jurov I., Cvijić M., Toplišek J. (2023). Predicting VO2max in competitive cyclists: is the FRIEND equation the optimal choice? Front. Physiol. 14, 987006. doi:10.3389/fphys.2023.987006
Kaufmann S., Latzel R., Beneke R., Hoos O. (2022). Reliability of the 3-component model of aerobic, anaerobic lactic, and anaerobic alactic energy distribution (PCr-la-O2) for energetic profiling of continuous and intermittent exercise. Int. J. Sports Physiol. Perform. 17 (11), 1642–1648. doi:10.1123/ijspp.2022-0115
Kercher V. M., Kercher K., Levy P., Bennion T., Alexander C., Amaral P. C., et al. (2023). 2023 fitness trends from around the globe. ACSM's Health Fit. J. 27 (1), 19–30. doi:10.1249/fit.0000000000000836
Kerr N. R., Booth F. W. (2022). Contributions of physical inactivity and sedentary behavior to metabolic and endocrine diseases. Trends Endocrinol. Metab. 33 (12), 817–827. doi:10.1016/j.tem.2022.09.002
Khoshnaw D. M., Ghadge A. A. (2021). Yoga as a complementary therapy for metabolic syndrome: A narrative review. J. Integr. Med. 19 (1), 6–12. doi:10.1016/j.joim.2020.09.002
Knuiman P., Hopman M. T., Mensink M. (2015). Glycogen availability and skeletal muscle adaptations with endurance and resistance exercise. Nutr. Metab. (Lond) 12 (1), 59–11. doi:10.1186/s12986-015-0055-9
Kodama S., Saito K., Tanaka S., Maki M., Yachi Y., Asumi M., et al. (2009). Cardiorespiratory fitness as a quantitative predictor of all-cause mortality and cardiovascular events in healthy men and women: A meta-analysis. Jama 301 (19), 2024–2035. doi:10.1001/jama.2009.681
Kohn T., Essén-Gustavsson B., Myburgh K. (2011). Specific muscle adaptations in type II fibers after high-intensity interval training of well-trained runners. Scand. J. Med. Sci. Sports 21 (6), 765–772. doi:10.1111/j.1600-0838.2010.01136.x
Kristensen D. E., Albers P. H., Prats C., Baba O., Birk J. B., Wojtaszewski J. F. (2015). Human muscle fibre type-specific regulation of AMPK and downstream targets by exercise. J. Physiol. 593 (8), 2053–2069. doi:10.1113/jphysiol.2014.283267
Krustrup P., Mohr M., Ellingsgaard H., Bangsbo J. (2005). Physical demands during an elite female soccer game: importance of training status. Med. Sci. Sports Exerc 37 (7), 1242–1248. doi:10.1249/01.mss.0000170062.73981.94
Kubukeli Z. N., Noakes T. D., Dennis S. C. (2002). Training techniques to improve endurance exercise performances. Sports Med. 32, 489–509. doi:10.2165/00007256-200232080-00002
Larson-Meyer D. E. (2016). A systematic review of the energy cost and metabolic intensity of yoga. Med. Sci. Sports Exerc 48 (8), 1558–1569. doi:10.1249/MSS.0000000000000922
Laursen P. B., Jenkins D. G. (2002). The scientific basis for high-intensity interval training: optimising training programmes and maximising performance in highly trained endurance athletes. Sports Med. 32 (1), 53–73. doi:10.2165/00007256-200232010-00003
Lee K.-H., Ju H.-M., Yang W.-H. (2021). Metabolic energy contributions during high-intensity hatha yoga and physiological comparisons between active and passive (savasana) recovery. Front. Physiol. 12, 743859. doi:10.3389/fphys.2021.743859
Little J. P., Safdar A., Bishop D., Tarnopolsky M. A., Gibala M. J. (2011). An acute bout of high-intensity interval training increases the nuclear abundance of PGC-1α and activates mitochondrial biogenesis in human skeletal muscle. Am. J. Physiol. Regul. Integr. Comp. Physiol. 300, R1303–R1310. R1303-R1310. doi:10.1152/ajpregu.00538.2010
MacInnis M. J., Gibala M. J. (2017). Physiological adaptations to interval training and the role of exercise intensity. J. Physiol. 595 (9), 2915–2930. doi:10.1113/JP273196
McGinley C., Bishop D. J. (2016). Influence of training intensity on adaptations in acid/base transport proteins, muscle buffer capacity, and repeated-sprint ability in active men. J. Appl. Physiol. 121(6), 1290–1305. doi:10.1152/japplphysiol.00630.2016
Metcalfe R. S., Koumanov F., Ruffino J. S., Stokes K. A., Holman G. D., Thompson D., et al. (2015). Physiological and molecular responses to an acute bout of reduced-exertion high-intensity interval training (REHIT). Eur. J. Appl. Physiol. 115, 2321–2334. doi:10.1007/s00421-015-3217-6
Meyler S., Bottoms L., Muniz-Pumares D. (2021). Biological and methodological factors affecting VO2max response variability to endurance training and the influence of exercise intensity prescription. Exp. Physiol. 106 (7), 1410–1424. doi:10.1113/EP089565
Midgley A. W., McNaughton L. R., Polman R., Marchant D. (2007). Criteria for determination of maximal oxygen uptake: A brief critique and recommendations for future research. Sports Med. 37, 1019–1028. doi:10.2165/00007256-200737120-00002
Mody B. S. (2011). Acute effects of Surya Namaskar on the cardiovascular & metabolic system. J. Bodyw. Mov. Ther. 15 (3), 343–347. doi:10.1016/j.jbmt.2010.05.001
Niemeyer M., Knaier R., Beneke R. (2021). The oxygen uptake plateau-A critical review of the frequently misunderstood phenomenon. Sports Med. 51 (9), 1815–1834. doi:10.1007/s40279-021-01471-4
Ormsbee M. J., Ward E. G., Bach C. W., Arciero P. J., McKune A. J., Panton L. B. (2015). The impact of a pre-loaded multi-ingredient performance supplement on muscle soreness and performance following downhill running. J. Int. Soc. Sports Nutr. 12 (1), 2. doi:10.1186/s12970-014-0063-6
Papp M. E., Lindfors P., Nygren-Bonnier M., Gullstrand L., Wändell P. E. (2016). Effects of high-intensity hatha yoga on cardiovascular fitness, adipocytokines, and apolipoproteins in healthy students: A randomized controlled study. J. Altern. Complement. Med. 22 (1), 81–87. doi:10.1089/acm.2015.0082
Patwardhan A. R. (2017). Yoga research and public health: is research aligned with the stakeholders’ needs? J. Prim. Care Community Health 8 (1), 31–36. doi:10.1177/2150131916664682
Perry C. G., Heigenhauser G. J., Bonen A., Spriet L. L. (2008). High-intensity aerobic interval training increases fat and carbohydrate metabolic capacities in human skeletal muscle. Appl. Physiol. Nutr. Metab. 33 (6), 1112–1123. doi:10.1139/H08-097
Potiaumpai M., Martins M. C. M., Rodriguez R., Mooney K., Signorile J. F. (2016). Differences in energy expenditure during high-speed versus standard-speed yoga: A randomized sequence crossover trial. Complement. Ther. Med. 29, 169–174. doi:10.1016/j.ctim.2016.10.002
Predel H.-G. (2014). Marathon run: cardiovascular adaptation and cardiovascular risk. Eur. Heart J. 35 (44), 3091–3098. doi:10.1093/eurheartj/eht502
Protzen G. V., Bartel C., Coswig V. S., Gentil P., Del Vecchio F. B. (2020). Physiological aspects and energetic contribution in 20s: 10s high-intensity interval exercise at different intensities. PeerJ 8, e9791. doi:10.7717/peerj.9791
Riboli A., Coratella G., Rampichini S., Limonta E., Esposito F. (2022). Testing protocol affects the velocity at VO2max in semi-professional soccer players. Res. Sports Med. 30 (2), 182–192. doi:10.1080/15438627.2021.1878460
Ross R., Blair S. N., Arena R., Church T. S., Després J.-P., Franklin B. A., et al. (2016). Importance of assessing cardiorespiratory fitness in clinical practice: A case for fitness as a clinical vital sign: A scientific statement from the American heart association. Circulation 134 (24), e653–e699. doi:10.1161/CIR.0000000000000461
Sabag A., Little J. P., Johnson N. A. (2022). Low-volume high-intensity interval training for cardiometabolic health. J. Physiol. 600 (5), 1013–1026. doi:10.1113/JP281210
San-Millán I., Brooks G. A. (2018). Assessment of metabolic flexibility by means of measuring blood lactate, fat, and carbohydrate oxidation responses to exercise in professional endurance athletes and less-fit individuals. Sports Med. 48 (2), 467–479. doi:10.1007/s40279-017-0751-x
Sandbakk Ø., Sandbakk S. B., Ettema G., Welde B. (2013). Effects of intensity and duration in aerobic high-intensity interval training in highly trained junior cross-country skiers. J. Strength Cond. Res. 27 (7), 1974–1980. doi:10.1519/JSC.0b013e3182752f08
Santos A. C., Willumsen J., Meheus F., Ilbawi A., Bull F. C. (2023). The cost of inaction on physical inactivity to public health-care systems: A population-attributable fraction analysis. Lancet Glob. Health 11 (1), e32–e39. doi:10.1016/S2214-109X(22)00464-8
Scheltens P., De Strooper B., Kivipelto M., Holstege H., Chételat G., Teunissen C. E., et al. (2021). Alzheimer's disease. Lancet 397 (10284), 1577–1590. doi:10.1016/S0140-6736(20)32205-4
Schmalzl L., Powers C., Henje Blom E. (2015). Neurophysiological and neurocognitive mechanisms underlying the effects of yoga-based practices: towards a comprehensive theoretical framework. Front. Hum. Neurosci. 9, 235. doi:10.3389/fnhum.2015.00235
Schmitz B., Rolfes F., Schelleckes K., Mewes M., Thorwesten L., Krüger M., et al. (2018). Longer work/rest intervals during high-intensity interval training (HIIT) lead to elevated levels of miR-222 and miR-29c. Front. Physiol. 9, 395. doi:10.3389/fphys.2018.00395
Schünemann F., Park S. Y., Wawer C., Theis C., Yang W. H., Gehlert S. (2023). Diagnostics of νLa.max and glycolytic energy contribution indicate individual characteristics of anaerobic glycolytic energy metabolism contributing to rowing performance. Metabolites 13 (3), 317. doi:10.3390/metabo13030317
Seiler S., Jøranson K., Olesen B. V., Hetlelid K. J. (2013). Adaptations to aerobic interval training: interactive effects of exercise intensity and total work duration. Scand. J. Med. Sci. Sports 23 (1), 74–83. doi:10.1111/j.1600-0838.2011.01351.x
Seiler S. (2010). What is best practice for training intensity and duration distribution in endurance athletes? Int. J. Sports Physiol. Perform. 5 (3), 276–291. doi:10.1123/ijspp.5.3.276
Sheykhlouvand M., Gharaat M., Khalili E., Agha-Alinejad H., Rahmaninia F., Arazi H. (2018). Low-volume high-intensity interval versus continuous endurance training: effects on hematological and cardiorespiratory system adaptations in professional canoe polo athletes. J. Strength Cond. Res. 32 (7), 1852–1860. doi:10.1519/JSC.0000000000002112
Sperlich P. F., Holmberg H.-C., Reed J. L., Zinner C., Mester J., Sperlich B. (2015). Individual versus standardized running protocols in the determination of VO2max. J. Sports Sci. Med. 14 (2), 386–393.
Talanian J. L., Holloway G. P., Snook L. A., Heigenhauser G. J., Bonen A., Spriet L. L. (2010). Exercise training increases sarcolemmal and mitochondrial fatty acid transport proteins in human skeletal muscle. Am. J. Physiol. Endocrinol. Metab. 299 (2), E180–E188. doi:10.1152/ajpendo.00073.2010
Tunstall R. J., Mehan K. A., Wadley G. D., Collier G. R., Bonen A., Hargreaves M., et al. (2002). Exercise training increases lipid metabolism gene expression in human skeletal muscle. Am. J. Physiol. Endocrinol. Metab. 283 (1), E66–E72. doi:10.1152/ajpendo.00475.2001
Udhan V. D., WankheDe S. G., Phatale S. R. (2018). Effect of yoga on cardio-respiratory health markers: physical fitness index and maximum oxygen consumption (VO 2 max). J. Clin. Diagn. Res. 12 (8), 21–23. doi:10.7860/JCDR/2018/36819.11932
Van loon L. J., Greenhaff P. L., Constantin-Teodosiu D., Saris W. H., Wagenmakers A. J. (2001). The effects of increasing exercise intensity on muscle fuel utilisation in humans. J. Physiol. 536 (1), 295–304. doi:10.1111/j.1469-7793.2001.00295.x
Warren J. L., Hunter G. R., Gower B. A., Bamman M. M., Windham S. T., Moellering D. R., et al. (2020). Exercise effects on mitochondrial function and lipid metabolism during energy balance. Med. Sci. Sports Exerc 52 (4), 827–834. doi:10.1249/MSS.0000000000002190
Weiner R. B., Baggish A. L. (2012). Exercise-induced cardiac remodeling. Prog. Cardiovasc Dis. 54 (5), 380–386. doi:10.1016/j.pcad.2012.01.006
Wiecha S., Kasiak P. S., Cieśliński I., Takken T., Palka T., Knechtle B., et al. (2023). External validation of VO2max prediction models based on recreational and elite endurance athletes. PLoS One 18 (1), e0280897. doi:10.1371/journal.pone.0280897
Wisløff U., Støylen A., Loennechen J. P., Bruvold M., Rognmo Ø., Haram P. M., et al. (2007). Superior cardiovascular effect of aerobic interval training versus moderate continuous training in heart failure patients: A randomized study. Circulation 115 (24), 3086–3094. doi:10.1161/CIRCULATIONAHA.106.675041
Yang W.-H., Heine O., Grau M. (2018). Rapid weight reduction does not impair athletic performance of Taekwondo athletes–A pilot study. PLoS One 13 (4), e0196568. doi:10.1371/journal.pone.0196568
Yang W.-H., Park J.-H., Park S.-Y., Park Y. (2022a). Energetic contributions including gender differences and metabolic flexibility in the general population and athletes. Metabolites 12 (10), 965. doi:10.3390/metabo12100965
Yang W.-H., Park S.-Y., Kim T., Jeon H.-J., Heine O., Gehlert S. (2023). A modified formula using energy system contributions to calculate pure maximal rate of lactate accumulation during a maximal sprint cycling test. Front. Physiol. 14, 1147321. doi:10.3389/fphys.2023.1147321
Keywords: metabolism, physiological adaptation, lactate, aerobic capacity, V˙O2max
Citation: Park S-Y and Yang W-H (2023) Applied high-intensity interval cardio yoga improves cardiometabolic fitness, energetic contributions, and metabolic flexibility in healthy adults. Front. Physiol. 14:1279505. doi: 10.3389/fphys.2023.1279505
Received: 18 August 2023; Accepted: 21 September 2023;
Published: 17 October 2023.
Edited by:
Hun-Young Park, Konkuk University, Republic of KoreaReviewed by:
Małgorzata Grabara, Institute of Sport Sciences, PolandWon-Sang Jung, Dongseo University, Republic of Korea
Copyright © 2023 Park and Yang. This is an open-access article distributed under the terms of the Creative Commons Attribution License (CC BY). The use, distribution or reproduction in other forums is permitted, provided the original author(s) and the copyright owner(s) are credited and that the original publication in this journal is cited, in accordance with accepted academic practice. No use, distribution or reproduction is permitted which does not comply with these terms.
*Correspondence: Woo-Hwi Yang, eXdoMTIzNUBjaGEuYWMua3I=
†ORCID:So-Young Park, orcid.org/0000-0002-1871-8366; Woo-Hwi Yang, orcid.org/0000-0001-6618-1834