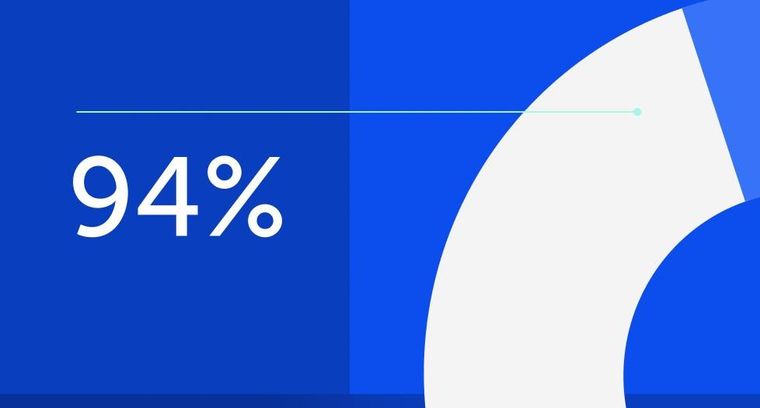
94% of researchers rate our articles as excellent or good
Learn more about the work of our research integrity team to safeguard the quality of each article we publish.
Find out more
REVIEW article
Front. Physiol., 01 September 2023
Sec. Developmental Physiology
Volume 14 - 2023 | https://doi.org/10.3389/fphys.2023.1250134
This article is part of the Research TopicIntrauterine Growth Restriction: Screening and OutcomesView all 5 articles
Intrauterine growth restriction (IUGR) arises when maternal stressors coincide with peak placental development, leading to placental insufficiency. When the expanding nutrient demands of the growing fetus subsequently exceed the capacity of the stunted placenta, fetal hypoxemia and hypoglycemia result. Poor fetal nutrient status stimulates greater release of inflammatory cytokines and catecholamines, which in turn lead to thrifty growth and metabolic programming that benefits fetal survival but is maladaptive after birth. Specifically, some IUGR fetal tissues develop enriched expression of inflammatory cytokine receptors and other signaling cascade components, which increases inflammatory sensitivity even when circulating inflammatory cytokines are no longer elevated after birth. Recent evidence indicates that greater inflammatory tone contributes to deficits in skeletal muscle growth and metabolism that are characteristic of IUGR offspring. These deficits underlie the metabolic dysfunction that markedly increases risk for metabolic diseases in IUGR-born individuals. The same programming mechanisms yield reduced metabolic efficiency, poor body composition, and inferior carcass quality in IUGR-born livestock. The ω-3 polyunsaturated fatty acids (PUFA) are diet-derived nutraceuticals with anti-inflammatory effects that have been used to improve conditions of chronic systemic inflammation, including intrauterine stress. In this review, we highlight the role of sustained systemic inflammation in the development of IUGR pathologies. We then discuss the potential for ω-3 PUFA supplementation to improve inflammation-mediated growth and metabolic deficits in IUGR offspring, along with potential barriers that must be considered when developing a supplementation strategy.
Fetal intrauterine growth restriction (IUGR) occurs in an estimated 1 out of every 5 pregnancies worldwide, resulting in almost 30 million babies being affected by the condition each year (Kesavan and Devaskar, 2019). Asymmetrical IUGR is a pathological cause of low birth weight and small-for-gestational-age (SGA) newborns. Unlike genetic causes of SGA, IUGR is an adaptive response to chronic fetal hypoxemia, hypoglycemia, and other sustained nutritional stresses, since slower fetal growth rates require less nutrients (Sharma et al., 2016; Kesavan and Devaskar, 2019). However, these adaptations also reduce newborn vigor and substantially increases the risk for early morbidity and mortality (Garite et al., 2004). Globally, IUGR-born infants suffer 3-fold greater perinatal demise than babies born at appropriate size for their gestational age (Garite et al., 2004). Most commonly, IUGR-born babies survive but are at much greater risk for chronic metabolic disorders, including obesity, hyperlipidemia, and type 2 diabetes (Hales and Barker, 2001; Hicks and Yates, 2021). This is because the programmed metabolic thrift that underlies IUGR and benefits the nutrient-deprived fetus becomes an environmental mismatch after birth, as offspring are no longer hypoxemic and typically have better nutritional opportunities (Gibbs et al., 2020; Posont et al., 2021). Hales and Barker were the first to link IUGR-induced low birthweight to lifelong metabolic dysfunction and the myriad associated health disorders (Hales et al., 1991; Hales and Barker, 1992). In the decades since, research has begun to identify the root causes for this link. For example, IUGR-born infants typically undergo compensatory catch-up growth prior to adolescence that is driven more so by fat deposition than by lean tissue growth (Ong et al., 2000; Dulloo et al., 2012). Greater adiposity compounds programmed metabolic thrift and further increases the risk for insulin resistance, high blood pressure, and greater body mass index (Ong and Dunger, 2000; Mericq et al., 2005; Lurbe et al., 2009). In adulthood, these risks often manifest in diabetes, hypertension, central obesity, hyperlipidemia, and heart disease (Barker et al., 1993; Barker et al., 2002). Although modern medical advances have markedly improved global IUGR infant survival rates, prevalence of the condition has remained static for decades (Goldenberg and Culhane, 2007; Mathews and Driscoll, 2017). Developing countries in South Asia and Sub-Saharan Africa remain at the highest risk for IUGR pregnancies, but rates in developed countries have also increased since 1981 (Gurung et al., 2022). Moreover, rates among African-Americans and among individuals from low-income areas have increased disproportionately in the US (Goldenberg and Culhane, 2007).
Prenatal stressors in livestock and mammalian wildlife species induce the same fetal programming mechanisms responsible for IUGR in humans (De Blasio et al., 2007a; Yates et al., 2019). The lack of perinatal vigor makes IUGR-born animals more susceptible to starvation and predation, which is a major animal welfare issue that costs the US livestock industry on average about 8% of its annual product (Milligan BNF and Kramer, 2002; Wu et al., 2006). Like humans, most IUGR-born animals survive but exhibit inefficient growth and less desirable carcasses that create a great economic burden (Greenwood et al., 1998; Ogata et al., 1999; Milligan BNF and Kramer, 2002). There is an explicitly-recognized need for more efficient livestock production to feed the world’s rapidly increasing population, which is expected to double by 2050 (Godfray et al., 2010). Improving growth efficiency in IUGR-born livestock would allow more food to be produced from the same number of animals without overgrowing normal animals. The clear impact of IUGR on lifelong health in humans and on sustainability for the livestock industry makes strategies to target maladaptive fetal programming a fundamental need. This review presents the evidence for the role of inflammatory programming in IUGR-associated metabolic pathologies and discusses the potential nutraceutical efficacy of anti-inflammatory omega-3 polyunsaturated fatty acids (ω-3 PUFA) in mitigating those outcomes.
Fetal IUGR can result from any maternofetal stressor that stunts placental development or otherwise limits fetal nutrient supply. For humans, environmental and social stresses, nutritional imbalance due to poor or limited diet, unhealthy lifestyle choices, and pregnancies occurring after the age of 35 are some of the many factors that increase the risk of impaired placental development and growth (Beard et al., 2009; Nardozza et al., 2017). For livestock, common causes of fetal and placental growth restriction include chronic heat stress, restricted feed intake due to drought or mismanagement, and grazing of noxious forages (Greenwood and Cafe, 2007; Robinson et al., 2013). Placental insufficiency is also common in small ruminants carrying multi-fetal pregnancies and in swine carrying large litters (Milligan BNF and Kramer, 2002; De Blasio et al., 2007a). Inadequate blood flow and/or nutrient supply during the critical window for placental development (mid-gestation for most species) causes stunting that ultimately prevents the placenta from fulfilling the expanding nutritional needs of the growing fetus, even when the cause of stunting has been resolved (Burton and Jauniaux, 2018). As the fetus outgrows the stunted placenta in late gestation, progressive IUGR ensues in a predictable pattern (Nardozza et al., 2017). Several animal models have been developed to study IUGR, from pigs (Tang and Xiong, 2022) to non-human primates (Chassen et al., 2020). Sheep are particularly good for IUGR research. The clinical characteristics and developmental milestones of sheep pregnancies are remarkably comparable to humans and other ruminant livestock, as previously described in detail (Yates et al., 2018; Beede et al., 2019). Sheep fetuses are uncommonly resilient, and ewes are typically easy to obtain and house (Beede et al., 2019). A popular model for natural IUGR induction is to expose pregnant ewes to chronic heat stress during peak placental growth, which reliably induces placental insufficiency and in turn fetal IUGR (Beede et al., 2019). Other natural IUGR sheep models include maternal nutrient restriction, maternofetal inflammation, high-altitude hypoxemia, and behavioral stress (Zhang et al., 1998; Beede et al., 2019). Placental insufficiency can also be created artificially via placental embolization, umbilical artery ligation, or carunclectomy (Beede et al., 2019).
Placental stunting is the result of stress-induced alterations in maternal nutrient flux. Normally, robust repartitioning of maternal nutrients occurs to support the gravid uterus, which is facilitated in part by greater uterine blood flow (Thaler et al., 1990). Chronic stress reduces maternal nutrient repartitioning to the uterus by slowing uterine blood flow up to 50% in sheep models (Thureen et al., 1992; Lang et al., 2000; Wallace et al., 2008). Reduced uterine O2 delivery is particularly damaging, as a rodent model of maternal hypoxemia yielded placental and fetal IUGR despite a compensatory increase in uterine blood flow (Lane et al., 2020). Placental vasculature expands rapidly beginning about 0.3 of pregnancy, and stress-induced suppression of vasculogenesis during this critical window cannot be recovered later in pregnancy (Burton and Jauniaux, 2018). Underdeveloped placental villi and poor fetoplacental angiogenesis in IUGR pregnancies culminate in as much as two-fold reductions of placentome volume and maternofetal vascular interface (Mayhew et al., 2004; Edwards et al., 2020). Indeed, reduced peripheral capillary and villous surface areas are hallmarks of the IUGR placenta (Teasdale, 1984). Not surprisingly, two key angiogenic factors are dysregulated in IUGR placental tissues: vascular endothelial growth factor (VEGF) and placental growth factor (PlGF) (Regnault et al., 2002; Regnault et al., 2003). Diminished maternofetal interface slows the movement of O2, glucose, and other molecules that cross the placenta via simple or facilitated diffusion (Regnault et al., 2003). Studies in IUGR sheep indicate a 50%–70% disparity in maternofetal O2 gradients (Limesand et al., 2007; Beer et al., 2021). Nutrients that cross via facilitated diffusion or active transport are also slowed by reduced placental expression of transporters. For example, sheep pregnancies made IUGR by maternal overfeeding or heat stress had reduced placental glucose transport (Wallace et al., 2003; Brown et al., 2015) that coincided with less of the Glut1, Glut3, and Glut8 glucose transporters (Wallace et al., 2005). Placental transport of amino acids was also reduced in IUGR sheep pregnancies (Brown et al., 2012). In humans and rodents, this coincided with downregulation of the Na+-dependent neutral amino acid transporter A system, which moves alanine, serine, glutamine and glycine (Jansson et al., 2006; Shibata et al., 2008; Alkhalefah et al., 2021). Conversely, placental fatty acid transporters were downregulated in IUGR mice but increased in the placenta of nutrient-restricted baboons and in human pregnancies complicated by IUGR (Assumpcao et al., 2017; Xu et al., 2019; Chassen et al., 2020).
Stress-stunted placentas can typically fulfill the relatively modest fetal nutrient demands in early and mid-gestation, but exponential fetal growth during late gestation pushes O2 and nutrient requirements beyond the capacity of the stunted placenta (Limesand et al., 2013; Macko et al., 2013). As the fetus continues to grow, nutrient deficits progressively worsen. Natural sheep models for IUGR produce up to 50% reductions in fetal blood glucose near term (Saker et al., 1999; Limesand et al., 2007; Yates et al., 2016; Edwards et al., 2020). Endogenous hepatic glucose production is engaged in the IUGR fetus but only partially compensates for its hypoglycemia (Fowden and Silver, 1995; Limesand et al., 2007; Thorn et al., 2009; Thorn et al., 2013). In response to limited nutrient availability, the fetus engages its own nutrient repartitioning adaptations that prioritize vital nervous and endocrine tissues over others, particularly skeletal muscle (Poudel et al., 2015). Greater circulating lactate concentrations observed when IUGR fetal sheep were experimentally made hyperglycemic were consistent with a shift in muscle glucose metabolism from oxidative phosphorylation to anerobic glycolysis (Thorn et al., 2013; Lacey et al., 2021). Such shift coincides with and is perhaps necessitated by the hypoxemic state of the fetus (Regnault et al., 2003; Brown et al., 2015). However, less oxidative phosphorylation diminishes energy status by reducing production of ATP (Cree-Green et al., 2018). Despite this, IUGR fetuses exhibited greater circulating CO2 due to compromised placental gas transfer, which can affect acid-base balance (Macko et al., 2013; Lacey et al., 2021). Disruptions in lipid homeostasis included 10%–25% lower circulating cholesterol, which were reported in IUGR human and rodent fetuses at term despite markedly higher precursor concentrations and slower clearance rates (Cadaret et al., 2019a; Pecks et al., 2019). This indicates that deficits were due at least partially to impaired de novo cholesterol synthesis by the IUGR fetal liver rather than solely due to deficient placental transport. Conversely, ∼15% reductions in circulating triglycerides coincided with less placental fatty acid transporter expression and greater disparity in maternofetal triglyceride gradients in IUGR fetal models (Meyer et al., 2010; Cadaret et al., 2019a; Xu et al., 2019). Diminished placental amino acid transporter expression together with less effective Na+/K+-ATPase support is reflected in circulating amino acid profiles in IUGR fetuses (Stremming et al., 2020; Rosario et al., 2021). Brown et al. (2012) observed reduced placental transport of isoleucine, leucine, phenylalanine, tryptophan, methionine, and tyrosine, which notably lowered fetal circulating arginine and methionine and muscle protein synthesis patterns in IUGR fetal sheep. Interestingly, concentrations of some amino acids were actually increased in these fetuses, which was presumably a byproduct of reduced protein synthesis (Armstrong, 1973). Even electrolyte homeostasis can be disrupted by placental insufficiency, as fetal blood concentrations of Na+, K+, Ca++, and Cl− were increased in IUGR fetal sheep (Jansson et al., 2006; Bacchetta et al., 2009; Beer et al., 2021; Lacey et al., 2021).
Nutrient and O2 paucities stimulate fetal stress responses that include systemic inflammatory and adrenergic components (Yates et al., 2012a; Berbets et al., 2021). Hypoxemia, and to a lesser extent hypoglycemia, stimulate secretion of the catecholamines norepinephrine and epinephrine from the fetal adrenal medulla (Gardner et al., 2002; Ly et al., 2011; Yates et al., 2012a). Circulating norepinephrine (the primary fetal adrenal catecholamine) was elevated by as much as 8-fold in IUGR fetal sheep, and hypercatecholaminemia was among the earliest indicators of placental insufficiency (Macko et al., 2013; Chang et al., 2019a). Catecholamines are most associated with physiological mechanisms aimed at immediate survival, and sustained exposure of tissues can disrupt β adrenergic programming, the details and implications of which have been reviewed elsewhere (Yates et al., 2011; Posont et al., 2017; Gibbs and Yates, 2021). Perhaps the most consequential effect of fetal hypercatecholaminemia is suppressed insulin activity. Basal circulating insulin concentrations were often modestly decreased in IUGR fetal sheep, but glucose-stimulated insulin secretion was almost completely suppressed (Leos et al., 2010; Macko et al., 2013; Cadaret et al., 2019b). In contrast, maternal nutrient restriction-induced IUGR did not affect insulin concentrations (Edwards et al., 2020), which indicates that hypoxemia and hypercatecholaminemia are the suppressors of insulin secretion. Not surprisingly, circulating IGF-1 concentrations were likewise reduced in IUGR fetal sheep during mid- and late-gestation (Thorn et al., 2009; Macko et al., 2013; Rozance et al., 2018). As hypoxemia stimulates the adrenal medulla, hypoglycemic conditions stimulate the adrenal cortex to secrete cortisol, a steroid stress hormone associated with changes in intermediary metabolism (Thorn et al., 2013; Jonker et al., 2018). This has been documented across many IUGR models and among several species (Sutherland et al., 2012; Li et al., 2013; Thorn et al., 2013), and it may ultimately lead to reduced sensitivity of the cortisol axis (Iwata et al., 2019). Despite the anti-inflammatory effects of cortisol, IUGR fetuses exhibited elevated circulating inflammatory cytokines, including tumor necrosis factor α (TNFα), interleukin-1β (IL-1β), and IL-6 (Cadaret et al., 2019b; Zhang et al., 2021), and lower concentrations of anti-inflammatory IL-10 and IL-12 (Huang et al., 2019). Cytokines mediate inflammatory responses to pathogens, reactive oxygen species, and toxins, but they are also responsive to hypoxemia and other physiological stressors (Lacy and Stow, 2011; Hicks and Yates, 2021). They are released in greatest volume from circulating white blood cells and resident tissue macrophages and mast cells, and they act on tissues throughout the body (Tracey and Cerami, 1993; Lacy and Stow, 2011). Elevated inflammatory cytokines in IUGR fetuses can increase catabolism of skeletal muscle and affect mobilization of nutrient stores (Hicks and Yates, 2021). The concurrent appearance of systemic inflammation and hypercatecholaminemia in near-term IUGR fetuses is somewhat paradoxical, as macrophagic release of TNFα and IL-6 is suppressed by adrenergic stimulation under normal conditions (Donnelly et al., 2010; Papandreou et al., 2016). Nonetheless, the combined heightened inflammatory and adrenergic tones mediate many of the changes in metabolism and growth that IUGR fetuses exhibit (Macko et al., 2013; Hicks and Yates, 2021).
The bodyweights of IUGR fetal sheep and pigs were reduced by as much as 55% near term (Tree et al., 2016; Cadaret et al., 2019c; Tang and Xiong, 2022), and IUGR-born offspring remained lighter well into the neonatal period (Gibbs et al., 2020; Shoji et al., 2020). However, growth restriction is not equivalent among all fetal tissues. In fact, sustained nutrient insufficiency and the resulting fetal stress response yields hallmark asymmetric growth by disproportionally slowing muscle accretion relative to cranial and skeletal growth (Lapillonne et al., 1997). This was reflected in reduced muscle protein accretion rates, muscle morphometrics, and body length-to-mass ratios of IUGR fetal sheep (Yates et al., 2016; Rozance et al., 2018; Cadaret et al., 2019b). In human fetuses for whom adiposity is naturally high, disproportionally slower fat deposition was also apparent from ultrasound diagnosis of IUGR (Padoan et al., 2004; Ikenoue et al., 2021). The fetal hypoxemia-hypercatecholaminemia-hypoinsulinemia cascade appears instrumental in muscle growth restriction, as experimental induction of each factor individually produced some degree of IUGR in sheep (Philipps et al., 1991; Bassett and Hanson, 1998; Rozance et al., 2018). Hypoxemia-induced inflammation also limited muscle mass by increasing protein catabolism, reducing protein accretion, and altering muscle stem cell function (Li W. et al., 2009; Wang et al., 2014; Madaro et al., 2018; Posont et al., 2022). Less severe reductions in body length, head circumference, and cannon bone length observed in IUGR fetal lambs reflected the modest nature of structural growth restriction (Rozance et al., 2018; Cadaret et al., 2019c; Sandoval et al., 2020). As with bodyweight, however, these mild deficits in structural metrics persisted postnatal (Gibbs et al., 2020; Shoji et al., 2020). Ultimately, most IUGR-born offspring undergo a period of catch-up growth that diminishes or eliminates their weight disparity (Dulloo et al., 2006). However, this compensatory weight gain results from greater fat accumulation and does not reflect recovery of muscle mass (Ong et al., 2000; Gibbs et al., 2020). Consequently, IUGR-born adolescents and adults are more likely to develop high body mass indices (Fagerberg et al., 2004; Zinkhan et al., 2018). For humans, altered body composition was associated with a higher risk for metabolic and cardiovascular dysfunction later in life (Ong et al., 2000; Fagerberg et al., 2004). In food animals, IUGR-altered body composition resulted in smaller and less valuable carcasses (Greenwood et al., 2005; Greenwood and Bell, 2019).
Preferential reappropriation of fetal nutrients from muscle and other peripheral soft tissues to vital brain and endocrine tissues is reflected in blood flow patterns. In IUGR fetal sheep, blood flow was maintained or increased in every region of the brain, and pancreatic and adrenal blood flow increased by up to 2-fold (Poudel et al., 2015). Conversely, blood flow to the hindlimb, which is about 45% skeletal muscle (Hicks et al., 2021), was reduced by half (Poudel et al., 2015; Hicks et al., 2021). In a baboon model of IUGR, less hindlimb blood flow was associated with decreased size and distensibility of the external iliac and femoral arteries, which was sustained into adulthood (Kuo et al., 2018). In contrast, lower ultrasound-estimated resistance in the carotid artery of human IUGR fetuses reflected the brain-sparing characteristic of asymmetric growth restriction (Groenenberg et al., 1989). Preferential delivery of blood and nutrients maintained brain weights across sheep models for IUGR (Yates et al., 2016; Rozance et al., 2018; Wallace et al., 2020; Posont et al., 2021) even when heart, lung, and liver weights were reduced (Hyatt et al., 2007; Cadaret et al., 2019b; Wallace et al., 2020). Not surprisingly, weights of individual hindlimb muscles relative to fetal weight or hindlimb length were reduced in IUGR fetal sheep by up to 40% (Rozance et al., 2018; Lacey et al., 2021).
Impaired myoblast function and slower protein synthesis each contributed to reduced IUGR muscle mass (Yates et al., 2014; Soto et al., 2017; Rozance et al., 2018; Felicioni et al., 2020), which persisted after birth (De Blasio et al., 2007a; Gosby et al., 2009; Gibbs et al., 2020). Myoblasts are the myogenic stem cells that facilitate muscle fiber hypertrophy in late gestation and after birth when fiber numbers have become largely static (Maier et al., 1992; Wilson et al., 1992). Myoblasts undergo rate-limiting proliferation and differentiation steps before fusing with existing muscle fibers to increase the myonuclear content/protein capacity of the fiber (Allen et al., 1979; Yates et al., 2012b). Histological and ex vivo assessments indicated that differentiation capacity was impaired in myoblasts from IUGR fetal lambs (Yates et al., 2014; Yates et al., 2016; Soto et al., 2017; Posont et al., 2022). Myoblast proliferation was also typically reduced by IUGR intrauterine conditions, although proliferation was increased in one model induced by maternofetal inflammation (Soto et al., 2017; Cadaret et al., 2019a; Posont et al., 2022). Myoblast dysfunction is reflected in expression of key myogenic transcription factors, which hint at mechanisms for IUGR myoblast deficits. Specifically, differentiation factors myoD and myogenin were reduced in IUGR fetal myoblasts near term, whether assessed in vivo or ex vivo (Yates et al., 2014; Chang et al., 2019b; Rozance et al., 2021; Posont et al., 2022). Reduced myoblast proliferation, differentiation, and fusion slowed myonuclear accumulation in all muscle fiber types, which in IUGR fetal lambs restricted cross-sectional fiber area by as much as 60% (Yates et al., 2016; Chang et al., 2019b). Smaller muscle fibers have also been documented in IUGR piglets and rats (Cadaret et al., 2019a; Felicioni et al., 2020). Although some variation exists among IUGR models, species, and even specific muscles, disproportional reduction of muscle and other soft tissues as a means of sparing brain and structural growth is a well-conserved phenotype. Table 1 illustrates the distinct size reductions of specific tissues and organs that yield asymmetric body composition.
Limiting skeletal muscle growth contributes to fetal nutrient sparing necessitated by placental insufficiency (Brown, 2014). Additionally, the IUGR fetus alters tissue-specific utilization, metabolism, and storage of nutrients (Rozance and Wolfsdorf, 2019; Yates et al., 2019; Zhang et al., 2021). As with growth restriction, these changes disproportionally affect muscle. Several IUGR fetal sheep models found that muscle-specific glucose oxidation rates were reduced by up to 80% near term, which along with reduced hepatic oxidative metabolism produced a ∼50% reduction in whole-fetus glucose oxidation (Peterside et al., 2003; Limesand et al., 2007; Brown et al., 2015; Cadaret et al., 2019b). This deficit was observed under normal resting conditions and experimental hyperinsulinemia, and it persisted well after birth (Gibbs et al., 2021; Posont et al., 2021; Cadaret et al., 2022). Impaired glucose oxidation also occurred despite normal rates of glucose uptake by muscle, which has been observed before (Rozance et al., 2018; Cadaret et al., 2019c) and after birth (Gibbs et al., 2021; Posont et al., 2021; Cadaret et al., 2022). Although IUGR muscle expressed less of the insulin-dependent glucose transporter Glut4 in some studies (De Blasio et al., 2012; Duan et al., 2016; Yates et al., 2019; Jones et al., 2022), glucose uptake may have been rescued by an increase in the insulin-independent transporter Glut1 (Brown et al., 2015; Yates et al., 2019). Moreover, it should be noted that some studies found no changes in IUGR muscle content of Glut1 or Glut4 (Limesand et al., 2007; Garg et al., 2009). Not surprisingly, Glut1 expression in the brain was increased by over 60% in IUGR fetal lambs and rats as well as in IUGR-born neonatal rats (Sadiq et al., 1999; Limesand et al., 2007), which is consistent with brain sparing during chronic hypoglycemia (Gibbs and Yates, 2021).
Reduced skeletal muscle glucose oxidation rates coincided with lower proportions of slow oxidative (type I) fibers relative to intermediate (type IIa) and fast glycolytic (type IIx) fibers in hindlimb muscles of IUGR fetal sheep and loin muscles of IUGR fetal pigs (Wang et al., 2013; Yates et al., 2016; Stremming et al., 2022). Furthermore, IUGR muscle exhibited reduced activity of pyruvate dehydrogenase and citrate synthase enzymes that generate Krebs cycle intermediates, as well as increased expression of (inhibitory) pyruvate dehydrogenase kinase and impaired function of Electron Transport Chain Complex I (Brown et al., 2015; Pendleton et al., 2019; Stremming et al., 2022). These observations, combined with reduced O2 utilization, normal glucose utilization, and greater lactate dehydrogenase B content (Brown et al., 2015; Pendleton et al., 2019), indicated that much of the reduction in glucose oxidative phosphorylation was replaced by greater glycolytic lactate production. This is evident in elevated circulating lactate concentrations, which were as much as 3-fold greater in IUGR fetuses, particularly during experimental hyperglycemia or hyperinsulinemia (Limesand et al., 2007; Davis et al., 2021; Camacho et al., 2022). Lactate-O2 quotient was also 2-fold greater, meaning that more lactate was produced from each mole of O2 consumed (Rozance et al., 2018). Like other IUGR pathologies, hyperlactatemia appears to be driven primarily by hypoxemia-induced hypercatecholaminemia. In fetal sheep made experimentally hypoxemic (but not hypoglycemic) for 9 days, the 3-fold increase in circulating norepinephrine resulted in 20% less glucose oxidation, 3.5-fold greater circulating lactate, and 2-fold greater fetal lactate production (Jones et al., 2022). This model also demonstrated that hyperlactatemia may indicate condition severity, as circulating lactate concentrations were elevated by robust fetal hypercatecholaminemia (3-fold or greater increase) but not by a more modest 1.4-fold increase in norepinephrine (Jones et al., 2019; Rozance et al., 2021; Jones et al., 2022). Catecholamine-induced hyperlactatemia also persisted in IUGR-born neonates (Chen et al., 2010). Some studies have reported normal blood lactate in IUGR fetuses (Wai et al., 2018; Cadaret et al., 2019b; Pendleton et al., 2020), but this does not necessarily mean that lactate was produced at normal rates. Rather, IUGR fetuses were shown to engage hepatic gluconeogenesis that converts lactate and other substrates to glucose, a mechanism that is largely idle under normal intrauterine conditions (Limesand et al., 2007). This was reflected in elevated expression of gluconeogenic enzymes such as phosphoenolpyruvate carboxykinase and glucose 6-phosphatase and their transcriptional promoters in IUGR sheep, pigs, and rodents in late gestation and after birth (Peterside et al., 2003; Vuguin et al., 2004; Limesand et al., 2007; Thorn et al., 2009; Brown et al., 2015; Ying et al., 2017). Hepatic gluconeogenesis allows the IUGR fetus to utilize greater skeletal muscle lactate production to partially offset poor glucose supply via the Cori cycle (Soeters et al., 2021).
The IUGR fetus’s diminished protein supply affects utilization of amino acids for tissue accretion and for energy production. Foundational work by researchers at the University of Colorado School of Medicine found that hindlimb protein accretion was reduced by 55% in IUGR fetal sheep (Rozance et al., 2018; Wai et al., 2018). This coincided with a comparable reduction in total amino acid uptake rates by the hindlimb, although rates among individual amino acids were not affected uniformly (Rozance et al., 2018). For example, hindlimb uptake rates of the essential branched-chain amino acids leucine, valine, and isoleucine, were reduced by up to 73% in IUGR fetal sheep, whereas alanine, glutamine, and glycine were actually secreted from the hindlimb, despite slightly less protein breakdown (Chang et al., 2019a). These differential fluxes are presumably an attempt at metabolic thrift, as branched-chain amino acids can be converted into alanine, glutamine, and glycine, which are substrates for hepatic gluconeogenesis (Chang et al., 2019a). Indeed, expression of enzymes that facilitate this conversion was greater for IUGR fetal rats and sheep (Kloesz et al., 2001; Chang et al., 2019a). Interestingly, long-term infusion of essential amino acids into IUGR fetal sheep did not consistently increase protein accretion, indicating that amino acid utilization was restricted by more than just the short supply (Wai et al., 2018). Together, these studies show definitively that protein accretion in the IUGR fetus is impeded primarily by slower protein synthesis and not by greater protein breakdown. Like amino acid uptake, the impact of IUGR on individual amino acid oxidation rates is not uniform. For example, IUGR fetal sheep oxidized 47% less threonine (Anderson et al., 1997) but 2-fold more lysine (Limesand et al., 2009). Leucine oxidation rates were reduced by 34% in one early study of IUGR fetal sheep (Ross et al., 1996) but were normal in others (Rozance et al., 2018; Wai et al., 2018). Moreover, leucine oxidation was not affected in fetal sheep made chronically hypoxemic (Rozance et al., 2021), hypoglycemic (Carver et al., 1997), hyperglucagonemic (Cilvik et al., 2021), or hyperinsulinemic (Brown et al., 2016) late in gestation, but it was markedly reduced by elevated circulating IGF-1 (Stremming et al., 2021). A greater amount of leucine was oxidized when normal fetuses were experimentally administered excess amino acids (Rozance et al., 2009; Maliszewski et al., 2012), but this effect was blunted in IUGR fetuses (Brown et al., 2012). Amino acids and glucose are normally competitive oxidative substrates (Brown et al., 2017). However, the apparent lack of compensatory amino acid oxidation in IUGR fetuses despite substantial deficits in glucose oxidation may have been the result of mitochondrial deficits. Indeed, IUGR fetal skeletal muscle had less citrate synthase, which is an indicator of intact and functional mitochondria (Stremming et al., 2022). It also expressed less BCAT1 and BCAT2, the transaminase enzymes that convert leucine and isoleucine into the Krebs cycle intermediate succinate, and less ALT, the enzyme that converts alanine to pyruvate (Chang et al., 2019a). The coincident deficits in glucose and amino acid oxidation ultimately diminished ATP content in IUGR fetal muscle (Stremming et al., 2020).
The impact of IUGR on lipid homeostasis is perhaps less clear. Elevated triglycerides and non-esterified (or free) fatty acids (NEFA) in cord blood at birth is a hallmark of IUGR in humans (Youssef et al., 2021; Chassen et al., 2022) and has been used for decades as a clinical indicator of fetal stress (Elphick et al., 1978). High blood lipid concentrations in late gestation and after birth have also been documented in piglets with spontaneous IUGR (i.e., litter runts) (Li et al., 2018) and in several IUGR sheep models (Wallace et al., 2014; Wallace et al., 2020; Posont et al., 2021; Zhang et al., 2021), although we are aware of at least one study that found reduced circulating NEFA in young IUGR-born lambs (Duffield et al., 2009). The impact of IUGR on circulating cholesterol is similarly inconsistent and may depend in part upon the lipoprotein to which cholesterol is bound. For example, Youssef et al. (2021) observed 23% greater total cholesterol but 20% less HDL-C in cord blood from IUGR babies, whereas Pecks et al. (2012) reported reductions in both total cholesterol and HDL-C. Circulating cholesterol was elevated in several IUGR fetal sheep studies and correlated tightly with fetal weights (Meyer et al., 2010; Zywicki et al., 2016; Sun et al., 2018; Zhang et al., 2021). As neonates, however, IUGR-born lambs had normal or even reduced circulating cholesterol, although HDL-C was still elevated (Wallace et al., 2014; Posont et al., 2021). Changes in intracellular lipids within IUGR tissues may also differ between prenatal and postnatal stages. In fetal sheep, for example, IUGR due to nutrient restriction or fetal crowding increased blood NEFA and triglycerides by as much as 50% but reduced liver triglycerides and hepatic lipase, the enzyme responsible for their hydrolysis (Meyer et al., 2010; Zywicki et al., 2016; Sun et al., 2018; Zhang et al., 2021). In IUGR fetal guinea pigs, hepatic utilization of palmitate (the most common saturated fatty acid in animals and plants) was reduced by about 50% (Detmer et al., 1992). Conversely, IUGR-born neonatal goats had greater liver accumulation of NEFA, triglycerides, and cholesterol (Liu et al., 2022), and IUGR-born runt piglets had more expansive hepatic lipid droplets, particularly after undergoing catch-up growth (Wang et al., 2022). IUGR-induced changes in lipid metabolism were largely tissue-specific, as were changes in de novo synthesis. Shortly after birth, IUGR infants exhibited greater rates of whole-body triglyceride mobilization and fatty acid oxidation (Patel and Kalhan, 1992). Moreover, gene expression for the key β-oxidation facilitator PPARα was increased in liver tissue from IUGR-born runt piglets (Wang et al., 2022) and in cardiac muscle from IUGR-born adult guinea pigs (Botting et al., 2018). Gene expression for CPT1 and HADHA, which play rate-limiting roles for fatty acid β-oxidation, was reduced in liver tissues of IUGR-born rats (Lane et al., 2001a). However, skeletal muscle from these rats had greater CPT1 and HADHA expression along with greater triglyceride content (Lane et al., 2001b). Cardiac muscle from IUGR fetal sheep also expressed less mRNA for CPT1 and for enzymes associated with β-oxidation and esterification of fatty acids (Drake et al., 2022). This coincided with greater circulating acylcarnitines, which are indicative of impaired or incomplete fatty acid oxidation (Drake et al., 2022). Fatty acid synthesis rates were reduced in liver and lung tissues of IUGR rat fetuses, but not in brain tissue (Vileisis et al., 1982). Interestingly, maternofetal O2 supplementation did not recover fatty acid synthesis in these fetuses, which indicates that the deficit was not a product of hypoxemia (Vileisis, 1985). As adults, fatty acid synthesis was normal in liver and muscle from IUGR-born rats but was elevated in adipose tissues (Yee et al., 2016). Disruptions in lipid homeostasis almost certainly contribute to greater adiposity in IUGR-born offspring (Ong et al., 2000; Zinkhan et al., 2018). Indeed, IUGR-born lambs exhibited markedly greater insulin sensitivity for fat deposition (De Blasio et al., 2007b). They also produced muted increases in circulating NEFA in response to epinephrine challenge, indicating a reduced capacity for lipid mobilization (Harwell et al., 1990; Chen et al., 2010).
Deficient insulin production and secretion is a key contributor to poor growth and metabolic dysfunction in IUGR fetuses and offspring (Limesand et al., 2006; Xing et al., 2019). Large reductions in basal circulating insulin and complete inhibition of nutrient-stimulated insulin secretion observed in IUGR fetal sheep (Limesand et al., 2007; Cadaret et al., 2019b) were the direct result of fetal hypoxemia and hypoglycemia. To illustrate, when normoxemia and euglycemia were experimentally restored for a 5-day period in IUGR fetal sheep, basal insulin concentrations normalized and glucose-stimulated insulin secretion was rescued (Camacho et al., 2022). Conversely, acute or chronic experimental hypoxemia or hypoglycemia in otherwise uncompromised fetal sheep reduced insulin secretion (Rozance et al., 2006; Yates et al., 2012a; Benjamin et al., 2017). Basal hypoinsulinemia was also resolved postnatal, as IUGR-born offspring were no longer hypoxemic or hypoglycemic after birth (Posont et al., 2021; Cadaret et al., 2022). The suppressive effects of these conditions, particularly hypoxemia, on pancreatic β cell function are mediated in large part by adrenergic signaling. In fact, blocking the adrenergic response to IUGR conditions via pharmaceutical antagonists or adrenal demedullation not only rescued insulin secretion, but in most cases revealed a compensatory enhancement in β cell stimulus-secretion coupling (Leos et al., 2010; Yates et al., 2012a; Macko et al., 2013; Macko et al., 2016). Comparable enhancements in β cell function were observed following chronic norepinephrine infusion into otherwise normal fetal sheep or rats (Chen et al., 2014; Chen et al., 2017; Li et al., 2021). Despite greater sensitivity of β cells, IUGR pancreatic islets are poorly developed. Specific islet deficits are reviewed in detail elsewhere (Boehmer et al., 2017) but include smaller size with fewer β cells/islet, less production and sensitivity to growth factors, and poor vascularity (Limesand et al., 2005; Styrud et al., 2005; Limesand et al., 2006; Ham et al., 2009; Rozance et al., 2015).
Systemic inflammation has been observed in IUGR fetuses of several species. Although the role of inflammation in IUGR pathologies are not fully characterized, overexposure to inflammatory cytokines is known to disrupt progenitor cell function, tissue growth, and metabolism, as detailed in previous reviews (Joanisse and Parise, 2016; Hicks and Yates, 2021; Most and Yates, 2021). Inflammatory cytokines such as TNFα, IL-1β, and IL-6 were increased in blood and liver of IUGR fetal lambs and mice (Hudalla et al., 2018; Cadaret et al., 2019b; Zhang et al., 2021) and in cord blood of IUGR infants (Amarilyo et al., 2011). In humans, piglets, and rats, circulating inflammatory cytokines remained elevated for the first few hours, days, or even weeks after birth (Krajewski et al., 2014; Riddle et al., 2014; Chisaka et al., 2015; Huang et al., 2019). Eventually, however, high circulating cytokine concentrations subsided and in many cases even fell below normal; plasma TNFα was reduced by 50% in IUGR-born neonatal lambs (Posont et al., 2021), and IFNγ, IL-1β, IL-4, and IL-8 concentrations were reduced in young IUGR-born piglets (Zhong et al., 2012). Resting blood TNFα, IL-6, and IL-1β concentrations were normal in IUGR-born adult rats but were 25%–40% less elevated in response to immune challenge (Desai et al., 2009; Rueda-Clausen et al., 2011). We should note that at least one study of IUGR-born adult rats observed modestly greater circulating inflammatory cytokines (He et al., 2018), although the reason for this unusual finding was not clear. Moreover, when these researchers produced IUGR piglets using the same model of maternal nutrient restriction, they found postnatal circulating cytokine concentrations that were indeed below normal (Dong et al., 2015).
Postnatal reductions in circulating inflammatory cytokines are likely a compensatory response to heightened inflammatory sensitivity that develops in several IUGR fetal tissues and persists after birth (Hicks and Yates, 2021). In a landmark example from humans, the soluble form of the TNFα receptor, TNFR1, was observed to be 2.5-fold greater in the umbilical cord of IUGR newborn infants (Laskowska et al., 2007). Enhanced inflammatory sensitivity is also apparent in IUGR skeletal muscle, as hindlimb muscles from IUGR fetal sheep and rats exhibited greater gene expression for TNFR1, the IL-6 receptor (IL6R), and even the TWEAK receptor (Fn14) near term (Cadaret et al., 2019a; Posont et al., 2022). Muscle from these IUGR fetal sheep also contained less of the NFκB arrest protein, IκBα (Posont et al., 2022). As neonates, IUGR-born lambs continued to exhibit greater skeletal muscle TNFR1 content, although IκBα content was increased, perhaps in compensation (Posont et al., 2021). Adaptive enrichment of inflammatory signaling components in the muscle of IUGR-born offspring may arise in part from intrauterine programming of muscle progenitors, which are established prenatal but are incorporated into muscle over the entire lifespan (Allen et al., 1979). Indeed, myoblasts isolated from IUGR fetal sheep had greater gene expression for TNFR1, IL6R, and TLR4 and also had more c-Fos, a cytokine-responsive protein that can disrupt cellular differentiation (Posont et al., 2022). In culture, these IUGR fetal myoblasts exhibited greater phosphorylation of NFκB when stimulated with TNFα and were more sensitive to inhibitors of the canonical signaling enzyme, IκB kinase, under basal conditions (Posont et al., 2022). The impact of enhanced inflammatory sensitivity in skeletal muscle on growth and metabolic homeostasis is substantial, as the tissue comprises about 60% of total juvenile body mass and expands substantially between fetal and juvenile stages (Calnan et al., 2021; Hicks et al., 2021). As illustrated in Figure 1, however, it is not the only tissue to develop this phenotype. Fat tissues of IUGR-born juvenile rats exhibited greater gene and protein expression for TNFα and TNFR1 (Riddle et al., 2014), and transcriptome analyses of pancreatic islets from IUGR fetal sheep indicated enriched TNFα signaling pathways (Kelly et al., 2017). Liver content of TNFα, IL-6, IL-1, TLR4, MyD88, phosphorylated and total NFκB, phosphorylated IκBα, and phosphorylated IκB kinase were elevated in IUGR fetal sheep and newborn mice, although the latter also exhibited tempered hepatic TNFα production in response to acute LPS-stimulation (Zarate et al., 2021; Zhang et al., 2021). Greater hepatic TNFα, IL-6, and TLR4 were also observed in IUGR-born pigs at weaning and in IUGR-born rats in adulthood, which corresponded to greater percentages of phosphorylated IκBα and NFκB (Liu et al., 2014; Tarry-Adkins et al., 2016; He et al., 2018). Intestinal tissues of newborn IUGR piglets exhibited a paradoxical reduction of IFNγ but had increased IL-4 and the inflammation-mediating transcription factor FOXO3a (Zhong et al., 2012). Intestinal tissues from these piglets also exhibited less of the anti-inflammatory cytokine, IL-10 (Zhong et al., 2012). By weaning, intestinal gene and protein expression for cytokines and their canonical pathways were robustly enhanced, which corresponded to greater phospho-activation and nuclear translocation of NFκB and greater activation of the inflammation-mediating kinase, JNK (Niu et al., 2021; Cui et al., 2022). Lung tissue from IUGR-born rats likewise exhibited greater gene expression for inflammatory cytokines and reduced expression for the anti-inflammatory IL-10, which corresponded to greater phospho-activation of the inflammatory mediator, STAT3 (Alejandre Alcazar et al., 2012). Even skin tissues of IUGR-born rats exhibited evidence of enhanced inflammation, as indicated by greater macrophage infiltration and increased gene expression for the interleukin receptor, IL7R, and its cofactor, cytokine receptor-like factor 2 (Polányi et al., 2020). One noteworthy exception for this phenotype appears to be white blood cells, as IUGR-born piglets and lambs had little or no change in circulating leukocyte profiles (Zhong et al., 2012; Amdi et al., 2020; Posont et al., 2021; Cadaret et al., 2022). Moreover, IL-1β production and proliferation by IUGR leukocytes was less responsive to LPS stimulation (Zhong et al., 2012; Amdi et al., 2020).
FIGURE 1. Stress-induced programming of enriched inflammatory signaling pathways in IUGR sheep tissues. Findings compiled from the literature are shown for skeletal muscle (Llovera et al., 1997; Bodell et al., 2009; Gray et al., 2009; Cadaret et al., 2017; Cadaret et al., 2019c; Hicks and Yates, 2021; Posont et al., 2022), liver (Fitzgerald et al., 2003; Zarate et al., 2021; Zhang et al., 2021; Wang et al., 2022), pancreas (Hadjivassiliou et al., 1998; Andersson et al., 2005; Ellingsgaard et al., 2011; Kelly et al., 2017), and adipose tissue (Kras et al., 2000; Sharkey et al., 2009; Beer, 2022). Green boxes indicate signaling components reported to be upregulated and red boxes indicate downregulated components.
Inflammation and oxidative stress are inherently-linked stress conditions. The major reactive oxygen species that mediate oxidative stress are listed in Figure 2. These are physiological byproducts that serve important roles in cellular communication, but excessive accumulation causes cellular damage and increases risk for chronic inflammatory diseases (Pizzino et al., 2017). Reactive oxygen species stimulate greater cytokine secretion from many cell types (Bulua et al., 2011; Enoki et al., 2016). They also directly stimulate inflammatory pathways by activating IκB kinase, stimulating IκB/NFκB dissociation, and facilitating greater nuclear NFκB dimerization (Lugrin et al., 2014). Coincidentally, inflammatory stimulation often increases production of reactive oxygen species (Floyd et al., 1999; Reid and Li, 2001; Langen et al., 2002; Sriram et al., 2011). For example, skeletal muscle of IUGR-born juvenile rats overexpressed components of NADPH oxidase 2, a membrane-bound enzyme that produces reactive oxygen species for immune signaling but is also a prominent source for overproduction (Chisaka et al., 2015; Tarry-Adkins et al., 2016). The short-lived nature of reactive oxygen species makes them difficult to measure in situ, but greater hepatic reduction of oxidized glutathione was indicative of severe oxidative stress in IUGR-born neonatal pigs (Niu et al., 2019). Hepatic concentrations of malondialdehyde and protein carbonyl, the primary indicators of lipid peroxidation and oxidative protein damage, respectively, were also increased in IUGR-born adult rats and neonatal pigs (He et al., 2018; Niu et al., 2019). Tissues of IUGR-born offspring are more susceptible to reactive oxygen species due to maladaptive reductions in several intracellular antioxidant compounds. Hepatic concentrations of the antioxidant enzyme glutathione reductase, which clears oxidized glutathione, was reduced in IUGR-born pigs (Niu et al., 2019). Glutathione reductase was one of several antioxidant proteins for which hepatic gene expression was downregulated in IUGR-born neonatal piglets and rats (Tarry-Adkins et al., 2016; Niu et al., 2019), which culminated in an almost 50% reduction in total antioxidant capacity of the liver (He et al., 2018; Niu et al., 2019).
FIGURE 2. Common stress-induced reactive oxygen species. These are natural cellular byproducts that can be damaging when overproduced during inflammation or other stress conditions (Turrens, 2003; Collin, 2019; Juan et al., 2021).
Nutraceutical use of two natural bioactive ω-3 PUFA, eicosapentaenoic acid (EPA; 20:5 ω-3) and docosahexaenoic acid (DHA; 22:6 ω-3), has increased in popularity as their anti-inflammatory and antioxidant effects have been more firmly established (Shahidi and Ambigaipalan, 2018). Although EPA and DHA can be synthesized de novo from α-linolenic acid in modest amounts, additional dietary sources result in health benefits (Russell and Burgin-Maunder, 2012). Many ocean fish and algae species are particularly rich sources of exogenous ω-3 PUFA and are used to produce commercial fish oil-extract supplements (Shahidi and Ambigaipalan, 2018). Consumer interest in ω-3 PUFA has led to the marketing of food products such as milk and bacon that are enriched with exogenous EPA and DHA (Bernal-Santos et al., 2010; Meadus et al., 2010).
Studies performed in vivo and in vitro report notable anti-inflammatory and antioxidant effects of ω-3 PUFA. Incubation of cultured macrophages with either EPA or DHA for only 48 h mitigated the normally robust LPS-induced secretion of TNFα, IL-1β, and IL-6 by up to 90% (Weldon et al., 2007). This effect was facilitated by stimulation of the GPR120 receptor pathway, which suppresses NFκB binding to DNA and thus inhibits transcription of additional inflammatory factors, including cytokines and toll-like receptors (Weldon et al., 2007; Oh et al., 2010; Block et al., 2012). Even in generally healthy individuals, daily EPA and DHA supplementation reduced circulating IL-6 and TNF-α concentrations by about 15% (Kiecolt-Glaser et al., 2011; Kiecolt-Glaser et al., 2012). In mice genetically engineered to overproduce EPA and DHA, endotoxin-stimulated blood TNFα concentrations were 5-fold less than in normal mice, and hepatic gene expression was reduced for several inflammatory cytokines (Schmocker et al., 2007). Rats supplemented ω-3 PUFA via daily inhalation likewise had less severe elevation of circulating inflammatory cytokines in response to endotoxin (Kocherlakota et al., 2022). Biomedical studies showed that ω-3 PUFA were particularly effective for individuals with chronic inflammatory conditions, including type 2 diabetes, cancers, and cardiovascular diseases (Natto et al., 2019; Xiao et al., 2022; da Silva et al., 2016; Koppelmann et al., 2021). Moreover, DHA and EPA were shown to downregulate production of reactive oxygen species and were effective scavengers of superoxide anions (da Silva et al., 2016; Richard et al., 2008). Indeed, supplementation of ω-3 PUFAs mitigated both systemic inflammation and excessive production of reactive oxygen species in acute and chronic disease states (Li et al., 2017; Alorabi et al., 2022; Gortan Cappellari et al., 2022; Khan et al., 2022). Although few studies have investigated ω-3 PUFA supplementation in IUGR outcomes, extensive literature documenting their antioxidant and anti-inflammatory functions indicate their potential for ameliorating IUGR pathologies.
Inflammatory cytokines are dynamic regulators of myoblast function and thus play a complex role in postnatal muscle growth (Bodell et al., 2009; Alvarez et al., 2020). In vitro studies found that proliferation rates increased when myoblasts were incubated for short periods with low or moderate concentrations of TNFα, IL-1β, or IL-6 (Otis et al., 2014; Alvarez et al., 2020). For TNFα and IL-6 incubations, greater proliferation was coincident with impaired differentiation (Langen et al., 2001; Alvarez et al., 2020; Posont et al., 2022). Indeed, exposure to either cytokine substantially reduced the early differentiation transcription factor, myoD, and modestly reduced the later differentiation factor, myogenin (Alvarez et al., 2020). However, nuclear expression of the myoblast-specific proliferation factor, pax7, was also reduced by these incubations (Alvarez et al., 2020). Furthermore, high physiological concentrations of IL-6 actually diminished myoblast proliferation, and incubation for longer periods of time dampened the inhibitory effect on differentiation (Steyn et al., 2019). These idiosyncratic outcomes were associated with the respective up or downregulation of IL6R by moderate or substantial IL-6 exposure (Steyn et al., 2019). Moreover, IL6R inhibition diminished not only the effects of IL-6 but those of TNFα and IL-1β as well (Alvarez et al., 2020). Nevertheless, all cytokine effects were lost when myoblasts were incubated with NFκB inhibitors (Otis et al., 2014). Disruption of the balance between myoblast proliferation and differentiation clearly reduces the capacity for muscle hypertrophy. To illustrate, intramuscular IL-6 infusion in young rats decreased muscle growth by about 10% over 2 weeks (Bodell et al., 2009). This coincided with greater gene expression for cytokine signaling components, including TNFα, TNFR1, and atrogin-1 (Haddad et al., 2005; Bodell et al., 2009). In TNFα-infused mice, an observed increase in muscle catabolism was attributed to greater ubiquitin-dependent proteolysis (Llovera et al., 1997). The combination of greater relative muscle catabolism and decreased myoblast-facilitated muscle hypertrophy associated with experimentally-elevated cytokine exposure was comparable to the phenotype observed in the IUGR fetus (Yates et al., 2014; Soto et al., 2017; Rozance et al., 2018; Posont et al., 2022).
Like inflammation, oxidative stress impairs skeletal muscle hypertrophy in similar but independent fashion (Jang et al., 2020). Addition of even modest concentrations of reactive oxygen species to myoblast incubations increased proliferation (Sciancalepore et al., 2012) and inhibited differentiation (Langen et al., 2002; Sandiford et al., 2014), regardless of the presence of inflammatory cytokines. When primary mouse myoblasts were engineered to overproduce H2O2, only about half as many stained positive for myogenin, expressed myosin heavy chain protein, or fused with other myoblasts (Sandiford et al., 2014). Furthermore, accumulation of reactive oxygen species both directly and indirectly resulted in muscle catabolism (Jang et al., 2020). In addition to directly damaging cellular proteins, elevated reactive oxygen species in antioxidant-deficient mice upregulated cysteine proteases, which increased protein breakdown by the ubiquitin-proteasome and autophagy-lysosome systems (Muller et al., 2006; Jang et al., 2020). These mice were characterized by a reduction in muscle mass of up to 50%, along with poor exercise performance (Muller et al., 2006).
Sustained exposure to elevated inflammatory cytokines or reactive oxygen species dysregulates skeletal muscle glucose metabolism and impairs insulin signaling and secretion. Brief incubations with high physiological IL-6 concentrations increased glucose uptake, oxidation, and incorporation into glycogen and also reduced lactate production in primary human and rodent muscle, as well as in culture-derived myotubes (Al-Khalili et al., 2006; Glund et al., 2007; Gray et al., 2009; Cadaret et al., 2017). Glucose uptake was stimulated at much lower IL-6 concentrations when its receptor was added to the incubation in concert (Gray et al., 2009). In primary rat muscle and myotubes, TNFα similarly elevated glucose uptake and oxidation (Yamasaki et al., 1996; Cadaret et al., 2017). Moreover, greater glucose oxidation was observed in vivo when healthy young adults were infused with moderate amounts of IL-6 over several hours (Carey et al., 2006). Some of these studies indicated that cytokine regulation of muscle glucose utilization was independent of insulin and its major pathway components, IRS1 and Akt (Yamasaki et al., 1996; Glund et al., 2007; Gray et al., 2009; Cadaret et al., 2017). Rather, cytokines appeared to stimulate translocation of sequestered Glut4 glucose transporters to the membrane by activating the kinase enzyme, AMPK (Al-Khalili et al., 2006; Carey et al., 2006). In fact, longer periods of cytokine exposure generally disrupted insulin signaling (Rotter et al., 2003; Al-Khalili et al., 2006; Cadaret et al., 2017), although the direct effects on glucose utilization remained. This was demonstrated particularly well by in vitro incubation of myoblast cell lines with TNFα, which increased basal glucose uptake by almost 3-fold but reduced insulin-stimulated glucose uptake by about 75% (Yamasaki et al., 1996; Grzelkowska-Kowalczyk and Wieteska-Skrzeczynska, 2006; Remels et al., 2015). Infusion of TNFα into mice resulted in a 50% reduction of insulin-stimulated hindlimb glucose uptake but did not affect resting glucose uptake rates (Youd et al., 2000). The primary target of cytokines within the insulin signaling pathway appears to be the phospho-activation of Akt, a hub that facilitates myriad insulin effects (Ji et al., 2022). Incubation of primary rat muscle with moderate concentrations of TNFα or IL-6 for as little as 2 h reduced insulin-stimulated Akt phosphorylation by more than 50% (Cadaret et al., 2017). Similar deficits were observed in culture-derived myotubes after 6-day incubation with TNFα (Grzelkowska-Kowalczyk and Wieteska-Skrzeczynska, 2006) and in primary bovine adipocytes after 12 h (Du et al., 2022). In addition to disrupting insulin signaling, cytokines also impair insulin stimulus-secretion coupling in pancreatic islets, although this effect is quite dependent upon duration of exposure. In fact, when mice were injected with IL-6, glucose-stimulated insulin secretion was increased over the first 45 min, but this effect was gone by 1 h (Ellingsgaard et al., 2011). Incubation of primary mouse islets with IL-1β alone or in combination with IFNγ increased glucose-stimulated insulin secretion for the first few hours but reduced it after 20 h (Andersson et al., 2005). Likewise, 48-h incubation with TNFα alone or together with IL-1β and IFNγ had no effect on basal insulin secretion but completely inhibited glucose-stimulated insulin secretion in primary rat islets and in INS-1 rat β cells (Hadjivassiliou et al., 1998; Zhang et al., 2022). This combination of cytokines also reduced insulin content in primary human islets after 48 h (Hadjivassiliou et al., 1998). The 40% reduction in glucose-stimulated insulin secretion and 25% reduction in pyruvate-stimulated insulin secretion observed in INS-1 rat β cells after 24 h with IL-1β and IFNγ coincided with reduced indicators of oxidative metabolism, the facilitating mechanism for β cell stimulus-secretion coupling (Rozance et al., 2007; Barlow et al., 2018).
Like cytokines, in vitro exposure of primary rat muscle to reactive oxygen species for less than 1 h increased glucose uptake by up to 48% and glycogen synthase activity by about 20% by enhancing canonical insulin signaling (Kim et al., 2006; Higaki et al., 2008). In fact, concurrent exposure to insulin and reactive oxygen species for 20 min had an additive effect on glucose uptake (Higaki et al., 2008). Short-term oxidative stress reflects the normal microenvironment of muscle and is not particularly harmful, but sustained exposure becomes deleterious (Higaki et al., 2008; Diamond-Stanic et al., 2011). Incubation of rat muscle with high concentrations of reactive oxygen species ceased stimulating basal glucose uptake after 6 h (Diamond-Stanic et al., 2011). Insulin-stimulated glucose uptake and Akt phosphorylation were hindered by reactive oxygen species after only 2 h (Diamond-Stanic et al., 2011). The adverse effects of longer exposure on insulin signaling components were mediated in part by p38 MAPK and were evident even at modest reactive oxygen species concentrations (Archuleta et al., 2009; Diamond-Stanic et al., 2011). Interestingly, reactive oxygen species did not appear to affect function of primary rat or human islets after 48 h of incubation (Hadjivassiliou et al., 1998).
Inflammatory cytokines help to regulate lipid homeostasis and metabolism by skeletal muscle in coordination with their effects on muscle glucose utilization. Infusion of IL-6 into healthy individuals for 3 hours increased total fatty acid oxidation rates during and for several hours after the infusion period (van Hall et al., 2003). In primary rat muscle, 1-h incubations with high IL-6 concentrations increased palmitate oxidation but not deposition, whereas 1-h incubation with TNFα increased deposition but not oxidation (Bruce and Dyck, 2004). Conversely, 3-h incubation of culture-derived human myotubes with IL-6 increased palmitate uptake and oxidation rates (Al-Khalili et al., 2006). Greater oxidation occurred in the presence and absence of insulin and was mediated primarily by AMPK pathways (Bruce and Dyck, 2004; Al-Khalili et al., 2006). To facilitate greater lipid utilization by muscle, cytokines concurrently mobilize lipid deposits from adipose and other tissues. This was demonstrated by infusions of moderate or high concentrations of IL-6 into humans, both of which elevated circulating NEFA and triglycerides (van Hall et al., 2003). Moreover, blocking IL-6 signaling by administering a long-lasting IL6R antagonist reduced indicators of fatty acid mobilization in adult men during various degrees of physical activity and across a range of body mass indices (Trinh et al., 2021). In primary rat muscle, IL-6 stimulated lipid mobilization in part by disrupting insulin’s lipogenic effects (Bruce and Dyck, 2004). Unlike IL-6, TNFα had no impact on endogenous or exogenous skeletal muscle fatty acid oxidation (Bruce and Dyck, 2004). However, several in vitro studies have shown that even modestly elevated TNFα concentrations stimulated lipolysis (Ryden et al., 2004; Lee and Fried, 2012; Du et al., 2022). This was recapitulated by 7-day TNFα infusion into mice, which elevated their circulating triglycerides and NEFA concentrations (Li L. et al., 2009). Moreover, both glycerol and NEFA were released from adipocytes at greater rates when stimulated with TNFα in vitro (Green et al., 2004; Ryden et al., 2004). TNFα-mediated lipolysis was facilitated in large part by downregulation of perilipin, a protein that mediates hormone-sensitive lipase activity in lipid droplets (Morin et al., 1995; Wu et al., 2004). Studies in rats and cultured adipocytes found that TNFα also reduced activity and expression of lipoprotein lipase, an enzyme that breaks down circulating lipoproteins for deposition into adipocytes (Morin et al., 1995; Wu et al., 2004). The increased production of reactive oxygen species in cytokine-stimulated adipocytes further contributed to lipolysis (García et al., 2021), an effect that was dampened by concurrent inhibition of superoxide production in culture (Krawczyk et al., 2012; Issa et al., 2018). The impact of reactive oxygen species on adipocyte lipolysis coincided with phospho-activation of hormone sensitive lipase (Krawczyk et al., 2012; Issa et al., 2018).
Benefits of ω-3 PUFA supplementation during critical windows for fetal development on growth and metabolic outcomes have been documented in humans and animals. Meta-analyses of global health records and clinical trials indicated that dietary ω-3 PUFA supplementation over the 2nd half of gestation was associated with reductions of up to 73% in perinatal mortality rates as well as fewer neonatal intensive care stays (Saccone et al., 2015; Saccone et al., 2016; Ali et al., 2017; Middleton et al., 2018). The supplements were also associated with lower incidence of IUGR in many world populations (Cetin et al., 2002; Agostoni et al., 2005; Fares et al., 2015; Saccone et al., 2015; Gholami et al., 2020). In one prime example, DHA supplementation in primigravidae women in Mexico reduced IUGR rates by half (Ramakrishnan et al., 2010). Better pregnancy outcomes coincided with positive effects on uteroplacental tissues, as clinical trials found that ω-3 PUFA supplements increased uterine and umbilical blood flow and reduced placental apoptosis (Wietrak et al., 2015; Ali et al., 2017). Moreover, dietary ω-3 PUFA supplementation in pregnant rats reduced indicators of placental oxidative stress and increased placental and fetal mass (Jones et al., 2013). Even direct infusion of EPA into the bloodstream of IUGR fetal sheep benefitted placental function, as maternofetal glucose and O2 gradients were improved (Beer et al., 2021). Importantly, maternal ω-3 PUFA supplements directly benefit the fetus as well as the placenta. Clinical trials found that IUGR fetuses and newborns were deficient in ω-3 PUFA due to impaired de novo production and that maternal supplementation was effective in increasing circulating concentrations in the fetus/newborn (Cetin et al., 2002; Agostoni et al., 2005; Llanos et al., 2005; Fares et al., 2015; Wietrak et al., 2015). These findings were recapitulated in rats, where IUGR-born neonates were found to be DHA-deficient, but maternal supplementation increased circulating DHA concentrations in these offspring by 3.25-fold (Morand et al., 1981; Joss-Moore et al., 2010). In pigs, maternal ω-3 PUFA supplementation increased DHA and EPA and reduced ω-6:ω-3 PUFA ratios in fetal blood and skeletal muscle near term, which coincided with greater blood glucose, less cholesterol, and a reduction in the incidence of IUGR from 22% to 14% (Heras-Molina et al., 2021; Heras-Molina et al., 2022). These benefits persisted after birth, as weaning-aged pigs born to ω-3 PUFA-supplemented sows had less circulating total cholesterol, HDL-C, and LDL-C as well as greater total ω-3 PUFA content and lower ω-6:ω-3 PUFA ratios in adipose, muscle, and liver tissues (Heras-Molina et al., 2020). When pregnant rodents carrying IUGR pregnancies were supplemented DHA, birthweight and neonatal growth of their offspring were improved without increased adiposity (Bagley et al., 2013; Velten et al., 2014). Offspring from dams supplemented DHA or ω-3 PUFA-rich fish oil had greater circulating adiponectin, smaller adipocytes, and adipose tissue that expressed more PPARγ, adiponectin, and adiponectin receptors R1 and R2 (Bringhenti et al., 2011; Bagley et al., 2013; Ghnaimawi et al., 2022). Supplementing pregnant cows with ω-3 PUFA (delivered as Ca2+ salts for ruminal protection) during late gestation increased circulating DHA in the dam by 5-fold, and calves born to supplemented cows exhibited 6%–10% better average daily gain in the feedlot (Marques et al., 2017). At harvest, these calves produced marginally larger carcasses and loin muscles with ∼10% greater marbling (Marques et al., 2017). In sheep, maternal supplementation of ω-3 PUFA Ca2+ salts for the final trimester of gestation had no adverse effects on dam or fetus and increased blood concentrations of EPA and DHA in the ewe by ∼40% and in the newborn lamb by ∼50% (Coleman et al., 2018; Rosa Velazquez et al., 2020; Rosa-Velazquez et al., 2022). Greater fetal ω-3 PUFA availability appeared to be particularly beneficial to IUGR muscle and pancreatic islets. In mice, protein content for the insulin receptor and the myogenic transcription factor, myoD, as well as several genes regulating myogenesis were upregulated in skeletal muscle of newborn pups born to ω-3 PUFA-supplemented dams (Ghnaimawi et al., 2022). Moreover, 5-day infusion of EPA directly into IUGR fetal sheep improved growth of several muscles, restored normal fiber type proportions in the semitendinosus, and modestly improved deficits in muscle glucose uptake and oxidation (Lacey, 2021; Lacey et al., 2021). It also recovered about 50% of the deficit in glucose-stimulated insulin secretion (Lacey, 2021; Lacey et al., 2021). These benefits coincided with anti-inflammatory indicators, as EPA infusion ameliorated the elevated circulating TNFα concentrations and reduced total circulating white blood cells observed in IUGR fetal sheep (Lacey, 2021; Lacey et al., 2021). Likewise, maternal ω-3 PUFA supplementation reduced oxidative stress in brain tissues of fetal rats and resolved the heightened macrophage invasion observed in lung tissues of IUGR newborn mice pups (Velten et al., 2014).
Postnatal supplementation of ω-3 PUFA to IUGR-born offspring is also effective in improving metabolic deficits. For example, IUGR-born rats exhibited 50% reductions in circulating triglycerides and 33% reductions in blood urea nitrogen when nursing foster dams that were fed diets high in ω-3 PUFA (i.e., supplemented to offspring via milk) (Voggel et al., 2022). Likewise, directly feeding ω-3 PUFA-enriched diets or supplementing ω-3 PUFA-rich fish oil to IUGR-born juvenile rats partially resolved their hyperlipidemia and reduced their adiposity (Wyrwoll et al., 2006; Bringhenti et al., 2011; Chen et al., 2016; Chicco et al., 2016). It also decreased adipocyte size and leptin secretion, ameliorated inflammatory markers in adipose tissues, and resolved elevated TNFα in circulation (Wyrwoll et al., 2006; Bringhenti et al., 2011; Mark et al., 2014). Postnatal fish oil supplementation improved HOMA-estimated insulin sensitivity, glucose tolerance, and markers of systemic inflammation in IUGR-born rats (Bringhenti et al., 2011; Chen et al., 2016).
As of this writing, fish oil and other ω-3 PUFA supplements are considered safe for pregnant women by the American Pregnancy Association, which is supported by several Cochrane meta-analyses (Kar et al., 2016; Middleton et al., 2018; Wieland, 2019). Challenges regarding the use of ω-3 PUFA as dietary supplements in livestock include post-ingestive feedback (Freidin et al., 2011), bioavailability in ruminants (Chikunya et al., 2004), and meat sensory traits when fed at high concentrations (Burnett et al., 2020). Reduced palatability and ruminal microbiome changes associated with fish oil can cause animals to eat less when too much is included in a dietary ration, and livestock studies have indicated that the threshold limit is around 2% of the diet. Indeed, inclusion of fish oil at 1% of the diet in dairy cows did not affect ad libitum intake, but inclusion at 2% and 3% reduced intake by up to 11% and 32%, respectively (Donovan et al., 2000; Whitlock et al., 2002; Alizadeh et al., 2012; Kairenius et al., 2018). Although milk yield was increased by dietary fish oil at 1% and 2% in these cows, the large drop in dietary intake at 3% caused milk yield to fall (Donovan et al., 2000). Calves fed milk replacer enriched with 0.5% fish oil maintained their intake from birth to 2 months of age, which facilitated a modest increase in their average daily gain (Melendez et al., 2022). Lambs fed Ca2+ salts of ω-3 PUFA at 1.5% also maintained dry matter intake (Carranza Martin et al., 2018). In Angus feedlot steers, dry matter intake was reduced by dietary inclusion of fish oil at 2.4% but not at 0.8% or 1.6% (Shingfield et al., 2010). Limits on dietary inclusion could be problematic for ruminant livestock, as bioavailability of ω-3 PUFA is reduced by the markedly high rates of microbial biohydrogenation (i.e., saturation of previously unsaturated fatty acids) in the rumen (Jenkins et al., 2008). To illustrate, 93% of EPA and DHA from unprotected sources were absorbed unmodified across the intestinal wall of the rat (Chen et al., 1985), but as little as 7% were absorbed unmodified in cows (Dewhurst and Moloney, 2013). Similar outcomes were created by the rumen microbiome in sheep, where biohydrogenation rates were upward of 75% (Chikunya et al., 2004; Jenkins et al., 2008). Processing techniques for ω-3 PUFA-rich feed ingredients like flaxseed increased bioavailability of EPA by 30% and total ω-3 PUFA by 23% in lambs (Kronberg et al., 2012). In young goats, heat treatment of linseed oil diets increased DHA bioavailability by 62% and total ω-3 PUFA bioavailability by 19%, which corresponded with greater liver and adipose tissue concentrations (Wang et al., 2019). Freeze-drying of a microalgae-based supplement reduced ruminal biohydrogenation of EPA in lambs from 80% to about 45% (Vítor et al., 2021). Supplements utilizing Ca2+ salts of ω-3 PUFA helped maintain or increase bioavailability through ruminal passage without producing off-target effects such as reduced intake and milk fat (Castañeda-Gutiérrez et al., 2007; Oyebade et al., 2020). It is worth noting that even unprotected dietary sources still deliver meaningful amounts of absorbable ω-3 PUFA in ruminants. For example, inclusion of 1.1% fish oil in a silage-based diet for dairy cows increased the amount of EPA and DHA bypassing the rumen microbiome by 2-fold and 3-fold, respectively, despite 78% and 83% biohydrogenation rates (Kairenius et al., 2018). In meat animals, ω-3 PUFA supplementation strategies must consider the effects on product appearance and shelf life. Like all fatty acids, ω-3 PUFA are prone to oxidation that can discolor the surface of meat products (Burnett et al., 2020). Steers fed diets with 3% fish oil had 1.5-fold–2-fold greater carcass EPA and DHA content at harvest (Vatansever et al., 2000). Hamburger patties from these steers reached unacceptable discoloration 1–3 days sooner than normal and cooked sirloin scored about 11% lower in overall liking, despite no reduction in flavor score (Vatansever et al., 2000). It should be noted that most of the individual fatty acids measured in this study were increased in meat from fish oil-supplemented animals and that linseed oil supplementation in the same study increased ω-3 PUFA content without the same adverse sensory effects. Thus, adverse sensory traits may be attributable more to the use of a high volume of fish oil as a source than to the increase in ω-3 PUFA per se. Indeed, palatability and tolerability of German sausage products were not affected by enrichment with ∼1% purified EPA, DHA, and α-linolenic acid (Köhler et al., 2017). Nevertheless, fish products are the most common source for ω-3 PUFA supplements in livestock (Burnett et al., 2020), and lamb cuts from animals fed diets with 9% fish meal for 6 weeks were scored ∼9% lower in juiciness by a trained sensory panel, although flavor, aroma, and palatability were not affected (Ponnampalam et al., 2002). Likewise, meat from lambs fed diets enriched with 1.5% fish oil for 6 weeks was scored 14% lower in overall palatability, despite no reduction in individual scores for flavor, aroma, or juiciness and no issues with discoloration or shelf life (Ponnampalam et al., 2001; Ponnampalam et al., 2002). Because of the potential for reduced meat sensory traits, investigation into the benefit of withdrawing ω-3 PUFA supplements prior to harvest may be warranted.
Fetal IUGR is a common condition in humans and animals that impacts metabolic function and growth capacity before and after birth. It is most often associated with prenatal stressors during the critical window for placental development, which functionally and structurally stunts placental tissues. Placental insufficiency limits O2 and nutrient delivery to the growing fetus, which by late gestation can no longer keep up with fetal requirements for growth. Consequently, the fetus undergoes a prolonged period of progressive hypoxemia and hypoglycemia that in turn increases fetal adrenergic and inflammatory tones and induces oxidative stress. These changes drive nutrient-sparing adaptations, which manifest in asymmetric fetal growth restriction and thrifty metabolic function that persist postnatal. Mechanisms for these adaptations include reduced adrenergic responsiveness, enhanced inflammatory sensitivity, and increased oxidative stress. IUGR adaptations aid fetal survival, but after birth they increase health risks in humans and reduce growth efficiency in livestock. Enhanced inflammatory sensitivity in IUGR skeletal muscle and other tissues appears to be a key underlying factor in growth and metabolic pathologies. Consequently, anti-inflammatory nutraceuticals such as ω-3 PUFA have yielded promise as potential supplemental strategies for recovering health and performance outcomes in IUGR fetuses and offspring. However, several potential factors must be considered, including issues of palatability, bioavailability, and potential off-target effects. Nevertheless, initial proof-of-concept studies indicate that dietary ω-3 PUFA supplements could provide the basis for recovering growth and metabolic function in IUGR-born individuals.
MW and DY were responsible for the conceptualization and production of the manuscript. All authors contributed to the article and approved the submitted version.
This manuscript was supported in part by the USDA National Institute of Food and Agriculture Foundational Grants 2019-67015-29448 and 2020-67015-30825, the Nebraska Agricultural Experiment Station with funding from the Hatch Act (accession number 1009410), and Hatch Multistate Research capacity funding program (accession numbers 1011055, 1009410) through the USDA National Institute of Food and Agriculture.
The authors declare that the research was conducted in the absence of any commercial or financial relationships that could be construed as a potential conflict of interest.
All claims expressed in this article are solely those of the authors and do not necessarily represent those of their affiliated organizations, or those of the publisher, the editors and the reviewers. Any product that may be evaluated in this article, or claim that may be made by its manufacturer, is not guaranteed or endorsed by the publisher.
Agostoni, C., Galli, C., Riva, E., Colombo, C., Giovannini, M., and Marangoni, F. (2005). Reduced docosahexaenoic acid synthesis may contribute to growth restriction in infants born to mothers who smoke. J. Pediatr. 147 (6), 854–856. doi:10.1016/j.jpeds.2005.05.040
Al-Khalili, L., Bouzakri, K., Glund, S., Lonnqvist, F., Koistinen, H. A., and Krook, A. (2006). Signaling specificity of interleukin-6 action on glucose and lipid metabolism in skeletal muscle. Mol. Endocrinol. 20 (12), 3364–3375. doi:10.1210/me.2005-0490
Alejandre Alcazar, M. A., Ostreicher, I., Appel, S., Rother, E., Vohlen, C., Plank, C., et al. (2012). Developmental regulation of inflammatory cytokine-mediated Stat3 signaling: the missing link between intrauterine growth restriction and pulmonary dysfunction? J. Mol. Med. Berl. 90 (8), 945–957. doi:10.1007/s00109-012-0860-9
Ali, M. K., Amin, M. E., Amin, A. F., and Abd El Aal, D. E. M. (2017). Evaluation of the effectiveness of low-dose aspirin and omega 3 in treatment of asymmetrically intrauterine growth restriction: A randomized clinical trial. Eur. J. Obstet. Gynecol. Reprod. Biol. 210, 231–235. doi:10.1016/j.ejogrb.2017.01.002
Alizadeh, A. R., Alikhani, M., Ghorbani, G. R., Rahmani, H. R., Rashidi, L., and Loor, J. J. (2012). Effects of feeding roasted safflower seeds (variety IL-111) and fish oil on dry matter intake, performance and milk fatty acid profiles in dairy cattle. J. Anim. Physiol. Anim. Nutr. Berl. 96 (3), 466–473. doi:10.1111/j.1439-0396.2011.01165.x
Alkhalefah, A., Dunn, W. B., Allwood, J. W., Parry, K. L., Houghton, F. D., Ashton, N., et al. (2021). Maternal intermittent fasting during pregnancy induces fetal growth restriction and down-regulated placental system A amino acid transport in the rat. Clin. Sci. (Lond). 135 (11), 1445–1466. doi:10.1042/CS20210137
Allen, R. E., Merkel, R. A., and Young, R. B. (1979). Cellular aspects of muscle growth: myogenic cell proliferation. J. Anim. Sci. 49 (1), 115–127. doi:10.2527/jas1979.491115x
Alorabi, M., Mohammed, D. S., Mostafa-Hedeab, G., El-Sherbeni, S. A., Negm, W. A., Mohammed, A. I. A., et al. (2022). Combination treatment of omega-3 fatty acids and vitamin C exhibited promising therapeutic effect against oxidative impairment of the liver in methotrexate-intoxicated mice. Biomed. Res. Int. 2022, 4122166. doi:10.1155/2022/4122166
Alvarez, A. M., DeOcesano-Pereira, C., Teixeira, C., and Moreira, V. (2020). IL-1β and TNF-α modulation of proliferated and committed myoblasts: IL-6 and COX-2-derived prostaglandins as key actors in the mechanisms involved. Cells 9 (9), 2005. doi:10.3390/cells9092005
Amarilyo, G., Oren, A., Mimouni, F. B., Ochshorn, Y., Deutsch, V., and Mandel, D. (2011). Increased cord serum inflammatory markers in small-for-gestational-age neonates. J. Perinatol. 31 (1), 30–32. doi:10.1038/jp.2010.53
Amdi, C., Lynegaard, J. C., Thymann, T., and Williams, A. R. (2020). Intrauterine growth restriction in piglets alters blood cell counts and impairs cytokine responses in peripheral mononuclear cells 24 days post-partum. Sci. Rep. 10 (1), 4683. doi:10.1038/s41598-020-61623-w
Anderson, A. H., Fennessey, P. V., Meschia, G., Wilkening, R. B., and Battaglia, F. C. (1997). Placental transport of threonine and its utilization in the normal and growth-restricted fetus. Am. J. Physiol. 272 (1), E892–E900. doi:10.1152/ajpendo.1997.272.5.E892
Andersson, A. K., Börjesson, A., Sandgren, J., and Sandler, S. (2005). Cytokines affect PDX-1 expression, insulin and proinsulin secretion from iNOS deficient murine islets. Mol. Cell Endocrinol. 240 (1-2), 50–57. doi:10.1016/j.mce.2005.06.001
Archuleta, T. L., Lemieux, A. M., Saengsirisuwan, V., Teachey, M. K., Lindborg, K. A., Kim, J. S., et al. (2009). Oxidant stress-induced loss of IRS-1 and IRS-2 proteins in rat skeletal muscle: role of p38 MAPK. Free Radic. Biol. Med. 47 (10), 1486–1493. doi:10.1016/j.freeradbiomed.2009.08.014
Armstrong, D. G. (1973). Amino acid requirements and amino acid supply in the sheep. Proc. Nutr. Soc. 32 (2), 107–113. doi:10.1079/pns19730023
Assumpcao, R. P., Mucci, D. B., Fonseca, F. C. P., Marcondes, H., Sardinha, F. L. C., Citelli, M., et al. (2017). Fatty acid profile of maternal and fetal erythrocytes and placental expression of fatty acid transport proteins in normal and intrauterine growth restriction pregnancies. Prostagl. Leukot. Essent. Fat. Acids 125, 24–31. doi:10.1016/j.plefa.2017.08.011
Bacchetta, J., Harambat, J., Dubourg, L., Guy, B., Liutkus, A., Canterino, I., et al. (2009). Both extrauterine and intrauterine growth restriction impair renal function in children born very preterm. Kidney Int. 76 (4), 445–452. doi:10.1038/ki.2009.201
Bagley, H. N., Wang, Y., Campbell, M. S., Yu, X., Lane, R. H., and Joss-Moore, L. A. (2013). Maternal docosahexaenoic acid increases adiponectin and normalizes IUGR-induced changes in rat adipose deposition. J. Obes. 2013, 312153. doi:10.1155/2013/312153
Barker, D. J., Eriksson, J. G., Forsen, T., and Osmond, C. (2002). Fetal origins of adult disease: strength of effects and biological basis. Int. J. Epidemiol. 31 (6), 1235–1239. doi:10.1093/ije/31.6.1235
Barker, D. J., Hales, C. N., Fall, C. H., Osmond, C., Phipps, K., and Clark, P. M. (1993). Type 2 (non-insulin-dependent) diabetes mellitus, hypertension and hyperlipidaemia (syndrome X): relation to reduced fetal growth. Diabetologia 36 (1), 62–67. doi:10.1007/BF00399095
Barlow, J., Solomon, T. P. J., and Affourtit, C. (2018). Pro-inflammatory cytokines attenuate glucose-stimulated insulin secretion from INS-1E insulinoma cells by restricting mitochondrial pyruvate oxidation capacity - novel mechanistic insight from real-time analysis of oxidative phosphorylation. PLoS One 13 (6), e0199505. doi:10.1371/journal.pone.0199505
Bassett, J. M., and Hanson, C. (1998). Catecholamines inhibit growth in fetal sheep in the absence of hypoxemia. Am. J. Physiol. 274 (6), R1536–R1545. doi:10.1152/ajpregu.1998.274.6.R1536
Beard, J. R., Lincoln, D., Donoghue, D., Taylor, D., Summerhayes, R., Dunn, T. M., et al. (2009). Socioeconomic and maternal determinants of small-for-gestational age births: patterns of increasing disparity. Acta Obstet. Gynecol. Scand. 88 (5), 575–583. doi:10.1080/00016340902818170
Beede, K. A., Limesand, S. W., Petersen, J. L., and Yates, D. T. (2019). Real supermodels wear wool: summarizing the impact of the pregnant sheep as an animal model for adaptive fetal programming. Anim. Front. 9 (3), 34–43. doi:10.1093/af/vfz018
Beer, H. N. (2022). Continuous video monitoring of zoo cheetahs demonstrates differential engagement patterns for six different types of environmental enrichment; placental insufficiency indicators improve in intrauterine growth-restricted fetal sheep receiving daily ω-3 polyunsaturated fatty acid infusions; investigation of differentially expressed transcripts of the adipose tissue in fetal and neonatal IUGR sheep. The University of Nebraska-Lincoln. doi:10.13140/RG.2.2.13682.58562
Beer, H. N., Lacey, T. A., Gibbs, R. L., Most, M. S., Hicks, Z. M., Grijalva, P. C., et al. (2021). Placental insufficiency improves when intrauterine growth-restricted fetal sheep are administered daily ω-3 polyunsaturated fatty acid infusions. Transl. Animal Sci. 5, S6–S10. doi:10.1093/tas/txab166
Benjamin, J. S., Culpepper, C. B., Brown, L. D., Wesolowski, S. R., Jonker, S. S., Davis, M. A., et al. (2017). Chronic anemic hypoxemia attenuates glucose-stimulated insulin secretion in fetal sheep. Am. J. Physiol. Regul. Integr. Comp. Physiol. 312 (4), R492–R500. doi:10.1152/ajpregu.00484.2016
Berbets, A., Koval, H., Barbe, A., Albota, O., and Yuzko, O. (2021). Melatonin decreases and cytokines increase in women with placental insufficiency. J. Matern. Fetal Neonatal Med. 34 (3), 373–378. doi:10.1080/14767058.2019.1608432
Bernal-Santos, G., O'Donnell, A. M., Vicini, J. L., Hartnell, G. F., and Bauman, D. E. (2010). Hot topic: enhancing omega-3 fatty acids in milk fat of dairy cows by using stearidonic acid-enriched soybean oil from genetically modified soybeans. J. Dairy Sci. 93 (1), 32–37. doi:10.3168/jds.2009-2711
Block, R. C., Dier, U., Calderonartero, P., Shearer, G. C., Kakinami, L., Larson, M. K., et al. (2012). The effects of EPA+DHA and aspirin on inflammatory cytokines and angiogenesis factors. World J. Cardiovasc Dis. 2 (1), 14–19. doi:10.4236/wjcd.2012.21003
Bodell, P. W., Kodesh, E., Haddad, F., Zaldivar, F. P., Cooper, D. M., and Adams, G. R. (2009). Skeletal muscle growth in young rats is inhibited by chronic exposure to IL-6 but preserved by concurrent voluntary endurance exercise. J. Appl. Physiol. (1985) 106 (2), 443–453. doi:10.1152/japplphysiol.90831.2008
Boehmer, B. H., Limesand, S. W., and Rozance, P. J. (2017). The impact of IUGR on pancreatic islet development and beta-cell function. J. Endocrinol. 235 (2), R63–R76. doi:10.1530/JOE-17-0076
Botting, K. J., Loke, X. Y., Zhang, S., Andersen, J. B., Nyengaard, J. R., and Morrison, J. L. (2018). IUGR decreases cardiomyocyte endowment and alters cardiac metabolism in a sex- and cause-of-IUGR-specific manner. Am. J. Physiol. Regul. Integr. Comp. Physiol. 315 (1), R48–r67. doi:10.1152/ajpregu.00180.2017
Bringhenti, I., Schultz, A., Rachid, T., Bomfim, M. A., Mandarim-de-Lacerda, C. A., and Aguila, M. B. (2011). An early fish oil-enriched diet reverses biochemical, liver and adipose tissue alterations in male offspring from maternal protein restriction in mice. J. Nutr. Biochem. 22 (11), 1009–1014. doi:10.1016/j.jnutbio.2010.08.013
Brown, L. D. (2014). Endocrine regulation of fetal skeletal muscle growth: impact on future metabolic health. J. Endocrinol. 221 (2), R13–R29. doi:10.1530/JOE-13-0567
Brown, L. D., Kohn, J. R., Rozance, P. J., Hay, W. W., and Wesolowski, S. R. (2017). Exogenous amino acids suppress glucose oxidation and potentiate hepatic glucose production in late gestation fetal sheep. Am. J. Physiol. Regul. Integr. Comp. Physiol. 312 (5), R654–R663. doi:10.1152/ajpregu.00502.2016
Brown, L. D., Rozance, P. J., Bruce, J. L., Friedman, J. E., Hay, W. W., and Wesolowski, S. R. (2015). Limited capacity for glucose oxidation in fetal sheep with intrauterine growth restriction. Am. J. Physiol. Regul. Integr. Comp. Physiol. 309 (8), R920–R928. doi:10.1152/ajpregu.00197.2015
Brown, L. D., Rozance, P. J., Thorn, S. R., Friedman, J. E., and Hay, W. W. (2012). Acute supplementation of amino acids increases net protein accretion in IUGR fetal sheep. Am. J. Physiol. Endocrinol. Metab. 303 (3), E352–E364. doi:10.1152/ajpendo.00059.2012
Brown, L. D., Wesolowski, S. R., Kailey, J., Bourque, S., Wilson, A., Andrews, S. E., et al. (2016). Chronic hyperinsulinemia increases myoblast proliferation in fetal sheep skeletal muscle. Endocrinol. 157 (6), 2447–2460. doi:10.1210/en.2015-1744
Bruce, C. R., and Dyck, D. J. (2004). Cytokine regulation of skeletal muscle fatty acid metabolism: effect of interleukin-6 and tumor necrosis factor-alpha. Am. J. Physiol. Endocrinol. Metab. 287 (4), E616–E621. doi:10.1152/ajpendo.00150.2004
Bulua, A. C., Simon, A., Maddipati, R., Pelletier, M., Park, H., Kim, K. Y., et al. (2011). Mitochondrial reactive oxygen species promote production of proinflammatory cytokines and are elevated in TNFR1-associated periodic syndrome (TRAPS). J. Exp. Med. 208 (3), 519–533. doi:10.1084/jem.20102049
Burnett, D. D., Legako, J. F., Phelps, K. J., and Gonzalez, J. M. (2020). Biology, strategies, and fresh meat consequences of manipulating the fatty acid composition of meat. J. Anim. Sci. 98 (2), skaa033. doi:10.1093/jas/skaa033
Burton, G. J., and Jauniaux, E. (2018). Pathophysiology of placental-derived fetal growth restriction. Am. J. Obstet. Gynecol. 218 (2S), S745–S761. doi:10.1016/j.ajog.2017.11.577
Cadaret, C. N., Beede, K. A., Riley, H. E., and Yates, D. T. (2017). Acute exposure of primary rat soleus muscle to zilpaterol HCl (β2 adrenergic agonist), TNFα, or IL-6 in culture increases glucose oxidation rates independent of the impact on insulin signaling or glucose uptake. Cytokine 96, 107–113. doi:10.1016/j.cyto.2017.03.014
Cadaret, C. N., Merrick, E. M., Barnes, T. L., Beede, K. A., Posont, R. J., Petersen, J. L., et al. (2019b). Sustained maternal inflammation during the early third-trimester yields intrauterine growth restriction, impaired skeletal muscle glucose metabolism, and diminished beta-cell function in fetal sheep. J. Anim. Sci. 97 (12), 4822–4833. doi:10.1093/jas/skz321
Cadaret, C. N., Posont, R. J., Beede, K. A., Riley, H. E., Loy, J. D., and Yates, D. T. (2019a). Maternal inflammation at midgestation impairs subsequent fetal myoblast function and skeletal muscle growth in rats, resulting in intrauterine growth restriction at term. Transl. Anim. Sci. 3 (2), txz037. doi:10.1093/tas/txz037
Cadaret, C. N., Posont, R. J., Swanson, R. M., Beard, J. K., Barnes, T. L., Beede, K. A., et al. (2019c). Intermittent maternofetal O(2) supplementation during late gestation rescues placental insufficiency-induced intrauterine growth restriction and metabolic pathologies in the neonatal lamb. Transl. Anim. Sci. 3 (1), 1696–1700. doi:10.1093/tas/txz060
Cadaret, C. N., Posont, R. J., Swanson, R. M., Beard, J. K., Gibbs, R. L., Barnes, T. L., et al. (2022). Intermittent maternofetal oxygenation during late gestation improved birthweight, neonatal growth, body symmetry, and muscle metabolism in intrauterine growth-restricted lambs. J. Anim. Sci. 100 (1), skab358. doi:10.1093/jas/skab358
Calnan, H., Williams, A., Peterse, J., Starling, S., Cook, J., Connaughton, S., et al. (2021). A prototype rapid dual energy X-ray absorptiometry (DEXA) system can predict the CT composition of beef carcases. Meat Sci. 173, 108397. doi:10.1016/j.meatsci.2020.108397
Camacho, L. E., Davis, M. A., Kelly, A. C., Steffens, N. R., Anderson, M. J., and Limesand, S. W. (2022). Prenatal oxygen and glucose therapy normalizes insulin secretion and action in growth-restricted fetal sheep. Endocrinol 163 (6), bqac053. doi:10.1210/endocr/bqac053
Carey, A. L., Steinberg, G. R., Macaulay, S. L., Thomas, W. G., Holmes, A. G., Ramm, G., et al. (2006). Interleukin-6 increases insulin-stimulated glucose disposal in humans and glucose uptake and fatty acid oxidation in vitro via AMP-activated protein kinase. Diabetes 55 (10), 2688–2697. doi:10.2337/db05-1404
Carranza Martin, A. C., Coleman, D. N., Garcia, L. G., Furnus, C. C., and Relling, A. E. (2018). Prepartum fatty acid supplementation in sheep. III. Effect of eicosapentaenoic acid and docosahexaenoic acid during finishing on performance, hypothalamus gene expression, and muscle fatty acids composition in lambs. J. Anim. Sci. 96 (12), 5300–5310. doi:10.1093/jas/sky360
Carver, T. D., Quick, A. A., Teng, C. C., Pike, A. W., Fennessey, P. V., and Hay, W. W. (1997). Leucine metabolism in chronically hypoglycemic hypoinsulinemic growth-restricted fetal sheep. Am. J. Physiol. 272 (1), E107–E117. doi:10.1152/ajpendo.1997.272.1.E107
Castañeda-Gutiérrez, E., de Veth, M. J., Lock, A. L., Dwyer, D. A., Murphy, K. D., and Bauman, D. E. (2007). Effect of supplementation with calcium salts of fish oil on n-3 fatty acids in milk fat. J. Dairy Sci. 90 (9), 4149–4156. doi:10.3168/jds.2006-856
Cetin, I., Giovannini, N., Alvino, G., Agostoni, C., Riva, E., Giovannini, M., et al. (2002). Intrauterine growth restriction is associated with changes in polyunsaturated fatty acid fetal-maternal relationships. Pediatr. Res. 52 (5), 750–755. doi:10.1203/00006450-200211000-00023
Chang, E. I., Rozance, P. J., Wesolowski, S. R., Nguyen, L. M., Shaw, S. C., Sclafani, R. A., et al. (2019b). Rates of myogenesis and myofiber numbers are reduced in late gestation IUGR fetal sheep. J. Endocrinol. 244 (2), 339–352. doi:10.1530/JOE-19-0273
Chang, E. I., Wesolowski, S. R., Gilje, E. A., Baker, P. R., Reisz, J. A., D'Alessandro, A., et al. (2019a). Skeletal muscle amino acid uptake is lower and alanine production is greater in late gestation intrauterine growth-restricted fetal sheep hindlimb. Am. J. Physiol. Regul. Integr. Comp. Physiol. 317 (5), R615–R629. doi:10.1152/ajpregu.00115.2019
Chang, E. I., Hetrick, B., Wesolowski, S. R., McCurdy, C. E., Rozance, P. J., and Brown, L. D. (2021). A Two-Week insulin infusion in intrauterine growth restricted fetal sheep at 75% gestation increases skeletal myoblast replication but did not restore muscle mass or increase fiber number. Front. Endocrinol. (Lausanne) 12, 785242. doi:10.3389/fendo.2021.785242
Chassen, S. S., Ferchaud-Roucher, V., Palmer, C., Li, C., Jansson, T., Nathanielsz, P. W., et al. (2020). Placental fatty acid transport across late gestation in a baboon model of intrauterine growth restriction. J. Physiol. 598 (12), 2469–2489. doi:10.1113/JP279398
Chassen, S. S., Zemski-Berry, K., Raymond-Whish, S., Driver, C., Hobbins, J. C., and Powell, T. L. (2022). Altered cord blood lipid concentrations correlate with birth weight and Doppler velocimetry of fetal vessels in human fetal growth restriction pregnancies. Cells 11 (19), 3110. doi:10.3390/cells11193110
Chen, I. S., Subramaniam, S., Cassidy, M. M., Sheppard, A. J., and Vahouny, G. V. (1985). Intestinal absorption and lipoprotein transport of (omega-3) eicosapentaenoic acid. J. Nutr. 115 (2), 219–225. doi:10.1093/jn/115.2.219
Chen, L. H., Liang, L., Fang, Y. L., Wang, Y. M., and Zhu, W. F. (2016). Fish oil improves lipid profile in juvenile rats with intrauterine growth retardation by altering the transcriptional expression of lipid-related hepatic genes. J. Matern. Fetal Neonatal Med. 29 (20), 3292–3298. doi:10.3109/14767058.2015.1123244
Chen, X., Fahy, A. L., Green, A. S., Anderson, M. J., Rhoads, R. P., and Limesand, S. W. (2010). β2-Adrenergic receptor desensitization in perirenal adipose tissue in fetuses and lambs with placental insufficiency-induced intrauterine growth restriction. J. Physiol. 588 (18), 3539–3549. doi:10.1113/jphysiol.2010.192310
Chen, X., Green, A. S., Macko, A. R., Yates, D. T., Kelly, A. C., and Limesand, S. W. (2014). Enhanced insulin secretion responsiveness and islet adrenergic desensitization after chronic norepinephrine suppression is discontinued in fetal sheep. Am. J. Physiol. Endocrinol. Metab. 306 (1), E58–E64. doi:10.1152/ajpendo.00517.2013
Chen, X., Kelly, A. C., Yates, D. T., Macko, A. R., Lynch, R. M., and Limesand, S. W. (2017). Islet adaptations in fetal sheep persist following chronic exposure to high norepinephrine. J. Endocrinol. 232 (2), 285–295. doi:10.1530/JOE-16-0445
Chicco, A., Creus, A., Illesca, P., Hein, G. J., Rodriguez, S., and Fortino, A. (2016). Effects of post-suckling n-3 polyunsaturated fatty acids: prevention of dyslipidemia and liver steatosis induced in rats by a sucrose-rich diet during pre- and post-natal life. Food Funct. 7 (1), 445–454. doi:10.1039/c5fo00705d
Chikunya, S., Demirel, G., Enser, M., Wood, J. D., Wilkinson, R. G., and Sinclair, L. A. (2004). Biohydrogenation of dietary n-3 PUFA and stability of ingested vitamin E in the rumen, and their effects on microbial activity in sheep. Br. J. Nutr. 91 (4), 539–550. doi:10.1079/BJN20031078
Chisaka, T., Mogi, M., Nakaoka, H., Kan-no, H., Tsukuda, K., Wang, X-L., et al. (2015). Low-protein diet-induced fetal growth restriction leads to exaggerated proliferative response to vascular injury in postnatal life. Am. J. Hypertens. 29 (1), 54–62. doi:10.1093/ajh/hpv072
Cilvik, S. N., Wesolowski, S. R., Anthony, R. V., Brown, L. D., and Rozance, P. J. (2021). Late gestation fetal hyperglucagonaemia impairs placental function and results in diminished fetal protein accretion and decreased fetal growth. J. Physiol. 599 (13), 3403–3427. doi:10.1113/JP281288
Coleman, D. N., Rivera-Acevedo, K. C., and Relling, A. E. (2018). Prepartum fatty acid supplementation in sheep I. Eicosapentaenoic and docosahexaenoic acid supplementation do not modify Ewe and lamb metabolic status and performance through weaning. J. Anim. Sci. 96 (1), 364–374. doi:10.1093/jas/skx012
Collin, F. (2019). Chemical basis of reactive oxygen species reactivity and involvement in neurodegenerative diseases. Int. J. Mol. Sci. 20 (10), 2407. doi:10.3390/ijms20102407
Cree-Green, M., Scalzo, R. L., Harrall, K., Newcomer, B. R., Schauer, I. E., Huebschmann, A. G., et al. (2018). Supplemental oxygen improves in vivo mitochondrial oxidative phosphorylation flux in sedentary obese adults with type 2 diabetes. Diabetes 67 (7), 1369–1379. doi:10.2337/db17-1124
Cui, C., Wu, C., Wang, J., Ma, Z., Zheng, X., Zhu, P., et al. (2022). Restored intestinal integrity, nutrients transporters, energy metabolism, antioxidative capacity and decreased harmful microbiota were associated with IUGR piglet's catch-up growth before weanling. J. Anim. Sci. Biotechnol. 13 (1), 129. doi:10.1186/s40104-022-00770-8
da Silva, E. P., Nachbar, R. T., Levada-Pires, A. C., Hirabara, S. M., and Lambertucci, R. H. (2016). Omega-3 fatty acids differentially modulate enzymatic anti-oxidant systems in skeletal muscle cells. Cell Stress Chaperones 21 (1), 87–95. doi:10.1007/s12192-015-0642-8
Davis, M. A., Camacho, L. E., Pendleton, A. L., Antolic, A. T., Luna-Ramirez, R. I., Kelly, A. C., et al. (2021). Augmented glucose production is not contingent on high catecholamines in fetal sheep with IUGR. J. Endocrinol. 249 (3), 195–207. doi:10.1530/JOE-21-0071
De Blasio, M. J., Gatford, K. L., Harland, M. L., Robinson, J. S., and Owens, J. A. (2012). Placental restriction reduces insulin sensitivity and expression of insulin signaling and glucose transporter genes in skeletal muscle, but not liver, in young sheep. Endocrinol 153 (5), 2142–2151. doi:10.1210/en.2011-1955
De Blasio, M. J., Gatford, K. L., McMillen, I. C., Robinson, J. S., and Owens, J. A. (2007b). Placental restriction of fetal growth increases insulin action, growth, and adiposity in the young lamb. Endocrinology 148 (3), 1350–1358. doi:10.1210/en.2006-0653
De Blasio, M. J., Gatford, K. L., Robinson, J. S., and Owens, J. A. (2007a). Placental restriction of fetal growth reduces size at birth and alters postnatal growth, feeding activity, and adiposity in the young lamb. Am. J. Physiol. Regul. Integr. Comp. Physiol. 292 (2), R875–R886. doi:10.1152/ajpregu.00430.2006
Desai, M., Gayle, D. A., Casillas, E., Boles, J., and Ross, M. G. (2009). Early undernutrition attenuates the inflammatory response in adult rat offspring. J. Maternal-Fetal Neonatal Med. 22 (7), 571–575. doi:10.1080/14767050902874105
Detmer, A., Carter, A. M., and Thomas, C. R. (1992). The metabolism of free fatty acids and triacylglycerols by the fetal liver in a Guinea pig model of intrauterine growth retardation. Pediatr. Res. 32 (4), 441–446. doi:10.1203/00006450-199210000-00014
Dewhurst, R., and Moloney, A. (2013). Modification of animal diets for the enrichment of dairy and meat products with omega-3 fatty acids. Food Enrich. omega-3 Fat. acids, 257–287. doi:10.1533/9780857098863.3.257
Diamond-Stanic, M. K., Marchionne, E. M., Teachey, M. K., Durazo, D. E., Kim, J. S., and Henriksen, E. J. (2011). Critical role of the transient activation of p38 MAPK in the etiology of skeletal muscle insulin resistance induced by low-level in vitro oxidant stress. Biochem. Biophys. Res. Commun. 405 (3), 439–444. doi:10.1016/j.bbrc.2011.01.049
Dong, L., Zhong, X., Zhang, L., Kong, L., Kong, Y., Kou, T., et al. (2015). Impaired intestinal mucosal immunity is associated with the imbalance of T lymphocyte sub-populations in intrauterine growth-restricted neonatal piglets. Immunobiology 220 (6), 775–781. doi:10.1016/j.imbio.2014.12.017
Donnelly, L. E., Tudhope, S. J., Fenwick, P. S., and Barnes, P. J. (2010). Effects of formoterol and salmeterol on cytokine release from monocyte-derived macrophages. Eur. Respir. J. 36 (1), 178–186. doi:10.1183/09031936.00158008
Donovan, D. C., Schingoethe, D. J., Baer, R. J., Ryali, J., Hippen, A. R., and Franklin, S. T. (2000). Influence of dietary fish oil on conjugated linoleic acid and other fatty acids in milk fat from lactating dairy cows. J. Dairy Sci. 83 (11), 2620–2628. doi:10.3168/jds.S0022-0302(00)75155-1
Drake, R. R., Louey, S., and Thornburg, K. L. (2022). Intrauterine growth restriction elevates circulating acylcarnitines and suppresses fatty acid metabolism genes in the fetal sheep heart. J. Physiol. 600 (3), 655–670. doi:10.1113/JP281415
Du, X., Liu, M., Tai, W., Yu, H., Hao, X., Loor, J. J., et al. (2022). Tumor necrosis factor-α promotes lipolysis and reduces insulin sensitivity by activating nuclear factor kappa B and c-Jun N-terminal kinase in primary bovine adipocytes. J. Dairy Sci. 105 (10), 8426–8438. doi:10.3168/jds.2022-22009
Duan, C., Liu, M., Xu, H., Tang, W., Liu, J., Hou, L., et al. (2016). Decreased expression of GLUT4 in male CG-IUGR rats may play a vital role in their increased susceptibility to diabetes mellitus in adulthood. Acta Biochim. Biophys. Sin. (Shanghai). 48 (10), 872–882. doi:10.1093/abbs/gmw088
Duffield, J. A., Vuocolo, T., Tellam, R., McFarlane, J. R., Kauter, K. G., Muhlhausler, B. S., et al. (2009). Intrauterine growth restriction and the sex specific programming of leptin and peroxisome proliferator-activated receptor gamma (PPARgamma) mRNA expression in visceral fat in the lamb. Pediatr. Res. 66 (1), 59–65. doi:10.1203/PDR.0b013e3181a7c121
Dulloo, A. G., Jacquet, J., and Montani, J. P. (2012). How dieting makes some fatter: from a perspective of human body composition autoregulation. Proc. Nutr. Soc. 71 (3), 379–389. doi:10.1017/S0029665112000225
Dulloo, A. G., Jacquet, J., Seydoux, J., and Montani, J. P. (2006). The thrifty 'catch-up fat' phenotype: its impact on insulin sensitivity during growth trajectories to obesity and metabolic syndrome. Int. J. Obes. 30 (S4), S23–S35. doi:10.1038/sj.ijo.0803516
Edwards, A. K., McKnight, S. M., Askelson, K., McKnight, J. R., Dunlap, K. A., and Satterfield, M. C. (2020). Adaptive responses to maternal nutrient restriction alter placental transport in ewes. Placenta 96, 1–9. doi:10.1016/j.placenta.2020.05.002
Ellingsgaard, H., Hauselmann, I., Schuler, B., Habib, A. M., Baggio, L. L., Meier, D. T., et al. (2011). Interleukin-6 enhances insulin secretion by increasing glucagon-like peptide-1 secretion from L cells and alpha cells. Nat. Med. 17 (11), 1481–1489. doi:10.1038/nm.2513
Elphick, M. C., Harrison, A. T., Lawlor, J. P., and Hull, D. (1978). Cord blood hypertriglyceridaemia as an index of fetal stress: use of a simple screening test and results of further biochemical analysis. Br. J. Obstet. Gynaecol. 85 (4), 303–310. doi:10.1111/j.1471-0528.1978.tb10503.x
Enoki, Y., Watanabe, H., Arake, R., Sugimoto, R., Imafuku, T., Tominaga, Y., et al. (2016). Indoxyl sulfate potentiates skeletal muscle atrophy by inducing the oxidative stress-mediated expression of myostatin and atrogin-1. Sci. Rep. 6, 32084. doi:10.1038/srep32084
Fagerberg, B., Bondjers, L., and Nilsson, P. (2004). Low birth weight in combination with catch-up growth predicts the occurrence of the metabolic syndrome in men at late middle age: the atherosclerosis and insulin resistance study. J. Intern Med. 256 (3), 254–259. doi:10.1111/j.1365-2796.2004.01361.x
Fares, S., Sethom, M. M., Kacem, S., Khouaja-Mokrani, C., Feki, M., and Kaabachi, N. (2015). Plasma arachidonic and docosahexaenoic acids in Tunisian very low birth weight infants: status and association with selected neonatal morbidities. J. Health Popul. Nutr. 33, 1. doi:10.1186/s41043-015-0011-3
Felicioni, F., Pereira, A. D., Caldeira-Brant, A. L., Santos, T. G., Paula, T. M. D., Magnabosco, D., et al. (2020). Postnatal development of skeletal muscle in pigs with intrauterine growth restriction: morphofunctional phenotype and molecular mechanisms. J. Anat. 236 (5), 840–853. doi:10.1111/joa.13152
Fitzgerald, K. A., Rowe, D. C., Barnes, B. J., Caffrey, D. R., Visintin, A., Latz, E., et al. (2003). LPS-TLR4 signaling to IRF-3/7 and NF-kappaB involves the toll adapters TRAM and TRIF. J. Exp. Med. 198 (7), 1043–1055. doi:10.1084/jem.20031023
Floyd, R. A., Hensley, K., Jaffery, F., Maidt, L., Robinson, K., Pye, Q., et al. (1999). Increased oxidative stress brought on by pro-inflammatory cytokines in neurodegenerative processes and the protective role of nitrone-based free radical traps. Life Sci. 65 (18-19), 1893–1899. doi:10.1016/s0024-3205(99)00443-9
Fowden, A. L., and Silver, M. (1995). Glucose and oxygen metabolism in the fetal foal during late gestation. Am. J. Physiol. 269 (6 Pt 2), R1455–R1461. doi:10.1152/ajpregu.1995.269.6.R1455
Freidin, E., Catanese, F., Didoné, N., and Distel, R. A. (2011). Mechanisms of intake induction of a low-nutritious food in sheep (Ovis aries). Behav. Process. 87 (3), 246–252. doi:10.1016/j.beproc.2011.04.005
García, J. G., de Miguel, C., Milagro, F. I., Zalba, G., and Ansorena, E. (2021). Endothelial NOX5 expression modulates thermogenesis and lipolysis in mice fed with a high-fat diet and 3T3-L1 adipocytes through an interleukin-6 dependent mechanism. Antioxidants (Basel) 11 (1), 30. doi:10.3390/antiox11010030
Gardner, D. S., Fletcher, A. J., Bloomfield, M. R., Fowden, A. L., and Giussani, D. A. (2002). Effects of prevailing hypoxaemia, acidaemia or hypoglycaemia upon the cardiovascular, endocrine and metabolic responses to acute hypoxaemia in the ovine fetus. J. Physiol. 540 (Pt 1), 351–366. doi:10.1113/jphysiol.2001.013434
Ganguly, A., Touma, M., Thamotharan, S., De Vivo, D. C., and Devaskar, S. U. (2016). Maternal calorie restriction causing uteroplacental insufficiency differentially affects mammalian placental glucose and leucine transport molecular mechanisms. Endocrinol. 157 (10), 4041–4054. doi:10.1210/en.2016-1259
Garg, M., Thamotharan, M., Oak, S. A., Pan, G., Maclaren, D. C., Lee, P. W., et al. (2009). Early exercise regimen improves insulin sensitivity in the intrauterine growth-restricted adult female rat offspring. Am. J. Physiol. Endocrinol. Metab. 296 (2), E272–E281. doi:10.1152/ajpendo.90473.2008
Garite, T. J., Clark, R., and Thorp, J. A. (2004). Intrauterine growth restriction increases morbidity and mortality among premature neonates. Am. J. Obstet. Gynecol. 191 (2), 481–487. doi:10.1016/j.ajog.2004.01.036
Ghnaimawi, S., Zhang, S., Baum, J. I., and Huang, Y. (2022). The effects of maternal intake of EPA and DHA enriched diet during pregnancy and lactation on offspring's muscle development and energy homeostasis. Front. Physiol. 13, 881624. doi:10.3389/fphys.2022.881624
Gholami, N., Abotorabi, S., Lalooha, F., and Oveisi, S. (2020). Effects of fish oil supplementation on pregnancy outcomes in pregnant women referred to kosar hospital. Iran. J. Pharm. Res. 19 (3), 241–247. doi:10.22037/ijpr.2019.13976.12041
Gibbs, R. L., Swanson, R. M., Beard, J. K., Schmidt, T. B., Petersen, J. L., and Yates, D. T. (2020). Deficits in growth, muscle mass, and body composition following placental insufficiency-induced intrauterine growth restriction persisted in lambs at 60 d of age but were improved by daily clenbuterol supplementation. Transl. Anim. Sci. 4 (Suppl. 1), S53–S57. doi:10.1093/tas/txaa097
Gibbs, R. L., Swanson, R. M., Beard, J. K., Schmidt, T. B., Petersen, J. L., and Yates, D. T. (2021). Deficits in skeletal muscle glucose metabolism and whole-body oxidative metabolism in the intrauterine growth-restricted juvenile lamb are improved by daily treatment with clenbuterol. Transl. Anim. Sci. 5 (Suppl. 1), S20–S24. doi:10.1093/tas/txab187
Gibbs, R. L., and Yates, D. T. (2021). The price of surviving on adrenaline: developmental programming responses to chronic fetal hypercatecholaminemia contribute to poor muscle growth capacity and metabolic dysfunction in IUGR-born offspring. Front. Anim. Sci. 2, 769334. doi:10.3389/fanim.2021.769334
Glund, S., Deshmukh, A., Long, Y. C., Moller, T., Koistinen, H. A., Caidahl, K., et al. (2007). Interleukin-6 directly increases glucose metabolism in resting human skeletal muscle. Diabetes 56 (6), 1630–1637. doi:10.2337/db06-1733
Godfray, H. C., Beddington, J. R., Crute, I. R., Haddad, L., Lawrence, D., Muir, J. F., et al. (2010). Food security: the challenge of feeding 9 billion people. Science 327 (5967), 812–818. doi:10.1126/science.1185383
Goldenberg, R. L., and Culhane, J. F. (2007). Low birth weight in the United States. Am. J. Clin. Nutr. 85 (2), 584S–90S. doi:10.1093/ajcn/85.2.584S
Gortan Cappellari, G., Semolic, A., Ruozi, G., Barbetta, D., Bortolotti, F., Vinci, P., et al. (2022). n-3 PUFA dietary lipid replacement normalizes muscle mitochondrial function and oxidative stress through enhanced tissue mitophagy and protects from muscle wasting in experimental kidney disease. Metabolism 133, 155242. doi:10.1016/j.metabol.2022.155242
Gosby, A. K., Stanton, L. M., Maloney, C. A., Thompson, M., Briody, J., Baxter, R. C., et al. (2009). Postnatal nutrition alters body composition in adult offspring exposed to maternal protein restriction. Br. J. Nutr. 101 (12), 1878–1884. doi:10.1017/S0007114508135851
Gray, S. R., Ratkevicius, A., Wackerhage, H., Coats, P., and Nimmo, M. A. (2009). The effect of interleukin-6 and the interleukin-6 receptor on glucose transport in mouse skeletal muscle. Exp. Physiol. 94 (8), 899–905. doi:10.1113/expphysiol.2009.048173
Green, A., Rumberger, J. M., Stuart, C. A., and Ruhoff, M. S. (2004). Stimulation of lipolysis by tumor necrosis factor-alpha in 3T3-L1 adipocytes is glucose dependent: implications for long-term regulation of lipolysis. Diabetes 53 (1), 74–81. doi:10.2337/diabetes.53.1.74
Greenwood, P., Cafe, L., Hearnshaw, H., and Hennessy, D. (2005). Consequences of nutrition and growth retardation early in life for growth and composition of cattle and eating quality of beef. Recent Adv. Animal Nutr. Aust. 15, 183–195.
Greenwood, P. L., and Bell, A. W. (2019). Developmental programming and growth of livestock tissues for meat production. Vet. Clin. North Am. Food Anim. Pract. 35 (2), 303–319. doi:10.1016/j.cvfa.2019.02.008
Greenwood, P. L., and Cafe, L. M. (2007). Prenatal and pre-weaning growth and nutrition of cattle: long-term consequences for beef production. Anim 1 (9), 1283–1296. doi:10.1017/S175173110700050X
Greenwood, P. L., Hunt, A. S., Hermanson, J. W., and Bell, A. W. (1998). Effects of birth weight and postnatal nutrition on neonatal sheep: I. Body growth and composition, and some aspects of energetic efficiency. J. Anim. Sci. 76 (9), 2354–2367. doi:10.2527/1998.7692354x
Groenenberg, I. A., Wladimiroff, J. W., and Hop, W. C. (1989). Fetal cardiac and peripheral arterial flow velocity waveforms in intrauterine growth retardation. Circulation 80 (6), 1711–1717. doi:10.1161/01.cir.80.6.1711
Grzelkowska-Kowalczyk, K., and Wieteska-Skrzeczynska, W. (2006). Exposure to TNF-alpha but not IL-1beta impairs insulin-dependent phosphorylation of protein kinase B and p70S6k in mouse C2C12 myogenic cells. Pol. J. Vet. Sci. 9 (1), 1–10.
Gurung, S., Tong, H. H., Bryce, E., Katz, J., Lee, A. C., Black, R. E., et al. (2022). A systematic review on estimating population attributable fraction for risk factors for small-for-gestational-age births in 81 low- and middle-income countries. J. Glob. Health 12, 04024. doi:10.7189/jogh.12.04024
Haddad, F., Zaldivar, F., Cooper, D. M., and Adams, G. R. (2005). IL-6-induced skeletal muscle atrophy. J. Appl. Physiol. (1985) 98 (3), 911–917. doi:10.1152/japplphysiol.01026.2004
Hadjivassiliou, V., Green, M. H., James, R. F., Swift, S. M., Clayton, H. A., and Green, I. C. (1998). Insulin secretion, DNA damage, and apoptosis in human and rat islets of Langerhans following exposure to nitric oxide, peroxynitrite, and cytokines. Nitric Oxide 2 (6), 429–441. doi:10.1006/niox.1998.0203
Hales, C. N., Barker, D. J., Clark, P. M., Cox, L. J., Fall, C., Osmond, C., et al. (1991). Fetal and infant growth and impaired glucose tolerance at age 64. Br. Med. J. 303 (6809), 1019–1022. doi:10.1136/bmj.303.6809.1019
Hales, C. N., and Barker, D. J. (2001). The thrifty phenotype hypothesis. BrMedBull 60, 5–20. doi:10.1093/bmb/60.1.5
Hales, C. N., and Barker, D. J. P. (1992). Type 2 (non-insulin-dependent) diabetes mellitus: the thrifty phenotype hypothesis. Diabetologia 35 (7), 595–601. doi:10.1007/BF00400248
Ham, J. N., Crutchlow, M. F., Desai, B. M., Simmons, R. A., and Stoffers, D. A. (2009). Exendin-4 normalizes islet vascularity in intrauterine growth restricted rats: potential role of VEGF. Pediatr. Res. 66 (1), 42–46. doi:10.1203/PDR.0b013e3181a282a5
Harwell, C. M., Padbury, J. F., Anand, R. S., Martinez, A. M., Ipp, E., Thio, S. L., et al. (1990). Fetal catecholamine responses to maternal hypoglycemia. Am. J. Physiol. 259 (6 Pt 2), R1126–R1130. doi:10.1152/ajpregu.1990.259.6.R1126
He, J., Niu, Y., Wang, F., Wang, C., Cui, T., Bai, K., et al. (2018). Dietary curcumin supplementation attenuates inflammation, hepatic injury and oxidative damage in a rat model of intra-uterine growth retardation. Br. J. Nutr. 120 (5), 537–548. doi:10.1017/S0007114518001630
Heras-Molina, A., Escudero, R., Pesántez-Pacheco, J. L., García-Contreras, C., Vázquez-Gómez, M., Astiz, S., et al. (2022). Maternal supplementation with polyphenols and omega-3 fatty acids during pregnancy: prenatal effects on fetal fatty acid composition in the iberian pig. Anim. (Basel) 12 (16), 2140. doi:10.3390/ani12162140
Heras-Molina, A., Pesantez-Pacheco, J. L., Astiz, S., Garcia-Contreras, C., Vazquez-Gomez, M., Encinas, T., et al. (2020). Maternal supplementation with polyphenols and omega-3 fatty acids during pregnancy: effects on growth, metabolism, and body composition of the offspring. Anim. (Basel) 10 (11), 1946. doi:10.3390/ani10111946
Heras-Molina, A., Pesántez-Pacheco, J. L., Garcia-Contreras, C., Vázquez-Gómez, M., López, A., Benítez, R., et al. (2021). Maternal supplementation with polyphenols and omega-3 fatty acids during pregnancy: prenatal effects on growth and metabolism. Anim. (Basel) 11 (6), 1699. doi:10.3390/ani11061699
Hicks, Z. H., Beer, H. N., Herrera, N. J., Gibbs, R. L., Lacey, T. A., Grijalva, P. C., et al. (2021). Hindlimb tissue composition shifts between the fetal and juvenile stages in the lamb. Transl. Anim. Sci. 5 (Suppl. 1), S38–S40. doi:10.1093/tas/txab164
Hicks, Z. M., and Yates, D. T. (2021). Going up inflame: reviewing the underexplored role of inflammatory programming in stress-induced intrauterine growth restricted livestock. Front. Anim. Sci. 2, 761421. doi:10.3389/fanim.2021.761421
Higaki, Y., Mikami, T., Fujii, N., Hirshman, M. F., Koyama, K., Seino, T., et al. (2008). Oxidative stress stimulates skeletal muscle glucose uptake through a phosphatidylinositol 3-kinase-dependent pathway. Am. J. Physiol. Endocrinol. Metab. 294 (5), E889–E897. doi:10.1152/ajpendo.00150.2007
Huang, S., Li, N., Liu, C., Li, T., Wang, W., Jiang, L., et al. (2019). Characteristics of the gut microbiota colonization, inflammatory profile, and plasma metabolome in intrauterine growth restricted piglets during the first 12 hours after birth. J. Microbiol. 57 (9), 748–758. doi:10.1007/s12275-019-8690-x
Hudalla, H., Karenberg, K., Kuon, R-J., Pöschl, J., Tschada, R., and Frommhold, D. (2018). LPS-induced maternal inflammation promotes fetal leukocyte recruitment and prenatal organ infiltration in mice. Pediatr. Res. 84 (5), 757–764. doi:10.1038/s41390-018-0030-z
Hyatt, M. A., Gopalakrishnan, G. S., Bispham, J., Gentili, S., McMillen, I. C., Rhind, S. M., et al. (2007). Maternal nutrient restriction in early pregnancy programs hepatic mRNA expression of growth-related genes and liver size in adult male sheep. J. Endocrinol. 192 (1), 87–97. doi:10.1677/joe.1.06801
Ikenoue, S., Kasuga, Y., Endo, T., Tanaka, M., and Ochiai, D. (2021). Newer insights into fetal growth and body composition. Front. Endocrinol. (Lausanne) 12, 708767. doi:10.3389/fendo.2021.708767
Issa, N., Lachance, G., Bellmann, K., Laplante, M., Stadler, K., and Marette, A. (2018). Cytokines promote lipolysis in 3T3-L1 adipocytes through induction of NADPH oxidase 3 expression and superoxide production. J. Lipid Res. 59 (12), 2321–2328. doi:10.1194/jlr.M086504
Iwata, S., Kinoshita, M., Okamura, H., Tsuda, K., Saikusa, M., Harada, E., et al. (2019). Intrauterine growth and the maturation process of adrenal function. PeerJ 7, e6368. doi:10.7717/peerj.6368
Jang, Y. C., Rodriguez, K., Lustgarten, M. S., Muller, F. L., Bhattacharya, A., Pierce, A., et al. (2020). Superoxide-mediated oxidative stress accelerates skeletal muscle atrophy by synchronous activation of proteolytic systems. Geroscience 42 (6), 1579–1591. doi:10.1007/s11357-020-00200-5
Jansson, N., Pettersson, J., Haafiz, A., Ericsson, A., Palmberg, I., Tranberg, M., et al. (2006). Down-regulation of placental transport of amino acids precedes the development of intrauterine growth restriction in rats fed a low protein diet. J. Physiol. 576 (Pt 3), 935–946. doi:10.1113/jphysiol.2006.116509
Jenkins, T. C., Wallace, R. J., Moate, P. J., and Mosley, E. E. (2008). Board-invited review: recent advances in biohydrogenation of unsaturated fatty acids within the rumen microbial ecosystem. J. Anim. Sci. 86 (2), 397–412. doi:10.2527/jas.2007-0588
Ji, Y., Li, M., Chang, M., Liu, R., Qiu, J., Wang, K., et al. (2022). Inflammation: roles in skeletal muscle atrophy. Antioxidants (Basel) 11 (9), 1686. doi:10.3390/antiox11091686
Joanisse, S., and Parise, G. (2016). Cytokine mediated control of muscle stem cell function. Adv. Exp. Med. Biol. 900, 27–44. doi:10.1007/978-3-319-27511-6_2
Jones, A. K., Brown, L. D., Rozance, P. J., Serkova, N. J., Hay, W. W., Friedman, J. E., et al. (2019). Differential effects of intrauterine growth restriction and a hypersinsulinemic-isoglycemic clamp on metabolic pathways and insulin action in the fetal liver. Am. J. Physiol. Regul. Integr. Comp. Physiol. 316 (5), R427–R440. doi:10.1152/ajpregu.00359.2018
Jones, A. K., Wang, D., Goldstrohm, D. A., Brown, L. D., Rozance, P. J., Limesand, S. W., et al. (2022). Tissue-specific responses that constrain glucose oxidation and increase lactate production with the severity of hypoxemia in fetal sheep. Am. J. Physiol. Endocrinol. Metab. 322 (2), E181–E196. doi:10.1152/ajpendo.00382.2021
Jones, M. L., Mark, P. J., Mori, T. A., Keelan, J. A., and Waddell, B. J. (2013). Maternal dietary omega-3 fatty acid supplementation reduces placental oxidative stress and increases fetal and placental growth in the rat. Biol. Reprod. 88 (2), 37. doi:10.1095/biolreprod.112.103754
Jonker, S. S., Kamna, D., Loturco, D., Kailey, J., and Brown, L. D. (2018). IUGR impairs cardiomyocyte growth and maturation in fetal sheep. J. Endocrinol. 239, 253–265. doi:10.1530/JOE-18-0382
Joss-Moore, L. A., Wang, Y., Baack, M. L., Yao, J., Norris, A. W., Yu, X., et al. (2010). IUGR decreases PPARγ and SETD8 Expression in neonatal rat lung and these effects are ameliorated by maternal DHA supplementation. Early Hum. Dev. 86 (12), 785–791. doi:10.1016/j.earlhumdev.2010.08.026
Juan, C. A., Pérez de la Lastra, J. M., Plou, F. J., and Pérez-Lebeña, E. (2021). The chemistry of reactive oxygen species (ROS) revisited: outlining their role in biological macromolecules (DNA, lipids and proteins) and induced pathologies. Int. J. Mol. Sci. 22 (9), 4642. doi:10.3390/ijms22094642
Kairenius, P., Leskinen, H., Toivonen, V., Muetzel, S., Ahvenjärvi, S., Vanhatalo, A., et al. (2018). Effect of dietary fish oil supplements alone or in combination with sunflower and linseed oil on ruminal lipid metabolism and bacterial populations in lactating cows. J. Dairy Sci. 101 (4), 3021–3035. doi:10.3168/jds.2017-13776
Kar, S., Wong, M., Rogozinska, E., and Thangaratinam, S. (2016). Effects of omega-3 fatty acids in prevention of early preterm delivery: A systematic review and meta-analysis of randomized studies. Eur. J. Obstet. Gynecol. Reprod. Biol. 198, 40–46. doi:10.1016/j.ejogrb.2015.11.033
Kelly, A. C., Bidwell, C. A., McCarthy, F. M., Taska, D. J., Anderson, M. J., Camacho, L. E., et al. (2017). RNA sequencing exposes adaptive and immune responses to intrauterine growth restriction in fetal sheep islets. Endocrinol 158 (4), 743–755. doi:10.1210/en.2016-1901
Kesavan, K., and Devaskar, S. U. (2019). Intrauterine growth restriction: postnatal monitoring and outcomes. Pediatr. Clin. North Am. 66 (2), 403–423. doi:10.1016/j.pcl.2018.12.009
Khan, S. A., Damanhouri, G. A., Ahmed, T. J., Halawani, S. H., Ali, A., Makki, A., et al. (2022). Omega 3 fatty acids - potential modulators for oxidative stress and inflammation in the management of sickle cell disease. J. Pediatr. (Rio J. 98 (5), 513–518. doi:10.1016/j.jped.2022.01.001
Kiecolt-Glaser, J. K., Belury, M. A., Andridge, R., Malarkey, W. B., and Glaser, R. (2011). Omega-3 supplementation lowers inflammation and anxiety in medical students: A randomized controlled trial. Brain Behav. Immun. 25 (8), 1725–1734. doi:10.1016/j.bbi.2011.07.229
Kiecolt-Glaser, J. K., Belury, M. A., Andridge, R., Malarkey, W. B., Hwang, B. S., and Glaser, R. (2012). Omega-3 supplementation lowers inflammation in healthy middle-aged and older adults: A randomized controlled trial. Brain Behav. Immun. 26 (6), 988–995. doi:10.1016/j.bbi.2012.05.011
Kim, J. S., Saengsirisuwan, V., Sloniger, J. A., Teachey, M. K., and Henriksen, E. J. (2006). Oxidant stress and skeletal muscle glucose transport: roles of insulin signaling and p38 MAPK. Free Radic. Biol. Med. 41 (5), 818–824. doi:10.1016/j.freeradbiomed.2006.05.031
Kloesz, J. L., Serdikoff, C. M., Maclennan, N. K., Adibi, S. A., and Lane, R. H. (2001). Uteroplacental insufficiency alters liver and skeletal muscle branched-chain amino acid metabolism in intrauterine growth-restricted fetal rats. Pediatr. Res. 50 (5), 604–610. doi:10.1203/00006450-200111000-00012
Kocherlakota, C., Nagaraju, B., Arjun, N., Srinath, A., Kothapalli, K. S. D., and Brenna, J. T. (2022). Inhalation of nebulized omega-3 fatty acids mitigate LPS-induced acute lung inflammation in rats: implications for treatment of COPD and COVID-19. Prostagl. Leukot. Essent. Fat. Acids 179, 102426. doi:10.1016/j.plefa.2022.102426
Köhler, A., Heinrich, J., and von Schacky, C. (2017). Bioavailability of dietary omega-3 fatty acids added to a variety of sausages in healthy individuals. Nutrients 9 (6), 629. doi:10.3390/nu9060629
Koppelmann, T., Pollak, Y., Ben-Shahar, Y., Gorelik, G., and Sukhotnik, I. (2021). The mechanisms of the anti-inflammatory and anti-apoptotic effects of omega-3 polyunsaturated fatty acids during methotrexate-induced intestinal damage in cell line and in a rat model. Nutrients 13 (3), 888. doi:10.3390/nu13030888
Krajewski, P., Sieroszewski, P., Karowicz-Bilinska, A., Kmiecik, M., Chudzik, A., Strzalko-Gloskowska, B., et al. (2014). Assessment of interleukin-6, interleukin-8 and interleukin-18 count in the serum of IUGR newborns. J. Maternal-Fetal Neonatal Med. 27 (11), 1142–1145. doi:10.3109/14767058.2013.851186
Kras, K. M., Hausman, D. B., and Martin, R. J. (2000). Tumor necrosis factor-alpha stimulates cell proliferation in adipose tissue-derived stromal-vascular cell culture: promotion of adipose tissue expansion by paracrine growth factors. Obes. Res. 8 (2), 186–193. doi:10.1038/oby.2000.20
Krawczyk, S. A., Haller, J. F., Ferrante, T., Zoeller, R. A., and Corkey, B. E. (2012). Reactive oxygen species facilitate translocation of hormone sensitive lipase to the lipid droplet during lipolysis in human differentiated adipocytes. PLoS One 7 (4), e34904. doi:10.1371/journal.pone.0034904
Kronberg, S. L., Scholljegerdes, E. J., Murphy, E. J., Ward, R. E., Maddock, T. D., and Schauer, C. S. (2012). Treatment of flaxseed to reduce biohydrogenation of α-linolenic acid by ruminal microbes in sheep and cattle, and increase n-3 fatty acid concentrations in red meat. J. Anim. Sci. 90 (12), 4618–4624. doi:10.2527/jas.2011-4774
Kuo, A. H., Li, C., Huber, H. F., Clarke, G. D., and Nathanielsz, P. W. (2018). Intrauterine growth restriction results in persistent vascular mismatch in adulthood. J. Physiol. 596 (23), 5777–5790. doi:10.1113/JP275139
Lacey, T. A. (2021). The effects of omega-3 PUFA infusions during late gestation on developmental pathologies in the intrauterine growth restricted fetus. Theses Diss. Animal Sci. 226.
Lacey, T. L., Gibbs, R. L., Most, M. S., Beer, H. N., Hicks, Z. M., Grijalva, P. C., et al. (2021). Decreased fetal biometrics and impaired β-cell function in IUGR fetal sheep are improved by daily ω-3 PUFA infusion. Transl. Anim. Sci. 5 (Suppl. ment_S1), S41–S45. doi:10.1093/tas/txab168
Lacy, P., and Stow, J. L. (2011). Cytokine release from innate immune cells: association with diverse membrane trafficking pathways. Blood 118 (1), 9–18. doi:10.1182/blood-2010-08-265892
Lane, R. H., Kelley, D. E., Gruetzmacher, E. M., and Devaskar, S. U. (2001a). Uteroplacental insufficiency alters hepatic fatty acid-metabolizing enzymes in juvenile and adult rats. Am. J. Physiol. Regul. Integr. Comp. Physiol. 280 (1), R183–R190. doi:10.1152/ajpregu.2001.280.1.R183
Lane, R. H., Kelley, D. E., Ritov, V. H., Tsirka, A. E., and Gruetzmacher, E. M. (2001b). Altered expression and function of mitochondrial beta-oxidation enzymes in juvenile intrauterine-growth-retarded rat skeletal muscle. Pediatr. Res. 50 (1), 83–90. doi:10.1203/00006450-200107000-00016
Lane, S. L., Doyle, A. S., Bales, E. S., Lorca, R. A., Julian, C. G., and Moore, L. G. (2020). Increased uterine artery blood flow in hypoxic murine pregnancy is not sufficient to prevent fetal growth restriction†. Biol. Reprod. 102 (3), 660–670. doi:10.1093/biolre/ioz208
Lang, U., Baker, R. S., Khoury, J., and Clark, K. E. (2000). Effects of chronic reduction in uterine blood flow on fetal and placental growth in the sheep. Am. J. Physiol. Regul. Integr. Comp. Physiol. 279 (1), R53–R59. doi:10.1152/ajpregu.2000.279.1.R53
Langen, R. C., Schols, A. M., Kelders, M. C., Van Der Velden, J. L., Wouters, E. F., and Janssen-Heininger, Y. M. (2002). Tumor necrosis factor-alpha inhibits myogenesis through redox-dependent and -independent pathways. Am. J. Physiol. Cell Physiol. 283 (3), C714–C721. doi:10.1152/ajpcell.00418.2001
Langen, R. C., Schols, A. M., Kelders, M. C., Wouters, E. F., and Janssen-Heininger, Y. M. (2001). Inflammatory cytokines inhibit myogenic differentiation through activation of nuclear factor-kappaB. FASEB J. 15 (7), 1169–1180. doi:10.1096/fj.00-0463
Lapillonne, A., Peretti, N., Ho, P. S., Claris, O., and Salle, B. L. (1997). Aetiology, morphology and body composition of infants born small for gestational age. Acta Paediatr. Suppl. 423, 173–176. ; discussion 7. doi:10.1111/j.1651-2227.1997.tb18406.x
Laskowska, M., Laskowska, K., Leszczyńska-Gorzelak, B., and Oleszczuk, J. (2007). Maternal and umbilical sTNF-R1 in preeclamptic pregnancies with intrauterine normal and growth retarded fetus. Hypertens. Pregnancy 26 (1), 13–21. doi:10.1080/10641950601146483
Lee, M. J., and Fried, S. K. (2012). Glucocorticoids antagonize tumor necrosis factor-alpha-stimulated lipolysis and resistance to the antilipolytic effect of insulin in human adipocytes. Am. J. Physiol. Endocrinol. Metab. 303 (9), E1126–E1133. doi:10.1152/ajpendo.00228.2012
Leos, R. A., Anderson, M. J., Chen, X., Pugmire, J., Anderson, K. A., and Limesand, S. W. (2010). Chronic exposure to elevated norepinephrine suppresses insulin secretion in fetal sheep with placental insufficiency and intrauterine growth restriction. Am. J. Physiol. Endocrinol. Metab. 298 (4), E770–E778. doi:10.1152/ajpendo.00494.2009
Li, C., Ramahi, E., Nijland, M. J., Choi, J., Myers, D. A., Nathanielsz, P. W., et al. (2013). Up-regulation of the fetal baboon hypothalamo-pituitary-adrenal axis in intrauterine growth restriction: coincidence with hypothalamic glucocorticoid receptor insensitivity and leptin receptor down-regulation. Endocrinol 154 (7), 2365–2373. doi:10.1210/en.2012-2111
Li, L., Yang, G., Shi, S., Yang, M., Liu, H., and Boden, G. (2009b). The adipose triglyceride lipase, adiponectin and visfatin are downregulated by tumor necrosis factor-alpha (TNF-alpha) in vivo. Cytokine 45 (1), 12–19. doi:10.1016/j.cyto.2008.10.006
Li, Q., Yu, Q., Na, R., and Liu, B. (2017). Omega-3 polyunsaturated fatty acids prevent murine dilated cardiomyopathy by reducing oxidative stress and cardiomyocyte apoptosis. Exp. Ther. Med. 14 (6), 6152–6158. doi:10.3892/etm.2017.5338
Li, R., Huang, H., Limesand, S. W., and Chen, X. (2021). Pancreatic islets exhibit dysregulated adaptation of insulin secretion after chronic epinephrine exposure. Curr. Issues Mol. Biol. 43 (1), 240–250. doi:10.3390/cimb43010020
Li, W., Li, B., Lv, J., Dong, L., Zhang, L., and Wang, T. (2018). Choline supplementation improves the lipid metabolism of intrauterine-growth-restricted pigs. Asian-Australas J. Anim. Sci. 31 (5), 686–695. doi:10.5713/ajas.15.0810
Li, W., Moylan, J. S., Chambers, M. A., Smith, J., and Reid, M. B. (2009a). Interleukin-1 stimulates catabolism in C2C12 myotubes. Am. J. Physiol. Cell Physiol. 297 (3), C706–C714. doi:10.1152/ajpcell.00626.2008
Limesand, S. W., Jensen, J., Hutton, J. C., and Hay, W. W. (2005). Diminished beta-cell replication contributes to reduced beta-cell mass in fetal sheep with intrauterine growth restriction. Am. J. Physiol. Regul. Integr. Comp. Physiol. 288 (5), R1297–R1305. doi:10.1152/ajpregu.00494.2004
Limesand, S. W., Rozance, P. J., Brown, L. D., and Hay, W. W. (2009). Effects of chronic hypoglycemia and euglycemic correction on lysine metabolism in fetal sheep. Am. J. Physiol. Endocrinol. Metab. 296 (4), E879–E887. doi:10.1152/ajpendo.90832.2008
Limesand, S. W., Rozance, P. J., Macko, A. R., Anderson, M. J., Kelly, A. C., and Hay, W. W. (2013). Reductions in insulin concentrations and beta-cell mass precede growth restriction in sheep fetuses with placental insufficiency. Am. J. Physiol. Endocrinol. Metab. 304 (5), E516–E523. doi:10.1152/ajpendo.00435.2012
Limesand, S. W., Rozance, P. J., Smith, D., and Hay, W. W. (2007). Increased insulin sensitivity and maintenance of glucose utilization rates in fetal sheep with placental insufficiency and intrauterine growth restriction. Am. J. Physiol. Endocrinol. Metab. 293 (6), E1716–E1725. doi:10.1152/ajpendo.00459.2007
Limesand, S. W., Rozance, P. J., Zerbe, G. O., Hutton, J. C., and Hay, W. W. (2006). Attenuated insulin release and storage in fetal sheep pancreatic islets with intrauterine growth restriction. Endocrinol 147 (3), 1488–1497. doi:10.1210/en.2005-0900
Liu, J., He, J., Yang, Y., Yu, J., Mao, X., Yu, B., et al. (2014). Effects of intrauterine growth retardation and postnatal high-fat diet on hepatic inflammatory response in pigs. Arch. Anim. Nutr. 68 (2), 111–125. doi:10.1080/1745039X.2014.897532
Liu, T., Li, R., Luo, N., Lou, P., Limesand, S. W., Yang, Y., et al. (2022). Hepatic lipid accumulation and dysregulation associate with enhanced reactive oxygen species and pro-inflammatory cytokine in low-birth-weight goats. Anim. (Basel) 12 (6), 766. doi:10.3390/ani12060766
Limesand, S. W., Rozance, P. J., Macko, A. R., Anderson, M. J., Kelly, A. C., Hay, W. W., et al. (2013). Reductions in insulin concentrations and beta-cell mass precede growth restriction in sheep fetuses with placental insufficiency. Am. J. Physiol. Endocrinol. Metab. 304 (5), E516–523. doi:10.1152/ajpendo.00435.2012
Llanos, A., Lin, Y., Mena, P., Salem, N., and Uauy, R. (2005). Infants with intrauterine growth restriction have impaired formation of docosahexaenoic acid in early neonatal life: A stable isotope study. Pediatr. Res. 58 (4), 735–740. doi:10.1203/01.PDR.0000180542.68526.A2
Llovera, M., Garcia-Martinez, C., Agell, N., Lopez-Soriano, F. J., and Argiles, J. M. (1997). TNF can directly induce the expression of ubiquitin-dependent proteolytic system in rat soleus muscles. Biochem. Biophys. Res. Commun. 230 (2), 238–241. doi:10.1006/bbrc.1996.5827
Lugrin, J., Rosenblatt-Velin, N., Parapanov, R., and Liaudet, L. (2014). The role of oxidative stress during inflammatory processes. Biol. Chem. 395 (2), 203–230. doi:10.1515/hsz-2013-0241
Lurbe, E., Carvajal, E., Torro, I., Aguilar, F., Alvarez, J., and Redon, J. (2009). Influence of concurrent obesity and low birth weight on blood pressure phenotype in youth. Hypertension 53 (6), 912–917. doi:10.1161/HYPERTENSIONAHA.109.129155
Ly, T. T., Hewitt, J., Davey, R. J., Lim, E. M., Davis, E. A., and Jones, T. W. (2011). Improving epinephrine responses in hypoglycemia unawareness with real-time continuous glucose monitoring in adolescents with type 1 diabetes. Diabetes Care 34 (1), 50–52. doi:10.2337/dc10-1042
Macko, A. R., Yates, D. T., Chen, X., Green, A. S., Kelly, A. C., Brown, L. D., et al. (2013). Elevated plasma norepinephrine inhibits insulin secretion, but adrenergic blockade reveals enhanced beta-cell responsiveness in an ovine model of placental insufficiency at 0.7 of gestation. J. Dev. Orig. Health Dis. 4 (5), 402–410. doi:10.1017/S2040174413000093
Macko, A. R., Yates, D. T., Chen, X., Shelton, L. A., Kelly, A. C., Davis, M. A., et al. (2016). Adrenal demedullation and oxygen supplementation independently increase glucose-stimulated insulin concentrations in fetal sheep with intrauterine growth restriction. Endocrinol 157 (5), 2104–2115. doi:10.1210/en.2015-1850
Madaro, L., Passafaro, M., Sala, D., Etxaniz, U., Lugarini, F., Proietti, D., et al. (2018). Denervation-activated STAT3-IL-6 signalling in fibro-adipogenic progenitors promotes myofibres atrophy and fibrosis. Nat. Cell Biol. 20 (8), 917–927. doi:10.1038/s41556-018-0151-y
Maier, A., McEwan, J. C., Dodds, K. G., Fischman, D. A., Fitzsimons, R. B., and Harris, A. J. (1992). Myosin heavy chain composition of single fibres and their origins and distribution in developing fascicles of sheep tibialis cranialis muscles. J. Musc Res. Cell Mobil. 13 (5), 551–572. doi:10.1007/BF01737997
Maliszewski, A. M., Gadhia, M. M., O'Meara, M. C., Thorn, S. R., Rozance, P. J., and Brown, L. D. (2012). Prolonged infusion of amino acids increases leucine oxidation in fetal sheep. Am. J. Physiol. Endocrinol. Metab. 302 (12), E1483–E1492. doi:10.1152/ajpendo.00026.2012
Mark, P. J., Wyrwoll, C. S., Zulkafli, I. S., Mori, T. A., and Waddell, B. J. (2014). Rescue of glucocorticoid-programmed adipocyte inflammation by omega-3 fatty acid supplementation in the rat. Reprod. Biol. Endocrinol. 12, 39. doi:10.1186/1477-7827-12-39
Marques, R. S., Cooke, R. F., Rodrigues, M. C., Brandão, A. P., Schubach, K. M., Lippolis, K. D., et al. (2017). Effects of supplementing calcium salts of polyunsaturated fatty acids to late-gestating beef cows on performance and physiological responses of the offspring. J. Anim. Sci. 95 (12), 5347–5357. doi:10.2527/jas2017.1606
Mathews, T. J., and Driscoll, A. K. (2017). Trends in infant mortality in the United States, 2005-2014. NCHS Data Brief. (279), 1–8.
Mayhew, T. M., Wijesekara, J., Baker, P. N., and Ong, S. S. (2004). Morphometric evidence that villous development and fetoplacental angiogenesis are compromised by intrauterine growth restriction but not by pre-eclampsia. Placenta 25 (10), 829–833. doi:10.1016/j.placenta.2004.04.011
Meadus, W. J., Duff, P., Uttaro, B., Aalhus, J. L., Rolland, D. C., Gibson, L. L., et al. (2010). Production of docosahexaenoic acid (DHA) enriched bacon. J. Agric. Food Chem. 58 (1), 465–472. doi:10.1021/jf9028078
Melendez, P., Roeschmann, C. F., Baudo, A., Tao, S., Pinedo, P., Kalantari, A., et al. (2022). Effect of fish oil and canola oil supplementation on immunological parameters, feed intake, and growth of Holstein calves. J. Dairy Sci. 105 (3), 2509–2520. doi:10.3168/jds.2021-21134
Mericq, V., Ong, K. K., Bazaes, R., Pena, V., Avila, A., Salazar, T., et al. (2005). Longitudinal changes in insulin sensitivity and secretion from birth to age three years in small- and appropriate-for-gestational-age children. Diabetologia 48 (12), 2609–2614. doi:10.1007/s00125-005-0036-z
Meyer, K. M., Koch, J. M., Ramadoss, J., Kling, P. J., and Magness, R. R. (2010). Ovine surgical model of uterine space restriction: interactive effects of uterine anomalies and multifetal gestations on fetal and placental growth. Biol. Reprod. 83 (5), 799–806. doi:10.1095/biolreprod.110.085381
Middleton, P., Gomersall, J. C., Gould, J. F., Shepherd, E., Olsen, S. F., and Makrides, M. (2018). Omega-3 fatty acid addition during pregnancy. Cochrane Database Syst. Rev. 11 (11), CD003402. doi:10.1002/14651858.CD003402.pub3
Milligan Bnf, D., and Kramer, D. L. (2002). Within-litter birth weight variation in the domestic pig and its relation to pre-weaning survival, weight gain, and variation in weaning weights. Livest. Prod. Sci. 76 (1-2), 181–191. doi:10.1016/s0301-6226(02)00012-x
Miranda, J., Paules, C., Nair, S., Lai, A., Palma, C., Scholz-Romero, K., et al. (2018). Placental exosomes profile in maternal and fetal circulation in intrauterine growth restriction - Liquid biopsies to monitoring fetal growth. Placenta 64, 34–43. doi:10.1016/j.placenta.2018.02.006
Morand, O., Chanez, C., Masson, M., Dumont, O., Flexor, M. A., Baumann, N., et al. (1981). Intrauterine growth retardation (malnutrition by vascular ligation) induces modifications in fatty acid composition of neurons and oligodendrocytes. J. Neurochem. 37 (4), 1057–1060. doi:10.1111/j.1471-4159.1981.tb04498.x
Morin, C. L., Schlaepfer, I. R., and Eckel, R. H. (1995). Tumor necrosis factor-alpha eliminates binding of NF-Y and an octamer-binding protein to the lipoprotein lipase promoter in 3T3-L1 adipocytes. J. Clin. Invest. 95 (4), 1684–1689. doi:10.1172/JCI117844
Most, M. S., and Yates, D. T. (2021). Inflammatory mediation of heat stress-induced growth deficits in livestock and its potential role as a target for nutritional interventions: A review. Anim. (Basel) 11 (12), 3539. doi:10.3390/ani11123539
Muller, F. L., Song, W., Liu, Y., Chaudhuri, A., Pieke-Dahl, S., Strong, R., et al. (2006). Absence of CuZn superoxide dismutase leads to elevated oxidative stress and acceleration of age-dependent skeletal muscle atrophy. Free Radic. Biol. Med. 40 (11), 1993–2004. doi:10.1016/j.freeradbiomed.2006.01.036
Nardozza, L. M., Caetano, A. C., Zamarian, A. C., Mazzola, J. B., Silva, C. P., Marcal, V. M., et al. (2017). Fetal growth restriction: current knowledge. Arch. Gynecol. Obstet. 295 (5), 1061–1077. doi:10.1007/s00404-017-4341-9
Natto, Z. S., Yaghmoor, W., Alshaeri, H. K., and Van Dyke, T. E. (2019). Omega-3 fatty acids effects on inflammatory biomarkers and lipid profiles among diabetic and cardiovascular disease patients: A systematic review and meta-analysis. Sci. Rep. 9 (1), 18867. doi:10.1038/s41598-019-54535-x
Niu, Y., He, J., Ahmad, H., Shen, M., Zhao, Y., Gan, Z., et al. (2019). Dietary curcumin supplementation increases antioxidant capacity, upregulates Nrf2 and Hmox1 levels in the liver of piglet model with intrauterine growth retardation. Nutrients 11 (12), 2978. doi:10.3390/nu11122978
Niu, Y., Zhao, Y., He, J., Yun, Y., Shen, M., Gan, Z., et al. (2021). Dietary dihydroartemisinin supplementation alleviates intestinal inflammatory injury through TLR4/NOD/NF-κB signaling pathway in weaned piglets with intrauterine growth retardation. Anim. Nutr. 7 (3), 667–678. doi:10.1016/j.aninu.2020.12.009
Ogata, Y., Nakao, T., Takahashi, K., Abe, H., Misawa, T., Urushiyama, Y., et al. (1999). Intrauterine growth retardation as a cause of perinatal mortality in Japanese black beef calves. Zentralbl Veterinarmed A 46 (6), 327–334. doi:10.1046/j.1439-0442.1999.00221.x
Oh, D. Y., Talukdar, S., Bae, E. J., Imamura, T., Morinaga, H., Fan, W., et al. (2010). GPR120 is an omega-3 fatty acid receptor mediating potent anti-inflammatory and insulin-sensitizing effects. Cell 142 (5), 687–698. doi:10.1016/j.cell.2010.07.041
Ong, K. K., Ahmed, M. L., Emmett, P. M., Preece, M. A., and Dunger, D. B. (2000). Association between postnatal catch-up growth and obesity in childhood: prospective cohort study. BMJ 320 (7240), 967–971. doi:10.1136/bmj.320.7240.967
Ong, K. K., and Dunger, D. B. (2000). Thrifty genotypes and phenotypes in the pathogenesis of type 2 diabetes mellitus. J. Pediatr. Endocrinol. Metab. 13 (Suppl. 6), 1419–1424. doi:10.1515/jpem-2000-s616
Otis, J. S., Niccoli, S., Hawdon, N., Sarvas, J. L., Frye, M. A., Chicco, A. J., et al. (2014). Pro-inflammatory mediation of myoblast proliferation. PLoS One 9 (3), e92363. doi:10.1371/journal.pone.0092363
Oyebade, A., Lifshitz, L., Lehrer, H., Jacoby, S., Portnick, Y., and Moallem, U. (2020). Saturated fat supplemented in the form of triglycerides decreased digestibility and reduced performance of dairy cows as compared to calcium salt of fatty acids. Anim 14 (5), 973–982. doi:10.1017/S1751731119002465
Padoan, A., Rigano, S., Ferrazzi, E., Beaty, B. L., Battaglia, F. C., and Galan, H. L. (2004). Differences in fat and lean mass proportions in normal and growth-restricted fetuses. Am. J. Obst Gynecol. 191 (4), 1459–1464. doi:10.1016/j.ajog.2004.06.045
Paolini, C. L., Marconi, A. M., Ronzoni, S., Di Noio, M., Fennessey, P. V., Pardi, G., et al. (2001). Placental transport of leucine, phenylalanine, glycine, and proline in intrauterine growth-restricted pregnancies. J. Clin. Endocrinol. Metab. 86 (11), 5427–5432. doi:10.1210/jcem.86.11.8036
Papandreou, V., Kavrochorianou, N., Katsoulas, T., Myrianthefs, P., Venetsanou, K., and Baltopoulos, G. (2016). Adrenergic effect on cytokine release after ex vivo healthy volunteers' whole blood LPS stimulation. Inflammation 39 (3), 1069–1075. doi:10.1007/s10753-016-0338-y
Patel, D., and Kalhan, S. (1992). Glycerol metabolism and triglyceride-fatty acid cycling in the human newborn: effect of maternal diabetes and intrauterine growth retardation. Pediatr. Res. 31 (1), 52–58. doi:10.1203/00006450-199201000-00010
Pecks, U., Bornemann, V., Klein, A., Segger, L., Maass, N., Alkatout, I., et al. (2019). Estimating fetal cholesterol synthesis rates by cord blood analysis in intrauterine growth restriction and normally grown fetuses. Lipids Health Dis. 18 (1), 185. doi:10.1186/s12944-019-1117-1
Pecks, U., Brieger, M., Schiessl, B., Bauerschlag, D. O., Piroth, D., Bruno, B., et al. (2012). Maternal and fetal cord blood lipids in intrauterine growth restriction. J. Perinat. Med. 40 (3), 287–296. doi:10.1515/jpm.2011.135
Pendleton, A. L., Antolic, A. T., Kelly, A. C., Davis, M. A., Camacho, L. E., Doubleday, K., et al. (2020). Lower oxygen consumption and Complex I activity in mitochondria isolated from skeletal muscle of fetal sheep with intrauterine growth restriction. Am. J. Physiol. Endocrinol. Metab. 319 (1), E67–e80. doi:10.1152/ajpendo.00057.2020
Pendleton, A. L., Humphreys, L. R., Davis, M. A., Camacho, L. E., Anderson, M. J., and Limesand, S. W. (2019). Increased pyruvate dehydrogenase activity in skeletal muscle of growth-restricted ovine fetuses. Am. J. Physiol. Regul. Integr. Comp. Physiol. 317 (4), R513–R520. doi:10.1152/ajpregu.00106.2019
Peterside, I. E., Selak, M. A., and Simmons, R. A. (2003). Impaired oxidative phosphorylation in hepatic mitochondria in growth-retarded rats. Am. J. Physiol. Endocrinol. Metab. 285 (6), E1258–E1266. doi:10.1152/ajpendo.00437.2002
Philipps, A. F., Rosenkrantz, T. S., Clark, R. M., Knox, I., Chaffin, D. G., and Raye, J. R. (1991). Effects of fetal insulin deficiency on growth in fetal lambs. Diabetes 40 (1), 20–27. doi:10.2337/diab.40.1.20
Pizzino, G., Irrera, N., Cucinotta, M., Pallio, G., Mannino, F., Arcoraci, V., et al. (2017). Oxidative stress: harms and benefits for human health. Oxid. Med. Cell Longev. 2017, 8416763. doi:10.1155/2017/8416763
Polányi, L., Niessen, C. M., Vohlen, C., Stinn, J., Kretschmer, T., Jentgen, V., et al. (2020). Intrauterine growth restriction induces skin inflammation, increases TSLP and impairs epidermal barrier function. J. Mol. Med. Berl. 98 (2), 279–289. doi:10.1007/s00109-019-01867-w
Ponnampalam, E. N., Sinclair, A. J., Egan, A. R., Ferrier, G. R., and Leury, B. J. (2002). Dietary manipulation of muscle long-chain omega-3 and omega-6 fatty acids and sensory properties of lamb meat. Meat Sci. 60 (2), 125–132. doi:10.1016/s0309-1740(01)00113-9
Ponnampalam, E. N., Trout, G. R., Sinclair, A. J., Egan, A. R., and Leury, B. J. (2001). Comparison of the color stability and lipid oxidative stability of fresh and vacuum packaged lamb muscle containing elevated omega-3 and omega-6 fatty acid levels from dietary manipulation. Meat Sci. 58 (2), 151–161. doi:10.1016/s0309-1740(00)00143-1
Posont, R. J., Cadaret, C. N., Barnes, T. L., and Yates, D. T. (2017). A potential role for mTORC1/2 in β2 adrenergic regulation of skeletal muscle glucose oxidation in models of intrauterine growth restriction. Diabesity 3 (3), 9–12. doi:10.15562/diabesity.2017.40
Posont, R. J., Cadaret, C. N., Beard, J. K., Swanson, R. M., Gibbs, R. L., Marks-Nelson, E. S., et al. (2021). Maternofetal inflammation induced for 2 wk in late gestation reduced birth weight and impaired neonatal growth and skeletal muscle glucose metabolism in lambs. J. Anim. Sci. 99 (5), skab102. doi:10.1093/jas/skab102
Posont, R. J., Most, M. S., Cadaret, C. N., Marks-Nelson, E. S., Beede, K. A., Limesand, S. W., et al. (2022). Primary myoblasts from intrauterine growth-restricted fetal sheep exhibit intrinsic dysfunction of proliferation and differentiation that coincides with enrichment of inflammatory cytokine signaling pathways. J. Animal Sci. 100 (8), skac145. doi:10.1093/jas/skac145
Poudel, R., McMillen, I. C., Dunn, S. L., Zhang, S., and Morrison, J. L. (2015). Impact of chronic hypoxemia on blood flow to the brain, heart, and adrenal gland in the late-gestation IUGR sheep fetus. Am. J. Physiol. Regul. Integr. Comp. Physiol. 308 (3), R151–R162. doi:10.1152/ajpregu.00036.2014
Ramakrishnan, U., Stein, A. D., Parra-Cabrera, S., Wang, M., Imhoff-Kunsch, B., Juarez-Marquez, S., et al. (2010). Effects of docosahexaenoic acid supplementation during pregnancy on gestational age and size at birth: randomized, double-blind, placebo-controlled trial in Mexico. Food Nutr. Bull. 31 (2 Suppl. l), S108–S116. doi:10.1177/15648265100312S203
Regnault, T. R., de Vrijer, B., Galan, H. L., Davidsen, M. L., Trembler, K. A., Battaglia, F. C., et al. (2003). The relationship between transplacental O2 diffusion and placental expression of PlGF, VEGF and their receptors in a placental insufficiency model of fetal growth restriction. J. Physiol. 550 (Pt 2), 641–656. doi:10.1113/jphysiol.2003.039511
Regnault, T. R., Orbus, R. J., de Vrijer, B., Davidsen, M. L., Galan, H. L., Wilkening, R. B., et al. (2002). Placental expression of VEGF, PlGF and their receptors in a model of placental insufficiency-intrauterine growth restriction (PI-IUGR). Placenta 23 (2-3), 132–144. doi:10.1053/plac.2001.0757
Reid, M. B., and Li, Y. P. (2001). Cytokines and oxidative signalling in skeletal muscle. Acta Physiol. Scand. 171 (3), 225–232. doi:10.1046/j.1365-201x.2001.00824.x
Remels, A. H., Gosker, H. R., Verhees, K. J., Langen, R. C., and Schols, A. M. (2015). TNF-α-induced NF-κB activation stimulates skeletal muscle glycolytic metabolism through activation of HIF-1α. Endocrinol 156 (5), 1770–1781. doi:10.1210/en.2014-1591
Richard, D., Kefi, K., Barbe, U., Bausero, P., and Visioli, F. (2008). Polyunsaturated fatty acids as antioxidants. Pharmacol. Res. 57 (6), 451–455. doi:10.1016/j.phrs.2008.05.002
Riddle, E. S., Campbell, M. S., Lang, B. Y., Bierer, R., Wang, Y., Bagley, H. N., et al. (2014). Intrauterine growth restriction increases TNF α and activates the unfolded protein response in male rat pups. J. Obes. 2014, 829862. doi:10.1155/2014/829862
Robinson, D. L., Cafe, L. M., and Greenwood, P. L. (2013). Meat science and muscle biology symposium: developmental programming in cattle: consequences for growth, efficiency, carcass, muscle, and beef quality characteristics. J. Anim. Sci. 91 (3), 1428–1442. doi:10.2527/jas.2012-5799
Rosa Velazquez, M., Batistel, F., Pinos Rodriguez, J. M., and Relling, A. E. (2020). Effects of maternal dietary omega-3 polyunsaturated fatty acids and methionine during late gestation on fetal growth, DNA methylation, and mRNA relative expression of genes associated with the inflammatory response, lipid metabolism and DNA methylation in placenta and offspring's liver in sheep. J. Anim. Sci. Biotechnol. 11 (1), 111. doi:10.1186/s40104-020-00513-7
Rosa-Velazquez, M., Pinos-Rodriguez, J. M., Parker, A. J., and Relling, A. E. (2022). Maternal supply of a source of omega-3 fatty acids and methionine during late gestation on the offspring’s growth, metabolism, carcass characteristic, and liver’s mRNA expression in sheep. J. Anim. Sci. 100 (4), skac032. doi:10.1093/jas/skac032
Rosario, F. J., Kramer, A., Li, C., Galan, H. L., Powell, T. L., Nathanielsz, P. W., et al. (2021). Reduction of in vivo placental amino acid transport precedes the development of intrauterine growth restriction in the non-human primate. Nutrients 13 (8), 2892. doi:10.3390/nu13082892
Ross, J. C., Fennessey, P. V., Wilkening, R. B., Battaglia, F. C., and Meschia, G. (1996). Placental transport and fetal utilization of leucine in a model of fetal growth retardation. Am. J. Physiol. 270 (3 Pt 1), E491–E503. doi:10.1152/ajpendo.1996.270.3.E491
Rotter, V., Nagaev, I., and Smith, U. (2003). Interleukin-6 (IL-6) induces insulin resistance in 3T3-L1 adipocytes and is, like IL-8 and tumor necrosis factor-alpha, overexpressed in human fat cells from insulin-resistant subjects. J. Biol. Chem. 278 (46), 45777–45784. doi:10.1074/jbc.M301977200
Rozance, P. J., Anderson, M., Martinez, M., Fahy, A., Macko, A. R., Kailey, J., et al. (2015). Placental insufficiency decreases pancreatic vascularity and disrupts hepatocyte growth factor signaling in the pancreatic islet endothelial cell in fetal sheep. Diabetes 64 (2), 555–564. doi:10.2337/db14-0462
Rozance, P. J., Crispo, M. M., Barry, J. S., O'Meara, M. C., Frost, M. S., Hansen, K. C., et al. (2009). Prolonged maternal amino acid infusion in late-gestation pregnant sheep increases fetal amino acid oxidation. Am. J. Physiol. Endocrinol. Metab. 297 (3), E638–E646. doi:10.1152/ajpendo.00192.2009
Rozance, P. J., Limesand, S. W., and Hay, W. W. (2006). Decreased nutrient stimulated insulin secretion in chronically hypoglycemic late gestation fetal sheep is due to an intrinsic islet defect. Am. J. Physiol. EndocrinolMetab 291 (2), E404–E411. doi:10.1152/ajpendo.00643.2005
Rozance, P. J., Limesand, S. W., Zerbe, G. O., and Hay, W. W. (2007). Chronic fetal hypoglycemia inhibits the later steps of stimulus-secretion coupling in pancreatic beta-cells. Am. J. Physiol. Endocrinol. Metab. 292 (5), E1256–E1264. doi:10.1152/ajpendo.00265.2006
Rozance, P. J., Wesolowski, S. R., Jonker, S. S., and Brown, L. D. (2021). Anemic hypoxemia reduces myoblast proliferation and muscle growth in late-gestation fetal sheep. Am. J. Physiol. Regul. Integr. Comp. Physiol. 321 (3), R352–R363. doi:10.1152/ajpregu.00342.2020
Rozance, P. J., and Wolfsdorf, J. I. (2019). Hypoglycemia in the newborn. Pediatr. Clin. North Am. 66 (2), 333–342. doi:10.1016/j.pcl.2018.12.004
Rozance, P. J., Zastoupil, L., Wesolowski, S. R., Goldstrohm, D. A., Strahan, B., Cree-Green, M., et al. (2018). Skeletal muscle protein accretion rates and hindlimb growth are reduced in late gestation intrauterine growth-restricted fetal sheep. J. Physiol. 596 (1), 67–82. doi:10.1113/JP275230
Rueda-Clausen, C. F., Dolinsky, V. W., Morton, J. S., Proctor, S. D., Dyck, J. R., and Davidge, S. T. (2011). Hypoxia-induced intrauterine growth restriction increases the susceptibility of rats to high-fat diet-induced metabolic syndrome. Diabetes 60 (2), 507–516. doi:10.2337/db10-1239
Russell, F. D., and Burgin-Maunder, C. S. (2012). Distinguishing health benefits of eicosapentaenoic and docosahexaenoic acids. Mar. Drugs 10 (11), 2535–2559. doi:10.3390/md10112535
Ryden, M., Arvidsson, E., Blomqvist, L., Perbeck, L., Dicker, A., and Arner, P. (2004). Targets for TNF-alpha-induced lipolysis in human adipocytes. Biochem. Biophys. Res. Commun. 318 (1), 168–175. doi:10.1016/j.bbrc.2004.04.010
Saccone, G., Berghella, V., Maruotti, G. M., Sarno, L., and Martinelli, P. (2015). Omega-3 supplementation during pregnancy to prevent recurrent intrauterine growth restriction: systematic review and meta-analysis of randomized controlled trials. Ultrasound Obstet. Gynecol. 46 (6), 659–664. doi:10.1002/uog.14910
Saccone, G., Saccone, I., and Berghella, V. (2016). Omega-3 long-chain polyunsaturated fatty acids and fish oil supplementation during pregnancy: which evidence? J. Matern. Fetal Neonatal Med. 29 (15), 2389–2397. doi:10.3109/14767058.2015.1086742
Sadiq, H. F., Das, U. G., Tracy, T. F., and Devaskar, S. U. (1999). Intra-uterine growth restriction differentially regulates perinatal brain and skeletal muscle glucose transporters. Brain Res. 823 (1-2), 96–103. doi:10.1016/s0006-8993(99)01145-2
Saker, F., Voora, D. M., Mahajan, S. D., Kilic, I., Ismail-Beigi, F., and Kalhan, S. C. (1999). Effect of reduced inspired oxygen on fetal growth and maternal glucose metabolism in rat pregnancy. Metabolism 48 (6), 738–744. doi:10.1016/s0026-0495(99)90173-7
Sandiford, S. D., Kennedy, K. A., Xie, X., Pickering, J. G., and Li, S. S. (2014). Dual oxidase maturation factor 1 (DUOXA1) overexpression increases reactive oxygen species production and inhibits murine muscle satellite cell differentiation. Cell Commun. Signal 12, 5. doi:10.1186/1478-811X-12-5
Sharma, R., Maimanuku, L. R., Morse, Z., and Pack, A. R. (2007). Preterm low birth weights associated with periodontal disease in the Fiji Islands. Int. Dent. J. 57 (4), 257–260. doi:10.1111/j.1875-595x.2007.tb00129.x
Sandoval, C., Lambo, C. A., Beason, K., Dunlap, K. A., and Satterfield, M. C. (2020). Effect of maternal nutrient restriction on skeletal muscle mass and associated molecular pathways in SGA and Non-SGA sheep fetuses. Domest. Anim. Endocrinol. 72, 106443. doi:10.1016/j.domaniend.2020.106443
Schmocker, C., Weylandt, K. H., Kahlke, L., Wang, J., Lobeck, H., Tiegs, G., et al. (2007). Omega-3 fatty acids alleviate chemically induced acute hepatitis by suppression of cytokines. Hepatology 45 (4), 864–869. doi:10.1002/hep.21626
Sciancalepore, M., Luin, E., Parato, G., Ren, E., Giniatullin, R., Fabbretti, E., et al. (2012). Reactive oxygen species contribute to the promotion of the ATP-mediated proliferation of mouse skeletal myoblasts. Free Radic. Biol. Med. 53 (7), 1392–1398. doi:10.1016/j.freeradbiomed.2012.08.002
Shahidi, F., and Ambigaipalan, P. (2018). Omega-3 polyunsaturated fatty acids and their health benefits. Annu. Rev. Food Sci. Technol. 9, 345–381. doi:10.1146/annurev-food-111317-095850
Sharkey, D., Symonds, M. E., and Budge, H. (2009). Adipose tissue inflammation: developmental ontogeny and consequences of gestational nutrient restriction in offspring. Endocrinol 150 (8), 3913–3920. doi:10.1210/en.2008-1784
Sharma, D., Shastri, S., Farahbakhsh, N., and Sharma, P. (2016). Intrauterine growth restriction - part 1. J. Matern. Fetal Neonatal Med. 29 (24), 3977–3987. doi:10.3109/14767058.2016.1152249
Shibata, E., Hubel, C. A., Powers, R. W., von Versen-Hoeynck, F., Gammill, H., Rajakumar, A., et al. (2008). Placental system A amino acid transport is reduced in pregnancies with small for gestational age (SGA) infants but not in preeclampsia with SGA infants. Placenta 29 (10), 879–882. doi:10.1016/j.placenta.2008.07.001
Shingfield, K. J., Lee, M. R., Humphries, D. J., Scollan, N. D., Toivonen, V., Reynolds, C. K., et al. (2010). Effect of incremental amounts of fish oil in the diet on ruminal lipid metabolism in growing steers. Br. J. Nutr. 104 (1), 56–66. doi:10.1017/S0007114510000292
Shoji, H., Watanabe, A., Awaji, A., Ikeda, N., Hosozawa, M., Ohkawa, N., et al. (2020). Intrauterine growth restriction affects z-scores of anthropometric parameters during the first 6 years in very low-birth-weight-children born at less than 30 weeks of gestation. J. Dev. Orig. Health Dis. 11 (1), 44–48. doi:10.1017/S2040174419000369
Soeters, P. B., Shenkin, A., Sobotka, L., Soeters, M. R., de Leeuw, P. W., and Wolfe, R. R. (2021). The anabolic role of the Warburg, Cori-cycle and Crabtree effects in health and disease. Clin. Nutr. 40 (5), 2988–2998. doi:10.1016/j.clnu.2021.02.012
Souza, L. M., Cruz, S. S., Gomes-Filho, I. S., Amico, P., Barreto, M. L, Passos-Soares, J. S., et al. (2016). Effect of maternal periodontitis and low birth weight—a case control study. Acta Odontol Scand 74 (1), 73–80. doi:10.3109/00016357.2015.1049374
Soto, S. M., Blake, A. C., Wesolowski, S. R., Rozance, P. J., Barthel, K. B., Gao, B., et al. (2017). Myoblast replication is reduced in the IUGR fetus despite maintained proliferative capacity in vitro. J. Endocrinol. 232 (3), 475–491. doi:10.1530/JOE-16-0123
Sriram, S., Subramanian, S., Sathiakumar, D., Venkatesh, R., Salerno, M. S., McFarlane, C. D., et al. (2011). Modulation of reactive oxygen species in skeletal muscle by myostatin is mediated through NF-κB. Aging Cell 10 (6), 931–948. doi:10.1111/j.1474-9726.2011.00734.x
Steyn, P. J., Dzobo, K., Smith, R. I., and Myburgh, K. H. (2019). Interleukin-6 induces myogenic differentiation via JAK2-STAT3 signaling in mouse C2C12 myoblast cell line and primary human myoblasts. Int. J. Mol. Sci. 20 (21), 5273. doi:10.3390/ijms20215273
Stremming, J., Chang, E. I., Knaub, L. A., Armstrong, M. L., Baker, P. R., Wesolowski, S. R., et al. (2022). Lower citrate synthase activity, mitochondrial complex expression, and fewer oxidative myofibers characterize skeletal muscle from growth-restricted fetal sheep. Am. J. Physiol. Regul. Integr. Comp. Physiol. 322 (3), R228–R240. doi:10.1152/ajpregu.00222.2021
Stremming, J., Heard, S., White, A., Chang, E. I., Shaw, S. C., Wesolowski, S. R., et al. (2021). IGF-1 infusion to fetal sheep increases organ growth but not by stimulating nutrient transfer to the fetus. Am. J. Physiol. Endocrinol. Metab. 320 (3), E527–E538. doi:10.1152/ajpendo.00453.2020
Stremming, J., Jansson, T., Powell, T. L., Rozance, P. J., and Brown, L. D. (2020). Reduced Na(+) K(+) -ATPase activity may reduce amino acid uptake in intrauterine growth restricted fetal sheep muscle despite unchanged ex vivo amino acid transporter activity. J. Physiol. 598 (8), 1625–1639. doi:10.1113/JP278933
Styrud, J., Eriksson, U. J., Grill, V., and Swenne, I. (2005). Experimental intrauterine growth retardation in the rat causes a reduction of pancreatic B-cell mass, which persists into adulthood. Biol. Neonate 88 (2), 122–128. doi:10.1159/000086136
Sun, L., Zhang, H., Wang, Z., Fan, Y., Guo, Y., and Wang, F. (2018). Dietary rumen-protected arginine and N-carbamylglutamate supplementation enhances fetal growth in underfed ewes. Reprod. Fertil. Dev. 30 (8), 1116–1127. doi:10.1071/RD17164
Sutherland, A. E., Crossley, K. J., Allison, B. J., Jenkin, G., Wallace, E. M., and Miller, S. L. (2012). The effects of intrauterine growth restriction and antenatal glucocorticoids on ovine fetal lung development. Pediatr. Res. 71 (6), 689–696. doi:10.1038/pr.2012.19
Tang, X., and Xiong, K. (2022). Intrauterine growth retardation affects intestinal health of suckling piglets via altering intestinal antioxidant capacity, glucose uptake, tight junction, and immune responses. Oxid. Med. Cell Longev. 2022, 2644205. doi:10.1155/2022/2644205
Tarry-Adkins, J. L., Fernandez-Twinn, D. S., Hargreaves, I. P., Neergheen, V., Aiken, C. E., Martin-Gronert, M. S., et al. (2016). Coenzyme Q10 prevents hepatic fibrosis, inflammation, and oxidative stress in a male rat model of poor maternal nutrition and accelerated postnatal growth. Am. J. Clin. Nutr. 103 (2), 579–588. doi:10.3945/ajcn.115.119834
Teasdale, F. (1984). Idiopathic intrauterine growth retardation: histomorphometry of the human placenta. Placenta 5 (1), 83–92. doi:10.1016/s0143-4004(84)80051-x
Thaler, I., Manor, D., Itskovitz, J., Rottem, S., Levit, N., Timor-Tritsch, I., et al. (1990). Changes in uterine blood flow during human pregnancy. Am. J. Obstet. Gynecol. 162 (1), 121–125. doi:10.1016/0002-9378(90)90834-t
Thorn, S. R., Brown, L. D., Rozance, P. J., Hay, W. W., and Friedman, J. E. (2013). Increased hepatic glucose production in fetal sheep with intrauterine growth restriction is not suppressed by insulin. Diabetes 62 (1), 65–73. doi:10.2337/db11-1727
Thorn, S. R., Regnault, T. R., Brown, L. D., Rozance, P. J., Keng, J., Roper, M., et al. (2009). Intrauterine growth restriction increases fetal hepatic gluconeogenic capacity and reduces messenger ribonucleic acid translation initiation and nutrient sensing in fetal liver and skeletal muscle. Endocrinol 150 (7), 3021–3030. doi:10.1210/en.2008-1789
Thureen, P. J., Trembler, K. A., Meschia, G., Makowski, E. L., and Wilkening, R. B. (1992). Placental glucose transport in heat-induced fetal growth retardation. Am. J. Physiol. 263 (3 Pt 2), R578–R585. doi:10.1152/ajpregu.1992.263.3.R578
Tracey, K. J., and Cerami, A. (1993). Tumor necrosis factor, other cytokines and disease. Annu. Rev. Cell Biol. 9, 317–343. doi:10.1146/annurev.cb.09.110193.001533
Tree, K., Viemari, J. C., Cayetanot, F., and Peyronnet, J. (2016). Growth restriction induced by chronic prenatal hypoxia affects breathing rhythm and its pontine catecholaminergic modulation. J. Neurophysiol. 116 (4), 1654–1662. doi:10.1152/jn.00869.2015
Trinh, B., Peletier, M., Simonsen, C., Plomgaard, P., Karstoft, K., Klarlund Pedersen, B., et al. (2021). Blocking endogenous IL-6 impairs mobilization of free fatty acids during rest and exercise in lean and obese men. Cell Rep. Med. 2 (9), 100396. doi:10.1016/j.xcrm.2021.100396
Turrens, J. F. (2003). Mitochondrial formation of reactive oxygen species. J. Physiol. 552 (Pt 2), 335–344. doi:10.1113/jphysiol.2003.049478
van Hall, G., Steensberg, A., Sacchetti, M., Fischer, C., Keller, C., Schjerling, P., et al. (2003). Interleukin-6 stimulates lipolysis and fat oxidation in humans. J. Clin. Endocrinol. Metab. 88 (7), 3005–3010. doi:10.1210/jc.2002-021687
Vatansever, L., Kurt, E., Enser, M., Nute, G., Scollan, N., Wood, J., et al. (2000). Shelf life and eating quality of beef from cattle of different breeds given diets differing in n-3 polyunsaturated fatty acid composition. Animal Sci. 71 (3), 471–482. doi:10.1017/s135772980005548x
Velten, M., Britt, R. D., Heyob, K. M., Tipple, T. E., and Rogers, L. K. (2014). Maternal dietary docosahexaenoic acid supplementation attenuates fetal growth restriction and enhances pulmonary function in a newborn mouse model of perinatal inflammation. J. Nutr. 144 (3), 258–266. doi:10.3945/jn.113.179259
Vileisis, R. A. (1985). Effect of maternal oxygen inhalation on the fetus with growth retardation. Pediatr. Res. 19 (3), 324–327. doi:10.1203/00006450-198503000-00015
Vileisis, R. A., Fain, J., and Oh, W. (1982). Fatty acid synthesis in rat fetuses with intrauterine growth retardation. Metabolism 31 (3), 217–222. doi:10.1016/0026-0495(82)90056-7
Vítor, A. C. M., Francisco, A. E., Silva, J., Pinho, M., Huws, S. A., Santos-Silva, J., et al. (2021). Freeze-dried Nannochloropsis oceanica biomass protects eicosapentaenoic acid (EPA) from metabolization in the rumen of lambs. Sci. Rep. 11 (1), 21878. doi:10.1038/s41598-021-01255-w
Voggel, J., Fink, G., Zelck, M., Wohlfarth, M., Post, J. M., Bindila, L., et al. (2022). Elevated n-3/n-6 PUFA ratio in early life diet reverses adverse intrauterine kidney programming in female rats. J. Lipid Res. 63 (11), 100283. doi:10.1016/j.jlr.2022.100283
Vuguin, P., Raab, E., Liu, B., Barzilai, N., and Simmons, R. (2004). Hepatic insulin resistance precedes the development of diabetes in a model of intrauterine growth retardation. Diabetes 53 (10), 2617–2622. doi:10.2337/diabetes.53.10.2617
Wai, S. G., Rozance, P. J., Wesolowski, S. R., Hay, W. W., and Brown, L. D. (2018). Prolonged amino acid infusion into intrauterine growth-restricted fetal sheep increases leucine oxidation rates. Am. J. Physiol. Endocrinol. Metab. 315 (6), E1143–E1153. doi:10.1152/ajpendo.00128.2018
Wallace, J. M., Bourke, D. A., Aitken, R. P., Milne, J. S., and Hay, W. W. (2003). Placental glucose transport in growth-restricted pregnancies induced by overnourishing adolescent sheep. J. Physiol. 547 (Pt 1), 85–94. doi:10.1113/jphysiol.2002.023333
Wallace, J. M., Milne, J. S., Aitken, B. W., Aitken, R. P., and Adam, C. L. (2020). Ovine prenatal growth-restriction and sex influence fetal adipose tissue phenotype and impact postnatal lipid metabolism and adiposity in vivo from birth until adulthood. PLoS One 15 (2), e0228732. doi:10.1371/journal.pone.0228732
Wallace, J. M., Milne, J. S., Aitken, R. P., and Adam, C. L. (2014). Influence of birth weight and gender on lipid status and adipose tissue gene expression in lambs. J. Mol. Endocrinol. 53 (1), 131–144. doi:10.1530/JME-14-0123
Wallace, J. M., Milne, J. S., Matsuzaki, M., and Aitken, R. P. (2008). Serial measurement of uterine blood flow from mid to late gestation in growth restricted pregnancies induced by overnourishing adolescent sheep dams. Placenta 29 (8), 718–724. doi:10.1016/j.placenta.2008.05.006
Wallace, J. M., Regnault, T. R., Limesand, S. W., Hay, W. W., and Anthony, R. V. (2005). Investigating the causes of low birth weight in contrasting ovine paradigms. J. Physiol. 565 (Pt 1), 19–26. doi:10.1113/jphysiol.2004.082032
Wang, D. T., Yin, Y., Yang, Y. J., Lv, P. J., Shi, Y., Lu, L., et al. (2014). Resveratrol prevents TNF-alpha-induced muscle atrophy via regulation of Akt/mTOR/FoxO1 signaling in C2C12 myotubes. Int. Immunopharmacol. 19 (2), 206–213. doi:10.1016/j.intimp.2014.02.002
Wang, J., Zhu, P., Zheng, X., Ma, Z., Cui, C., Wu, C., et al. (2022). Altered liver metabolism, mitochondrial function, oxidative status, and inflammatory response in intrauterine growth restriction piglets with different growth patterns before weaning. Metabolites 12 (11), 1053. doi:10.3390/metabo12111053
Wang, T., Liu, C., Feng, C., Wang, X., Lin, G., Zhu, Y., et al. (2013). IUGR alters muscle fiber development and proteome in fetal pigs. Front. Biosci. (Landmark Ed. 18 (2), 598–607. doi:10.2741/4123
Wang, X., Martin, G. B., Liu, S., Shi, B., Guo, X., Zhao, Y., et al. (2019). The mechanism through which dietary supplementation with heated linseed grain increases n-3 long-chain polyunsaturated fatty acid concentration in subcutaneous adipose tissue of cashmere kids. J. Anim. Sci. 97 (1), 385–397. doi:10.1093/jas/sky386
Weldon, S. M., Mullen, A. C., Loscher, C. E., Hurley, L. A., and Roche, H. M. (2007). Docosahexaenoic acid induces an anti-inflammatory profile in lipopolysaccharide-stimulated human THP-1 macrophages more effectively than eicosapentaenoic acid. J. Nutr. Biochem. 18 (4), 250–258. doi:10.1016/j.jnutbio.2006.04.003
Whitlock, L. A., Schingoethe, D. J., Hippen, A. R., Kalscheur, K. F., Baer, R. J., Ramaswamy, N., et al. (2002). Fish oil and extruded soybeans fed in combination increase conjugated linoleic acids in milk of dairy cows more than when fed separately. J. Dairy Sci. 85 (1), 234–243. doi:10.3168/jds.S0022-0302(02)74072-1
Wieland, L. S. (2019). Omega-3 fatty acid addition during pregnancy: summary of a Cochrane review. Explore (NY) 15 (2), 168–169. doi:10.1016/j.explore.2018.12.005
Wietrak, E., Kaminski, K., Leszczynska-Gorzelak, B., and Oleszczuk, J. (2015). Effect of docosahexaenoic acid on apoptosis and proliferation in the placenta: preliminary report. Biomed. Res. Int. 2015, 482875. doi:10.1155/2015/482875
Wilson, S. J., McEwan, J. C., Sheard, P. W., and Harris, A. J. (1992). Early stages of myogenesis in a large mammal: formation of successive generations of myotubes in sheep tibialis cranialis muscle. J. Musc Res. Cell Mobil. 13 (5), 534–550. doi:10.1007/BF01737996
Wu, G., Bazer, F. W., Wallace, J. M., and Spencer, T. E. (2006). Board-invited review: intrauterine growth retardation: implications for the animal sciences. J. Anim. Sci. 84 (9), 2316–2337. doi:10.2527/jas.2006-156
Wu, G., Brouckaert, P., and Olivecrona, T. (2004). Rapid downregulation of adipose tissue lipoprotein lipase activity on food deprivation: evidence that TNF-alpha is involved. Am. J. Physiol. Endocrinol. Metab. 286 (5), E711–E717. doi:10.1152/ajpendo.00257.2003
Wyrwoll, C. S., Mark, P. J., Mori, T. A., Puddey, I. B., and Waddell, B. J. (2006). Prevention of programmed hyperleptinemia and hypertension by postnatal dietary omega-3 fatty acids. Endocrinol 147 (1), 599–606. doi:10.1210/en.2005-0748
Xiao, Y., Zhang, Q., Liao, X., Elbelt, U., and Weylandt, K. H. (2022). The effects of omega-3 fatty acids in type 2 diabetes: A systematic review and meta-analysis. Prostagl. Leukot. Essent. Fat. Acids 182, 102456. doi:10.1016/j.plefa.2022.102456
Xing, Y., Zhang, J., Wei, H., Zhang, H., Guan, Y., Wang, X., et al. (2019). Reduction of the PI3K/Akt related signaling activities in skeletal muscle tissues involves insulin resistance in intrauterine growth restriction rats with catch-up growth. PLoS One 14 (5), e0216665. doi:10.1371/journal.pone.0216665
Xiao, X. M., and Li, L. P. (2005). L-Arginine treatment for asymmetric fetal growth restriction. Int. J. Gynaecol. Obstet. 88 (1), 15–18. doi:10.1016/j.ijgo.2004.09.017
Xu, P., Guo, H., Wang, H., Lee, S. C., Liu, M., Pan, Y., et al. (2019). Downregulations of placental fatty acid transporters during cadmium-induced fetal growth restriction. Toxicology 423, 112–122. doi:10.1016/j.tox.2019.05.013
Yamasaki, H., Yamaguchi, Y., Takino, H., Matsuo, H., Matsumoto, K., Uotani, S., et al. (1996). TNF-alpha stimulates glucose uptake in L6 myoblasts. Diabetes Res. Clin. Pract. 32 (1-2), 11–18. doi:10.1016/0168-8227(96)01221-1
Yates, D., Green, A., and Limesand, S. W. (2011). Catecholamines mediate multiple fetal adaptations during placental insufficiency that contribute to intrauterine growth restriction: lessons from hyperthermic sheep. J. pregnancy 2011, 740408. doi:10.1155/2011/740408
Yates, D. T., Macko, A. R., Chen, X., Green, A. S., Kelly, A. C., Anderson, M. J., et al. (2012a). Hypoxaemia-induced catecholamine secretion from adrenal chromaffin cells inhibits glucose-stimulated hyperinsulinaemia in fetal sheep. J. Physiol. 590 (21), 5439–5447. doi:10.1113/jphysiol.2012.237347
Yates, D., Macko, A., Nearing, M., Chen, X., Rhoads, R., and Limesand, S. W. (2012b). Developmental programming in response to intrauterine growth restriction impairs myoblast function and skeletal muscle metabolism. J. pregnancy 2012, 631038. doi:10.1155/2012/631038
Yates, D. T., Cadaret, C. N., Beede, K. A., Riley, H. E., Macko, A. R., Anderson, M. J., et al. (2016). Intrauterine growth-restricted sheep fetuses exhibit smaller hindlimb muscle fibers and lower proportions of insulin-sensitive Type I fibers near term. Am. J. Physiol. Regul. Integr. Comp. Physiol. 310 (11), R1020–R1029. doi:10.1152/ajpregu.00528.2015
Yates, D. T., Camacho, L. E., Kelly, A. C., Steyn, L. V., Davis, M. A., Antolic, A. T., et al. (2019). Postnatal β2 adrenergic treatment improves insulin sensitivity in lambs with IUGR but not persistent defects in pancreatic islets or skeletal muscle. J. Physiol. 597 (24), 5835–5858. doi:10.1113/JP278726
Yates, D. T., Clarke, D. S., Macko, A. R., Anderson, M. J., Shelton, L. A., Nearing, M., et al. (2014). Myoblasts from intrauterine growth-restricted sheep fetuses exhibit intrinsic deficiencies in proliferation that contribute to smaller semitendinosus myofibres. J. Physiol. 592 (14), 3113–3125. doi:10.1113/jphysiol.2014.272591
Yates, D. T., Petersen, J. L., Schmidt, T. B., Cadaret, C. N., Barnes, T. L., Posont, R. J., et al. (2018). ASAS-SSR triennnial reproduction symposium: looking back and moving forward-how reproductive Physiology has evolved: fetal origins of impaired muscle growth and metabolic dysfunction: lessons from the heat-stressed pregnant Ewe. J. Anim. Sci. 96 (7), 2987–3002. doi:10.1093/jas/sky164
Yee, J. K., Han, G., Vega, J., Lee, W. P., Ross, M. G., and Desai, M. (2016). Fatty acid de Novo synthesis in adult intrauterine growth-restricted offspring, and adult male response to a high fat diet. Lipids 51 (12), 1339–1351. doi:10.1007/s11745-016-4199-9
Ying, Z., Zhang, H., Su, W., Zhou, L., Wang, F., Li, Y., et al. (2017). Dietary methionine restriction alleviates hyperglycemia in pigs with intrauterine growth restriction by enhancing hepatic protein kinase B signaling and glycogen synthesis. J. Nutr. 147 (10), 1892–1899. doi:10.3945/jn.117.253427
Youd, J. M., Rattigan, S., and Clark, M. G. (2000). Acute impairment of insulin-mediated capillary recruitment and glucose uptake in rat skeletal muscle in vivo by TNF-alpha. Diabetes 49 (11), 1904–1909. doi:10.2337/diabetes.49.11.1904
Youssef, L., Simões, R. V., Miranda, J., García-Martín, M. L., Paules, C., Crovetto, F., et al. (2021). Paired maternal and fetal metabolomics reveal a differential fingerprint in preeclampsia versus fetal growth restriction. Sci. Rep. 11 (1), 14422. doi:10.1038/s41598-021-93936-9
Zarate, M. A., De Dios, R. K., Balasubramaniyan, D., Zheng, L., Sherlock, L. G., Rozance, P. J., et al. (2021). The acute hepatic NF-κB-Mediated proinflammatory response to endotoxemia is attenuated in intrauterine growth-restricted newborn mice. Front. Immunol. 12, 706774. doi:10.3389/fimmu.2021.706774
Zhang, H., Zhang, Y., Ma, Y., Elsabagh, M., Wang, H., and Wang, M. (2021). Dietary rumen-protected L-arginine or N-carbamylglutamate attenuated fetal hepatic inflammation in undernourished ewes suffering from intrauterine growth restriction. Anim. Nutr. 7 (4), 1095–1104. doi:10.1016/j.aninu.2021.06.004
Zhang, L., Xiao, D., and Bouslough, D. B. (1998). Long-term high-altitude hypoxia increases plasma nitrate levels in pregnant ewes and their fetuses. Am. J. Obstet. Gynecol. 179 (6 Pt 1), 1594–1598. doi:10.1016/s0002-9378(98)70031-6
Zhang, Y., Jin, W., Zhang, D., Lin, C., He, H., Xie, F., et al. (2022). TNF-Α antagonizes the effect of leptin on insulin secretion through FOXO1-dependent transcriptional suppression of LepRb in INS-1 cells. Oxid. Med. Cell Longev. 2022, 9142798. doi:10.1155/2022/9142798
Zhong, X., Li, W., Huang, X., Zhang, L., Yimamu, M., Raiput, N., et al. (2012). Impairment of cellular immunity is associated with overexpression of heat shock protein 70 in neonatal pigs with intrauterine growth retardation. Cell Stress Chaperones 17 (4), 495–505. doi:10.1007/s12192-012-0326-6
Zinkhan, E. K., Yu, B., Callaway, C. W., and McKnight, R. A. (2018). Intrauterine growth restriction combined with a maternal high-fat diet increased adiposity and serum corticosterone levels in adult rat offspring. J. Dev. Orig. Health Dis. 9 (3), 315–328. doi:10.1017/S2040174418000016
Keywords: adaptive fetal programming, developmental origins of health and disease, DOHAD, fetal growth restriction, intrauterine growth restriction, IUGR, low birthweight, metabolic programming
Citation: White MR and Yates DT (2023) Dousing the flame: reviewing the mechanisms of inflammatory programming during stress-induced intrauterine growth restriction and the potential for ω-3 polyunsaturated fatty acid intervention. Front. Physiol. 14:1250134. doi: 10.3389/fphys.2023.1250134
Received: 29 June 2023; Accepted: 14 August 2023;
Published: 01 September 2023.
Edited by:
Claire Stenhouse, The Pennsylvania State University (PSU), United StatesReviewed by:
Amelia Tanner, University of Colorado Anschutz Medical Campus, United StatesCopyright © 2023 White and Yates. This is an open-access article distributed under the terms of the Creative Commons Attribution License (CC BY). The use, distribution or reproduction in other forums is permitted, provided the original author(s) and the copyright owner(s) are credited and that the original publication in this journal is cited, in accordance with accepted academic practice. No use, distribution or reproduction is permitted which does not comply with these terms.
*Correspondence: Dustin T. Yates, ZHVzdGluLnlhdGVzQHVubC5lZHU=
Disclaimer: All claims expressed in this article are solely those of the authors and do not necessarily represent those of their affiliated organizations, or those of the publisher, the editors and the reviewers. Any product that may be evaluated in this article or claim that may be made by its manufacturer is not guaranteed or endorsed by the publisher.
Research integrity at Frontiers
Learn more about the work of our research integrity team to safeguard the quality of each article we publish.