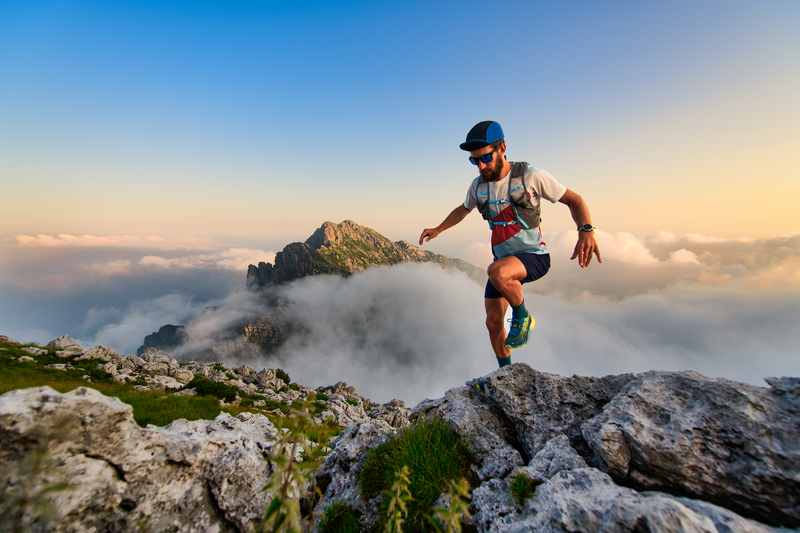
95% of researchers rate our articles as excellent or good
Learn more about the work of our research integrity team to safeguard the quality of each article we publish.
Find out more
REVIEW article
Front. Physiol. , 21 July 2023
Sec. Developmental Physiology
Volume 14 - 2023 | https://doi.org/10.3389/fphys.2023.1213757
This article is part of the Research Topic Women in Developmental Physiology: 2022 View all 7 articles
To date, the discussion concerning bile acids (BAs) during gestation is almost exclusively linked to pregnancy complications such as intrahepatic cholestasis of pregnancy (ICP) when maternal serum BA levels reach very high concentrations (>100 μM). Generally, the placenta is believed to serve as a protective barrier avoiding exposure of the growing fetus to excessive amounts of maternal BAs that might cause detrimental effects (e.g., intrauterine growth restriction and/or increased vulnerability to metabolic diseases). However, little is known about the precise role of the placenta in BA biosynthesis, transport, and metabolism in healthy pregnancies when serum BAs are at physiological levels (i.e., low maternal and high fetal BA concentrations). It is well known that primary BAs are synthesized from cholesterol in the liver and are later modified to secondary BA species by colonic bacteria. Besides the liver, BA synthesis in extrahepatic sites such as the brain elicits neuroprotective actions through inhibition of apoptosis as well as oxidative and endoplasmic reticulum stress. Even though historically BAs were thought to be only “detergent molecules” required for intestinal absorption of dietary fats, they are nowadays acknowledged as full signaling molecules. They modulate a myriad of signaling pathways with functional consequences on essential processes such as gluconeogenesis -one of the principal energy sources of the fetus- and cellular proliferation. The current manuscript discusses the potential multipotent roles of physiologically circulating BAs on developmental processes during gestation and provides a novel perspective in terms of the importance of the placenta as a previously unknown source of BAs. Since the principle “not too much, not too little” applicable to other signaling molecules may be also true for BAs, the risks associated with fetal exposure to excessive levels of BAs are discussed.
The primary bile acids (BAs) in humans, cholic acid (CA) and chenodeoxycholic acid (CDCA) are cholesterol derivatives produced in the liver through multistep synthetic processes involving several specific enzymes. These enzymes are hosted in subcellular compartments including peroxisomes. The BAs, bearing a pentanoic acid side chain and one to three hydroxyl groups are positioned at α3, α7, and α12 of the cholane, and are synthesized through two major routes, the classic (or neutral) pathway and the alternative (or acidic) pathway (Russell and Setchell, 1992; Russell, 2003; Chiang, 2009) (Figure 1). The classical pathway is initiated by the rate-limiting enzyme cytochrome P450 (CYP)7A1 and ends with the synthesis of CA. The alternative pathway, controlled among others by CYP27A1, produces CDCA. The alternative BA pathway begins with hydroxylation of the side chain of cholesterol, producing an oxysterol. The latter is then hydroxylated at the 7α-position by an oxysterol 7α-hydroxylase. Of note, depending on the organ, different pathways such as sterol 27-hydroxylase (CYP27A1, in the liver) and/or cholesterol 25-hydroxylase (CH25H, also in the liver) and/or cholesterol 24-hydroxylase (CYP46A1 and CH24H in liver and brain) initiate BA biogenesis (Figure 1). The resulting intermediate molecules, like 3β-hydroxy-5-cholenoic acid, can undergo 7α-hydroxylation and multistep conversions that end with the production of CDCA. The BA synthetic pathways are also present in other highly metabolic organs such as the brain (Parker et al., 2020; Monteiro-Cardoso et al., 2021) and the murine and human placenta (Russell, 2003; Ontsouka et al., 2023). Regardless of the synthetic pathway implicated, the final steps in BA biogenesis involve the enzymatic actions of BA-CoA synthase and BA CoA: amino acid N-acyltransferase (BAAT). These enzymes promote the conjugation (or amidation) of CA and CDCA with taurine to form tauro-CA (TCA) and tauro-CDCA (TCDCA), respectively. In addition, some primary BAs are conjugated with glycine to form glyco-CA (GCA) and glyco-CDCA (GCDCA), respectively (Russell, 2003; Chiang and Ferrell, 2019).
FIGURE 1. Scheme summarizing the biosynthesis of primary and secondary bile acids (BAs) in human tissues. The two major routes of BA synthesis, namely, the classic (or neutral) and the alternative (or acidic) pathways, are illustrated. The cholesterol derivates implicated in the classic and alternative BA synthesis pathways in the liver, brain, and potentially placenta are shown in conjunction with the resulting primary BAs synthesized. In addition, the corresponding secondary BAs resulting from the intestinal modification of primary BA are depicted. Figure created with BioRender.com.
The amino acid-conjugated BAs exhibit increased hydrophilicity and enhanced transmembrane transport, resulting in reduced cellular cytotoxicity. Within the intestinal lumen, primary BAs are deconjugated and dehydroxylated at the 7α-position under the enzymatic action of intestinal bacterial microflora (Niefan et al., 2015), giving rise to secondary BAs species (Begley et al., 2005; Ridlon et al., 2016; Sender et al., 2016). Specifically, internal modifications of CA and CDCA convert them into deoxycholic acid (DCA) and lithocholic acid (3α-hydroxy-5β-cholanoic acid; LCA), respectively. It is well documented that both primary and secondary BA species present in the intestine, are efficiently absorbed by the intestinal lumen, recycled back to the liver via the portal vein, and re-secreted into bile, a process known as enterohepatic circulation. This closed circuit allows only a small portion of BAs to bypass the enterohepatic circulation to enter the blood circulation, keeping the BA blood content relatively low (Hofmann, 2009).
The regulation of BA biogenesis is, among other factors, subject to diurnal variations. In rats and mice, BA synthesis peaks during the dark phase when they feed the most (Sundseth and Waxman, 1990), while in humans it peaks during daytime (Poole and Duane, 1988; Gälman et al., 2005). The rhythmicity of the hepatic BA synthesis is transcriptionally regulated (Sundseth and Waxman, 1990). Moreover, BA metabolism might be affected by autocrine, paracrine, and/or endocrine actions of other important molecules, such as estrogen, progesterone and their metabolites, and melatonin, all these being intrinsically produced by the placenta (Iwasaki et al., 2005; Lanoix et al., 2012; Karahoda et al., 2021). Estrogen, progesterone, and their metabolites increase hepatic BA production and reduce bile canaliculi release during pregnancy, leading to elevated total BA concentrations in the peripheral blood of pregnant women. Maternal melatonin blood levels gradually increase during pregnancy (Iwasaki et al., 2005; Lanoix et al., 2012), and melatonin seems to act as a protective molecule that balances oxidative effects and apoptosis caused by diverse effectors, including high circulating BAs (Zagrean et al., 2019; Chuffa et al., 2020; Gomes et al., 2021; Zhu et al., 2021). Melatonin freely crosses the biological membranes, acting intracellularly by modulating various signaling pathways (Chuffa et al., 2019; Zagrean et al., 2019). Unlike hepatic BA production in humans, melatonin rhythmically released by the pineal gland shows its highest level during the night. From a physiological point of view, it would be interesting to evaluate in future studies if BA biosynthesis in the liver and the placenta is rhythmically synchronized. Interestingly, one study in rats described concomitant increases of the BA contents in the maternal and fetal sera, in the liver, and in the placenta (Herraez et al., 2014), but the source of these BA was not investigated.
BAs exhibit marked effects on placental functions (Sepúlveda et al., 1991; Egan et al., 2012; Lofthouse et al., 2019) as well as on fetal development (De Aguiar Vallim et al., 2013; Li et al., 2020). Thus, for instance, exposure to high concentrations of BAs, particularly to LCA, exerted a constrictive effect on chorionic veins in humans (Sepúlveda et al., 1991; Lofthouse et al., 2019). Moreover, in the perfused cotyledon an increase in pressure and the constriction of chorionic arterial vessels were observed in the presence of taurine-conjugated CA (Lofthouse et al., 2019). However, the precise source of BAs acting on the placenta is not clearly described. The clarification of this important aspect implies the need to examine the probability and relevance of locally synthesized BAs, which could not only act on the placenta and surrounding tissues but may also impact fetal development and pregnancy outcomes.
From the original discovery in 1848 by Heinrich Otto Wieland until their identification as hormone-like molecules (Maruyama et al., 2002; Kawamata et al., 2003), BAs have been historically known as “detergent molecules” thanks to their physicochemical properties. The physicochemical effects of BAs are largely dependent on their hydrophobicity, which is also an important determinant of their toxicity. It is known that hydrophobic BAs can damage cell membranes and promote oxidative stress, necrosis and apoptosis, while hydrophilic BA species protect against oxidative stress and inhibit apoptosis (Perez and Britz, 2009). This feature is determined by the number, position, and orientation of the hydroxyl groups, and the amidation (i.e., conjugation) at position C-24. Thus, the magnitude of their hydrophobicity is UDCA (ursodeoxycholic acid) < CA < CDCA < DCA < LCA (Thomas et al., 2008). The sulfation of BAs leads to the production of water-soluble BA species (Alnouti, 2009). Placental sulfatase as well as sulfotransferase activities have been reported, allowing the placenta to convert unconjugated steroids (e.g., BAs and estrogens), into their sulfated forms and vice versa (Stanley et al., 2001; Miki et al., 2002; Dongning et al., 2004). These sulfatase and sulfotransferase activities of the placenta probably constitute a metabolic barrier to the transfer of potentially toxic hydrophobic BAs from the maternal to the fetal circulation.
BAs are nowadays fully acknowledged as signaling molecules that elicit functional effects by interacting with specific cell surface G-protein coupled receptors (GPCRs) expressed on the apical and basal sides of the syncytiotrophoblast (Figure 2). BAs are not only natural ligands for cell surface GPCRs, but also interact with nuclear receptors, such as farnesoid X receptors (FXRs), pregnane X receptor (PXR), vitamin D receptor (VDR), retinoid X receptors (RXR), and the constitutive androstane receptor (CAR). Receptor-mediated effects of BAs play key roles in the regulation of lipid, glucose, and energy metabolism (De Aguiar Vallim et al., 2013; Li et al., 2020), processes that are crucial for adequate placental function.
FIGURE 2. Model of the proposed entero-hepato-placental bile acid (BA) axis during a healthy pregnancy. The proposed action of this axis relies on the role of BAs as signaling molecules. Primary BAs are synthesized by the maternal liver, conjugated and unconjugated to an amino acid residue (in humans, glycine, or taurine) and are released into the maternal intestine where they can be modified to secondary BA by enzymatic activities of the maternal intestinal microbiota (right, black arrow). Circulating maternal primary and secondary BAs are efficiently transported across the intestine and recycled back to the liver (right, green arrow). A small proportion (5–10%) of the recycled intestinal BAs is directed towards the periphery, thereby reaching the placenta (right, green arrow), which they cross to enter the fetal circulation (center, green arrow). In turn, primary BAs synthesized by the mature fetal liver, conjugated and unconjugated to an amino acid residue and released into the fetal intestine (left, black arrow), do not undergo modifications in the fetal intestine since the latter is not yet colonized by specific intestinal microbiota (left, green arrow with symbol X) and the enterohepatic circulation is not yet functional. Therefore, the fetus-borne primary BAs and the potentially recycled maternally originating BAs (center, pink arrow) are directed towards the placenta where they are expelled into the maternal circulation. In addition, we propose that the placenta may locally synthesize primary BAs which are added to the overall BA pool and act on the placental, maternal, and fetal compartments (center, dashed arrows). The placenta allows the transport and action of the BA species in both directions, potentially including those that are synthesized in situ. Figure created with BioRender.com.
The G protein-coupled bile acid receptor 1 (GPBAR1), also known as Takeda G protein-coupled receptor 5 (TGR5), is activated by both primary and secondary BAs. It is mainly responsive to LCA, with the following affinity rank: LCA > DCA > CDCA > CA > UDCA (Maruyama et al., 2002; Kawamata et al., 2003; Schaap et al., 2014). In the human placenta, GPBAR1/TGR5 protein was identified in some regions of the trophoblasts at term, as well as in fetal macrophages. Upon BA-induced activation, GPBAR1/TGR5 mediates important functions, including smooth muscle contractility, inflammatory responses, regulation of mucosal permeability, and alteration of insulin release (González-Mariscal et al., 2008; Rajagopal et al., 2013; Guo et al., 2016; Pathak et al., 2018; Zhan et al., 2020). At the mRNA level, GPBAR1/TGR5 was detected in several tissues including the placenta and brain (Kawamata et al., 2003; Keitel et al., 2015; Guo et al., 2016; Klindt et al., 2019).
Sphingosine-1-phosphate (S1P), a natural ligand of the sphingosine-1-phosphate receptors (SP1R), is abundantly present in red blood cells, vascular endothelial cells, and activated platelets (Yatomi et al., 2001; Ryu et al., 2002). Expression of S1PR subtypes 1, 3, and 5 at the mRNA level, of S1PR1 at the protein level (Dobierzewska et al., 2016), and the presence of S1P kinase have been reported in the human placenta (Johnstone et al., 2005). The activation of S1PR1-5 is associated with the modulation of important physiological processes, such as vascular development during embryogenesis, wound repair, and cancer metastasis (Bartke and Hannun, 2009). S1PR subtypes 1 and 3 also appear to be reduced in preeclampsia (Dobierzewska et al., 2016). S1P and its synthesizing enzyme sphingosine kinase (SPHK) seem to have an angiogenic bioactive role in the process of trophoblast differentiation and invasion (Johnstone et al., 2005; Singh et al., 2012; Zhang et al., 2013) and placental angiogenesis (Mizugishi et al., 2007). Thus, the functional effects of BA might involve the activation of the S1PRs and influence placental and fetal development by improving nutrient delivery to the fetus.
Previous data further indicate that muscarinic acetylcholine receptors (M), a subfamily of GPCRs that regulate numerous fundamental functions of the central and peripheral nervous system, exhibit a high binding affinity for BAs (Raufman et al., 2002; Ibrahim et al., 2018; Ticho et al., 2019). The M subtype 2 is the predominant form found in human term placenta (Colmenero Salas et al., 2009), but M1-M4 were also detected (Tayebati et al., 1997). The functional consequences of the BA-induced activation of the Ms include the regulation of blood flow and fluid volume in placental vessels, the opening and closing of trophoblastic channels, the induction of the contractile properties of myofibroblasts, the facilitation of amino acid transport across the placenta, the release of placental hormones, and modulation of the formation of myometrial and placental prostaglandins in human parturition (Sastry, 1997).
BA species are transported to their sites of action by membrane proteins of the ATP-binding cassette (ABC) transporter and solute carrier (SLC) families. Some of these membrane transporters, such as the organic anion transporting polypeptide (OATP)1B1/SLCO1B1, OATP1B3/SLCO1B3, ABCG5, multidrug resistance-associated proteins (MRP) type 1 (MRP1/ABCC1), and bile salt export pump (BSEP/ABCB11) are known to be important for maintaining BA homeostasis in the human liver (Gerloff et al., 1998; Pradhan-Sundd and Monga, 2019) and intestine (Berge et al., 2000; König et al., 2000), but their role in the placenta is still unclear. BA transporters that were consistently shown to be expressed in the placenta and are assumed to be involved in the transcellular transfer of BA are discussed in the following sections.
The ABC transporters BCRP/ABCG2, MDR1/ABCB1, MRP2/ABCC2, MRP3/ABCC3, MRP4/ABCC4, the solute carriers OATP4A1/SLCO4A1 and ASBT/SLC10A2, and the P-type ATPase FIC1/ATP8B1 are reported to be localized at the apical side of the placental syncytium (Ontsouka et al., 2021) which is consistent with their role in the retrograde transport towards the maternal compartment (Figure 2). Many of the apical transporters mentioned above have partially overlapping substrate specificities. Substrates include cholesterol, nutritional compounds, xenobiotics, different BA species (e.g., taurocholate and cholate), estrogens and prostaglandins and their sulfated derivates (Allikmets et al., 1998; Zelcer et al., 2003; Lam et al., 2005). For some of these transporters consistent expression throughout gestation has been found in the human placenta. Depending on the study, ABCG2 gene expression has been shown to both increase and remain unchanged across gestation (Mathias et al., 2005; Meyer Zu Schwabedissen et al., 2005; Yeboah et al., 2006; Petrovic et al., 2015; Sieppi et al., 2016). The role of placental ABCG2 and MRP3 in the increase of maternal serum BAs was recently confirmed (Liu et al., 2021) implying a protective function of these transporters for the fetus. ABCB1 (both mRNA and protein) has been reported to be highly expressed in the first trimester compared to term placentas (Mathias et al., 2005). In rats, placental mRNA expression of Oatp1b2 decreased from gestational day 13 to day 20, then increased again immediately before birth, while the opposite pattern was reported for placental Oatp-4 mRNA levels (St-Pierre et al., 2004). In contrast, ATP8B1 mRNA expression was significantly reduced (33-fold) in the third compared to the first trimester in humans (Patel et al., 2003). In general, these data suggest that the investigated transporters are involved in the maintenance of maternal-fetal BA homeostasis at different gestational stages.
The ABC transporter MDR3/ABCB4 as well as the solute carriers OATP2B1/SLCO2B1, OATP3A1/SLCO3A1, NTCP/SLC10A1, OST-α/SLC51A1/, OST-β/SLC51B1, and the small protein mEH/EPHX1 were reported to be located at the basal side of the syncytiotrophoblast layer (Ontsouka et al., 2021). Given their transport directionality and specificity, these proteins modulate fetal exposure to a broad range of substrates, including nutritional compounds, sulfated steroids, and BA species (Dippe et al., 1996; Tamai et al., 2000; Huber et al., 2006; Roth et al., 2012). ABCB4 mRNA expression in the human placenta was increased 4-fold in the third trimester compared to the first trimester (Patel et al., 2003). Considering the unidirectionality of the substrate transport by ABCB4 towards the fetal circulation (Figure 2), its gestation-dependent increase may suggest an incremental role of ABCB4 in sustaining fetal development. ABCB4 is potentially also important in transporting BAs to their sites of receptor-mediated actions in the developing fetus as well as in the placenta itself. Moreover, given the BA transport directionality suggested for NTCP, OATP2B1, OATP3A1, OST-α/-β, and mEH in an earlier study (Ontsouka et al., 2021), these transporters may represent active players in the retrograde BA transport system towards the placenta/mother, thus protecting the fetus from overexposure to BA.
The most common BA-related disorder specific to pregnancy is intrahepatic cholestasis of pregnancy (ICP), a disease that can occur as early as the seventh week of gestation but appears more frequently in the third trimester. It is characterized by high levels of maternal BA concentrations with a cut-off value of 10 μmol/L (Manzotti et al., 2019) and up to a maximum value of 430 μmol/L (Lammert et al., 2000). ICP is clinically characterized by pruritus, abnormal liver function tests (liver transaminases), and supra-physiological levels of circulating BAs (Lammert et al., 2000). There is a well-documented correlation between increased maternal blood BA levels and increased rates of fetal complications. In cases of severe ICP, where maternal serum BA concentrations reach 100 μmol/L (Brouwers et al., 2015), a poor pregnancy outcome is expected, including preterm labor (Geenes and Williamson, 2009), fetal distress, fetal asphyxia, and even intra-uterine death (Fisk and Storey, 1988; Glantz et al., 2004; Williamson et al., 2004). In these cases, greater concentrations of BAs are detected in the amniotic fluid, similar to women who gave birth to infants suffering from prenatal intestinal obstruction (Délèze et al., 1977).
Mutations in some of the apical efflux transporters have been associated with pregnancy-related diseases (Jacquemin et al., 1999; Müllenbach et al., 2005; Aydın et al., 2020). As suggested by (Müllenbach et al., 2005; Aydın et al., 2020), mutations in ATP8B1/FIC1 were found to be linked to ICP in humans. This aminophospholipid translocase modulating membrane asymmetry (Tang et al., 1996) was speculated to cause or contribute to ICP, but it is still unclear how mutations in the translocase activities of ATP8B1/FIC1 (Müllenbach et al., 2005; Aydın et al., 2020), may be mechanistically involved in this disease. Clinical treatment of ICP patients using UDCA has been associated with alterations in the placental expression of some BA transporters. One study reported a significant increase in the placental expression of MRP2 (both protein and mRNA) while MRP3 protein levels were not significantly different in ICP patients treated with UCDA compared to controls (Azzaroli et al., 2007). Similarly, UDCA treatment in humans was associated with significant upregulation of placental mRNA and protein levels of ABCG2 (Azzaroli et al., 2013). On the other side, in patients with moderate ICP which were treated with UDCA, neither the mRNA expression of ABCG2 nor MRP2 was significantly different compared to controls (Ontsouka et al., 2021). Thus, the discrepancy regarding the MRP2 gene expression between the latter two studies (Azzaroli et al., 2007; Ontsouka et al., 2021) may be linked to the variable severity degree found in the ICP patients. Finally, an association between a mutation in the ABCB4 gene (located on the basal side of the syncytiotrophoblast layer) and the occurrence of ICP was also documented (Jacquemin et al., 1999), suggesting its possible mechanistic involvement in the pathogenesis of ICP. Similarly, treatment with UDCA was associated with significant downregulation of SLCO3A1 mRNA in ICP patient treated with UDCA as compared to controls (Ontsouka et al., 2021). A model summarizing the molecular mechanisms through which BAs interact with their receptors and transporters at the apical and basal sides of the syncytiotrophoblast layer is shown in Figure 2.
It is well established that the enterohepatic circuit determines serum BA levels (Hofmann, 2009). So far, the placenta has been primarily considered as a physical barrier between the maternal and fetal compartments, across which various substances including BAs and gases are exchanged (Herraez et al., 2014; Liu et al., 2021), thereby influencing the levels of these compounds in the maternal and fetal blood. Noticeably, the placenta is also a steroidogenic organ capable of the synthesis of diverse hormones such as progesterone using cholesterol as a precursor molecule (Karahoda et al., 2021). Interestingly, there is evidence that also extrahepatic tissues (e.g., the brain) are capable of synthesizing BAs and therefore contribute to the serum BA pool (Pan et al., 2017; Monteiro-Cardoso et al., 2021). In the context of pregnancy, previous studies have shown that serum BA levels are higher in pregnant compared to non-pregnant women and mice (Milona et al., 2010), regardless of the gestational age (Colombo et al., 1985; Egan et al., 2012). The concentration of BAs in healthy pregnancies is usually higher in the fetal blood than in the maternal circulation (Itoh et al., 1982; Colombo et al., 1985). In this physiological situation, the placenta serves as an exchange interface that prevents the accumulation of BAs in the fetal compartment to avoid deleterious effects on the fetus (Sewell et al., 1980; Colombo et al., 1985; St-Pierre et al., 2000). However, a study by Sasaki et al. reported that in 52.6% of the patients the BA content in the maternal serum was higher than in the umbilical cord, while in 36.8% of the cases the opposite was observed, and in 9.2% the BA content was similar (Sasaki, 1984). Overall, the vectorial transfer of the primary BAs across the placenta occurs mainly from the fetus to the mother (Figure 2), although secondary maternal BAs are transported from the mother into the fetal vessels (Colombo et al., 1987). A recent study performed in rats further suggested that also sex may play a role in BA concentrations since the total amount of placental BAs was two times higher in males than in females, but the opposite trend was observed in the fetal serum (Huang et al., 2021). Hence the factor sex adds another important variable influencing the selective allocation of BAs on both sides of the placental interface.
Given the central role of the placenta in facilitating the bi-directional transport of BAs (Figure 2), it is not surprising that placental dysfunction is a hallmark accompanying pregnancy-related metabolic diseases (e.g., preeclampsia, ICP). In ICP, particularly in severe cases (Brouwers et al., 2015), BAs are thought to be transported from the mother to the developing fetus (Meng et al., 1997; Tribe et al., 2010; Geenes et al., 2014; Liu et al., 2021; Ontsouka et al., 2021) exposing it to supraphysiological and toxic levels (Figure 2). Given the fetal over-exposure to BAs in ICP, one may question whether the placenta acts as an efficient BA protective barrier or rather as an exchange interface. It is important to note that elevated serum BAs in pregnant women do not necessarily imply ICP, since a subset of healthy pregnant women spontaneously presents levels above the mentioned cut-off value - a condition called asymptomatic hypercholanemia of pregnancy (AHP) - without suffering from ICP-related complications or other liver diseases (Pascual et al., 2002; Castaño et al., 2006). In this context, one study comparing the profiles of serum BAs between ICP and normal pregnancies including AHP suggested a shift towards a hydrophobic composition and free BAs in women with ICP (Castaño et al., 2006). This implies that the deleterious effects commonly attributed to this disease may result from the BA profile rather than the total amount of BAs. Moreover, it has been reported that some BA species —especially hydrophilic BAs such as UDCA and its taurine-conjugated derivative— exhibit beneficial health effects. Among others, they promote cytoprotective effects by inhibiting cellular oxidative stress and apoptosis (Perez and Britz, 2009). Serum BA profiles on both sides of the placenta are markedly different in healthy pregnancies (Sasaki, 1984; Geenes et al., 2014), highlighting the potential involvement of the placenta in the selective distribution of BA species between the maternal and fetal blood. Sasaki et al. (Sasaki, 1984) compared the BA distribution in maternal and umbilical cord sera in healthy pregnancies and showed that GCDCA was more prominent in the mother, while TCDCA was predominant in the umbilical cord (Sasaki, 1984). In contrast, the most abundant species in the fetal circulation in ICP is CA, which accounts for approximately 70%–80% of all circulating BAs (Tribe et al., 2010; Geenes et al., 2014). The observed differences imply an important role of the placenta in the transport, selection, and metabolism of the BAs arriving from both the mother and the fetus. Moreover, the placenta potentially complements the arriving BA pool with own locally synthesized BAs given its intrinsic placental steroidogenic machinery (see chapter 2.4.2.).
In a recent study, we reported the presence of mRNA transcripts of most of the BA synthesizing enzymes in the placentas of healthy mice and humans and proposed this organ as a potential new source of BA (Ontsouka et al., 2023). In this study, a species-specific expression profile of BA synthesizing enzymes was observed in placental tissues. Among others, CYP7A1, CYP46A1, and BAAT were undetected in human placental tissue, but their homologs were detected in the mouse placenta. Conversely, mRNA of Cyp8b1 and Hsd17b1 were missing in the mouse placenta, while their homologs were expressed in the human placenta. CYP39A1/Cyp39a1 and cholesterol 25-hydroxylase (CH25H/Ch25h) mRNA were detected in both species. It should be also noted that, even though mRNA transcripts of CYP7A1 were not consistently detected in the human placenta, they were found in isolated primary cytotrophoblast and syncytiotrophoblast cells. The apparent discrepancy between placental tissues and cell-based data could have resulted from a dilution effect occurring in placental tissue, which contains a myriad of cell types. The findings reported by (Ontsouka et al., 2023) are in line with studies where another extrahepatic organ —the brain —was found to exhibit BA biogenetic capabilities (Pan et al., 2017; Monteiro-Cardoso et al., 2021). To date, the majority of studies focused on investigating the role of the placenta exclusively as an interface across which BAs are transported between the mother to the fetus (Sewell et al., 1980; Itoh et al., 1982; Colombo et al., 1985; Meng et al., 1997; St-Pierre et al., 2000; Tribe et al., 2010; Geenes et al., 2014; Liu et al., 2021; Ontsouka et al., 2021). Available data at https://www.proteinatlas.org, supplemented with mRNA data from (Ontsouka et al., 2023), are compiled in Table 1 and provide indications regarding the potential capacity of the human placenta to synthesize BAs. For most of the enzymes involved in BA biosynthesis, an overlap exists between their expression profiles in the human liver, brain, and placenta (Table 1). However, it should be considered that the BA enzymatic machinery has been described so far only at the mRNA level (Ontsouka et al., 2023) and confirmation at the protein level is still lacking. At this stage, it is important to decipher whether primary and secondary BAs that cross the placenta are only of hepatic/intestinal origin or originate in situ in the placenta because BAs locally synthesized in the placenta may directly affect placental performance and fetal development (Sepúlveda et al., 1991; Egan et al., 2012; De Aguiar Vallim et al., 2013; Lofthouse et al., 2019; Li et al., 2020) (Figure 3). Considering both the recent molecular evidence implying potential placental BA biogenesis (Ontsouka et al., 2023), and the fact that serum BA levels are higher in pregnant compared to non-pregnant women and mice (Milona et al., 2010), the previous belief that solely the enterohepatic axis determines the serum concentration of BAs (Hofmann, 2009) should be re-evaluated. We propose that serum concentrations of BAs during healthy pregnancies (including AHP) are probably maintained thanks to the contribution of all three compartments (mother-placenta-fetus) of the entero-hepato-placental axis (Figure 3). Although the fetal liver and the placenta may contribute to the circulating BA levels within the entero-hepato-placental axis in addition to the maternal organs (Figure 3), their respective relative contributions must still be elucidated.
TABLE 1. Localization of bile acid synthesis-related enzymes in hepatic and selected extrahepatic human tissues.
FIGURE 3. Bile acid transport and signaling pathways in the human placenta. Overview of the molecular mechanisms through which bile acid (BA) transporters and receptors operate at the apical and basal side of the syncytiotrophoblast (STB) layer. On the maternal side (top) BAs interact in an endocrine and probably autocrine/paracrine manner with BA-specific cell surface receptors located on the apical STB membrane. The BA pool in the maternal circulation consists predominantly of primary and secondary BAs. Additional BAs originating from fetal synthesis as well as from potential local synthesis by the placenta are added to the BA pool. BA are transported in both directions across the apical STB layer by specific transporters. At the fetal side of the STB (bottom), BAs interact in an endocrine and possibly autocrine/paracrine manner with specific cell surface receptors located at the basal STB membrane. The BAs in the fetal compartment are a mix of fetal-originating BAs as well as BAs transported across the basal STB membrane originating from the maternal circulation and potentially from local placental synthesis. Within the STB, BAs interact with BA-sensitive nuclear receptors (not depicted) with functional consequences on BA homeostasis (modulation of BA transporters, receptors, and potentially BA synthesis-related enzymes expression). The transport of BAs is primarily mediated by anion/BA exchangers at the basal (fetal) side of the trophoblasts (SLCO2B1, SLCO3A1, SLC51A and SLC51B), whereas the retrograde transport from the trophoblasts towards the mother occurs primarily via ABC transporter proteins (ABCG2, ABCB1, ABCC2, ABCC3 and ABCC4). The predominant direction of BA transport in healthy pregnancies occurs from the fetus to the mother (vertical black arrow, left) while in intrahepatic cholestasis of pregnancy (ICP) there is exacerbated BA transport from the mother to the fetus, exposing the latter to toxic levels (vertical pink arrow, left). Figure created with BioRender.com.
The present manuscript discusses the physiological importance of BAs as signaling molecules and reviews the membrane proteins and mechanisms that sustain the transport of BAs across the placenta, which subsequently contributes to the BA levels in fetal and maternal circulations. Furthermore, the placenta may act not only as an exchange interface but may also serve as a steroidogenic organ capable of synthesizing BAs. These placentally synthesized BAs are possibly added to the maternal and fetal BA pools and may additionally exhibit receptor-mediated autocrine and paracrine effects. Although additional investigations are needed to unequivocally ascertain the capacity of the placenta to synthesize BAs, this possibility opens avenues to explore the importance of in situ BA secretion on the regulation of placental performance, fetal organ growth and maturation, as well as fetal programming of various vulnerabilities.
Conceptualization, EO and CA; writing-original draft preparation, EO; writing-reviewing it critically for important intellectual content review and editing, MS and CA; graphic design, MS. All authors contributed to the article and approved the submitted version.
This research was funded by the Swiss National Science Foundation [Grant No. 310030_197408, CA], the National Center of Competence in Research (NCCR) TransCure [Grant No 51NF40-185544, CA] as well as the Lindenhof Foundation, Bern, Switzerland [Grant No. 17-15-F, CA].
The authors declare that the research was conducted in the absence of any commercial or financial relationships that could be construed as a potential conflict of interest.
All claims expressed in this article are solely those of the authors and do not necessarily represent those of their affiliated organizations, or those of the publisher, the editors and the reviewers. Any product that may be evaluated in this article, or claim that may be made by its manufacturer, is not guaranteed or endorsed by the publisher.
Allikmets, R., Schriml, L. M., Hutchinson, A., Romano-Spica, V., and Dean, M. (1998). A human placenta-specific ATP-binding cassette gene (ABCP) on chromosome 4q22 that is involved in multidrug resistance. Cancer Res. 58, 5337–5339.
Alnouti, Y. (2009). Bile acid sulfation: A pathway of bile acid elimination and detoxification. Toxicol. Sci. 108, 225–246. doi:10.1093/toxsci/kfn268
Aydın, G. A., Özgen, G., and Görükmez, O. (2020). The role of genetic mutations in intrahepatic cholestasis of pregnancy. Taiwan. J. Obstet. Gynecol. 59, 706–710. doi:10.1016/j.tjog.2020.07.014
Azzaroli, F., Mennone, A., Feletti, V., Simoni, P., Baglivo, E., Montagnani, M., et al. (2007). Modulation of human placental multidrug resistance proteins in cholestasis of pregnancy by ursodeoxycholic acid. Aliment. Pharmacol. Ther. 26, 1139–1146.
Azzaroli, F., Raspanti, M. E., Simoni, P., Montagnani, M., Lisotti, A., Cecinato, P., et al. (2013). High doses of ursodeoxycholic acid up-regulate the expression of placental breast cancer resistance protein in patients affected by intrahepatic cholestasis of pregnancy. PLoS One 8, e64101–e64107. doi:10.1371/journal.pone.0064101
Bartke, N., and Hannun, Y. A. (2009). Bioactive sphingolipids: Metabolism and function. J. Lipid Res. 50, S91–S96. doi:10.1194/jlr.R800080-JLR200
Begley, M., Gahan, C. G. M., and Hill, C. (2005). The interaction between bacteria and bile. FEMS Microbiol. Rev. 29, 625–651. doi:10.1016/j.femsre.2004.09.003
Berge, K. E., Tian, H., Graf, G. A., Yu, L., Barnes, R., Hobbs, H. H., et al. (2000). Accumulation of dietary cholesterol in sitosterolemia caused by mutations in adjacent ABC transporters. , 290, 1771–1775. doi:10.1126/science.290.5497.1771
Brouwers, L., Koster, M. P. H., Page-christiaens, G. C. M. L., Kemperman, H., Boon, J., Evers, I. M., et al. (2015). Intrahepatic cholestasis of pregnancy: Maternal and fetal outcomes associated with elevated bile acid levels. Am. J. Obstet. Gynecol. 212, 100.e1–100.e7. doi:10.1016/j.ajog.2014.07.026
Castaño, G., Lucangioli, S., Sookoian, S., Mesquida, M., Lemberg, A., Di Scala, M., et al. (2006). Bile acid profiles by capillary electrophoresis in intrahepatic cholestasis of pregnancy. Clin. Sci. 110, 459–465. doi:10.1042/CS20050302
Chiang, J. Y. L. (2009). Bile acids: Regulation of synthesis. J. Lipid Res. 50, 1955–1966. doi:10.1194/jlr.R900010-JLR200
Chiang, J. Y. L., and Ferrell, J. M. (2019). Bile acids as metabolic regulators and nutrient sensors. Annu. Rev. Nutr. 39, 175–200. doi:10.1146/annurev-nutr-082018-124344
Chuffa, L. G. de A., Lupi, L. A., Cucielo, M. S., Silveira, H. S., Reiter, R. J., and Seiva, F. R. F. (2020). Melatonin promotes uterine and placental health: Potential molecular mechanisms. Int. J. Mol. Sci. 21, 300. doi:10.3390/ijms21010300
Chuffa, L. G. de A., Seiva, F. R. F., Cucielo, M. S., Silveira, H. S., Reiter, R. J., and Lupi, L. A. (2019). Clock genes and the role of melatonin in cancer cells: An overview. Melat. Res. 2, 133–157. doi:10.32794/mr11250026
Colmenero Salas, L. C., Levario Carrillo, M., Torres Reyes, A., González Horta, C., and Sánchez Ramírez, B. (2009). Expression of muscarinic cholinergic receptors in human term placenta. Ginecol. Obstet. Mex. 77, 323–328.
Colombo, C., Roda, A., Roda, E., Buscaglia, M., Dell’Agnola, C. A., Filippetti, P., et al. (1985). Correlation between fetal and maternal serum bile acid concentrations. Pediatr. Res. 19, 227–231. doi:10.1203/00006450-198502000-00018
Colombo, C., Zuliani, G., Ronchi, M., Breidenstein, J., and Setchell, K. D. R. (1987). Biliary bile acid composition of the human fetus in early gestation. Pediatr. Res. 2, 68–71. doi:10.1203/00006450-198702000-00017
De Aguiar Vallim, T. Q., Tarling, E. J., and Edwards, P. A. (2013). Pleiotropic roles of bile acids in metabolism. Cell Metab. 17, 657–669. doi:10.1016/j.cmet.2013.03.013
Délèze, G., Sidiropoulos, D., and Paumgartner, G. (1977). Determination of bile acid concentration in human amniotic fluid for prenatal diagnosis of intestinal obstruction. Pediatrics 59, 647–650. doi:10.1542/peds.59.5.647
Dippe, P. Von, Amoui, M., Stellwagen, R. H., and Levy, D. (1996). The functional expression of sodium-dependent bile acid transport in madin-darby canine kidney cells transfected with the cDNA for microsomal epoxide hydrolase. J. Biol. Chem. 271, 18176–18180. –18180. doi:10.1074/jbc.271.30.18176
Dobierzewska, A., Palominos, M., Sanchez, M., Dyhr, M., Helgert, K., Venegas-Araneda, P., et al. (2016). Impairment of angiogenic sphingosine kinase-1/sphingosine-1-phosphate receptors pathway in preeclampsia. PLoS One 11, e0157221. doi:10.1371/journal.pone.0157221
Dongning, H. E., Meloche, C. A., Dumas, N. A., Frost, A. R., and Falany, C. N. (2004). Different subcellular localization of sulphotransferase 2B1b in human placenta and prostate. Biochem. J. 379, 533–540. doi:10.1042/BJ20031524
Egan, N., Bartels, Ä., Khashan, A. S., Broadhurst, D. I., Joyce, C., O’Mullane, J., et al. (2012). Reference standard for serum bile acids in pregnancy. BJOG Int. J. Obstet. Gynaecol. 119, 493–498. doi:10.1111/j.1471-0528.2011.03245.x
Fisk, N., and Storey, G. (1988). Fetal outcome in obsteric cholestasis. Br. J. Obstet. Gynaecol. 95 (11), 1137–1143. doi:10.1111/j.1471-0528.1988.tb06791.x
Gälman, C., Angelin, B., and Rudling, M. (2005). Bile acid synthesis in humans has a rapid diurnal variation that is asynchronous with cholesterol synthesis. Gastroenterology 129, 1445–1453. doi:10.1053/j.gastro.2005.09.009
Geenes, V., Lövgren-Sandblom, A., Benthin, L., Lawrence, D., Chambers, J., Gurung, V., et al. (2014). The reversed feto-maternal bile acid gradient in intrahepatic cholestasis of pregnancy is corrected by ursodeoxycholic acid. PLoS One 9, 8. doi:10.1371/journal.pone.0083828
Geenes, V., and Williamson, C. (2009). Intrahepatic cholestasis of pregnancy. World J. Gastroenterol. 15, 2049–2066. doi:10.3748/wjg.15.2049
Gerloff, T., Stieger, B., Hagenbuch, B., Madon, J., Landmann, L., Roth, J., et al. (1998). The sister of P-glycoprotein represents the canalicular bile salt export pump of mammalian liver. J. Biol. Chem. 273, 10046–10050. doi:10.1074/jbc.273.16.10046
Glantz, A., Marschall, H.-U., and Mattsson, L.-A. (2004). Intrahepatic cholestasis of pregnancy: Relationships between bile acid levels and fetal complication rates. Hepatology 40, 467–474. doi:10.1002/hep.20336
Gomes, P. R. L., Motta-Teixeira, L. C., Gallo, C. C., Carmo Buonfiglio, D. do, Camargo, L. S., et al. (2021). Maternal pineal melatonin in gestation and lactation physiology, and in fetal development and programming. Gen. Comp. Endocrinol. 300, 113633. doi:10.1016/j.ygcen.2020.113633
González-Mariscal, L., Tapia, R., and Chamorro, D. (2008). Crosstalk of tight junction components with signaling pathways. Biochim. Biophys. Acta - Biomembr. 1778, 729–756. doi:10.1016/j.bbamem.2007.08.018
Guo, C., Chen, W. D., and Wang, Y. D. (2016). TGR5, not only a metabolic regulator. Front. Physiol. 7, 646. doi:10.3389/fphys.2016.00646
Herraez, E., Lozano, E., Poli, E., Keitel, V., De Luca, D., Williamson, C., et al. (2014). Role of macrophages in bile acid-induced inflammatory response of fetal lung during maternal cholestasis. J. Mol. Med. 92, 359–372. doi:10.1007/s00109-013-1106-1
Hofmann, A. F. (2009). The enterohepatic circulation of bile acids in mammals: form and functions. Front Biosci (Landmark Ed) 14 (7), 2584–2598. doi:10.2741/3399
Huang, W., Zhou, J., Guo, J., Hu, W., Chen, G., Li, B., et al. (2021). Dexamethasone induces an imbalanced fetal-placental-maternal bile acid circulation: Involvement of placental transporters. BMC Med. 19, 87. doi:10.1186/s12916-021-01957-y
Huber, R. D., Gao, B., Pfändler, M. S., Zhang-fu, W., Leuthold, S., Hagenbuch, B., et al. (2006). Characterization of two splice variants of human organic anion transporting polypeptide 3A1 isolated from human brain. Am. J. Physiol. Cell Physiol. 806, 795. doi:10.1152/ajpcell.00597.2005
Ibrahim, E., Diakonov, I., Arunthavarajah, D., Swift, T., Goodwin, M., McIlvride, S., et al. (2018). Bile acids and their respective conjugates elicit different responses in neonatal cardiomyocytes: Role of Gi protein, muscarinic receptors and TGR5. Sci. Rep. 8, 7110. doi:10.1038/s41598-018-25569-4
Itoh, S., Onishi, S., Isobe, K., Manabe, M., and Inukai, K. (1982). Foetomaternal relationships of serum bile acid pattern estimated by high-pressure liquid chromatography. Biochem. J. 204, 141–145. doi:10.1042/bj2040141
Iwasaki, S., Nakazawa, K., Sakai, J., Kometani, K., Iwashita, M., Yoshimura, Y., et al. (2005). Melatonin as a local regulator of human placental function. J. Pineal Res. 39, 261–265. doi:10.1111/j.1600-079X.2005.00244.x
Jacquemin, E., Cresteil, D., Manouvrier, S., Boute, O., and Hadchouel, M. (1999). Heterozygous non-sense mutation of the MDR3 gene in familial intrahepatic cholestasis of pregnancy. Lancet 353, 210–211. doi:10.1016/S0140-6736(05)77221-4
Johnstone, E. D., Chan, G., Sibley, C. P., Davidge, S. T., Lowen, B., and Guilbert, L. J. (2005). Sphingosine-1-phosphate inhibition of placental trophoblast differentiation through a Gi-coupled receptor response. J. Lipid Res. 46, 1833–1839. doi:10.1194/jlr.M500095-JLR200
Karahoda, R., Kallol, S., Groessl, M., Ontsouka, E., Anderle, P., Fluck, C., et al. (2021). Revisiting steroidogenic pathways in the human placenta and primary human trophoblast cells. Int. J. Mol. Sci. 22, 1704. doi:10.3390/ijms22041704
Kawamata, Y., Fujii, R., Hosoya, M., Harada, M., Yoshida, H., Miwa, M., et al. (2003). A G protein-coupled receptor responsive to bile acids. J. Biol. Chem. 278, 9435–9440. doi:10.1074/jbc.M209706200
Keitel, V., Reich, M., and Häussinger, D. (2015). TGR5: Pathogenetic role and/or therapeutic target in fibrosing cholangitis? Clin. Rev. Allergy Immunol. 48, 218–225. doi:10.1007/s12016-014-8443-x
Klindt, C., Reich, M., Hellwig, B., Stindt, J., Rahnenführer, J., Hengstler, J. G., et al. (2019). The G protein-coupled bile acid receptor TGR5 (Gpbar1) modulates endothelin-1 signaling in liver. Cells. 1. 1. doi:10.3390/cells8111467
König, J., Cui, Y., Nies, A. T., and Keppler, D. (2000). A novel human organic anion transporting polypeptide localized to the basolateral hepatocyte membrane. Am. J. Physiol. Gastrointest. Liver Physiol. 278, G156–G164. doi:10.1152/ajpgi.2000.278.1.G156
Lam, P., Wang, R., and Ling, V. (2005). Bile acid transport in sister of P-glycoprotein (ABCB11) knockout mice. Biochemistry 44, 12598–12605. doi:10.1021/bi050943e
Lammert, F., Marschall, H.-U., Glantz, A., and Matern, S. (2000). Intrahepatic cholestasis of pregnancy: Molecular pathogenesis, diagnosis and management. J. Hepatol. 33, 1012–1021. doi:10.1016/S0168-8278(00)80139-7
Lanoix, D., Guérin, P., and Vaillancourt, C. (2012). Placental melatonin production and melatonin receptor expression are altered in preeclampsia: New insights into the role of this hormone in pregnancy. J. Pineal Res. 53, 417–425. doi:10.1111/j.1600-079X.2012.01012.x
Li, S., Jia, Z. H., Wei, X. R., Ma, S., Lu, T. C., Li, T. T., et al. (2020). Role of bile acid on maintaining metabolic homeostasis. J. Shanghai Jiaot. Univ. Med. Sci. doi:10.3969/j.issn.1674-8115.2020.08.021
Liu, F., Zhou, J., Guo, J., Huang, W., Zhang, W., and Wang, H. (2021). Prenatal ethanol exposure increases maternal bile acids through placental transport pathway. Toxicology 458, 152848. doi:10.1016/j.tox.2021.152848
Lofthouse, E. M., Torrens, C., Manousopoulou, A., Nahar, M., Cleal, J. K., O’Kelly, M. I., et al. (2019). Ursodeoxycholic acid inhibits uptake and vasoconstrictor effects of taurocholate in human placenta. FASEB J. 33, 8211–8220. doi:10.1096/fj.201900015RR
Manzotti, C., Casazza, G., Stimac, T., Nikolova, D., and Gluud, C. (2019). Total serum bile acids or serum bile acid profile, or both, for the diagnosis of intrahepatic cholestasis of pregnancy. Cochrane Database Syst. Rev. 7, CD012546. doi:10.1002/14651858.CD012546.pub2
Maruyama, T., Miyamoto, Y., Nakamura, T., Tamai, Y., Okada, H., Sugiyama, E., et al. (2002). Identification of membrane-type receptor for bile acids (M-BAR). Biochem. Biophys. Res. Commun. 298, 714–719. doi:10.1016/S0006-291X(02)02550-0
Mathias, A. A., Hitti, J., and Unadkat, J. D. (2005). P-glycoprotein and breast cancer resistance protein expression in human placentae of various gestational ages. Am. J. Physiol. - Regul. Integr. Comp. Physiol. 289, 963–969. doi:10.1152/ajpregu.00173.2005
Meng, L. J., Reyes, H., Palma, J., Hernandez, I., Ribalta, J., and Sjövall, J. (1997). Profiles of bile acids and progesterone metabolites in the urine and serum of women with intrahepatic cholestasis of pregnancy. J. Hepatol. 27, 346–357. doi:10.1016/S0168-8278(97)80181-X
Meyer Zu Schwabedissen, H. E., Jedlitschky, G., Gratz, M., Haenisch, S., Linnemann, K., Fusch, C., et al. (2005). Variable expression of MRP2 (ABCC2) in human placenta: Influence of gestational age and cellular differentiation. Drug Metab. Dispos. 33, 896–904. doi:10.1124/dmd.104.003335
Miki, Y., Nakata, T., Suzuki, T., Darnel, A. D., Moriya, T., Kaneko, C., et al. (2002). Systemic distribution of steroid sulfatase and estrogen sulfotransferase in human adult and fetal tissues. J. Clin. Endocrinol. Metab. 87, 5760–5768. doi:10.1210/jc.2002-020670
Milona, A., Owen, B. M., Cobbold, J. F. L., Willemsen, E. C. L., Cox, I. J., Boudjelal, M., et al. (2010). Raised hepatic bile acid concentrations during pregnancy in mice are associated with reduced farnesoid X receptor function. Hepatology 52, 1341–1349. doi:10.1002/hep.23849
Mizugishi, K., Li, C., Olivera, A., Bielawski, J., Bielawska, A., Deng, C. X., et al. (2007). Maternal disturbance in activated sphingolipid metabolism causes pregnancy loss in mice. J. Clin. Invest. 117, 2993–3006. doi:10.1172/JCI30674
Monteiro-Cardoso, V. F., Corlianò, M., and Singaraja, R. R. (2021). Bile acids: A communication channel in the gut-brain Axis. NeuroMolecular Med. 23, 99–117. doi:10.1007/s12017-020-08625-z
Müllenbach, R., Bennett, A., Tetlow, N., Patel, N., Hamilton, G., Cheng, F., et al. (2005). ATP8B1 mutations in British cases with intrahepatic cholestasis of pregnancy. GUT 54, 829–834. doi:10.1136/gut.2004.058115
Niefan, Y., Hu, J., and Yan, X. hua (2015). Cross-talk between bile acids and intestinal microbiota in host metabolism and health. J. Zhejiang Univ. Sci. B 16, 436–446. doi:10.1631/jzus.B1400327
Ontsouka, E., Epstein, A., Kallol, S., Zaugg, J., Baumann, M., Schneider, H., et al. (2021). Placental expression of bile acid transporters in intrahepatic cholestasis of pregnancy. Int. J. Mol. Sci. 22, 10434,. doi:10.3390/ijms221910434
Ontsouka, E., Schroeder, M., Ok, L., Vaillancourt, C., Stroka, D., and Albrecht, C. (2023). The placenta — a new source of bile acids during healthy pregnancy ? First results of a gene expression study in humans and mice. Int. J. Mol. Sci. 24, 1–12. doi:10.3390/ijms24119511
Pan, X., Elliott, C. T., McGuinness, B., Passmore, P., Kehoe, P. G., Hölscher, C., et al. (2017). Metabolomic profiling of bile acids in clinical and experimental samples of Alzheimer’s disease. Metabolites 7, 28. doi:10.3390/metabo7020028
Parker, A., Fonseca, S., and Carding, S. R. (2020). Gut microbes and metabolites as modulators of blood-brain barrier integrity and brain health. Gut Microbes 11, 135–157. doi:10.1080/19490976.2019.1638722
Pascual, M. J., Serrano, M. A., El-Mir, M. Y., Macias, R. I. R., Jiménez, F., and Marin, J. J. G. (2002). Relationship between asymptomatic hypercholanaemia of pregnancy and progesterone metabolism. Clin. Sci. 102, 587. doi:10.1042/CS20010258
Patel, P., Weerasekera, N., Hitchins, M., Boyd, C., Johnston, D., and Williamson, C. (2003). Semi quantitative expression analysis of MDR3, FIC1, BSEP, OATP-A, OATP-C,OATP-D, OATP-E and NTCP gene transcripts in 1st and 3rd trimester human placenta. Placenta 24, 39–44. doi:10.1053/plac.2002.0879
Pathak, P., Xie, C., Nichols, R. G., Ferrell, J. M., Boehme, S., Krausz, K. W., et al. (2018). Intestine farnesoid X receptor agonist and the gut microbiota activate G-protein bile acid receptor-1 signaling to improve metabolism. Hepatology 68, 1574–1588. doi:10.1002/hep.29857
Perez, M. J., and Britz, O. (2009). Bile-acid-induced cell injury and protection. World J. Gastroenterol. 15, 1677–1689. doi:10.3748/wjg.15.1677
Petrovic, V., Kojovic, D., Cressman, A., and Piquette-Miller, M. (2015). Maternal bacterial infections impact expression of drug transporters in human placenta. Int. Immunopharmacol. 26, 349–356. doi:10.1016/j.intimp.2015.04.020
Poole, P. A., and Duane, W. C. (1988). Effects of bile acid administration on bile acid synthesis and its circadian rhythm in man. Hepatology 8, 1140–1146. doi:10.1002/hep.1840080530
Pradhan-Sundd, T., and Monga, S. P. (2019). Blood-bile barrier: Morphology, regulation, and pathophysiology. Gene Expr. J. Liver Res. 19, 69–87. doi:10.3727/105221619X15469715711907
Rajagopal, S., Kumar, D. P., Mahavadi, S., Bhattacharya, S., Zhou, R., Corvera, C. U., et al. (2013). Activation of G protein-coupled bile acid receptor, TGR5, induces smooth muscle relaxation via both Epac- and PKA-mediated inhibition of RhoA/Rho kinase pathway. Am. J. Physiol. - Gastrointest. Liver Physiol. 304, G527–G535. doi:10.1152/ajpgi.00388.2012
Raufman, J. P., Chen, Y., Cheng, K., Compadre, C., Compadre, L., and Zimniak, P. (2002). Selective interaction of bile acids with muscarinic receptors: A case of molecular mimicry. Eur. J. Pharmacol. 457, 77–84. doi:10.1016/S0014-2999(02)02690-0
Ridlon, J. M., Harris, S. C., Bhowmik, S., Kang, D. J., and Hylemon, P. B. (2016). Consequences of bile salt biotransformations by intestinal bacteria. Gut Microbes 7, 22–39. doi:10.1080/19490976.2015.1127483
Roth, M., Obaidat, A., and Hagenbuch, B. (2012). OATPs, OATs and OCTs: The organic anion and cation transporters of the SLCO and SLC22A gene superfamilies. Br. J. Pharmacol. 165, 1260–1287. doi:10.1111/j.1476-5381.2011.01724.x
Russell, D. W., and Setchell, K. D. R. (1992). Bile acid biosynthesis. Biochemistry 31, 4737–4749. doi:10.1021/bi00135a001
Russell, D. W. (2003). The enzymes, regulation, and genetics of bile acid synthesis. Annu. Rev. Biochem. 72, 137–174. doi:10.1146/annurev.biochem.72.121801.161712
Ryu, Y., Takuwa, N., Sugimoto, N., Sakurada, S., Usui, S., Okamoto, H., et al. (2002). Sphingosine-1-phosphate, a platelet-derived lysophospholipid mediator, negatively regulates cellular Rac activity and cell migration in vascular smooth muscle cells. Circ. Res. 90, 325–332. doi:10.1161/hh0302.104455
Sasaki, H. (1984). Development of bile acid metabolism in neonates during perinatal period Part 1: Bile acid levels in sera of mothers, fetuses and neonates. Pediatr. Int. 26, 150–160. doi:10.1111/j.1442-200X.1984.tb01807.x
Sastry, B. V. R. (1997). Human placental cholinergic system. Biochem. Pharmacol. 53, 1577–1586. doi:10.1016/S0006-2952(97)00017-8
Schaap, F. G., Trauner, M., and Jansen, P. L. M. (2014). Bile acid receptors as targets for drug development. Nat. Rev. Gastroenterol. Hepatol. 11, 55–67. doi:10.1038/nrgastro.2013.151
Sender, R., Fuchs, S., and Milo, R. (2016). Revised estimates for the number of human and bacteria cells in the body. PLoS Biol. 14, e1002533. doi:10.1371/journal.pbio.1002533
Sepúlveda, W. H., González, C., Cruz, M. A., and Rudolph, M. I. (1991). Vasoconstrictive effect of bile acids on isolated human placental chorionic veins. Eur. J. Obstet. Gynecol. Reprod. Biol. 42, 211–215. doi:10.1016/0028-2243(91)90222-7
Sewell, R. B., Hardy, K. J., Smallwood, R. A., and Hoffman, N. E. (1980). Fetal bile salt metabolism: Placental transfer of taurocholate in sheep. Am. J. Physiol. - Gastrointest. Liver Physiol. 239, G354–G357. doi:10.1152/ajpgi.1980.239.5.g354
Sieppi, E., Vähäkangas, K., Rautio, A., Ietta, F., Paulesu, L., and Myllynen, P. (2016). The xenoestrogens, bisphenol A and para-nonylphenol, decrease the expression of the ABCG2 transporter protein in human term placental explant cultures. Mol. Cell. Endocrinol. 429, 41–49. doi:10.1016/j.mce.2016.03.034
Singh, A. T., Dharmarajan, A., Aye, I. L. M. H., and Keelan, J. A. (2012). Sphingosine-sphingosine-1-phosphate pathway regulates trophoblast differentiation and syncytialization. Reprod. Biomed. Online. 24, 224–234. doi:10.1016/j.rbmo.2011.10.012
St-Pierre, M. V., Serrano, M. a., Macias, R. I., Dubs, U., Hoechli, M., Lauper, U., et al. (2000). Expression of members of the multidrug resistance protein family in human term placenta. Am. J. Physiol. Regul. Integr. Comp. Physiol. 279, R1495–R1503. doi:10.1152/ajpregu.2000.279.4.R1495
St-Pierre, M. V., Stallmach, T., Grundschober, A. F., Dufour, J., Serrano, M. A., Marin, J. J. G., et al. (2004). Temporal expression profiles of organic anion transport proteins in placenta and fetal liver of the rat. Am J Physiol Regul Integr Comp Physiol, 287, 1. doi:10.1152/ajpregu.00279.2003
Stanley, E. L., Hume, R., Visser, T. J., and Coughtrie, M. W. H. (2001). Differential expression of sulfotransferase enzymes involved in thyroid hormone metabolism during human placental development. J. Clin. Endocrinol. Metab. 86, 5944–5955. doi:10.1210/jcem.86.12.8081
Sundseth, S. S., and Waxman, D. J. (1990). Hepatic P-450 cholesterol 7 alpha-hydroxylase. Regulation in vivo at the protein and mRNA level in response to mevalonate, diurnal rhythm, and bile acid feedback. J. Biol. Chem. 265, 15090–15095. doi:10.1016/s0021-9258(18)77227-x
Tamai, I., Nezu, J. I., Uchino, H., Sai, Y., Oku, A., Shimane, M., et al. (2000). Molecular identification and characterization of novel members of the human organic anion transporter (OATP) family. Biochem. Biophys. Res. Commun. 273, 251–260. doi:10.1006/bbrc.2000.2922
Tang, X., Halleck, M., Schlegel, R., and Williamson, P. (1996). A subfamily of P-type ATPases with aminophospholipid transporting activity. , 272, 1495, 1497. doi:10.1126/science.272.5267.1495
Tayebati, S. K., Sabbatini, M., Zaccheo, D., and Amenta, F. (1997). Muscarinic cholinergic receptor subtypes expression by human placenta. Neurosci. Lett. 221, 208–212. doi:10.1016/S0304-3940(96)13311-5
Thomas, C., Pellicciari, R., Pruzanski, M., Auwerx, J., and Schoonjans, K. (2008). Targeting bile-acid signalling for metabolic diseases. Nat. Rev. Drug Discov. 7, 678–693. doi:10.1038/nrd2619
Ticho, A. L., Malhotra, P., Dudeja, P. K., Gill, R. K., and Alrefai, W. A. (2019). Bile acid receptors and gastrointestinal functions. Liver Res. 3, 31–39. doi:10.1016/j.livres.2019.01.001
Tribe, R. M., Dann, A. T., Kenyon, A. P., Seed, P., Shennan, A. H., and Mallet, A. (2010). Longitudinal profiles of 15 serum bile acids in patients with intrahepatic cholestasis of pregnancy. Am. J. Gastroenterol. 105, 585–595. doi:10.1038/ajg.2009.633
Williamson, C., Hems, L. M., Goulis, D. G., Walker, I., Chambers, J., Donaldson, O., et al. (2004). Clinical outcome in a series of cases of obstetric cholestasis identified via a patient support group. BJOG 111, 676–681. doi:10.1111/j.1471-0528.2004.00167.x
Yatomi, Y., Ozaki, Y., Ohmori, T., and Igarashi, Y. (2001). Sphingosine 1-phosphate: Synthesis and release. Prostagl. Other Lipid Mediat 64, 107–122. doi:10.1016/S0090-6980(01)00103-4
Yeboah, D., Sun, M., Kingdom, J., Baczyk, D., Lye, S. J., Matthews, S. G., et al. (2006). Expression of breast cancer resistance protein (BCRP/ABCG2) in human placenta throughout gestation and at term before and after labor. Can. J. Physiol. Pharmacol. 84, 1251–1258. doi:10.1139/Y06-078
Zagrean, A. M., Chitimus, D. M., Badiu, C., Panaitescu, A. M., Peltecu, G., and Zagrean, L. (2019). “The pineal gland and its function in pregnancy and lactation,” in Maternal-fetal and neonatal endocrinology: Physiology, pathophysiology, and clinical management. doi:10.1016/B978-0-12-814823-5.00002-7
Zelcer, N., Reid, G., Wielinga, P., Kuil, A., Heijden, I. V. A. N. D. E. R., Schuetz, J. D., et al. (2003). Steroid and bile acid conjugates are substrates of human multidrug-resistance protein (MRP) 4 (ATP-binding cassette C4). Biochem. J. 367, 361–367. doi:10.1042/BJ20021886
Zhan, K., Zheng, H., Li, J., Wu, H., Qin, S., Luo, L., et al. (2020). Gut microbiota-bile acid crosstalk in diarrhea-irritable bowel syndrome. Biomed. Res. Int. 2020, 3828249. doi:10.1155/2020/3828249
Zhang, J., Dunk, C. E., and Lye, S. J. (2013). Sphingosine signalling regulates decidual NK cell angiogenic phenotype and trophoblast migration. Hum. Reprod. 28, 3026–3037. doi:10.1093/humrep/det339
Zhu, H. L., Shi, X. T., Xu, X. F., Zhou, G. X., Xiong, Y. W., Yi, S. J., et al. (2021). Melatonin protects against environmental stress-induced fetal growth restriction via suppressing ROS-mediated GCN2/ATF4/BNIP3-dependent mitophagy in placental trophoblasts. Redox Biol. 40, 101854. doi:10.1016/j.redox.2021.101854
Keywords: bile acid signaling, bile acid synthesis, fetal development, placenta, pregnancy
Citation: Ontsouka E, Schroeder M and Albrecht C (2023) Revisited role of the placenta in bile acid homeostasis. Front. Physiol. 14:1213757. doi: 10.3389/fphys.2023.1213757
Received: 28 April 2023; Accepted: 03 July 2023;
Published: 21 July 2023.
Edited by:
Michelle M. Collins, University of Saskatchewan, CanadaReviewed by:
Stephanie Wesolowski, University of Colorado, United StatesCopyright © 2023 Ontsouka, Schroeder and Albrecht. This is an open-access article distributed under the terms of the Creative Commons Attribution License (CC BY). The use, distribution or reproduction in other forums is permitted, provided the original author(s) and the copyright owner(s) are credited and that the original publication in this journal is cited, in accordance with accepted academic practice. No use, distribution or reproduction is permitted which does not comply with these terms.
*Correspondence: Christiane Albrecht, Y2hyaXN0aWFuZS5hbGJyZWNodEB1bmliZS5jaA==
Disclaimer: All claims expressed in this article are solely those of the authors and do not necessarily represent those of their affiliated organizations, or those of the publisher, the editors and the reviewers. Any product that may be evaluated in this article or claim that may be made by its manufacturer is not guaranteed or endorsed by the publisher.
Research integrity at Frontiers
Learn more about the work of our research integrity team to safeguard the quality of each article we publish.