- 1Sports Coaching College, Beijing Sports University, Beijing, China
- 2Beijing Sport University, Beijing, China
- 3Capital Institute of Physical Education and Sports, Beijing, Beijing, China
Objective: This study aimed to compare and rank the effect of hypoxic practices on maximum oxygen consumption (VO2max) in athletes and determine the hypoxic dose-response correlation using network meta-analysis.
Methods: The Web of Science, PubMed, EMBASE, and EBSCO databases were systematically search for randomized controlled trials on the effect of hypoxc interventions on the VO2max of athletes published from inception until 21 February 2023. Studies that used live-high train-high (LHTH), live-high train-low (LHTL), live-high, train-high/low (HHL), intermittent hypoxic training (IHT), and intermittent hypoxic exposure (IHE) interventions were primarily included. LHTL was further defined according to the type of hypoxic environment (natural and simulated) and the altitude of the training site (low altitude and sea level). A meta-analysis was conducted to determine the standardized mean difference between the effects of various hypoxic interventions on VO2max and dose-response correlation. Furthermore, the hypoxic dosage of the different interventions were coordinated using the “kilometer hour” model.
Results: From 2,072 originally identified titles, 59 studies were finally included in this study. After data pooling, LHTL, LHTH, and IHT outperformed normoxic training in improving the VO2max of athletes. According to the P-scores, LHTL combined with low altitude training was the most effective intervention for improving VO2max (natural: 0.92 and simulated: 0.86) and was better than LHTL combined with sea level training (0.56). A reasonable hypoxic dose range for LHTH (470–1,130 kmh) and HL (500–1,415 kmh) was reported with an inverted U-shaped curve relationship.
Conclusion: Different types of hypoxic training compared with normoxic training serve as significant approaches for improving aerobic capacity in athletes. Regardless of the type of hypoxic training and the residential condition, LHTL with low altitude training was the most effective intervention. The characteristics of the dose-effect correlation of LHTH and LHTL may be associated with the negative effects of chronic hypoxia.
Introduction
Altitude training (AT) has been employed by well-trained athletes for almost 60 years since the 1968 Mexico Olympics (Buskirk et al., 1967; Wilber, 2007; Girard et al., 2023). Currently, this method has led to the development of several matured variants following the conventional “live-high train-high” approach (HH), i.e., living and training at low or moderate altitudes throughout the day (Buskirk et al., 1967). In contrast to the HH approach, the training site for “live-high train-low” (HL) is set at sea level or low altitudes (Levine and Stray-Gundersen, 1997; Bonetti and Hopkins, 2009). Besides, given the type of hypoxia in residential environments, HL can be simulated and natural (Coppel et al., 2015; Girard et al., 2023). However, considering the time involved in HH and HL training, IHE has induced wide attention over the past few years for its shorter but more intense hypoxia protocols during rest (Millet et al., 2010; Wilber, 2007). Training under short-term hypoxia is defined as an LLTH practice, which includes varying forms (e.g., intermittent hypoxic training [IHT]) (McLean et al., 2014). Live-high and train-high/low (HHL) has been reported as a novel hypoxic combination that is implemented during the application of HL, where athletes train under normoxic and hypoxic conditions (Millet et al., 2010).
Although AT has been extensively applied and is trusted by coaches and athletes (Daniels and Oldridge, 1970; Solli et al., 2017), its effectiveness on aerobic performance remains unclear (Millet and Brocherie, 2020; Siebenmann and Dempsey, 2020). In general, the ambiguity originates from two aspects: 1) Potential negative effects of chronic hypoxia exposure (various types of acute altitude sickness) (Imray et al., 2010; Schommer et al., 2012), which outweighs potential benefits and 2) unreasonable hypoxic doses (Wilber et al., 2007). Given the high cost of AT, its effectiveness should be determined, and a reasonable dosage range should be set. Although existing meta-analyses and dose-effect research have partially addressed the above-mentioned issues, most may contain flaws and controversies.
1. The above-described studies were inconclusive since there rarely was direct evidence on the comparison of different types of AT and since they failed to report a clear hierarchy (Bonetti and Hopkins, 2009; Hamlin et al., 2018; Lobigs et al., 2018)
2. Existing research has suggested that exposure to altitudes above 299 m negatively affects endurance performance; The risk of negative effects arising from hypoxic exposure becomes higher as the elevation rises, particularly above 1,000 m (Hamlin et al., 2015). However, most existing research has overlooked the actual hypoxic effect at low altitudes, and none of them has considered the potential differences in total hypoxic dose and benefits according to different training altitudes, including low altitude and sea level training sites of HL (Bonetti and Hopkins, 2009; Lancaster and Smart, 2012; Gore et al., 2013).
3. The continuity and severity of exposure are critical determinants of effective hypoxic dosage, and the deficiency in the study by Gore et al. lies in exploring the hypoxic dose-effect relationship only at a fixed altitude (Gore et al., 2013). The “kilometer hour” model proposed by Laura et al. can address the above limitation (Garvican-Lewis et al., 2016).
Accordingly, to fill the gap in comparing the effect of a wide variety of AT variants on athletes’ aerobic performance and explore reasonable hypoxic dose ranges, a network meta-analysis was conducted in this study. We aimed to compare and rank different hypoxic types for the improvement of VO2max in athletes. Subsequently, a novel technique (Bayesian model-based dose-response network meta-analysis) was adopted to examine the dose-response correlation between hypoxic doses and VO2max in athletes.
Methods
This review followed the Preferred Reporting Items for Systematic Reviews and Meta-Analyses for Network Meta-Analyses (PRISMA-NMA) guidelines (Shamseer et al., 2015). Moreover, this study is registered with PROSPERO (registration number: CRD42023401488).
Search strategy
The Web of Science, PubMed, EMBASE, and EBSCO databases were systematically searched for eligible studies published from database inception until 21 February 2023. The search terms [(hypoxia OR altitude OR hypoxic) AND (maximal oxygen consumption OR maximal oxygen uptake OR peak oxygen uptake OR maximal aerobic capacity)] were used for the search in “all fields,” whereas the terms (patients OR obese) were excluded (using NOT). Additional trials were retrieved from the cited references of relevant published meta-analyses and systematic reviews.
Inclusion and exclusion criteria
The inclusion criteria were as follows: 1) randomized and non-randomized controlled trials with at least one group completing hypoxic training (this was to increase generalizability and allow for a wider range of evidence); 2) studies that included athletes without a history of smoking, injuries, and hypoxic experience during the past 6 months; 3) studies that used only AT without additional intervention (e.g., blood flow restriction or heat environment); and 4) studies with outcomes including maximal aerobic capacity direct testing/not estimated performed at or near sea level.
The exclusion criteria were as follows: studies that were not available in English; that were conference abstracts, experience, comments, animal, or case experiments; with populations that were non-athletes; with irrelevant outcomes (e.g., disease, oxidative disorders, and sleep quality); and that assessed acute effects of altitude/hypoxic conditions.
Data extraction and quality evaluation
Studies that met the inclusion criteria were independently selected by two researchers. Subsequently, the main text and Supplementary Material S1 of the selected articles were independently reviewed to extract relevant information from the included trials. Moreover, the extracted studies were evaluated and rated by three reviewers based on the Cochrane Risk of Bias tool. Any differences between the reviewers in the above process were resolved through consensus and arbitration with other investigators in the review team.
The extracted information comprised publication information (e.g., first author, publication year, and title), study design (experimental type), study participants (e.g., sample size, projects, and sex), intervention measures [e.g., altitude mode, hypoxic dose (altitude, m and duration, h)], and outcome measures (test results of maximum oxygen uptake in a plain environment before and after the intervention). The means and standard deviations of the outcome measures data were extracted. When the required data could not be extracted, the authors attempted to contact the authors of the included studies at least three times within 4 weeks to request for the information.
Intervention coding and hypoxic dose model
The hypoxic dosage of the different hypoxic types was coordinated using the “kilometer hour” model (Garvican-Lewis et al., 2016), defined as kmh = (m/1,000)× h, where “m” represents the altitude of the exposure environment and “h” represents the total exposure duration. In this study, the altitude/hypoxic training methods used in the included studies were categorized into the following three levels: 1) encoding based on the methodological design of altitude/hypoxic training, including HH, HL, IHT, IHE, and HHL; 2) subsequently, based on the first level encoding, we further refined the categorization of HL according to the type of hypoxia in the residential environment and the altitude of the training locations as follows: including living in a real altitude (hypobaric hypoxia) and training at a low altitude of 1,000–1500 m (HL_NAT/TLA), training in a simulated altitude (normobaric hypoxia) and training at a low altitude of 1,000–1500 m (HL_SIM/TLA), and living in a simulated altitude (normobaric hypoxia) and training at an altitude near sea level at <600 m (HL_SIM/TSL). In addition, we expanded the altitude range of the HH and included some control groups (which are not usually explicitly defined as HH in research) that underwent experiments at low altitudes into the HH category. We used a random-effects network meta-analysis within a Bayesian framework to rank and evaluate the intervention effects of various measures at this level; and 3) finally, the third level of encoding was also based on the first level, where the intervention measures were encoded at the intersection of specific types and dosages of AT, and the random-effects Bayesian Model-Based Network MetaAnalysis (MBNMA) was applied at this level. Furthermore, to facilitate the meta-analysis connectivity of the third level, the AT methods were condensed into several groups based on the approximate values of the hypoxic dosage (detailed data presented in Supplementary Annex S1).
Statistical analysis
Network meta-analysis
The netmeta package in R software (version 3.6.3) was adopted to perform network meta-analysis, combining direct and indirect comparisons in the frequency model (Rücker, 2012; Shim et al., 2019). The standardized mean difference (SMD) and 95% confidence interval (CI) were employed as effect size indicators. A random-effect network meta-analysis model was adopted to assess the combined magnitude of the effect sizes of the included studies. In the network evidence plot of the network meta-analysis, the size of the dots represents the sample size, and the lines between the dots represent direct comparisons between the two types of exercise interventions. The increase in the thickness of the line indicate more direct comparisons between the two interventions; otherwise, the line is thinner. If there was no line between the two types of exercise interventions, an indirect comparison was performed using network meta-analysis. In addition, all paired comparisons of the combined SMD and 95% CI are presented in the league table, and the effect of the respective exercise interventions on the maximal oxygen uptake of the participants relative to the control group is presented in a forest plot. P-scores were adopted to rank the exercise types based on the improvement of athletes’ maximal oxygen uptake. The P-scores ranged from 0 to 1, with a higher score suggesting a greater improvement in aerobic capacity (Rücker and Schwarzer, 2015). Tau-squared (τ2) tests and p-values were employed to qualitatively analyze heterogeneity among the studies. The higher the τ2 value and the smaller the p-value, the greater the likelihood of heterogeneity; in a contrast situation, the smaller the heterogeneity. Moreover, heterogeneity among the results of the respective studies was quantitatively analyzed using I2. Its values ranged from 0% to 100%. When I2 was less than 25%, it indicated low heterogeneity; 25%–50% indicated moderate heterogeneity; and >75% indicated high heterogeneity. In brief, when I2 was >50%, there was significant heterogeneity. Global and local methods were employed to test the inconsistency of study results. Global inconsistency was evaluated through the design-by-treatment test (Higgins et al., 2012). Furthermore, local inconsistency tests were performed using the node-splitting method in the R netmeta package (Dias et al., 2010). Next, the statistical analysis of funnel plots was conducted using Egger’s method. A p-value less than 0.05 indicated publication bias. The sensitivity of this study was evaluated by repeating the respective network meta-analysis after the exclusion of studies with high risk of publication bias.
Model-based network meta-analysis
Network meta-analysis based on random-effect Bayesian-based MBNMA (Mawdsley et al., 2016) was conducted to summarize the dose-response correlation between hypoxic dose and VO2max. No evidence of violation of the critical assumptions of network meta-analysis (Ter Veer et al., 2019) and consistency and transitivity of the data was reported (Wheeler et al., 2010; White et al., 2012) (Supplementary File S2). All effect sizes were reported as Hedges’ SMD (Ozawa and Onodera, 2017), and the credibility of the estimates of this study was evaluated using 95% credible intervals (CrI) (Etzioni and Kadane, 1995). First, the observed effects of different altitude/hypoxic training types and hypoxic doses on VO2max were plotted. A series of recommended nonlinear functions such as Emax, random or non-random restricted cubic spline, non-parametric, and exponential models (Pedder et al., 2019) were adopted to model the data based on the observed shape. Next, we derived and compared different fitting indices (Evans, 2019) (i.e., deviance information criterion [DIC], standard deviation between studies, number of parameters in the model, and residual values) and the corresponding bias plots (Evans, 2019). The random-effects model with restricted cubic splines produced the optimal fit in all cases (Supplementary File S2) and was, therefore, used to evaluate the nonlinear dose-response correlation (Harrell, 2017; Hamza et al., 2021). Departure from linearity was evaluated using Wald’s test (Harrell, 2017; Hamza et al., 2021). The hypoxic dose at which the restricted cubic spline had the greatest significant impact on VO2max was evaluated based on the Beta coefficient. Capitalizing on novel meta-analytic techniques (i.e., model-based dose-response network meta-analysis under a Bayesian framework) and evidence stemming from existing controlled trials, the current report examined the dose-response correlation between hypoxic dose and the VO2max of athletes.
Results
Characteristics of included studies
A total of 2,072 citations were identified through the search, and 59 eligible articles were retrieved (Figure 1). In the controlled trials that met our inclusion criteria, a total of 1,821 athletes were recruited, with 494 participants assigned to the normoxic training group and 1,327 participants assigned to the altitude/hypoxic training group. The average sample size in respective groups was 9 individuals (range, 5–20), with an average intervention period of approximately 16 days (range, 11–56). In the included trials, male participants were predominant (80.6%), and the vast majority of the included studies recruited endurance athletes (54 studies, 1,175 participants, 91.7%). Further details are provided in Supplementary Appendix S4, Table 4.1. Except for IHE, most of the studies about other methods did not use the blinding method. This phenomenon led to the rating of a high proportion of the included studies as high risk, reaching 46% (see Supplementary Appendix S5 for details).
Results of the network meta-analysis
Figure 2 shows the network of eligible comparisons. All AT interventions had at least one normoxic training as a control intervention and were directly compared with at least one other form of AT. The forest plot (Figure 3) shows that all AT interventions, except for IHE and HHL, were significantly more effective than normoxic training in improving athletes’ VO2max, with SMDs ranging from 1.04 (95% CrI, 0.47–1.61) for HL_NAT/TLA and 0.36 (95% CrI, 0.10–0.61) for IHT. Based on P-scores, HL combined with low altitude training was the most effective intervention for improving athletes’ VO2max (HL_NAT/TLA: 0.92 and HL_SIM/TLA: 0.86) and was significantly better than HL combined with sea level training (0.56). The main results of the network meta-analysis are presented in the league table (Table 1). Regarding improvement of athletes’ VO2max, HL_NAT/TLA, HL_SIM/TLA, and HH were superior to IHE (SMDs ranged from 0.52 to 0.96). In addition, the improvement effect of IHT was not as good as that of HL_NAT/TLA (SMD, 0.68; CrI, 0.05–1.30) and HL_SIM/TLA (SMD, 0.55; CrI, 0.01–1.08). The τ value was 0.2089, which was considered small in the context of the observed changes in AT. The I2 value was 46.2% (moderate heterogeneity). The global Q score for inconsistency was 14.56, with a p-value of 0.6014.
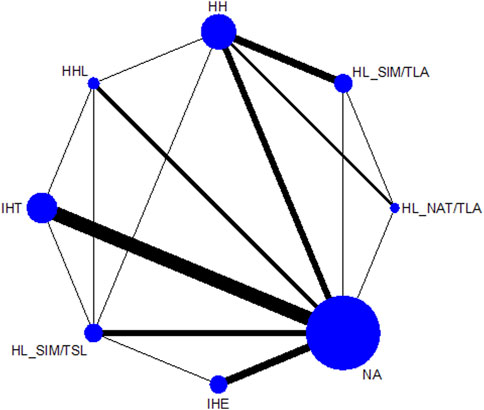
FIGURE 2. Network graphs for maximal oxygen consumption, and altitude/hypoxic training with direct comparisons are linked with a line; the thickness of connecting lines corresponds to the number of trials evaluating the comparison. HH “training high and living high,” HL_NAT/TLA “living in natural altitude but training at low altitude,” HL_SIM/TLA “training in simulated altitude but training at low altitude,” HL_SIM/TSL “living in a simulated altitude but training at sea level,” IHT “interment hypoxic training,” IHE “interment hypoxic expose,” HHL “living high and training low and high.”
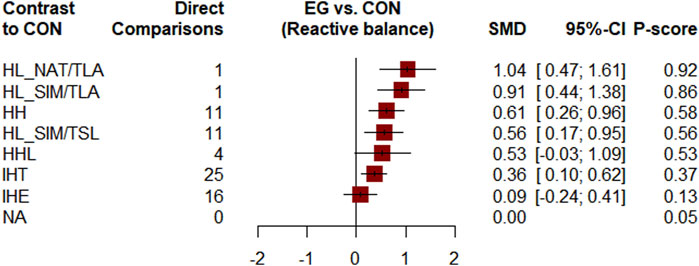
FIGURE 3. Forest plots for mean differences of altitude/hypoxic training compared with sea level/normoxic training. Confidence of outcomes was graded using the Confidence in Network Meta-Analysis application. HH “training high and living high,” HL_NAT/TLA “living in natural altitude but training at low altitude,” HL_SIM/TLA “training in simulated altitude but training at low altitude,” HL_SIM/TSL “living in a simulated altitude but training at sea level,” IHT “interment hypoxic training,” IHE “interment hypoxic expose,” HHL “living high and training low and high.”
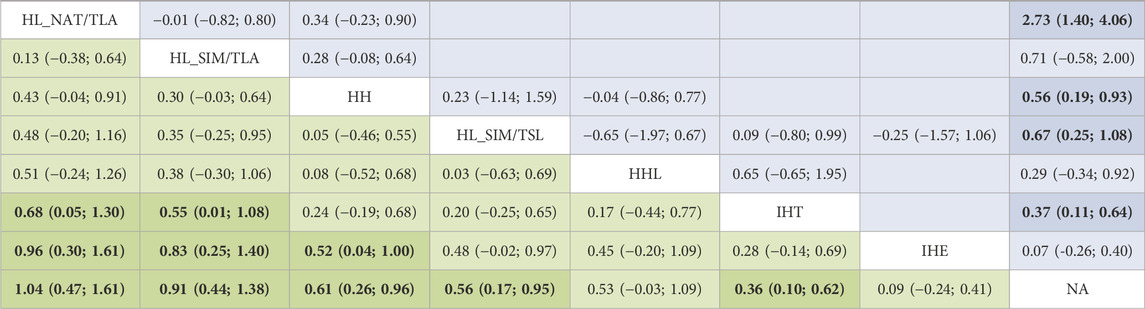
TABLE 1. League table for changes in maximal oxygen uptake associated with altitude/hypoxic training. For interpretation, a number larger than zero favours the column-defining treatment of a cell, i.e., this treatment leads to an increase in VO2max. Values depicted are mean differences with associated 95% confidence intervals. The upper half is results of direct comparison and the lower half of mixed comparison. HH “training high and living high,” HL_NAT/TLA “living in natural altitude but training at low altitude,” HL_SIM/TLA “training in simulated altitude but training at low altitude,” HL_SIM/TSL “living in a simulated altitude but training at sea level,” IHT “interment hypoxic training,” IHE “interment hypoxic expose,” HHL “living high and training low and high.”
Dose-response correlation
Figure 4 plots the dose-effect curves for the wide variety of interventions used in the included studies. An inverted u-shaped curve relationship between hypoxic dose and VO2max was identified for IHT, HH, and HL. A reasonable hypoxic dose range of HH (from 470 kmh to 1,130 kmh) led to a notable increase of the VO2max, and the reasonable dose for HL ranged from 500 kmh to 1,415 kmh. The effectiveness of IHT was no longer significant when the hypoxic dosage exceeded 42.5 kmh. Lastly, we found that HHL was not significantly correlated with IHE.
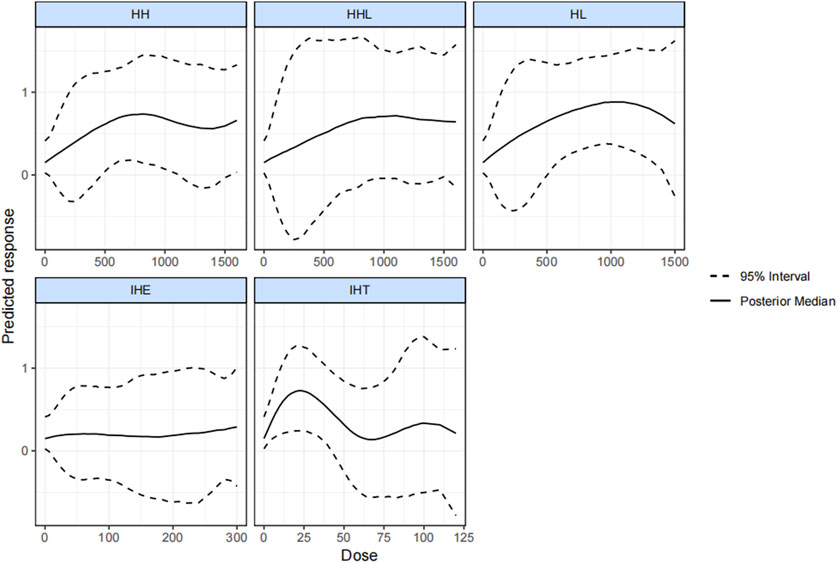
FIGURE 4. Dose-response associations between different types of altitude training and hypoxia dosage and change in VO2max. HH “training high and living high,” HL “living high but living low,” IHT “interment hypoxic training,” IHE “interment hypoxic expose,” HHL “living high and training low and high.”
Discussion
In this study, the effect of varying hypoxic methods on athlete’s VO2max was comprehensively compared and ranked, and the reasonable hypoxic doses range for the respective form was explored. In general, the results showed that HL, HH, and IHT, not IHE and HHL, significantly outperformed normoxic training in enhancing VO2max. HL with low altitude training was the optimal effective method (natural HL slightly better than simulated HL), whereas the effects of IHT reached the minimum. Besides, as indicated by the result, the hypoxic dosage of HL, HH, and IHT displayed a U-shaped relationship with a change in VO2max. The effective dosage for HH ranged from 470 kmh to 1,130 kmh. For HL, the effective dosage was from 500 kmh to 1,415 kmh. Furthermore, for IHT, the significant effect disappeared when the dosage exceeded 42.5 kmh.
In this study, the efficacy of HL in enhancing aerobic performance at sea level was confirmed (Fulco et al., 2000; Bonetti and Hopkins, 2009), and the reasonable range of hypoxic dosages was determined [a U-shaped relationship was found between the change in VO2max and hypoxic dosages (500–1,415 kmh)]. Despite variations in selected indicators, the effective hypoxic dosage in previous studies aligns closely with the reasonable range explored in this study (Rusko et al., 2004; Wilber, 2007; Gore et al., 2013). The U-shaped curve and upper limit of the reasonable dosage are novel findings that may arise from the adverse effects exerted by prolonged chronic hypoxia such as disrupted sleep quality (Kinsman et al., 2005; Sargent et al., 2013), oxidative disorders or pulmonary edema (Gaur et al., 2021; Rytz et al., 2022), accumulated fatigue (Bergeron et al., 2012; Koehle et al., 2014), etc.
Moreover, the effect of training site altitude and type of hypoxic residential environment on the efficacy of HL was investigated. The results showed that the altitude of the training location is the primary determinant of the efficacy of HL, whereas the type of hypoxia in a residential environment has little impact on the outcomes. Extensive research has suggested that the discernible variations in effective HL camps with different training heights are primarily attributed to two underlying factors. 1) Differences in total hypoxic dose: compared with training at sea level, training at low altitudes can provide additional hypoxic exposure (low degree) accompanied with an increase in hypoxic dose, this appropriate dose escalation is capable of inducing greater physiological adaptations accompanied by improvements in blood oxygen carrying capacity and VO2max and 2) the dual stimulation of environmental and exercise hypoxia: exposure to low altitudes compared with exposure to sea level altitudes can provide moderate hypoxic stress relative, such that appropriate metabolic and neural adaptations can be stimulated (without excessive damage to training quality such as training in moderate altitudes) (Zoll et al., 2006; Rodríguez et al., 2015). The findings of the influence of training altitude on the intervention efficacy of HL camps are highly relevant to the selection of an altitude base. No marked difference in chronic adaptive changes between simulated HL (HL_SIM/TAL) and natural HL (HL_NAT/TAL) was found when the altitude of the training site was similar. This observation is supported by several previous studies (Hauser et al., 2016; Saugy et al., 2016; Bourdillon et al., 2017), although athletes exhibit varying physiological reactions to acute normobaric and hypobaric hypoxia exposure (Loeppky et al., 1997; Girard et al., 2012; Beidleman et al., 2014; Coppel et al., 2015). In practical applications, simulating HL appears to be a more favorable method due to lower adverse impact risks (Saugy et al., 2016), economic and time costs (Carr et al., 2019; Millet et al., 2010), and more flexible operations (Millet et al., 2010).
Although HL has become the dominant training method in academic circles, classic HH remains a preferred choice among many elite athletes worldwide (Álvarez-Herms et al., 2015). This study indicates that HH camps with a recommended dosage range of 470–1,130 kmh can significantly improve athletes’ VO2max compared with that observed with normoxic training. Regarding effectiveness, HH ranked lower than HL with low altitude training. The above-mentioned observations may be explained by previous reports that the critical limitation of HH relative to HL is the heightened hypoxic stress at moderate altitudes (Levine and Stray-Gundersen, 1997; Carr et al., 2015), which can impede the generation of necessary training intensities for eliciting beneficial adaptations (Wehrlin and Hallén, 2006). Furthermore, except for the interference of various confounding factors, the lower upper limit and wider CI for the dose-response curve may correlate with an additional drawback of HH; the risk of adverse reactions in an HH condition is higher than in an HL condition due to greater hypoxic continuity (Brocherie et al., 2017; Mujika et al., 2018). Thus, to minimize this potential risk and maximize the benefits of AT, an effective health and load monitoring system should be developed (Sperlich et al., 2016; Schmitt et al., 2018; Mujika et al., 2019) and adequate nutritional support should be provided (Govus et al., 2015; Stellingwerff et al., 2019). Currently, the investigated lower limit of the hypoxic dose of HH is lower than the previous study results (Millet et al., 2010). Our findings indicate that athletes who partake in 2–3 weeks of low altitude camps can effectively enhance their VO2max (Gore et al., 1997; Carr et al., 2015). Compared with moderate and sea level altitudes, low altitude provides less hypoxic stimulation but maintains higher training quality and also offers dual stimulation of hypoxia and training. This makes low altitude camping a valuable adaptive measure for athletes preparing for higher altitude training or competitions (Saunders et al., 2009). Lastly, it is worth noting that HL with sea level training had effects similar to those of HH, which has also been reported in a similar study by Carr et al. (2019). The above perspective shows that passive hypoxia exposure alone may not maximize performance gains.
It is currently understood that the noteworthy enhancement of VO2max following HH or HL stems predominantly from hematological adaptation following chronic hypoxia exposure, which increases the total amount of hemoglobin accompanied by the enhancement of oxygen-carrying capacity (Hahn and Gore, 2001). Multiple studies have presented compelling evidence on aerobic adaptation under hypoxic conditions, with molecular mechanisms underlying the above-described hematological changes being elucidated. Furthermore, multiple studies have presented molecular-level evidence supporting these alterations in hematology such as hypoxia-inducible factor-1 (HIF-1), which is vital to the transcriptional regulation of erythropoietin (Bhattarai et al., 2018; Koyasu et al., 2018). However, Saunders et al.’s study shows a correlation between VO2max and hemoglobin mass changes with an r2 of only 0.15, suggesting that factors beyond hematological indices may account for 85% of the variation in VO2max (P. U. Saunders et al., 2013). Besides activating the EPO transcription gene, HIF-1 is also required for inducing other genes (e.g., encoding glycolytic enzymes, VEGF, GLUT-1, and metabolic proteins), which causes molecular changes and triggers non-hematological physiological adaptations such as increased citrate synthase activity, mitochondrial density, capillary-to-fiber ratio, and muscle buffering capacity (Wang et al., 1995; Vogt et al., 2001; Gore et al., 2007). The above changes may partially account for the improvement in VO2max independent of hematological adaptions.
This study confirmed the positive effect of IHT on athletes’ VO2max, which is consistent with a recent meta-analysis (Westmacott et al., 2022). Besides, we found two novel observations: 1) the minimum effect by IHT and 2) the reasonable hypoxic dosage range. First, the effectiveness of IHT may arise from its specific underlying mechanism or methodological design (Czuba, Fidos-Czuba et al., 2018). Unlike HH and HL, numerous studies have indicated that the hypoxic exposure of IHT is inadequate to substantially alter hematological parameters (Czuba et al., 2014; Czuba et al., 2019; Ambroży et al., 2020; Kim et al., 2021; Park et al., 2022; Teległów et al., 2022), and the potential mechanism for enhancing aerobic capacity primarily involves specific molecular adaptations in peripheral tissues (Ponsot et al., 2006; Zoll et al., 2006; Neya et al., 2007). It is worth noting that Czuba et al. posited that a hematological mechanism is more effective in enhancing aerobic capacity (Czuba et al., 2018), and the diverse adaptations due to IHT contributes greatly to exercise (Green et al., 2000; Hamlin et al., 2010; Park et al., 2016; Saunders et al., 2004), which can also serve as a valuable metric for evaluating aerobic performance (Levine and Stray-Gundersen, 2005). In summary, the physiological adaptations induced by IHT are unable to increase VO2max as significantly as that observed with HH and HL.
Although this study has explored a relatively robust dose-effect model for IHT, we found, after reviewing the included studies, that the model is unreasonable due to the oversight of a crucial indicator, training intensity under hypoxia, which is essential for promoting muscle and metabolic adaptations (McLean et al., 2014). Unfortunately, it is difficult to standardize the specific training intensity in each study and simultaneously consider the combined effects of hypoxic and training loads. Notably, several studies have stated that IHT can enhance athletes’ anaerobic capacity (Hendriksen and Meeuwsen, 2003; Millet et al., 2014; Morton and Cable, 2005), which is to an extent significant in team and high-level endurance sports. In summary, although IHT had the least impact on VO2max, it considerably important in practice due to the low cost and low risk of negative effects (Jung et al., 2020).
Theoretically, variant combining HHL with HL and LLTH should produce positive effects (Millet et al., 2010). However, our finding was not consistent with the theoretical expectation. Possible reasons include limited quantity of relevant experiments performed in the included studies, small sample sizes, and low statistical power. Furthermore, as revealed by most included studies, HHL can directly enhance time-trial performance (Robertson et al., 2010; Czuba et al., 2014), and some studies have reported no significant correlation between performance enhancement and changes in blood oxygen-carrying capacity (Czuba et al., 2014; Rodríguez et al., 2015). Although using VO2max as the sole evaluation criterion for HHL is insufficient, the lack in both the depth and breadth of research makes it difficult to comprehensively assess the effect of HHL. In-depth research should prioritize exploring HL to identify the most effective combination with other training methods.
The effectiveness of IHE in augmenting the aerobic performance of athletes has become a contentious topic (Millet et al., 2010; Viscor et al., 2018), with detractors contending that IHE fails to elicit significant physiological adaptations (Brugniaux et al., 2006; Rodríguez et al., 2007). For physiological or kinematic indicators, IHE failed to demonstrate any substantial improvement as indicated by over half of the included studies (6/9). Compared with other practices, IHE employs an extremely lower overall hypoxic dose and lacks supplementary high-intensity training stimuli during hypoxia exposure. This relatively limited and inadequate stimulation of athletes makes the feasibility of IHE questionable, and the driving force behind the development of this method may be more commercial than scientific (Lundby and Robach, 2016).
Limititation
The absence of blinding in most existing studies posed a high risk of bias in quality assessment and undermines the ability to rule out placebo effects. A cross-over design can be employed although blinding may not be feasible for AT in natural environments. Future relevant studies should adopt more rigorous experimental designs to improve the evidence on effectiveness and dosage accuracy. The efficacy of AT is not solely determined by the degree of hypoxia and exposure continuity, as various confounding factors that affect the final outcome have not been fully considered. These factors include the specific training content, load and intensity in hypoxic or normoxic environments, individual differences in hypoxic responses, and the criticality of various support work or specialty-specific factors (Burtscher et al., 2018). Unfortunately, quantifying the above-described variables is challenging and may increase the heterogeneity risk in this study.
Conclusion
The findings of this comprehensive network meta-analysis suggest that HL, HH, and IHT compared with normoxic training are capable of effectively improving the VO2max of athletes. The effect of HL with low altitude training was optimal, and the hypoxic type of residential environments (simulated or natural) did not affect the results. This observation can provide a useful reference for future site selection of an altitude base. In addition, this study also found a dose-effect relationship between hypoxic dose and VO2max variation for both HH and HL. As indicated by the results, hypoxic practices have maximum and minimum effective hypoxic dose thresholds, and both showed an inverted U-shaped curve between dose and efficacy. This observation may be correlated with the negative effects of chronic hypoxia, and it also provides sound recommendations regarding the time and height arrangements for altitude camps. Notably, as opposed to the previous study findings, HH camps organized at low altitudes can moderately enhance the VO2max of athletes, suggesting that it can be a valuable adaptive measure for athletes preparing for higher AT or competitions. The small number of studies on HHL in this meta-analysis limits the confidence of our findings. Following a brief review of other indicators, the combined approach seems to be optional, and the depth and breadth of relevant explorations should be improved in the future. Lastly, the quality of most studies was low. To maximize the exclusion of placebo effects, future controlled trials on simulated altitudes should consider using a blinded method, and controlled trials on real altitudes can attempt using the cross-over design.
Author contributions
XF and LZ designed the study and supervised the overall project; YC and ZW participated in collecting data; HL and CW participated in data collecting and analysis; XF and LZ provided the statistical analysis and wrote the manuscript. All authors contributed to the article and approved the submitted version.
Conflict of interest
The authors declare that the research was conducted in the absence of any commercial or financial relationships that could be construed as a potential conflict of interest.
Publisher’s note
All claims expressed in this article are solely those of the authors and do not necessarily represent those of their affiliated organizations, or those of the publisher, the editors and the reviewers. Any product that may be evaluated in this article, or claim that may be made by its manufacturer, is not guaranteed or endorsed by the publisher.
Supplementary material
The Supplementary Material for this article can be found online at: https://www.frontiersin.org/articles/10.3389/fphys.2023.1223037/full#supplementary-material
References
Álvarez-Herms, J., Julià-Sánchez, S., Hamlin, M. J., Corbi, F., Pagès, T., and Viscor, G. (2015). Popularity of hypoxic training methods for endurance-based professional and amateur athletes. Physiol. Behav. 143, 35–38. doi:10.1016/j.physbeh.2015.02.020
Ambroży, T., Maciejczyk, M., Klimek, A. T., Wiecha, S., Stanula, A., Snopkowski, P., et al. (2020). The effects of intermittent hypoxic training on anaerobic and aerobic power in boxers. Int. J. Environ. Res. Public Health 17 (24), 9361. doi:10.3390/ijerph17249361
Beidleman, B. A., Fulco, C. S., Staab, J. E., Andrew, S. P., and Muza, S. R. (2014). Cycling performance decrement is greater in hypobaric versus normobaric hypoxia. Extrem Physiol. Med. 3, 8. doi:10.1186/2046-7648-3-8
Bergeron, M. F., Bahr, R., Bärtsch, P., Bourdon, L., Calbet, J. A., Carlsen, K. H., et al. (2012). International Olympic Committee consensus statement on thermoregulatory and altitude challenges for high-level athletes. Br. J. Sports Med. 46 (11), 770–779. doi:10.1136/bjsports-2012-091296
Bhattarai, D., Xu, X., and Lee, K. (2018). Hypoxia-inducible factor-1 (HIF-1) inhibitors from the last decade (2007 to 2016): a "structure-activity relationship" perspective. Med. Res. Rev. 38 (4), 1404–1442. doi:10.1002/med.21477
Bonetti, D. L., and Hopkins, W. G. (2009). Sea-Level exercise performance following adaptation to hypoxia: a meta-analysis. Sports Med. 39 (2), 107–127. doi:10.2165/00007256-200939020-00002
Bourdillon, N., Saugy, J., Schmitt, L., Rupp, T., Yazdani, S., Vesin, J. M., et al. (2017). Acute and chronic changes in baroreflex sensitivity in hypobaric vs. normobaric hypoxia. Eur. J. Appl. Physiol. 117 (12), 2401–2407. doi:10.1007/s00421-017-3726-6
Brocherie, F., Schmitt, L., and Millet, G. P. (2017). Hypoxic dose, intensity distribution, and fatigue monitoring are paramount for "live high-train low" effectiveness. Eur. J. Appl. Physiol. 117 (10), 2119–2120. doi:10.1007/s00421-017-3664-3
Brugniaux, J. V., Schmitt, L., Robach, P., Nicolet, G., Fouillot, J. P., Moutereau, S., et al. (2006). Eighteen days of "living high, training low" stimulate erythropoiesis and enhance aerobic performance in elite middle-distance runners. J. Appl. Physiol. (1985) 100 (1), 203–211. doi:10.1152/japplphysiol.00808.2005
Burtscher, M., Niedermeier, M., Burtscher, J., Pesta, D., Suchy, J., and Strasser, B. (2018). Preparation for endurance competitions at altitude: physiological, psychological, dietary and coaching aspects. A narrative review. Front. Physiol. 9, 1504. doi:10.3389/fphys.2018.01504
Buskirk, E. R., Kollias, J., Akers, R. F., Prokop, E. K., and Reategui, E. P. (1967). Maximal performance at altitude and on return from altitude in conditioned runners. J. Appl. Physiol. 23 (2), 259–266. doi:10.1152/jappl.1967.23.2.259
Carr, A. J., Garvican-Lewis, L. A., Vallance, B. S., Drake, A. P., Saunders, P. U., Humberstone, C. E., et al. (2019). Training to compete at altitude:natural altitude or simulated live high:train low? Int. J. Sports Physiol. Perform. 14 (4), 509–517. doi:10.1123/ijspp.2018-0099
Carr, A. J., Saunders, P. U., Vallance, B. S., Garvican-Lewis, L. A., and Gore, C. J. (2015). Increased hypoxic dose after training at low altitude with 9h per night at 3000m normobaric hypoxia. J. Sports Sci. Med. 14 (4), 776–782.
Coppel, J., Hennis, P., Gilbert-Kawai, E., and Grocott, M. P. (2015). The physiological effects of hypobaric hypoxia versus normobaric hypoxia: a systematic review of crossover trials. Extrem Physiol. Med. 4, 2. doi:10.1186/s13728-014-0021-6
Czuba, M., Bril, G., Płoszczyca, K., Piotrowicz, Z., Chalimoniuk, M., Roczniok, R., et al. (2019). Intermittent hypoxic training at lactate threshold intensity improves aiming performance in well-trained biathletes with little change of cardiovascular variables. Biomed. Res. Int. 2019, 1287506. doi:10.1155/2019/1287506
Czuba, M., Fidos-Czuba, O., Płoszczyca, K., Zając, A., and Langfort, J. (2018). Comparison of the effect of intermittent hypoxic training vs. the live high, train low strategy on aerobic capacity and sports performance in cyclists in normoxia. Biol. Sport 35 (1), 39–48. doi:10.5114/biolsport.2018.70750
Czuba, M., Maszczyk, A., Gerasimuk, D., Roczniok, R., Fidos-Czuba, O., Zając, A., et al. (2014). The effects of hypobaric hypoxia on erythropoiesis, maximal oxygen uptake and energy cost of exercise under normoxia in elite biathletes. J. Sports Sci. Med. 13 (4), 912–920.
Daniels, J., and Oldridge, N. (1970). The effects of alternate exposure to altitude and sea level on world-class middle-distance runners. Med. Sci. Sports 2 (3), 107–112. doi:10.1249/00005768-197023000-00001
Dias, S., Welton, N. J., Caldwell, D. M., and Ades, A. E. (2010). Checking consistency in mixed treatment comparison meta-analysis. Stat. Med. 29 (7-8), 932–944. doi:10.1002/sim.3767
Etzioni, R. D., and Kadane, J. B. (1995). Bayesian statistical methods in public health and medicine. Annu. Rev. Public Health 16, 23–41. doi:10.1146/annurev.pu.16.050195.000323
Evans, N. J. (2019). Assessing the practical differences between model selection methods in inferences about choice response time tasks. Psychon. Bull. Rev. 26 (4), 1070–1098. doi:10.3758/s13423-018-01563-9
Fulco, C. S., Rock, P. B., and Cymerman, A. (2000). Improving athletic performance: is altitude residence or altitude training helpful? Aviat. Space Environ. Med. 71 (2), 162–171.
Garvican-Lewis, L. A., Sharpe, K., and Gore, C. J. (2016). Time for a new metric for hypoxic dose? J. Appl. Physiol. (1985) 121 (1), 352–355. doi:10.1152/japplphysiol.00579.2015
Gaur, P., Prasad, S., Kumar, B., Sharma, S. K., and Vats, P. (2021). High-altitude hypoxia induced reactive oxygen species generation, signaling, and mitigation approaches. Int. J. Biometeorol. 65 (4), 601–615. doi:10.1007/s00484-020-02037-1
Girard, O., Koehle, M. S., MacInnis, M. J., Guenette, J. A., Koehle, M. S., Verges, S., et al. (2012). Comments on point:counterpoint: hypobaric hypoxia induces/does not induce different responses from normobaric hypoxia. J. Appl. Physiol. (1985) 112 (10), 1788–1794. doi:10.1152/japplphysiol.00356.2012
Girard, O., Levine, B. D., Chapman, R. F., and Wilber, R. (2023). Living high-training low" for olympic medal performance: what have we learned 25 Years after implementation? Int. J. Sports Physiol. Perform. 18 (6), 563–572. doi:10.1123/ijspp.2022-0501
Gore, C. J., Clark, S. A., and Saunders, P. U. (2007). Nonhematological mechanisms of improved sea-level performance after hypoxic exposure. Med. Sci. Sports Exerc 39 (9), 1600–1609. doi:10.1249/mss.0b013e3180de49d3
Gore, C. J., Hahn, A. G., Burge, C. M., and Telford, R. D. (1997). VO2max and haemoglobin mass of trained athletes during high intensity training. Int. J. Sports Med. 18 (6), 477–482. doi:10.1055/s-2007-972667
Gore, C. J., Sharpe, K., Garvican-Lewis, L. A., Saunders, P. U., Humberstone, C. E., Robertson, E. Y., et al. (2013). Altitude training and haemoglobin mass from the optimised carbon monoxide rebreathing method determined by a meta-analysis. Br. J. Sports Med. 47 (1), i31–i39. doi:10.1136/bjsports-2013-092840
Govus, A. D., Garvican-Lewis, L. A., Abbiss, C. R., Peeling, P., and Gore, C. J. (2015). Pre-altitude serum ferritin levels and daily oral iron supplement dose mediate iron parameter and hemoglobin mass responses to altitude exposure. PLoS One 10 (8), e0135120. doi:10.1371/journal.pone.0135120
Green, H. J., Roy, B., Grant, S., Hughson, R., Burnett, M., Otto, C., et al. (2000). Increases in submaximal cycling efficiency mediated by altitude acclimatization. J. Appl. Physiol. (1985) 89 (3), 1189–1197. doi:10.1152/jappl.2000.89.3.1189
Hahn, A. G., and Gore, C. J. (2001). The effect of altitude on cycling performance: a challenge to traditional concepts. Sports Med. 31 (7), 533–557. doi:10.2165/00007256-200131070-00008
Hamlin, M. J., Hopkins, W. G., and Hollings, S. C. (2015). Effects of altitude on performance of elite track-and-field athletes. Int. J. Sports Physiol. Perform. 10 (7), 881–887. doi:10.1123/ijspp.2014-0261
Hamlin, M. J., Lizamore, C. A., and Hopkins, W. G. (2018). The effect of natural or simulated altitude training on high-intensity intermittent running performance in team-sport athletes: a meta-analysis. Sports Med. 48 (2), 431–446. doi:10.1007/s40279-017-0809-9
Hamlin, M. J., Marshall, H. C., Hellemans, J., Ainslie, P. N., and Anglem, N. (2010). Effect of intermittent hypoxic training on 20 km time trial and 30 s anaerobic performance. Scand. J. Med. Sci. Sports 20 (4), 651–661. doi:10.1111/j.1600-0838.2009.00946.x
Hamza, T., Cipriani, A., Furukawa, T. A., Egger, M., Orsini, N., and Salanti, G. (2021). A bayesian dose-response meta-analysis model: a simulations study and application. Stat. Methods Med. Res. 30 (5), 1358–1372. doi:10.1177/0962280220982643
Harrell, F. E. (2017). Regression modeling strategies. Bios 330 (2018), 14. doi:10.1007/978-3-319-19425-7
Hauser, A., Schmitt, L., Troesch, S., Saugy, J. J., Cejuela-Anta, R., Faiss, R., et al. (2016). Similar hemoglobin mass response in hypobaric and normobaric hypoxia in athletes. Med. Sci. Sports Exerc 48 (4), 734–741. doi:10.1249/mss.0000000000000808
Hendriksen, I. J., and Meeuwsen, T. (2003). The effect of intermittent training in hypobaric hypoxia on sea-level exercise: a cross-over study in humans. Eur. J. Appl. Physiol. 88 (4-5), 396–403. doi:10.1007/s00421-002-0708-z
Higgins, J. P., Jackson, D., Barrett, J. K., Lu, G., Ades, A. E., and White, I. R. (2012). Consistency and inconsistency in network meta-analysis: concepts and models for multi-arm studies. Res. Synth. Methods 3 (2), 98–110. doi:10.1002/jrsm.1044
Imray, C., Wright, A., Subudhi, A., and Roach, R. (2010). Acute mountain sickness: pathophysiology, prevention, and treatment. Prog. Cardiovasc Dis. 52 (6), 467–484. doi:10.1016/j.pcad.2010.02.003
Jung, W. S., Kim, S. W., and Park, H. Y. (2020). Interval hypoxic training enhances athletic performance and does not adversely affect immune function in middle- and long-distance runners. Int. J. Environ. Res. Public Health 17 (6), 1934. doi:10.3390/ijerph17061934
Kim, S. W., Jung, W. S., Kim, J. W., Nam, S. S., and Park, H. Y. (2021). Aerobic continuous and interval training under hypoxia enhances endurance exercise performance with hemodynamic and autonomic nervous system function in amateur male swimmers. Int. J. Environ. Res. Public Health 18 (8), 3944. doi:10.3390/ijerph18083944
Kinsman, T. A., Townsend, N. E., Gore, C. J., Hahn, A. G., Clark, S. A., Aughey, R. J., et al. (2005). Sleep disturbance at simulated altitude indicated by stratified respiratory disturbance index but not hypoxic ventilatory response. Eur. J. Appl. Physiol. 94 (5-6), 569–575. doi:10.1007/s00421-005-1368-6
Koehle, M. S., Cheng, I., and Sporer, B. (2014). Canadian academy of sport and exercise medicine position statement: athletes at high altitude. Clin. J. Sport Med. 24 (2), 120–127. doi:10.1097/jsm.0000000000000024
Koyasu, S., Kobayashi, M., Goto, Y., Hiraoka, M., and Harada, H. (2018). Regulatory mechanisms of hypoxia-inducible factor 1 activity: two decades of knowledge. Cancer Sci. 109 (3), 560–571. doi:10.1111/cas.13483
Lancaster, K., and Smart, N. (2012). Live-high train-low altitude training on maximal oxygen consumption in athletes: a systematic review and meta-analysis. Int. J. Sports Sci. Coach. 7 (1), 1–13. Retrieved from <Go to ISI>://WOS:000302513600002. doi:10.1260/1747-9541.7.1.1
Levine, B. D., and Stray-Gundersen, J. (2005). Point: positive effects of intermittent hypoxia (live high:train low) on exercise performance are mediated primarily by augmented red cell volume. J. Appl. Physiol. (1985) 99 (5), 2053–2055. doi:10.1152/japplphysiol.00877.2005
Levine, B. D., and Stray-Gundersen, J. (1997). Living high-training low": effect of moderate-altitude acclimatization with low-altitude training on performance. J. Appl. Physiol. (1985) 83 (1), 102–112. doi:10.1152/jappl.1997.83.1.102
Lobigs, L. M., Sharpe, K., Garvican-Lewis, L. A., Gore, C. J., Peeling, P., Dawson, B., et al. (2018). The athlete's hematological response to hypoxia: a meta-analysis on the influence of altitude exposure on key biomarkers of erythropoiesis. Am. J. Hematol. 93 (1), 74–83. doi:10.1002/ajh.24941
Loeppky, J. A., Icenogle, M., Scotto, P., Robergs, R., Hinghofer-Szalkay, H., and Roach, R. C. (1997). Ventilation during simulated altitude, normobaric hypoxia and normoxic hypobaria. Respir. Physiol. 107 (3), 231–239. doi:10.1016/s0034-5687(97)02523-1
Lundby, C., and Robach, P. (2016). Does 'altitude training' increase exercise performance in elite athletes? Exp. Physiol. 101 (7), 783–788. doi:10.1113/ep085579
Mawdsley, D., Bennetts, M., Dias, S., Boucher, M., and Welton, N. J. (2016). Model-based network meta-analysis: a framework for evidence synthesis of clinical trial data. CPT Pharmacometrics Syst. Pharmacol. 5 (8), 393–401. doi:10.1002/psp4.12091
McLean, B. D., Gore, C. J., and Kemp, J. (2014). Application of 'live low-train high' for enhancing normoxic exercise performance in team sport athletes. Sports Med. 44 (9), 1275–1287. doi:10.1007/s40279-014-0204-8
Millet, G., Bentley, D. J., Roels, B., Mc Naughton, L. R., Mercier, J., and Cameron-Smith, D. (2014). Effects of intermittent training on anaerobic performance and MCT transporters in athletes. PLoS One 9 (5), e95092. doi:10.1371/journal.pone.0095092
Millet, G. P., and Brocherie, F. (2020). Hypoxic training is beneficial in elite athletes. Med. Sci. Sports Exerc 52 (2), 515–518. doi:10.1249/mss.0000000000002142
Millet, G. P., Roels, B., Schmitt, L., Woorons, X., and Richalet, J. P. (2010). Combining hypoxic methods for peak performance. Sports Med. 40 (1), 1–25. doi:10.2165/11317920-000000000-00000
Morton, J. P., and Cable, N. T. (2005). Effects of intermittent hypoxic training on aerobic and anaerobic performance. Ergonomics 48 (11-14), 1535–1546. doi:10.1080/00140130500100959
Mujika, I., Halson, S., Burke, L. M., Balagué, G., and Farrow, D. (2018). An integrated, multifactorial approach to periodization for optimal performance in individual and team sports. Int. J. Sports Physiol. Perform. 13 (5), 538–561. doi:10.1123/ijspp.2018-0093
Mujika, I., Sharma, A. P., and Stellingwerff, T. (2019). Contemporary periodization of altitude training for elite endurance athletes: a narrative review. Sports Med. 49 (11), 1651–1669. doi:10.1007/s40279-019-01165-y
Neya, M., Enoki, T., Kumai, Y., Sugoh, T., and Kawahara, T. (2007). The effects of nightly normobaric hypoxia and high intensity training under intermittent normobaric hypoxia on running economy and hemoglobin mass. J. Appl. Physiol. (1985) 103 (3), 828–834. doi:10.1152/japplphysiol.00265.2007
Ozawa, T., and Onodera, O. (2017). Multiple system atrophy: clinicopathological characteristics in Japanese patients. Proc. Jpn. Acad. Ser. B Phys. Biol. Sci. 93 (5), 251–258. doi:10.2183/pjab.93.016
Park, H. Y., Jung, W. S., Kim, S. W., Kim, J., and Lim, K. (2022). Effects of interval training under hypoxia on hematological parameters, hemodynamic function, and endurance exercise performance in amateur female runners in korea. Front. Physiol. 13, 919008. doi:10.3389/fphys.2022.919008
Park, H. Y., Nam, S. S., Tanaka, H., and Lee, D. J. (2016). Hemodynamic, hematological, and hormonal responses to submaximal exercise in normobaric hypoxia in pubescent girls. Pediatr. Exerc Sci. 28 (3), 417–422. doi:10.1123/pes.2015-0176
Pedder, H., Dias, S., Bennetts, M., Boucher, M., and Welton, N. J. (2019). Modelling time-course relationships with multiple treatments: model-based network meta-analysis for continuous summary outcomes. Res. Synth. Methods 10 (2), 267–286. doi:10.1002/jrsm.1351
Ponsot, E., Dufour, S. P., Zoll, J., Doutrelau, S., N'Guessan, B., Geny, B., et al. (2006). Exercise training in normobaric hypoxia in endurance runners. II. Improvement of mitochondrial properties in skeletal muscle. J. Appl. Physiol. (1985) 100 (4), 1249–1257. doi:10.1152/japplphysiol.00361.2005
Robertson, E. Y., Saunders, P. U., Pyne, D. B., Gore, C. J., and Anson, J. M. (2010). Effectiveness of intermittent training in hypoxia combined with live high/train low. Eur. J. Appl. Physiol. 110 (2), 379–387. doi:10.1007/s00421-010-1516-5
Rodríguez, F. A., Iglesias, X., Feriche, B., Calderón-Soto, C., Chaverri, D., Wachsmuth, N. B., et al. (2015). Altitude training in elite swimmers for sea level performance (altitude project). Med. Sci. Sports Exerc 47 (9), 1965–1978. doi:10.1249/mss.0000000000000626
Rodríguez, F. A., Truijens, M. J., Townsend, N. E., Stray-Gundersen, J., Gore, C. J., and Levine, B. D. (2007). Performance of runners and swimmers after four weeks of intermittent hypobaric hypoxic exposure plus sea level training. J. Appl. Physiol. (1985) 103 (5), 1523–1535. doi:10.1152/japplphysiol.01320.2006
Rücker, G. (2012). Network meta-analysis, electrical networks and graph theory. Res. Synth. Methods 3 (4), 312–324. doi:10.1002/jrsm.1058
Rücker, G., and Schwarzer, G. (2015). Ranking treatments in frequentist network meta-analysis works without resampling methods. BMC Med. Res. Methodol. 15, 58. doi:10.1186/s12874-015-0060-8
Rusko, H. K., Tikkanen, H. O., and Peltonen, J. E. (2004). Altitude and endurance training. J. Sports Sci. 22 (10), 928–944. discussion 945. doi:10.1080/02640410400005933
Rytz, C. L., Pun, M., Mawhinney, J. A., Mounsey, C. A., Mura, M., Martin, A., et al. (2022). Differential effects of high-altitude exposure on markers of oxidative stress, antioxidant capacity, and iron profiles. Am. J. Physiol. Regul. Integr. Comp. Physiol. 323 (4), R445–r456. doi:10.1152/ajpregu.00321.2021
Sargent, C., Schmidt, W. F., Aughey, R. J., Bourdon, P. C., Soria, R., Claros, J. C., et al. (2013). The impact of altitude on the sleep of young elite soccer players (ISA3600). Br. J. Sports Med. 47 (1), i86–i92. doi:10.1136/bjsports-2013-092829
Saugy, J. J., Rupp, T., Faiss, R., Lamon, A., Bourdillon, N., and Millet, G. P. (2016). Cycling time trial is more altered in hypobaric than normobaric hypoxia. Med. Sci. Sports Exerc 48 (4), 680–688. doi:10.1249/mss.0000000000000810
Saunders, P. U., Garvican-Lewis, L. A., Schmidt, W. F., and Gore, C. J. (2013). Relationship between changes in haemoglobin mass and maximal oxygen uptake after hypoxic exposure. Br. J. Sports Med. 47 (1), i26–i30. doi:10.1136/bjsports-2013-092841
Saunders, P. U., Pyne, D. B., and Gore, C. J. (2009). Endurance training at altitude. High Alt. Med. Biol. 10 (2), 135–148. doi:10.1089/ham.2008.1092
Saunders, P. U., Telford, R. D., Pyne, D. B., Cunningham, R. B., Gore, C. J., Hahn, A. G., et al. (2004). Improved running economy in elite runners after 20 days of simulated moderate-altitude exposure. J. Appl. Physiol. (1985) 96 (3), 931–937. doi:10.1152/japplphysiol.00725.2003
Schmitt, L., Willis, S. J., Fardel, A., Coulmy, N., and Millet, G. P. (2018). Live high-train low guided by daily heart rate variability in elite Nordic-skiers. Eur. J. Appl. Physiol. 118 (2), 419–428. doi:10.1007/s00421-017-3784-9
Schommer, K., Menold, E., Subudhi, A. W., and Bärtsch, P. (2012). Health risk for athletes at moderate altitude and normobaric hypoxia. Br. J. Sports Med. 46 (11), 828–832. doi:10.1136/bjsports-2012-091270
Shamseer, L., Moher, D., Clarke, M., Ghersi, D., Liberati, A., Petticrew, M., et al. (2015). Preferred reporting items for systematic review and meta-analysis protocols (PRISMA-P) 2015: elaboration and explanation. Bmj-British Med. J. 349, g7647. doi:10.1136/bmj.g7647
Shim, S. R., Kim, S. J., Lee, J., and Rücker, G. (2019). Network meta-analysis: application and practice using R software. Epidemiol. Health 41, e2019013. doi:10.4178/epih.e2019013
Siebenmann, C., and Dempsey, J. A. (2020). Hypoxic training is not beneficial in elite athletes. Med. Sci. Sports Exerc 52 (2), 519–522. doi:10.1249/mss.0000000000002141
Solli, G. S., Tønnessen, E., and Sandbakk, Ø. (2017). The training characteristics of the world's most successful female cross-country skier. Front. Physiol. 8, 1069. doi:10.3389/fphys.2017.01069
Sperlich, B., Achtzehn, S., de Marées, M., von Papen, H., and Mester, J. (2016). Load management in elite German distance runners during 3-weeks of high-altitude training. Physiol. Rep. 4 (12), e12845. doi:10.14814/phy2.12845
Stellingwerff, T., Peeling, P., Garvican-Lewis, L. A., Hall, R., Koivisto, A. E., Heikura, I. A., et al. (2019). Nutrition and altitude: strategies to enhance adaptation, improve performance and maintain health: a narrative review. Sports Med. 49 (2), 169–184. doi:10.1007/s40279-019-01159-w
Teległów, A., Mardyła, M., Myszka, M., Pałka, T., Maciejczyk, M., Bujas, P., et al. (2022). Effect of intermittent hypoxic training on selected biochemical indicators, blood rheological properties, and metabolic activity of erythrocytes in rowers. Biol. (Basel) 11 (10), 1513. doi:10.3390/biology11101513
Ter Veer, E., van Oijen, M. G. H., and van Laarhoven, H. W. M. (2019). The use of (network) meta-analysis in clinical oncology. Front. Oncol. 9, 822. doi:10.3389/fonc.2019.00822
Viscor, G., Torrella, J. R., Corral, L., Ricart, A., Javierre, C., Pages, T., et al. (2018). Physiological and biological responses to short-term intermittent hypobaric hypoxia exposure: from sports and mountain medicine to new biomedical applications. Front. Physiol. 9, 814. doi:10.3389/fphys.2018.00814
Vogt, M., Puntschart, A., Geiser, J., Zuleger, C., Billeter, R., and Hoppeler, H. (2001). Molecular adaptations in human skeletal muscle to endurance training under simulated hypoxic conditions. J. Appl. Physiol. (1985) 91 (1), 173–182. doi:10.1152/jappl.2001.91.1.173
Wang, G. L., Jiang, B. H., Rue, E. A., and Semenza, G. L. (1995). Hypoxia-inducible factor 1 is a basic-helix-loop-helix-PAS heterodimer regulated by cellular O2 tension. Proc. Natl. Acad. Sci. U. S. A. 92 (12), 5510–5514. doi:10.1073/pnas.92.12.5510
Wehrlin, J. P., and Hallén, J. (2006). Linear decrease in.VO2max and performance with increasing altitude in endurance athletes. Eur. J. Appl. Physiol. 96 (4), 404–412. doi:10.1007/s00421-005-0081-9
Westmacott, A., Sanal-Hayes, N. E. M., McLaughlin, M., Mair, J. L., and Hayes, L. D. (2022). High-intensity interval training (hiit) in hypoxia improves maximal aerobic capacity more than hiit in normoxia: a systematic review, meta-analysis, and meta-regression. Int. J. Environ. Res. Public Health 19 (21), 14261. doi:10.3390/ijerph192114261
Wheeler, D. C., Hickson, D. A., and Waller, L. A. (2010). Assessing local model adequacy in bayesian hierarchical models using the partitioned deviance information criterion. Comput. Stat. Data Anal. 54 (6), 1657–1671. doi:10.1016/j.csda.2010.01.025
White, I. R., Barrett, J. K., Jackson, D., and Higgins, J. P. (2012). Consistency and inconsistency in network meta-analysis: model estimation using multivariate meta-regression. Res. Synth. Methods 3 (2), 111–125. doi:10.1002/jrsm.1045
Wilber, R. L. (2007). Application of altitude/hypoxic training by elite athletes. Med. Sci. Sports Exerc 39 (9), 1610–1624. doi:10.1249/mss.0b013e3180de49e6
Wilber, R. L., Stray-Gundersen, J., and Levine, B. D. (2007). Effect of hypoxic "dose" on physiological responses and sea-level performance. Med. Sci. Sports Exerc 39 (9), 1590–1599. doi:10.1249/mss.0b013e3180de49bd
Keywords: altitude/hypoxic training, maximal aerobic capacity, athletes, dose-response correlation, network meta-analysis
Citation: Feng X, Zhao L, Chen Y, Wang Z, Lu H and Wang C (2023) Optimal type and dose of hypoxic training for improving maximal aerobic capacity in athletes: a systematic review and Bayesian model-based network meta-analysis. Front. Physiol. 14:1223037. doi: 10.3389/fphys.2023.1223037
Received: 15 May 2023; Accepted: 17 August 2023;
Published: 05 September 2023.
Edited by:
Louise Deldicque, Université Catholique de Louvain, BelgiumReviewed by:
Gommaar D’Hulst, ETH Zürich, SwitzerlandIsmael Martínez Guardado, Nebrija University, Spain
Copyright © 2023 Feng, Zhao, Chen, Wang, Lu and Wang. This is an open-access article distributed under the terms of the Creative Commons Attribution License (CC BY). The use, distribution or reproduction in other forums is permitted, provided the original author(s) and the copyright owner(s) are credited and that the original publication in this journal is cited, in accordance with accepted academic practice. No use, distribution or reproduction is permitted which does not comply with these terms.
*Correspondence: Linlin Zhao, ZmVuZ3hpbm1pYW82NjZAMTYzLmNvbQ==