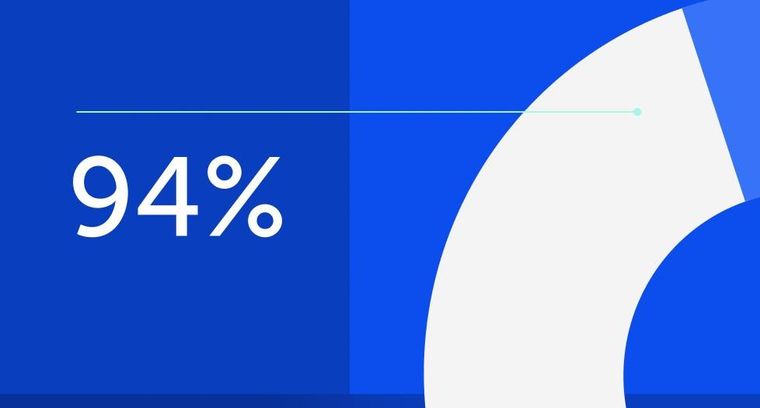
94% of researchers rate our articles as excellent or good
Learn more about the work of our research integrity team to safeguard the quality of each article we publish.
Find out more
HYPOTHESIS AND THEORY article
Front. Physiol., 03 October 2023
Sec. Invertebrate Physiology
Volume 14 - 2023 | https://doi.org/10.3389/fphys.2023.1263453
This article is part of the Research TopicInvertebrate Neurophysiology - of Currents, Cells, and CircuitsView all 15 articles
Nervous systems of vertebrates and invertebrates show a common modular theme in the flow of information for cost-benefit decisions. Sensory inputs are incentivized by integrating stimulus qualities with motivation and memory to affect appetitive state, a system of homeostatic drives, and labelled for directionality. Appetitive state determines action responses from a repertory of possibles and transmits the decision to a premotor system that frames the selected action in motor arousal and appropriate postural and locomotion commands. These commands are then sent to the primary motor pattern generators controlling the motorneurons, with feedback at each stage. In the vertebrates, these stages are mediated by forebrain pallial derivatives for incentive and directionality (olfactory bulb, cerebral cortex, pallial amygdala, etc.) interacting with hypothalamus (homeostasis, motivation, and reward) for action selection in the forebrain basal ganglia, the mid/hindbrain reticular formation as a premotor translator for posture, locomotion, and arousal state, and the spinal cord and cranial nuclei as primary motor pattern generators. Gastropods, like the predatory sea slug Pleurobranchaea californica, show a similar organization but with differences that suggest how complex brains evolved from an ancestral soft-bodied bilaterian along with segmentation, jointed skeletons, and complex exteroceptors. Their premotor feeding network combines functions of hypothalamus and basal ganglia for homeostasis, motivation, presumed reward, and action selection for stimulus approach or avoidance. In Pleurobranchaea, the premotor analogy to the vertebrate reticular formation is the bilateral “A-cluster” of cerebral ganglion neurons that controls posture, locomotion, and serotonergic motor arousal. The A-cluster transmits motor commands to the pedal ganglia analogs of the spinal cord, for primary patterned motor output. Apparent pallial precursors are not immediately evident in Pleurobranchaea’s central nervous system, but a notable candidate is a subepithelial nerve net in the peripheral head region that integrates chemotactile stimuli for incentive and directionality. Evolutionary centralization of its computational functions may have led to the olfaction-derived pallial forebrain in the ancestor’s vertebrate descendants and their analogs in arthropods and annelids.
Did the general neural organization of decision and action selection precede or follow the evolution of complex body form and behavior? We argue that despite broad differences in complexity (detail) of brain and body, soft-bodied invertebrates like gastropods even with primitive, incompletely centralized brains show a blueprint for a modular organization of the nervous system similar to vertebrates, although simpler in detail. Vertebrates, insects, and polychaete annelids likely evolved segmentation and articulated skeletons with jointed appendages independently (Mullins et al., 2011; Hochner, 2013; Katz, 2016). These acquisitions are adaptations for speed and agility beyond what is easily gotten by a soft body. The skeletal joints allow local sensory-motor regulation of segmental musculature, and sensory monitoring of joint angles for incorporating into central motor templates. Vertebrates and insects share analogous modular designs for computational functions that mediate decision, spatial mapping, and motivation (Loesel and Heuer, 2010; Tosches and Arendt, 2013; Holland, 2016). Such functions may have originated in the ancestral bilaterian with simpler anatomy and behavior or could have evolved independently multiple times. The above lineages each have more complicated body forms than the expected urbilaterian ancestor (Hejnol and Martindale, 2008).
For comparative analysis, it is useful to examine circuits in organisms more like the expected common ancestor. It is pertinent that a simple sea slug, with primitive ciliary locomotion and lacking segmentation and appendages, has modular design in neuronal network coordination similar to vertebrates for posture, locomotion, and general arousal. Thus, the general scheme controlling locomotion and posture could have preceded the evolution of segmented bodies and articulated appendages. Could then the neuronal circuitry of cost-benefit decision and action selection be also analogous, and possibly homologous in origin, among the phyla?
We previously discussed the evolution of the nervous system and developed hypotheses on the nature of the nervous system in the common urbilaterian ancestor (Gillette and Brown, 2015). As the ancestor is unavailable, we took a classic comparative approach. We used the sea slug Pleurobranchaea californica as a model system whose nervous system and behavior have been examined and are likely relatively close to the ancestor’s (Figure 1). The nervous system and behavior are also known in more detail than for many other animal systems, particularly with respect to mechanisms for decision-making, and are amenable to the comparative approach. Moreover, there is much useful information on brain and behavior of related gastropods. Among other model systems available, information on the brains and behaviors of vertebrate animals has been amassed over more than a century by thousands of researchers. Thus, we may compare markedly distant lineages that split over 500 Myr ago into protostome and deuterostome by examining functional analogies in brain and behavior.
FIGURE 1. Pleurobranchaea californica, head shot. The chemotactile oral veil is flared above the mouth. The oral veil papillae and the lateral tentacles have ciliated chemotactile receptors that feed into a peripheral subepithelial network to mediate incentive and stimulus location. Photo by Fred Zwicky.
Comparisons of functional analogies in brain and behavior of the streamlined Pleurobranchaea with the rather more complex vertebrates may show commonalities interpretable in terms of direct or parallel evolution. Gastropods like Pleurobranchaea boast systems that may be closer in brain, body, and behavior to the simple common ancestor than the more complex, segmented vertebrates, insects, and polychaete annelids with jointed appendages. The former lack complicating segmentation and jointed skeletons with appendages and many of them locomote primitively by myriads of cilia paddling their way through secreted mucus, as expected of the common ancestor. Moreover, especially for the opisthobranch sea slugs like Pleurobranchaea, their soft bodies and behaviors are quite simple, and they are hermaphroditic with minimal investment in offspring care, thereby further reducing neural complexities of animals with two different sexes that may brood their young.
Pleurobranchaea shows simple reward-based learning for approach or avoidance of prey (Noboa and Gillette, 2013). Their behavior and neuronal circuitry are characterized well enough to generate computational simulations which express cost-benefit decisions in autonomous agents with efficient, reward-based mechanisms for stimulus valuations. The algorithms used reproduce the basic modular functions of nervous systems as treated here (Brown et al., 2018; Gribkova et al., 2020).
Thus, a fuller answer to the nature and origin of complexity in human brain and behavior can begin to be sought in the simplicity of our very early ancestors. We may safely assume that the problems faced by the ancestors were basically the same as they are today, and that their nervous systems were designed by natural selection to generate appropriate behavioral algorithms. The threefold basic imperatives of life are: 1) acquisition of resources; 2) defense; and 3) reproduction. These three constraints of behavioral design are largely interconnected and interdependent. Even single cells may follow this design, where biochemical pathways do the computations of an integrating nervous system, connecting sensors to motor pathways for movement and secretion. We annotate these constraints briefly, below, and then continue on to document the organization of simple and complex nervous systems in resolving the imperatives.
The essential resource is energy, which underlies the abilities for defense and reproduction. More complicated faces of this basic resource come from derived characters aimed at maximizing and conserving energy, like shelter, clothing, and storage in fatty tissues. Even social characters such as attention to status and reputation, play, and aggression intimately involve the economics of energy. Other resources are the chemical building blocks of cells: amino acids, fats, carbohydrates, and water.
Once life evolved to a point where it could acquire energy and use it in reproduction, strong selective forces would have had it evolve mechanisms to keep from losing it, such as a cell membrane (which then involved a whole new set of problems resulting in the evolution of ion pumps). Once organisms had developed to conserve energy, they became treasure troves to some that devised ways to break into their stores and use them for themselves. This would have been an early manifestation of predation.
As early cells devised asexual and sexual ways of reproduction, impelled by the obvious selective forces excluding nonreproductive cells, reproductive predators evolved to hijack their mechanisms, becoming intracellular parasites, and perhaps degenerating from free-living cells to viruses (Forterre, 2006). Thus, the three design constraints are inextricably intertwined.
For most animal species that have been observed in detail, the cost-benefit decisions employed to solve the problems of acquisition, defense, and reproduction integrate internal state, sensory input, and memory. This holds for vertebrates, insects, and gastropod mollusks (see Gillette et al., 2000; Clore et al., 2021). The dynamic changes in internal state for motivation, in varying sensory input, and in memory plasticity may lead to a broad spectrum of cost-benefit responses tailored to each situation. There are sufficient data presently to compare the neural architectures that support such decision-making. First, we would like to review the functional modules of the vertebrate nervous system, then compare them with analogs in the sea slug.
In each of these functions there is a modular design for the flow of information from stimulus to response: incentive-directionality; reward expectation; approach-avoidance decision; decision implementation in motor response.
A modular organization of the mammalian nervous system, in terms of interacting subsystems, has been long recognized both anatomically, physiologically, and computationally (Arbib, 2007).
Nutrition, thermoregulation, hydration, and social behaviors like sex and parental care are homeostatic drives founded in the hypothalamus, whose neural activity represents appetitive state and biases behavioral choices for the different goals. Nutritional state is fairly well understood. In vertebrates, nutritional state is closely monitored by hypothalamic neurons sensitive to serum glucose, digestive hormones, and hormonal indicators of fat and glycogen storage. Motivation, in terms of hunger/satiation, regulates exploration after food resources or avoidance of such activity. Moreover, the hypothalamic circuits and hormones that mediate hunger and satiety appropriately regulate the sensitivity of the reward system (Cassidy and Tong, 2017).
Action selection for cost-benefit decisions is determined by the basal ganglia, which integrate sensory incentives weighed with motivation-related reward and memory of experience (Turner and Desmurget, 2010). The basal ganglia have access to cortical areas with memories laden with positive and negative affect related to context and can compare possible costs and benefits of potential decisions. Final summation leads to selection of motor actions that essentially represent approach or avoidance of a predicted situation. The decision outputs are sent to premotor areas in the reticular system of the brainstem that extends from the upper part of the midbrain to the lower part of the medulla. Important dopaminergic and serotonergic modulatory inputs to the basal ganglia come from the substantia nigra compacta and raphe nuclei, respectively, for reward and arousal.
Premotor patterning networks in the hindbrain reticular system are disinhibited by basal ganglia inputs to form premotor commands configured for the selected motor actions. From the reticular formation the commands for locomotion and posture descend to the spinal cord to be translated into direct motor action by the segmental pattern generators. The reticular system has critical roles in maintaining arousal state in behavioral and motor systems through ascending pathways to the forebrain and descending pathways to the spinal cord via the reticulospinal tracts. The raphe nuclei of the reticular system are critical serotonergic sources for modulation of mood, reward, and arousal (Teissier et al., 2015; Venkatraman et al., 2017). Ascending fibers from the anterior raphe nuclei innervate the basal ganglia and other forebrain derivatives, and descending fibers from the posterior nuclei innervate the spinal cord pattern generators and sensory pathways.
The spinal cord is segmented, and the coupled spinal pattern generators’ final outputs are through their motorneurons. Spinal pattern generators receive direct feedback from muscle and tendon stretch receptors and from descending inputs that can smooth coordination and aid adaptation of motor pattern to uneven terrain (Rossignol et al., 2006).
Olfactory bulb, cortex, pallial amygdala, and other pallial structures are thought to have evolved as elaborations of the olfactory system, with the addition of more sensory inputs from complex exteroceptors such as mediate vision, audition, proprioception, and taste (Reiner et al., 1998; Redgrave et al., 1999; Smeets et al., 2000; Aboitiz et al., 2003; Grillner et al., 2013; Aboitiz and Montiel, 2015; Jacobs, 2023). Functionally, these structures interact with each other and the subpallial striatum to integrate reward, motivation, affect, and memory to compute incentive for decision making and to place it in physical context with directional information. The motor cortex apparently only appeared in evolution with mammals and is not treated further here.
Comparison of the gastropod decision-making system, represented by Pleurobranchaea, with vertebrates’ finds general similarities in organization (Figure 2) However, a major exception regarding vertebrate forebrain is discussed below. Mainly, they differ in complexity of detail related to the elaborate bodies and exteroceptors of the vertebrates. Previous work described neuronal circuitry underlying cost-benefit and approach-avoidance decisions in Pleurobranchaea’s foraging (Jing and Gillette, 2000; Gillette and Jing, 2001; Yafremava et al., 2007; Hirayama et al., 2012; Hirayama and Gillette, 2012; Brown, 2014). These circuits share functional analogies with the vertebrate striatum and hypothalamus in that they express homeostatic state and instruct goal-directed action selection (Gillette and Brown, 2015), with the analogies extending to coordination of locomotion in goal-directed behavior. Thus, a cluster of neurons, the A-cluster, works analogously to the reticular system for posture, locomotion, and arousal (Lee et al., 2023), and a peripheral neuronal network may serve functions of forebrain pallial derivatives for incentive and simple place-coding. Of the several ganglia of Pleurobranchaea’s central nervous system (CNS), the cerebropleural ganglion contains the circuits in its cerebral lobes that function analogously to the combined basal ganglia-hypothalamus and the reticular system. Sensory input to the ganglion is integrated with motivation and memory to decide actions. These decisions descend to pattern generators in the pedal ganglia, which themselves have functions analogous to the vertebrate spinal cord in final patterning of direct motor output (Figure 2). A significant difference may be that Pleurobranchaea’s basal ganglia analog has only the direct neural pathway output integrating sensation, internal state, and memory, and lacks the explicit comparison of potential costs and benefits afforded by the indirect pathway originating in the vertebrate striatum, as may the insects (Strausfeld and Hirth, 2013).
FIGURE 2. Proposed analogs of Pleurobranchaea californica and vertebrate central nervous systems, as represented by the human brain and spinal cord. The feeding network (blue striped) in the cerebral lobes of the cerebropleural ganglion combines homeostatic functions of the vertebrate hypothalamus in motivation and decisive action selection of the basal ganglia. The C-cluster (magenta) is functionally analogous to the serotonergic portions of the vertebrate ascending reticular system. The A cluster (cyan) is functionally analogous to the vertebrate reticular system, projecting to pattern generators in the pedal ganglion, which are in turn analogous to the mammalian spinal cord in final motor pattern output. The serotonergic cells of the A-cluster are analogous to the serotonergic cells of the descending raphe nuclei. The chemotactile sensory subepithelial network (yellow) of Pleurobranchaea is proposed as resembling an ancestral precursor to pallial derivatives of the ancient olfactory system in evolution, such as the olfactory bulb, neocortex, hippocampus, basal ganglia, amygdaloid complex and others.
The distributed feeding motor network combines the homeostatic functions of the hypothalamus in motivation and the basal ganglia in decisive action selection. Hunger/satiety is represented in the excitation state and neuronal activity configuration of the feeding network (Hirayama et al., 2012; Hirayama and Gillette, 2012; Hirayama et al., 2014). Sensory input incentivized by memory summates with the neuronal representation of motivation to bring the network towards or away from exploring a stimulus. In cost-benefit decisions for approach-avoidance, hungry animals approach food odors, but when satiated they turn away from them. The feeding network excitation state sets the thresholds for active feeding, which can vary a million-fold between ravenous hunger and logy satiation (Davis and Gillette, 1978; London and Gillette, 1986; Hirayama et al., 2014). Incentivized input to the feeding network may be mediated by dopaminergic afferents from chemotactile areas (Kabotyanski et al., 2000; Brown et al., 2018), which may well include the effects of learned preference and avoidance (Elliott and Susswein, 2002). The functions of Pleurobranchaea’s homeostatic feeding network in action selection are located in the subpallial basal ganglia of the vertebrate, however still under the influence of the hypothalamus analog of the feeding network. Thus, in evolution the computations of homeostasis and action selection may have been either separated to different modules in evolution in the vertebrates or joined in the feeding network of gastropods.
Figure 3 shows a map of the neurons of the premotor feeding network, the A-cluster that controls posture and locomotion with its serotonergic As1-4 interneurons, and the anterior serotonergic C-cluster to which we will refer below.
FIGURE 3. Drawing of the dorsal surface of the cerebropleural and pedal ganglia showing identified neuronal somata of networks for the feeding network, the A-Cluster for posture, locomotion, and motor arousal, and the serotonergic C-Cluster. Filled circles show serotonin immunoreactive somata. The somata are bilaterally symmetrical, but only shown unilaterally. Abbreviations: MCG, metacerebral giant neuron; PCP, phasic paracerebral interneuron; PSE, polysynaptic excitor of the PCP; PCT, tonic paracerebral interneuron; I1, I2, Interneurons 1; and 2, Interneuron 2; BWN, body wall nerve; sBWN, small body wall nerve; CBC, cerebrobuccal connective; aCPC, anterior cerebropedal connective; pCPC, posterior cerebropedal connective; CVC, cerebrovisceral connective; MN, mouth nerve; OVN, oral veil nerve; RN, rhinophore nerve; SCC, subcerebral commissure; TN, tentacle nerve; aLBWN, anterior lateral body wall nerve; pLBWN, posterior lateral body wall nerve; PC, pedal commissure; pPC, parapedal commissure; aPN, anterior pedal nerve; mPN, medial pedal nerve; and pPN, posterior pedal nerve.
Action selections by the feeding network are quite simple choices between approach and avoidance of stimuli, and whether to locomote or not. Avoidance turns are the default responses to sensory stimuli in the absence of feeding network excitation. Higher activity in the feeding network converts the turn responses to approach, possibly by shunting sensory input to the turn network from one side to the other (Gillette et al., 2000; Hirayama and Gillette, 2012; Brown, 2014; Hirayama et al., 2014). Thus, partly satiated animals actively avoid weaker feeding stimuli and approach stronger ones. Active feeding causes inhibition of the premotor neurons that mediate turning and locomotion, which prevents the animal from overrunning its prey before finishing the meal. Figure 4 shows a simplest model based on synaptic disinhibition routing excitation to the opposite side of the turn motor network, reflecting similar downstream disinhibitory mechanisms by the basal ganglia in action selection.
FIGURE 4. A simplest model for control of the turn by excitation state of the feeding motor network. A simple neuronal switch mechanism under control of corollary discharge from the CPG mediating feeding is postulated. (A) When the activity of the feeding network is low, sensory inputs from the oral veil drive avoidance turns away from the site of the stimulus. (B) Increased activity of the network, through hunger or greater sensory stimulation, changes the avoidance response to an approach-turn toward the stimulus by a postulated disinhibitory mechanism that shifts activity to the contralateral side of the turn motor network. Figure modified from Gillette and Brown (2015).
In Pleurobranchaea, the analog of the vertebrate reticular formation lies in a bilaterally paired group of neurons, the A-cluster (Jing and Gillette, 1999; Jing and Gillette, 2000; Lee et al., 2023), posterior to the feeding network neurons in the cerebral lobes. A-cluster homologs are also found in other gastropods (Sudlow et al., 1998; Popescu and Frost, 2002; Jing et al., 2008; Jing et al., 2009). The A-cluster was first recognized in a search for the premotor pattern generator for the escape swim. This search was correctly based on a presumption that the swim network would incorporate the neurons of the turn network, which could be studied for approach-avoidance decisions. Thus, it was eventually found that the A-cluster in different states of coordination mediates the escape swim, approach-avoidance turns, crawling locomotion, and likely righting behavior (Jing and Gillette, 1995; Jing and Gillette, 1999; Jing and Gillette, 2000; Jing and Gillette, 2003). Thus, A-cluster neurons are concerned with different aspects of posture and locomotion, very similar to the functions of the reticular system. The major differences in complexity can be simply related to control of a soft body vs. an articulated, skeletonized body.
Moreover, as for the raphe nuclei of the reticular formation, the behavioral functions of the A cluster are critically dependent on a serotonergic network. The four bilaterally paired serotonergic As1-4 neurons lie at the core of the A-cluster and provide the neuromodulatory drive to swimming, turning, and crawling locomotion. They are interconnected both chemically by serotonergic synapses and electrically, and fire in different patterns with the different motor outputs. Unlike the concentrated localization of the ∼7 raphe nuclei in the vertebrate brain, serotonergic neurons of the gastropod brain are dispersed within and among the ganglia, lying within motor pattern generators like the A-cluster and the As1-4 cells and the feeding network and the C-cluster, where they drive motor arousal (Jing and Gillette, 2003; Gillette, 2006). However, their interconnections allow activity in one group to contribute to the arousal of another and thereby its motor network. If the serotonergic neurons of the gastropod were indeed homologous to those of the vertebrate raphe nuclei, it could mean either that the serotonergic neurons became concentrated in vertebrates or that they dispersed in the mollusks (Gillette, 2006).
Like the posterior raphe nuclei, the serotonergic As1-4 neurons send descending innervation to the pedal ganglia analogs of the spinal pattern generators to drive crawling locomotion and approach-avoidance turns in different states of coordination (Jing and Gillette, 2003; Lee et al., 2023). They are also chemically coupled to the serotonergic neurons of the feeding motor network. In coordination with other identified neurons of the A cluster, they sustain the swimming escape response episode (Jing and Gillette, 1999). Thus, their actions are obligatory to the actions of posture and locomotion mediated by the A-cluster analog of the reticular activating system.
The serotonergic C-cluster neurons and the metacerebral giant neurons of the cerebral ganglia lobes innervate the feeding motor network and the peripheral sensory neuronal network. They are functionally analogous to the serotonergic portions of the vertebrate ascending reticular formation (Figure 2), which innervates the hypothalamus, basal ganglia, and other pallial derivatives. The C-clusters of 12+ neurons each and the metacerebral giant neurons lie anteriorly in the cerebral lobes and are both electrically and chemically coupled (Gillette and Davis, 1977; Jing and Gillette, 2000). They are embedded in the feeding network where during the active feeding cycle they receive alternating synaptic excitation and inhibition during the retraction and protraction (swallowing and biting) phases of the feeding apparatus, respectively (Gillette and Davis, 1977). The C-cluster cells richly innervate the cerebral ganglia lobes and send axons to the periphery to innervate the subepithelial network (see below) of the chemotactile oral veil (Moroz et al., 1997), where ongoing work indicates that serotonin promotes sensory gain. The paired metacerebral neurons provide neuromodulatory input to the buccal ganglion’s feeding central pattern generator (CPG), but the majority of its outputs are peripheral: to the esophagus/crop, the musculature of the buccal mass, and the chemosensory mouth area (Gillette, 1991). Serotonergic modulation of the feeding network sets the basal excitation state and lowers the thresholds for both the approach turn and active feeding (Hirayama and Gillette, 2012; Hirayama et al., 2014). Serotonergic modulation of sensory gain in the gastropod peripheral nervous system may contribute to incentivization of stimuli and conceivably also contributes to attentional mechanisms and odor/taste learning.
Like the vertebrate spinal cord, the gastropod pedal ganglia mediate the larger part of direct motor patterning for posture and locomotion. They receive descending commands, including neuromodulatory serotonergic input, from the A-cluster in Pleurobranchaea, to express the motor outputs of approach and avoidance turns, escape swimming, body withdrawal, and locomotion. They correspond to the subesophageal ganglia of other ecdysozoans like the arthropods and annelids, which partially serially segment in development to form the ventral nerve cords, themselves similar to vertebrate spinal cord in development and molecular signatures (Vergara et al., 2017).
The pallial circuitry of the vertebrate brain is largely concerned with processing incentive on the basis of stimulus qualities, motivation, reward experience (hedonic affect), and memory, and with directional information for stimuli. Pleurobranchaea has no such central neuronal module that has yet been identified. However, there is a peripheral, subepithelial network (SeN) of interneurons postsynaptic to the primary receptors for chemotactile stimuli in the oral veil that does perform simple functions of incentivizing stimuli and estimating their directional location (Figure 2). Thus, it is quite possible that the origin of the telencephalic derivatives of the ancient olfactory system in evolution–the olfactory bulb, cortex, hippocampus, basal ganglia, amygdaloid complex and the others–may have lain in a peripheral SeN processing incentive and directionality like that in the oral veil of Pleurobranchaea, which became centralized and enhanced with the evolving body complexities of segmentation and jointed skeletons.
Olfaction is among the most primitive of senses (Cuschieri, 1976). Elaboration of olfactory systems and associated structures for odor learning is closely tied to the emergence of pallial structures. Directional olfaction may have formed the basis for the evolution of memory organization in the bilaterian brain, with hippocampal-like structures used for mapping and encoding the spatial distributions of novel odorants (Jacobs, 2012; 2023). Further, the olfactory system is the only sensory system that bypasses the thalamus, a structure for multi-modal sensory relay; instead, the olfactory bulb projects directly to amygdala and piriform cortex, which are important for emotion and memory (Kay and Sherman, 2007; Jacobs, 2023). Like the olfactory bulb, the SeN of Pleurobranchaea is notably dopaminergic and is richly innervated by the serotonergic neurons of the C-cluster.
The primary olfactory centers in vertebrate and insect brains seem to have similar neuronal types, sensory coding mechanisms, coherent oscillatory activity, and organization, including dense olfactory projections to structures critical for memory: vertebrate hippocampus and insect mushroom bodies (Strausfeld and Hildebrand, 1999; Tomer et al., 2010). Some gastropods share similar features, including presence of glomeruli and coherent oscillations in procerebral lobes of pulmonate mollusks, which are important for odor learning (Gelperin, 1999; Kay, 2015).
In Pleurobranchaea, while there is no clear analogue to mushroom bodies or procerebral lobes, learning and mapping of olfactory stimuli are supported by the oral veil SeN. Computations in the SeN allow Pleurobranchaea to map locations of environmental stimuli relative to its body. The cell bodies whose axons carry the location information have central cell bodies (unpublished observations). This somatotopic map of stimulus location and distribution over a target area can provide a template for directed motor reaction, and further, it offers a potential evolutionary substrate type for place coding, contextual memory and spatial memory, as seen in arthropod mushroom and ellipsoid bodies, and vertebrate hippocampus (Devaud et al., 2015; Eichenbaum, 2017).
The SeN suggests insights into the evolution of learning and memory circuits by its nature as an extensive interconnected sensory network with dopaminergic elements that also receives prominent central serotonergic innervation. The interactions of dopamine and serotonin are notable in other systems for supporting hetero-associative reward feedback underlying attention and short- and long-term memory. A basic form of associative learning is classical conditioning, where a neutral stimulus is paired with a biologically potent stimulus, such as food, reward, or even pain, to elicit a conditioned response. The simplest of associative memory systems that can support classical conditioning must have circuitry that handles at least two different kinds of input, such as a sensory signal and motor feedback signal, or a sensory signal paired with a reward feedback signal (e.g., Hawkins et al., 1998). Second-order conditioning involves the associative pairing of a neutral stimulus with an already conditioned (previously neutral) stimulus, to elicit a conditioned response (Brogden, 1939). Circuitry for second-order conditioning must therefore contain a neuron or set of neurons that responds to both types of neutral stimuli.
Thus, the higher in complexity a memory system is, the more intermediate circuitry it must have between neurons that respond to sensory input and those that receive reward or motor feedback. This is particularly apparent in systems capable of sequence learning and spatial memory, such as mammalian hippocampus and octopus’ vertical lobe, which have both extensive auto-associative and hetero-associative architectures. The SeN expresses potential precursors to these associative architectures as it consists of an extensive interconnected sensory network that also receives intricate serotonergic innervation, which could well provide hetero-associative reward feedback.
Spatial learning demands neuronal circuitry that can associate the order and strength of sequentially encountered stimuli, with their connectivity influenced by reward. In principle, this does not look complicated. Such learning may have been selected for in evolution in motile opportunists for efficiently exploiting particular territories, and for homing to a safe refuge when not foraging. While the nomadic and homeless Pleurobranchaea most likely lack spatial learning, they provide an interesting example of a potential neuronal precursor for more context-dependent substrates of associative learning. In particular, the Pleurobranchaea SeN is well-positioned for associatively pairing specific odor and reward stimuli, and it may even support pairing of the location of the specific olfactory stimulus relative to its body with reward feedback. This possible encoding of additional context, i.e., the somatotopic stimulus location, may enable the simple short-term working odor memory that may aid Pleurobranchaea in navigating its olfactory environment (Yafremava and Gillette, 2011). A recurrent inhibitory network was proposed as a simple averaging computation of stimulus loci for motor targeting (Yafremava and Gillette, 2011). Moreover, the serotonergic innervation of the SeN by the C-cluster cells could well provide a mechanism for working memory, attention, and long-term memory through heterosynaptic facilitation.
We have summarized marked analogies between the complex vertebrate systems, and to some degree those of insects, with the nervous systems of gastropods much simpler in body form and behavior. Figure 5 recapitulates the functional analogies, possible homologies and/or convergences, between the nervous systems of the gastropod Pleurobranchaea and vertebrates.
FIGURE 5. Functional analogs in nervous systems of Pleurobranchaea (A) and vertebrates (B); comparing the flow of information from sensory input to motor output. In the vertebrates the incentive and directionality functions of the gastropod subepithelial network are centralized and markedly enhanced in pallial cortex (entorhinal, hippocampal, etc.) for vision, audition, and posture. Action selection for approach-avoidance decisions in the vertebrates, which in Pleurobranchaea is the province of the feeding network, is separated to the subpallial basal ganglia. The motivational and homeostatic functions of the Pleurobranchaea feeding network are retained by the vertebrate hypothalamus. The multifunctional premotor network in the A-cluster neurons of Pleurobranchaea is analogous to the vertebrate reticular formation, framing action decisions in terms of motor arousal, posture, and locomotion. The pedal ganglia and spinal cord receive motor commands from the premotor networks and express the primary motor patterns.
The evolved neural complexities of the vertebrates and insects may be largely ascribed to their segmentation and jointed skeletons, which lend them speed and agility through ability to monitor body posture for motor planning, to more functional equilibrium organs, and to their high resolution exteroceptors for vision, vibration, and electroreception in some cases. However, we are able to describe a similar modular organization of the nervous system in the gastropod Pleurobranchaea, though so much simpler in body form and behavior, with many fewer neurons, and even incomplete centralization of the CNS. Figure 5 summarizes the analogous neural modules between Pleurobranchaea and vertebrates that mediate action selection and reward as feeding network/basal ganglia and hypothalamus. The analogies for posture, locomotion, and motor arousal are the neuron group A-cluster/reticular system of the mid-to-hindbrain. For the serotonergic raphe nuclei of the vertebrate reticular system, the Pleurobranchaea analogy is a distributed serotonergic network with ascending and descending projections, and for primary motor pattern generation, the spinal cord is analogous to the Pleurobranchaea pedal ganglia.
Finally, we introduced the SeN as a simplest analog of the pallial derivatives (olfactory bulb, cortex, etc.). The SeN receives interconnected input from primary sensory receptors to compute incentive from stimulus qualities and to estimate the averaged stimulus source direction (place) for directed motor response. Its final output targets are at the feeding network, where it sums with appetitive state, and at the turn motor network in the A cluster, where it sets the moment-to-moment amplitude of the approach-avoidance turn. The computations of incentive and stimulus location lend the SeN qualities which could have been precursor to the more detailed computations of the vertebrate forebrain for incentive, for the computations of more complicated place codes of more environmental stimuli transduced by complex eyes and ears, and for the eventual evolution of more complex cognitive ability in episodic memory for signatures in space and time.
In the vertebrates, these stages are mediated by pallial derivatives for incentive (olfactory bulb, cerebral cortex, amygdala, &c) interacting with hypothalamus (motivation and reward) for action selection. The reticular formation acts as a premotor translator for posture, locomotion, and arousal state, and the spinal cord and cranial nuclei as primary motor pattern generators.
Finally, our work here may contribute to an answer to whether the modular organizations of vertebrate and arthropod brains evolved independently or were inherited and embellished from a soft-bodied, unsegmented bilaterian ancestor. The marked similarities observed in gastropods are consistent with a common inheritance and may invite future comparative molecular and developmental analyses to cast further light on this compelling question.
The original contributions presented in the study are included in the article/Supplementary Material, further inquiries can be directed to the corresponding author.
EG: Conceptualization, Writing–original draft, Writing–review and editing. CL: Conceptualization, Writing–original draft, Writing–review and editing, Investigation. JB: Data curation, Writing–review and editing, Investigation. JC: Data curation, Investigation, Writing–review and editing. YL: Data curation, Investigation, Writing–review and editing. TN: Data curation, Investigation, Writing–review and editing. RG: Conceptualization, Funding acquisition, Writing–original draft.
The author(s) declare financial support was received for the research, authorship, and/or publication of this article. This work was supported by Office of Naval Research Grant N00014-19-1-2373. TN was also supported in part by the grants from the National Science Foundation (1557923) and the National Institute of Neurological Disorders and Stroke (R01NS114491) to Leonid Moroz.
The authors declare that the research was conducted in the absence of any commercial or financial relationships that could be construed as a potential conflict of interest.
All claims expressed in this article are solely those of the authors and do not necessarily represent those of their affiliated organizations, or those of the publisher, the editors and the reviewers. Any product that may be evaluated in this article, or claim that may be made by its manufacturer, is not guaranteed or endorsed by the publisher.
Aboitiz F., Montiel J. F. (2015). Olfaction, navigation, and the origin of isocortex. Front. Neurosci. 9, 402. doi:10.3389/fnins.2015.00402
Aboitiz F., Morales D., Montiel J. (2003). The evolutionary origin of the mammalian isocortex: towards an integrated developmental and functional approach. Behav. Brain Sci. 26 (5), 535–552. doi:10.1017/s0140525x03000128
Arbib M. A. (2007). Modular models of brain function. Scholarpedia 2 (3), 1869. doi:10.4249/scholarpedia.1869
Brown J. W. (2014). Reciprocal interactions between feeding and turning motor networks mediate foraging decisions in a predatory sea-slug. PhD thesis. Champaign: University of Illinois at Urbana-Champaign.
Brown J. W., Schaub B. M., Klusas B. L., Tran A. X., Duman A. J., Haney S. J., et al. (2018). A role for dopamine in the peripheral sensory processing of a gastropod mollusc. PLOS One 13 (12), e0208891. doi:10.1371/journal.pone.0208891
Cassidy R. M., Tong Q. (2017). Hunger and satiety gauge reward sensitivity. Front. Endocrinol. 8, 104. doi:10.3389/fendo.2017.00104
Clore G. L., Proffitt D. R., Zadra J. R. (2021). “Feeling, seeing, and liking: how bodily resources inform perception and emotion,” in Handbook of embodied psychology (Berlin, Germany: Springer), 43–64.
Cuschieri A. (1976). Functional anatomy of olfactory sense organs. St. Luke's Hosp. Gazette 11 (2), 98–106.
Davis W. J., Gillette R. (1978). Neural correlate of behavioral plasticity in command neurons of Pleurobranchaea. Science 199 (4330), 801–804. doi:10.1126/science.622572
Devaud J.-M., Papouin T., Carcaud J., Sandoz J.-C., Grünewald B., Giurfa M. (2015). Neural substrate for higher-order learning in an insect: mushroom bodies are necessary for configural discriminations. Proc. Natl. Acad. Sci. 112 (43), E5854–E5862. doi:10.1073/pnas.1508422112
Eichenbaum H. (2017). The role of the hippocampus in navigation is memory. J. Neurophysiology 117 (4), 1785–1796. doi:10.1152/jn.00005.2017
Elliott C., Susswein A. (2002). Comparative neuroethology of feeding control in molluscs. J. Exp. Biol. 205 (7), 877–896. doi:10.1242/jeb.205.7.877
Forterre P. (2006). The origin of viruses and their possible roles in major evolutionary transitions. Virus Res. 117 (1), 5–16. doi:10.1016/j.virusres.2006.01.010
Gelperin A. (1999). Oscillatory dynamics and information processing in olfactory systems. J. Exp. Biol. 202 (14), 1855–1864. doi:10.1242/jeb.202.14.1855
Gillette R., Brown J. W. (2015). The sea slug, Pleurobranchaea californica: A signpost species in the evolution of complex nervous systems and behavior. Integr. Comp. Biol. 55 (6), 1058–1069. doi:10.1093/icb/icv081
Gillette R., Davis W. J. (1977). The role of the metacerebral giant neuron in the feeding behavior of Pleurobranchaea. J. Comp. Physiology 116 (2), 129–159. doi:10.1007/bf00605400
Gillette R. (2006). Evolution and function in serotonergic systems. Integr. Comp. Biol. 46 (6), 838–846. doi:10.1093/icb/icl024
Gillette R., Huang R.-C., Hatcher N., Moroz L. L. (2000). Cost-benefit analysis potential in feeding behavior of a predatory snail by integration of hunger, taste, and pain. Proc. Natl. Acad. Sci. 97 (7), 3585–3590. doi:10.1073/pnas.97.7.3585
Gillette R., Jing J. (2001). The role of the escape swim motor network in the organization of behavioral hierarchy and arousal in Pleurobranchaea. Am. Zoologist 41 (4), 983–992. doi:10.1093/icb/41.4.983
Gillette R. (1991). On the significance of neuronal giantism in gastropods. Biol. Bull. 180 (2), 234–240. doi:10.2307/1542393
Gribkova E. D., Catanho M., Gillette R. (2020). Simple aesthetic sense and addiction emerge in neural relations of cost-benefit decision in foraging. Sci. Rep. 10, 9627. doi:10.1038/s41598-020-66465-0
Grillner S., Robertson B., Stephenson-Jones M. (2013). The evolutionary origin of the vertebrate basal ganglia and its role in action selection. J. Physiol. 591 (22), 5425–5431. doi:10.1113/jphysiol.2012.246660
Hawkins R. D., Greene W., Kandel E. R. (1998). Classical conditioning, differential conditioning, and second-order conditioning of the Aplysia gill-withdrawal reflex in a simplified mantle organ preparation. Behav. Neurosci. 112 (3), 636–645. doi:10.1037//0735-7044.112.3.636
Hejnol A., Martindale M. Q. (2008). Acoel development indicates the independent evolution of the bilaterian mouth and anus. Nature 456 (7220), 382–386. doi:10.1038/nature07309
Hirayama K., Catanho M., Brown J. W., Gillette R. (2012). A core circuit module for cost/benefit decision. Front. Neurosci. 6, 123. doi:10.3389/fnins.2012.00123
Hirayama K., Gillette R. (2012). A neuronal network switch for approach/avoidance toggled by appetitive state. Curr. Biol. 22 (2), 118–123. doi:10.1016/j.cub.2011.10.055
Hirayama K., Moroz L. L., Hatcher N. G., Gillette R. (2014). Neuromodulatory control of a goal-directed decision. PLOS One 9 (7), e102240. doi:10.1371/journal.pone.0102240
Hochner B. (2013). How nervous systems evolve in relation to their embodiment: what we can learn from octopuses and other molluscs. Brain, Behav. Evol. 82 (1), 19–30. doi:10.1159/000353419
Jacobs L. F. (2012). From chemotaxis to the cognitive map: the function of olfaction. Proc. Natl. Acad. Sci. 109 (1), 10693–10700. doi:10.1073/pnas.1201880109
Jacobs L. F. (2023). The PROUST hypothesis: the embodiment of olfactory cognition. Anim. Cogn. 26 (1), 59–72. doi:10.1007/s10071-022-01734-1
Jing J., Gillette R. (1999). Central pattern generator for escape swimming in the notaspid sea slug Pleurobranchaea californica. J. Neurophysiology 81 (2), 654–667. doi:10.1152/jn.1999.81.2.654
Jing J., Gillette R. (2003). Directional avoidance turns encoded by single interneurons and sustained by multifunctional serotonergic cells. J. Neurosci. 23 (7), 3039–3051. doi:10.1523/JNEUROSCI.23-07-03039.2003
Jing J., Gillette R. (2000). Escape swim network interneurons have diverse roles in behavioral switching and putative arousal in Pleurobranchaea. J. Neurophysiology 83 (3), 1346–1355. doi:10.1152/jn.2000.83.3.1346
Jing J., Gillette R. (1995). Neuronal elements that mediate escape swimming and suppress feeding behavior in the predatory sea slug Pleurobranchaea. J. Neurophysiology 74 (5), 1900–1910. doi:10.1152/jn.1995.74.5.1900
Jing J., Gillette R., Weiss K. R. (2009). Evolving concepts of arousal: insights from simple model systems. Rev. Neurosci. 20, 405–427. doi:10.1515/revneuro.2009.20.5-6.405
Jing J., Vilim F. S., Cropper E. C., Weiss K. R. (2008). Neural analog of arousal: persistent conditional activation of a feeding modulator by serotonergic initiators of locomotion. J. Neurosci. 28 (47), 12349–12361. doi:10.1523/JNEUROSCI.3855-08.2008
Kabotyanski E. A., Baxter D. A., Cushman S. J., Byrne J. H. (2000). Modulation of fictive feeding by dopamine and serotonin in Aplysia. J. Neurophysiology 83 (1), 374–392. doi:10.1152/jn.2000.83.1.374
Katz P. S. (2016). Phylogenetic plasticity in the evolution of molluscan neural circuits. Curr. Opin. Neurobiol. 41, 8–16. doi:10.1016/j.conb.2016.07.004
Kay L. M. (2015). Olfactory system oscillations across phyla. Curr. Opin. Neurobiol. 31, 141–147. doi:10.1016/j.conb.2014.10.004
Kay L. M., Sherman S. M. (2007). An argument for an olfactory thalamus. Trends Neurosci. 30 (2), 47–53. doi:10.1016/j.tins.2006.11.007
Lee C. A., Brown J. W., Gillette R. (2023). Coordination of locomotion by serotonergic neurons in the predatory gastropod Pleurobranchaea californica. J. Neurosci. 43 (20), 3647–3657. doi:10.1523/JNEUROSCI.1386-22.2023
Loesel R., Heuer C. M. (2010). The mushroom bodies–prominent brain centres of arthropods and annelids with enigmatic evolutionary origin. Acta Zool. 91 (1), 29–34. doi:10.1111/j.1463-6395.2009.00422.x
London J. A., Gillette R. (1986). Mechanism for food avoidance learning in the central pattern generator of feeding behavior of Pleurobranchae californica. Proc. Natl. Acad. Sci. 83 (11), 4058–4062. doi:10.1073/pnas.83.11.4058
Moroz L. L., Sudlow L. C., Jing J., Gillette R. (1997). Serotonin-immunoreactivity in peripheral tissues of the opisthobranch molluscs Pleurobranchaea californica and Tritonia diomedea. J. Comp. Neurology 382 (2), 176–188. doi:10.1002/(sici)1096-9861(19970602)382:2<176:aid-cne3>3.0.co;2-0
Mullins O. J., Hackett J. T., Buchanan J. T., Friesen W. O. (2011). Neuronal control of swimming behavior: comparison of vertebrate and invertebrate model systems. Prog. Neurobiol. 93 (2), 244–269. doi:10.1016/j.pneurobio.2010.11.001
Noboa V., Gillette R. (2013). Selective prey avoidance learning in the predatory sea-slug Pleurobranchaea californica. J. Exp. Biol. 216, 3231–3236. doi:10.1242/jeb.079384
Popescu I. R., Frost W. N. (2002). Highly dissimilar behaviors mediated by a multifunctional network in the marine mollusk Tritonia diomedea. J. Neurosci. 22 (5), 1985–1993. doi:10.1523/JNEUROSCI.22-05-01985.2002
Redgrave P., Prescott T. J., Gurney K. (1999). The basal ganglia: a vertebrate solution to the selection problem? Neuroscience 89, 1009–1023. doi:10.1016/S0306-4522(98)00319-4
Reiner A., Medina L., Veenman C. L. (1998). Structural and functional evolution of the basal ganglia in vertebrates. Brain Res. Rev. 28 (3), 235–285. doi:10.1016/s0165-0173(98)00016-2
Rossignol S., Dubuc R., Gossard J.-P. (2006). Dynamic sensorimotor interactions in locomotion. Physiol. Rev. 86 (1), 89–154. doi:10.1152/physrev.00028.2005
Smeets W. J., Marin O., Gonzalez A. (2000). Evolution of the basal ganglia: new perspectives through a comparative approach. J. Anat. 196 (4), 501–517. doi:10.1046/j.1469-7580.2000.19640501.x
Strausfeld N. J., Hildebrand J. G. (1999). Olfactory systems: common design, uncommon origins? Curr. Opin. Neurobiol. 9 (5), 634–639. doi:10.1016/S0959-4388(99)00019-7
Strausfeld N. J., Hirth F. (2013). Deep homology of arthropod central complex and vertebrate basal ganglia. Science 340 (6129), 157–161. doi:10.1126/science.1231828
Sudlow L. C., Jing J., Moroz L. L., Gillette R. (1998). Serotonin immunoreactivity in the central nervous system of the marine molluscs Pleurobranchaea californica and Tritonia diomedea. J. Comp. Neurology 395 (4), 466–480. doi:10.1002/(sici)1096-9861(19980615)395:4<466:aid-cne4>3.0.co;2-#
Teissier A., Chemiakine A., Inbar B., Bagchi S., Ray R. S., Palmiter R. D., et al. (2015). Activity of raphé serotonergic neurons controls emotional behaviors. Cell Rep. 13 (9), 1965–1976. doi:10.1016/j.celrep.2015.10.061
Tomer R., Denes A. S., Tessmar-Raible K., Arendt D. (2010). Profiling by image registration reveals common origin of annelid mushroom bodies and vertebrate pallium. Cell 142 (5), 800–809. doi:10.1016/j.cell.2010.07.043
Tosches M. A., Arendt D. (2013). The bilaterian forebrain: an evolutionary chimaera. Curr. Opin. Neurobiol. 23 (6), 1080–1089. doi:10.1016/j.conb.2013.09.005
Turner R. S., Desmurget M. (2010). Basal ganglia contributions to motor control: a vigorous tutor. Curr. Opin. Neurobiol. 20 (6), 704–716. doi:10.1016/j.conb.2010.08.022
Venkatraman A., Edlow B. L., Immordino-Yang M. H. (2017). The brainstem in emotion: a review. Front. Neuroanat. 11, 15. doi:10.3389/fnana.2017.00015
Vergara H. M., Bertucci P. Y., Hantz P., Tosches M. A., Achim K., Vopalensky P., et al. (2017). Whole-organism cellular gene-expression atlas reveals conserved cell types in the ventral nerve cord of Platynereis dumerilii. Proc. Natl. Acad. Sci. U. S. A. 114 (23), 5878–5885. doi:10.1073/pnas.1610602114
Yafremava L. S., Anthony C. W., Lane L., Campbell J. K., Gillette R. (2007). Orienting and avoidance turning are precisely computed by the predatory sea-slug Pleurobranchaea californica McFarland. J. Exp. Biol. 210 (4), 561–569. doi:10.1242/jeb.02697
Keywords: evolution, homology, analogy, pallium, reticular system, Pleurobranchaea
Citation: Gribkova ED, Lee CA, Brown JW, Cui J, Liu Y, Norekian T and Gillette R (2023) A common modular design of nervous systems originating in soft-bodied invertebrates. Front. Physiol. 14:1263453. doi: 10.3389/fphys.2023.1263453
Received: 19 July 2023; Accepted: 12 September 2023;
Published: 03 October 2023.
Edited by:
James Newcomb, New England College, United StatesReviewed by:
Angelique Christine Paulk, Massachusetts General Hospital and Harvard Medical School, United StatesCopyright © 2023 Gribkova, Lee, Brown, Cui, Liu, Norekian and Gillette. This is an open-access article distributed under the terms of the Creative Commons Attribution License (CC BY). The use, distribution or reproduction in other forums is permitted, provided the original author(s) and the copyright owner(s) are credited and that the original publication in this journal is cited, in accordance with accepted academic practice. No use, distribution or reproduction is permitted which does not comply with these terms.
*Correspondence: Rhanor Gillette, cmhhbm9yQGlsbGlub2lzLmVkdQ==
Disclaimer: All claims expressed in this article are solely those of the authors and do not necessarily represent those of their affiliated organizations, or those of the publisher, the editors and the reviewers. Any product that may be evaluated in this article or claim that may be made by its manufacturer is not guaranteed or endorsed by the publisher.
Research integrity at Frontiers
Learn more about the work of our research integrity team to safeguard the quality of each article we publish.