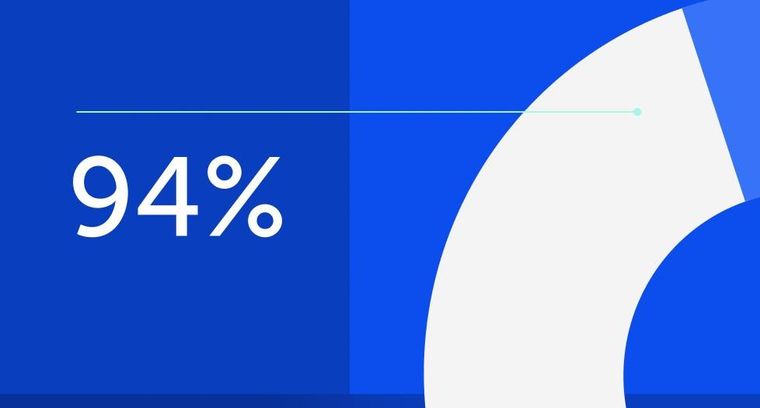
94% of researchers rate our articles as excellent or good
Learn more about the work of our research integrity team to safeguard the quality of each article we publish.
Find out more
ORIGINAL RESEARCH article
Front. Physiol., 21 June 2023
Sec. Invertebrate Physiology
Volume 14 - 2023 | https://doi.org/10.3389/fphys.2023.1213654
Parts of this article's content have been modified or rectified in:
Erratum: Roles of GFAT and PFK genes in energy metabolism of brown planthopper, Nilaparvata lugens
Glutamine:fructose-6-phosphate aminotransferases (GFATs) and phosphofructokinase (PFKs) are the principal rate-limiting enzymes involved in hexosamine biosynthesis pathway (HBP) and glycolysis pathway, respectively. In this study, the NlGFAT and NlPFK were knocked down through RNA interference (RNAi) in Nilaparvata lugens, the notorious brown planthopper (BPH), and the changes in energy metabolism were determined. Knockdown of either NlGFAT or NlPFK substantially reduced gene expression related to trehalose, glucose, and glycogen metabolism pathways. Moreover, trehalose content rose significantly at 72 h after dsGFAT injection, and glycogen content increased significantly at 48 h after injection. Glucose content remained unchanged throughout the experiment. Conversely, dsPFK injection did not significantly alter trehalose, but caused an extreme increase in glucose and glycogen content at 72 h after injection. The Knockdown of NlGFAT or NlPFK significantly downregulated the genes in the glycolytic pathway, as well as caused a considerable and significant decrease in pyruvate kinase (PK) activity after 48 h and 72 h of inhibition. After dsGFAT injection, most of genes in TCA cycle pathway were upregulated, but after dsNlPFK injection, they were downregulated. Correspondingly, ATP content substantially increased at 48 h after NlGFAT knockdown but decreased to an extreme extent by 72 h. In contrast, ATP content decreased significantly after NlPFK was knocked down and returned. The results have suggested the knockdown of either NlGFAT or NlPFK resulted in metabolism disorders in BPHs, highlighting the difference in the impact of those two enzyme genes on energy metabolism. Given their influence on BPHs energy metabolism, developing enzyme inhibitors or activators may provide a biological control for BPHs.
Insects depend on a constant intake of energy to maintain physiological processes, and energy metabolism occures throughout their body. Unlike mammals, insects primarily utilize trehalose for energy instead of glucose (Yasugi et al., 2017). Trehalose is a disaccharide that serves as the fundamental carbohydrate constituent of hemolymph, and is highly stable. It is found in various organisms, including bacteria, yeast, fungi, nematodes, insects, invertebrates, and plants, but is absent in mammals (Elbein et al., 2003; Wen et al., 2016; Wang et al., 2020). Trehalose plays crucial role in acclimating to various abiotic stresses, such as high or low temperatures, nutrition or starvation, oxidation stress, high osmotic pressure, toxic substances, and UV-B irradiation (Tamang et al., 2017; Chen et al., 2018). Moreover, trehalose can serve as an instant source of energy. Trehalose-6-phosphate synthase (TPS) is responsible for the conversion of uridine diphosphate glucose (UDP-glucose) and glucose-6-phosphate (G-6-P) to trehalose-6-phosphate, which then undergoes dephosphorylation by trehalose-6-phosphate phosphatase (TPP) to produce trehalose. This pathway of trehalose synthesis is the most important in insects known to date (Shukla et al., 2015; Tang et al., 2017; Chen et al., 2018).
Trehalase (TRE) is the sole disaccharidase that degrades trehalose in insects, catalyzing its conversion to glucose when energy is needed (Barraza and Sánchez, 2013; Luo et al., 2022). The resulting glucose is utilized in the glycolysis-tricarboxylic acid (glycolysis-TCA) cycle, which converts glucose into pyruvate via a series of enzymatic reactions. These reactions involve hexokinase (HK), glucose-6-phosphate isomerase (G6PI), phosphofructokinase (PFK) and pyruvate kinase (PK) (Hu et al., 2016; Hassan et al., 2022). The reverse process of glycolysis is gluconeogenesis, which is accomplished fructose-1,6-bisphosphatase (FBP). Pyruvate is then converted into acetyl-coenzyme A (acetyl-CoA) which combines with oxaloacetic acid to enter TCA cycle, resulting in the generation of multiple adenosine triphosphate (ATP) (Hu et al., 2016). Insects have the ability to regulate the rate of glycolysis-TCA cycle to accommodate various physiological activities. For example, Drosophila melanogaste, Bombyx mori, and Spodoptera litura, decrease the relative expression of genes encoding glycolysis or TCA metabolic enzymes during early pupal development in order to conserve energy for organogenesis (White et al., 1999; Tian et al., 2010; Hu et al., 2016).
In addition to being converted into trehalose, glucose from the environment in insects can also converted into glycogen, which is another critical metabolic and energy substance (Tolmasky et al., 2001; Liu et al., 2009). Glycogen is synthesized and stored in the fat body, and then can be quickly converted into glucose or trehalose and transported to other tissues (Tang et al., 2012). The synthesis and degradation of glycogen molecules are regulated by the activity of enzymes, primarily by glycogen synthase (GS) and glycogen phosphorylase (GP), respectively (Prats et al., 2005). GS use UDP-glucose as its sole substrate to synthesize glycogen from glucose monomers, while GP breaks down the glycogen. Notably, UDP-glucose is also critical for the synthesis of trehalose in insects (Tang et al., 2012). During high-energy behaviors like flight, insects transfer trehalose from their hemolymph to their flight muscles for instant energy supply. Therefore, glycogen stored in the fat body must be converted into trehalose to maintain sufficient hemolymph trehalose concentration (Yu et al., 2008). Furthermore, some insects, such as the ladybird Coccinella septempunctata Linnaeus (Ren et al., 2015) and Zygaena trifolii (Esper) (Lepidoptera: Zygaenidae), accumulate adequate glycogen in preparation for entering diapause (Wipking et al., 1995).
Fructose-6-phosphate (F-6-P) is produced by G6PI catalyzing the isomerization of G-6-P and is an important intermediate substance in the conversion process of trehalose, glucose, and glycogen, which is crucial in energy metabolism (Achari et al., 1981). F-6-P enters various metabolic pathways, depending on enzymatic conversion. One pathway for F-6-P is conversion into fructose-1,6-diphosphate, an important intermediate in glycolysis that is catalyzed by phosphofructokinase (PFK). PFK is a highly conserved enzyme in the glycolytic pathway and is the main rate-limiting enzyme. The activity of PFK has a significant impact on both glucose consumption and energy production (Jojima and Inui, 2015). PFK in vertebrates is activated by ADP, AMP, and fructose-2,6-diphosphate, while it is inhibited by physiological levels of ATP and citrate (Martínez-Costa et al., 2004). However, PFK in insect does not be inhibited by citrate (Newsholme et al., 1977; Nunes et al., 2016). Another pathway for F-6-P is to generate glucosamine-6-phosphate under the action of glutamine:fructose-6-phosphate aminotransferase (GFAT) and participate in hexosamine biosynthesis pathway (HBP) to chitin production (Zhu et al., 2016). Chitin is a linear polymer composed of N-acetylglucosamine units linked by β-1,4-glycoside bonds and it is a major component of exoskeleton, trachea and the peritrophic matrix that lines the midgut epithelium (Merzendorfer and Zimoch, 2003; Tharanathan and Kittur, 2003; Xi et al., 2015; Liu et al., 2019). Since GFAT is the first and rate-limiting enzyme of HBP, it significantly impacts on chitin synthesis (Denzel and Antebi, 2015). Overall, PFK and GFAT are two crucial enzymes in the energy metabolism pathway of insects.
Nilaparvata lugens, commonly known as the brown planthopper (BPH), is one of the most destructive and notorious rice pests in Asia and responsible for serious crop losses (Zhou et al., 2018; Li et al., 2021). BPHs have high fecundity and feed on rice sap, oviposit in rice tissues, and transmit viruses such as grassy stunt virus and rugged stunt virus (Sun et al., 2017; Bing et al., 2019). The BPH is not only abundant, but it is also prone to developing insecticide resistance easily (Mu et al., 2016). Therefore, it is difficult to manage BPH with standard chemical methods and should be manage through natural enemies (Liu et al., 2022). In this study, RNA interference (RNAi), a commonly used method for studying of insect gene function (Ullah et al., 2022; Zhou et al., 2022), was employed to suppress the expression of NlGFAT and NlPFK genes. This analysis revealed the impacts of these two enzymes on insect energy metabolism, opening up new opportunities for pest control.
The BPH colonies used in this experiment were provided by the China National Rice Research Institute (Hangzhou, China), and were kept in laboratory for at least 30 generations. The rice (Oryza sativa L.) used in this study was Taichung Native 1 (TN1) and was grown in cement tanks (60 cm × 30 cm × 100 cm). Insects were reared on fresh rice seedlings in an artificial climate chamber at 26°C ± 1°C, 70% relative humidity, and 16L:8D (light: dark) photoperiod.
The microinjected insects were first day, fifth instar nymph BPH. Each treatment comprised 240 nymphs divided into 12 replicates of 20 individuals per replicate. Three replicates were used for each of total RNA isolation, sugar content determination, and enzyme activity determination, while one additional replicate was used for ATP content determination. Insects were collected at 48 h and 72 h after injection and stored at −80°C for determination of gene expression, sugar content, enzyme activity, and ATP content. All experiments were independently conducted three times for biological replication.
Total RNA was extracted using Trizol from five randomly selected individuals for each treatment (Invitrogen, Carlsbad, California, United States), following the manufacturer’s instructions. RNA integrity was determined with 1% agarose gel electrophoresis, and RNA concentration and purity were assessed with a Nanodrop 2,000 spectrophotometer (Thermo Fisher Scientific, Waltham, MA, United States) by measuring absorbance at 260 nm. Purified RNA was stored at −80°C for subsequent experiments. First-strand complementary DNA (cDNA) was synthesized using the PrimeScript RT reagent kit with gDNA Eraser (Takara, Kyoto, Japan) following the manufacturer’s instructions and stored at −20°C.
The dsDNA fragments from NlGFAT (OR058797) and NlPFK (OR058799) genes were amplified by PCR using specific primers containing the T7 promoter sequence at their 5′ ends (Table 1). The PCR amplification was carried out under the following conditions: preincubation at 95°C for 3 min, 35 cycles at 95°C for 30 s, 55°C for 30 s, 72°C for 1 min, and a last extension at 72°C for 10 min. The purified amplification products of NlGFAT and NlPFK were used to synthesize dsRNA by in vitro transcription using T7 RiboMax Express RNAi System (Promega, Madison, WI). The dsRNA obtained from green fluorescence protein (GFP) gene was used as a control. The sense and anti-sense strands were first produced in two separate transcription procedures and then mixed for annealing. The reaction mixture was incubated at 70°C for 10 min and then cooled on an ice bath for 20 min. The dsRNAs were then precipitated with 95% ethanol and 3 M sodium acetate (pH 5.2), washed with 70% ethanol, air dried, and resuspended. The integrity and quantity of dsRNAs were evaluated by spectroscopy analysis with Nanodrop 2,000 and by 1% agarose gel electrophoresis (Zhang et al., 2017). The synthesized dsRNA was stored at −80°C.
The abdomen of each BPH (on 1st day of the 5th instar nymphs) between the second pair of peids and the third pair of pedis was injected with 3,000 ng of dsGFAT and dsPFK (of each) using an IM-31 microinjector (NARISHIGE, Tokyo, Japan). The control groups were injected with dsGFP. As the interference efficiency of the same dsRNA was previously determined in the study (Xu et al., 2021), it was not determined separately in current study.
The total RNA of BPHs collected after dsRNA injection were extracted and reverse transcribed into cDNA, which was used as a template. Specific primers were selected (Table 2). The relative expression of genes was estimated by qRT-PCR with a SYBR Green master mix (Takara) in a CFX96TM Real-Time PCR Detection System (Bio-Rad, Hercules, CA, United States). Each PCR was performed in a 20 μL volume, containing 1 μL cDNA, 1 μL (10 µM) each primer, 7 μL ultrapure water, and 10 μL SYBR buffer. The reaction was performed with following conditions: preincubation at 95°C for 2 min, followed by 39 cycles of 95°C for 5 s and annealing at 59°C for 30 s, with a melting curve at 65–95°C. Amplification of 18 S RNA was used as an internal control. The 2−△△CT method was used for the analysis of relative gene expression (Livak and Schmittgen, 2001).
TRE in BPH is classified into soluble trehalase (TRE1) and membrane-bound trehalase (TRE2). Thirty BPH individuals collected after dsRNA injection were homogenized in 200 μL phosphate-buffered saline (PBS; pH 7.0), and then mixed with 800 μL of PBS were added. Subsequently, the homogenate was centrifuged at 1,000 g for 20 min at 4°C. The supernatant (300 μL) was taken to detect concentration of protein, trehalose and glycogen and the resting the supernatant (350 μL) was removed and ultracentrifuged at 20,800 g for 60 min at 4°C. The supernatant (300 μL) obtained from ultracentrifugation was used to determine TRE1 activity and concentration of protein and glucose. The sediment was suspended in PBS (300 μL) and was used for the determination of TRE2 activity and concentration of protein and glucose.
The previous method with proper modifications was used to TRE activity assay (Tatun et al., 2008a; Tatun et al., 2008b). Anthrone method was used to determination of trehalose (Zhang et al., 2017). The glucose content was determined by glucose assay (Sigma-Aldrich, St. Louis, MO, United States). The glycogen content was also determined by glucose assay after converting to glucose under the action of amyloglucosidase (Sigma-Aldrich) (Yang et al., 2017). The protein concentration was determined to calculate the content of glycogen, trehalose and glucose, following the BCA Protein Assay Kit (Beyotime, Shanghai, China) according to the manufacturer’s instruction.
The BPHs were mixed with 1× PBS for grinding and crushing to obtain 10% homogenate, and the experiment was carried out according to the instruction of Pyruvate Kinase Assay Kit (Jiancheng, Nanjing, China) and Malate Dehydrogenase Assay Kit (Jiancheng). For ATP content measurement, it was measured following ATP Aassay Kit (Jiancheng).
The data were presented as the mean ± standard error (SE). After respectively testing for normality (Shapiro–Wilk test) and homogeneity variance (Levene’s tests), these data were further evaluated by a two-way analysis of variance (ANOVA) following by Dunnett’s post hoc test to determine the differences across various treatments. The difference was considered as significant or extremely significant when the p-value below 0.05 or 0.01, respectively.
The qPCR results have shown that the mRNA levels of TRE1-1, TRE1-2, TRE2, HK, PPGM1, PPGM2, UGPase, and TPS2 were significantly decreased at 48 h and 72 h after inhibition of GFAT or PFK (Figure 1). The expression levels of TPS1 were significantly decreased following dsPFK injection at 48 h and 72 h (Figure 1), while its mRNA level was downregulated at 48 h but return to the same level as the control group at 72 h after dsGFAT injection. Both gluconeogenesis and glycogenolysis result in the formation of G-6-P, which is hydrolyzed to glucose by G-6-pase (van and Gerin, 2002). G-6-pase was significantly downregulated after GFAT or PFK was knocked down (Figure 1). However, when GFAT or PFK was inhibited, the relative expressions of GS and GP were significantly decreased (Figure 1). These results suggest that both GS and GP genes, as well as other genes in the carbohydrate conversion pathway, were downregulated after GFAT or PFK was knocked down, respectively.
FIGURE 1. Relative expression of genes in carbohydrate conversion pathway at 48 h and 72 h after dsRNA injection. The qRT-PCR is used to detect the genes expression levels and the 18 s RNA is used as internal control. Bars are means ± SE (standard error) of three biological replicates. An asterisk (*) represents significant differences (p < 0.05); two asterisk (**) represents extremely significant differences (p < 0.01). TRE, trehalase; TPS, trehalose-6-phosphate synthase; PPGM, phosphoglucomutase; UGPase, UDP-Glucose pyrophosphorylase; GS, glycogen synthase; GP, glycogen phosphorylase; HK, hexokinase; G-6-pase, glucose-6-phosphatase.
The content of trehalose remained consistent after 48 h and subsequently decreased significantly at 72 h following the injection of dsGFAT (Figure 2A). Conversely, the glycogen content increased significantly at 48 h before returning to normal levels at 72 h following the injection of dsGFAT (Figure 2C). In contrast, there was no significant change in glucose content following the injection of dsGFAT (Figure 2B). Inhibition of the PFK gene resulted in the maintenance of trehalose content (Figure 2A), while both glucose and glycogen content showed no significant change at 48 h but increased significantly at 72 h (Figure 2C).
FIGURE 2. Content of trehalose, glucose and glycogen at 48 h and 72 h after dsRNA injection. Nilaparvata lugens on 1st of 5th instar were used to inject. Bars are means ± SE (standard error) of three biological replicates. An asterisk (*) represents significant differences (p < 0.05); two asterisk (**) represents extremely significant differences (p < 0.01). ns: not significant.
After GFAT was knocked down, the relative expression of G6PI1, G6PI2, PFK, PK1, PK2, PK3, PK4, PK8, PK9, and PK10 were downregulated both at 48 h and 72 h (Figure 3). The relative expression of G6PI3 was sharply upregulated (Figure 3), while PK5 and PK7 expression had no significance change at 48 h but decreased significantly at 72 h (Figure 3), and the mRNA level of PK6 extremely reduced at 48 h but increased significantly at 72 h (Figure 3). After PFK was knocked down, the relative expression of G6PI2, G6PI3, PFK, PK2, PK4, PK7, PK8, and PK9 decreased significantly (Figure 3). The relative expression of G6PI1 and PK1 significantly decreased at 48 h but significantly increased at 72 h (Figure 3), while PK3 and PK6 mRNA levels increased significantly at 48 h but decreased sharply (Figure 3). PK5 mRNA level was significantly reduced at 48 h but had had little change at 72 h (Figure 3), and PK10 mRNA level significantly increased at both 48 h and 72 h (Figure 3).
FIGURE 3. Relative expression of crucial genes in glycolytic pathway at 48 h and 72 h after dsRNA injection. The qRT-PCR is used to detect the genes expression levels and the 18 s RNA is used as internal control. Bars are means ± SE (standard error) of three biological replicates. An asterisk (*) represents significant differences (p < 0.05); two asterisk (**) represents extremely significant differences (p < 0.01). G6PI, glucose-6-phosphate isomerase; PFK, phosphofructokinase; PK, pyruvate kinase.
Following the GFAT gene inhibition, the relative expression of AH1 and AH2 significantly increased at 48 h and 72 h (Figure 4), whereas the relative expressions of MDH2 and MDH5 were significantly decreased at these time points (Figure 4). Additionally, the relative expression of MDH1 and MDH3 had no significant change at 48 h but significantly decreased at 72 h (Figure 4), and the relative expression of MDH4 dropped significantly at 48 h but increased at 72 h (Figure 4). After PFK gene inhibition, AH1 expression was upregulated significantly at 48 h and 72 h (Figure 4), while the expression levels of AH2 and MDH2 only showed a slight decrease at 48 h but decreased significantly at 72 h (Figure 4). Moreover, the relative expression level of MDH1 increased significantly at 48 h but decreased at 72 h (Figure 4), while the relative expression level of MDH3 and MDH5 decreased significantly at 48 h and 72 h (Figure 4). Lastly, the relative expression level of MDH4 expression level was significantly downregulated at 48 h but upregulated at 72 h (Figure 4).
FIGURE 4. Relative expression of crucial genes in tricarboxylic acid cycle at 48 h and 72 h after dsRNA injection. The qRT-PCR is used to detect the genes expression levels and the 18 s RNA is used as internal control. Bars are means ± SE (standard error) of three biological replicates. An asterisk (*) represents significant differences (p < 0.05); two asterisk (**) represents extremely significant differences (p < 0.01). AH, aconitate hydratase; MDH, malate dehydrogenase.
Inhibition of GFAT significantly reduced PK enzyme activity at 48 h and 72 h, and MDH activity significantly decreased at 48 h (Figure 5). Similarly, PFK inhibition resulted in a significant reduction in PK enzyme activity at 48 h and 72 h, with MDH activity showing no significant change at 48 h but significantly decreasing at 72 h (Figure 5).
FIGURE 5. Pyruvate kinase activity and malate dehydrogenase activity at 48 h and 72 h after dsRNA injection. Nilaparvata lugens on 1st of 5th instar were used to inject. Bars are means ± SE (standard error) of three biological replicates. An asterisk (*) represents significant differences (p < 0.05); two asterisk (**) represents extremely significant differences (p < 0.01). ns: not significant.
Following dsGFAT injection, ATP content significantly increased at 48 h but decreased at 72 h after (Figure 6). However, after PFK inhibition, ATP content significantly extremely at 48 h but returned to normal levels at 72 h (Figure 6).
FIGURE 6. Content of adenosine triphosphate (ATP) at 48 h and 72 h after dsRNA injection. Nilaparvata lugens on 1st of 5th instar were used to inject. Bars are means ± SE (standard error) of three biological replicates. An asterisk (*) represents significant differences (p < 0.05); two asterisk (**) represents extremely significant differences (p < 0.01). ns: not significant.
GFAT is the rate-limiting enzyme for glucose flux through the hexosamine pathway. However, there have been few studies investigating the GFAT gene in insects, with only a limited numbers of species, including Drosophila melanogaster, Aedes aegypti, Haemaphysalis longicornis, and Hyphantria cunea (Graack et al., 2001; Kato et al., 2002; Huang et al., 2007; Zou et al., 2022). In contrast, PFK is the important rate-limiting enzyme in the glycolytic pathway (Jojima and Inui, 2015). AMP-activated protein kinase (AMPK) inhibits GFAT activity, thereby regulating the hexosamine pathway according to the organism’s energy requirements (Chang et al., 2000; Eguchi et al., 2009; Zibrova et al., 2017; Ruegenberg et al., 2021). In the even of starvation, cAMP-dependent protein kinase A can redirect glucose metabolism into energy production, rather than synthetic pathways that require hexosamines (Chang et al., 2000). AMPK also activates PFK through phosphorylation to stimulate glycolysis (Marsin et al., 2000), and the two enzymes play crucial roles in energy metabolism. Previous study has showed that GFAT and PFK genes exhibit contrasting regulation of chitin metabolism in BPH (Xu et al., 2021). However, their different effects on energy metabolism remain unknown.
UDP-N-acetylglucosamine (UDP-GlcNAc) is the final product in HBP and a substrate for O-linked glycosylation of cellular proteins (Qian et al., 2011). High glucose levels in cancer patients increase the flux into HBP and improve GFAT enzyme levels (Vasconcelos-Dos-Santos et al., 2017), resulting in increased GFAT activity and inhibited glucose uptake and glycogen synthesis in insulin-resistant patients (Srinivasan et al., 2007). However, knockdown of NlGFAT in our study caused little change in glucose content, but glycogen concentration significantly increased 48 h after dsGFAT injection leading to a metabolic shift where glucose is stored as trehalose (Seo et al., 2018). This suggests an inhibition of glycogen synthesis within a short period, possibly due to decreased glycogen degradation, as the expression levels of NlGP were also extremely significantly decreased within 72 h after dsGFAT injection. In addition, the expression of NlTRE1-1, NlTRE1-2, NlTRE2, NlTPS1, and NlTPS2 also decreased extremely significantly simultaneously. Furthermore, the expression levels of NlPPGM1, NlPPGM2, NlUGPase, and NlHK significantly decreased at 48 and 72 h, indicating that BPHs can regulate sugar distribution by down-regulating the transcription levels of sugar metabolic enzyme genes after NlGFAT inhibition. NlGFAT expression was downregulated after inhibiting three NlTRE genes, while its expression was upregulated significantly at 72 h after NlTPS1 and NlTPS2 knockdown (Zhao et al., 2016; Yang et al., 2017). Despite the significant rise in glycogen content, the extremely significant decrease in NlGS expression at 48 and 72 h suggests that GS synthesis was inhibited, leading to the conversion of glycogen to trehalose (Seo et al., 2018). Additionally, NlG-6-pase expression significantly decreased at 48 and 72 h after NlGFAT knockdown, suggesting that glycogenolysis was also inhibited.
The expression of NlTRE1-1, NlTRE1-2, NlTRE2, NlTPS1, NlTPS2, NlPPGM1, NlPPGM2, NlUGPase, NlGS, NlGP, and NlHK were significantly downregulated after dsPFK injection. However, the changes in trehalose, glucose, and glycogen content were different from those observed with dsGFAT injection, suggesting that PFK may regulate the distribution of glucose (Gibb et al., 2017). Knocking down NlPFK resulted in little change to the trehalose content, but increased the glucose content and glycogen content after 72 h. Studies on cardiac myocytes suggest that when PFK activity was high, glucose uptake was increased, while glucose utilization is elevated, and when PFK activity was low, glucose uptake is minimally affected but glucose utilization is significantly reduced (Gibb et al., 2017). This could explain the significant increase in glucose content observed in our study, with some of the glucose being converted into glycogen for storage. The inhibition of key glycolytic enzymes favors gluconeogenesis (Belfiore et al., 1989).
Low PFK activity leads to a decrease in glycolysis metabolism (Gibb et al., 2017). The downregulation of NlPFK resulted in decreased expression levels of important enzyme in the glycolytic pathway and a sharp decrease in pyruvate kinase (PK) activity, which is in line with previous studies (Gibb et al., 2017). PK converts phosphoenolpyruvate and ADP to pyruvate and ATP in glycolysis, and pyruvate can be converted into acetyl-CoA, which combines with oxaloacetic acid to enter the TCA cycle (Israelsen and Vander Heiden, 2015). The TCA cycle, in combination with the subsequent electron transport chain, is one of the main metabolic pathways for providing energy under aerobic conditions (Gaster et al., 2012). The decrease of PK activity due to decreased NlPFK expression results in a decrease in pyruvate and subsequently a decrease in acetyl-CoA content. This decrease leads to the downregulation of enzyme genes in the TCA cycle, including NLAH2, NlMDH1, NlMDH2, NlMDH3, and NlMDH5, following dsPFK injection. Cytosolic MDH catalyzes the NADP-dependent oxidative decarboxylation of malate into pyruvate and carbon dioxide, generating NADPH. As an enzyme in production of NADPH, MDH is considered critical in TCA cycle (Farkas et al., 2002). Our results indicate that MDH activity also significantly decreased at 72 h after dsPFK injection. The decrease in enzyme genes expression in glycolysis-TCA cycle eventually led to an extreme decrease in ATP content 48 h after NlPFK knockdown.
According to Zhang et al. (2018), upregulated GFPT2 in fibroblasts leads to less change in genes responsible for glycolysis, the pentose phosphate pathway, and TCA cycle. However, our results differ markedly from theirs. After inhibiting NlGFAF, the relative expression levels of NlG6PI1, NlG6I2, NlPK1, NlPK2, NlPK3, NlPK4, NlPK5, NlPK7, NlPK8, NlPK9, and NlPK10 were significantly downregulated, and NlPFK expression also decreased significantly. Moreover, the PK activity also correspondingly decreased at 48 h and 72 h after dsGFAT injection. This suggested that the level of glycolytic metabolism also dropped sharply. However, the NlAH1 and NlAH2 expression increased extremely significantly after NlGFAT silencing, which is significantly different from dsPFK injection. Though the expression of NlMDH1, NlMDH2, NlMDH3, and NlMDH4 also decreased significantly, the MDH activity increased extremely significantly at 48 h after dsGFAT injection, and the ATP content also increased extremely significantly at 48 h after NlGFAT knockdown, but decreased extremely significantly at 72 h. Thus, after NlGFAT was inhibited by RNAi, the level of glycolytic metabolism dropped sharply whereas the level of TCA cycle increased dramatically. In the study of mouse C2C12 muscle cells, despite profound suppression of both glucose and pyruvate oxidation, TCA cycle metabolism still were maintained, and TCA flux was achieved through enhanced reliance on glutaminolysis through malic enzyme and pyruvate dehydrogenase (PDH) as well as fatty acid and branched chain amino acid oxidation (Vacanti et al., 2014). This may be the reason that in our study, the level of glycolytic metabolism also dropped sharply whereas the level of TCA cycle increased dramatically. Unfortunately, we were unable to detect changes in fat and amino acid levels, but this still provides ideas.
In conclusion, the inhibition of NlGFAT or NlPFK resulted in a disorder of energy metabolism in BPHs. The knockdown of NlGFAT or NlPFK resulted in changes in distribution of trehalose, glucose, and glycogen and decrease of glycolysis. However, the downregulation of NlGFAT led to an increase in TCA cycle level and ATP content, whereas the downregulation of NlPFK caused a decrease of TCA cycle level and ATP content. Since NlGFAT and NlPFK influence the energy metabolism of BPHs, the development of corresponding enzyme inhibitors or activators might be explored for biological control of BPHs.
The original contributions presented in the study are included in the article/supplementary material, further inquiries can be directed to the corresponding authors.
C-DX and P-JW conceived and designed the research work, and participate in the data analysis. H-RS and S-SS performed the research works, analyzed the data. Y-KL and L-YQ participate the manuscript writing. FL and QF helped in rice transplantation and insect rearing. All authors contributed to the article and approved the submitted version.
This work was supported by the “Pioneer” and “Leading Goose” R&D Program of Zhejiang (2022C02047), the National Natural Science Foundation of China (Grant No. 32272608), the Rice Pest Management Research Group of the Agricultural Science and Technology Innovation Program of China Academy of Agricultural Science (CAAS-ASTIP-2016-CNRRI), the Guizhou Provincial Science and Technology Foundation [ZK (2021) 210], and Hangzhou Normal University’s Starlight Plan in 2023 and Hangzhou Normal University Undergraduate Innovation Ability Improvement Project.
The authors declare that the research was conducted in the absence of any commercial or financial relationships that could be construed as a potential conflict of interest.
All claims expressed in this article are solely those of the authors and do not necessarily represent those of their affiliated organizations, or those of the publisher, the editors and the reviewers. Any product that may be evaluated in this article, or claim that may be made by its manufacturer, is not guaranteed or endorsed by the publisher.
Achari A., Marshall S. E., Muirhead H., Palmieri R. H., Noltmann E. A. (1981). Glucose-6-phosphate isomerase. Phil Trans. R. Soc. Lond B 293, 145–157. doi:10.1098/rstb.1981.0068
Barraza A., Sánchez F. (2013). Trehalases: A neglected carbon metabolism regulator? Plant Signal Behav. 8, e24778. doi:10.4161/psb.24778
Belfiore F., Rabuazzo A. M., Iannello S., Campione R., Castorina S., Urzi F. (1989). Extra-pancreatic action of glibenclamide in man: Reduction in vitro of the inhibitory effect of glucagon and epinephrine on the hepatic key glycolytic enzymes phosphofructokinase (PFK) and pyruvate kinase (PK). Eur. J. Clin. Investig. 19 (4), 367–371. doi:10.1111/j.1365-2362.1989.tb00243.x
Bing X. L., Zhao D. S., Hong X. Y. (2019). Bacterial reproductive manipulators in rice planthoppers. Arch. Insect Biochem. Physiol. 101 (2), e21548. doi:10.1002/arch.21548
Bonora M., Patergnani S., Rimessi A., De Marchi E., Suski J. M., Bononi A., et al. (2012). ATP synthesis and storage. Purinergic Signal 8 (3), 343–357. doi:10.1007/s11302-012-9305-8
Chang Q., Su K., Baker J. R., Yang X., Paterson A. J., Kudlow J. E. (2000). Phosphorylation of human glutamine: fructose-6-phosphate amidotransferase by cAMP-dependent protein kinase at serine 205 blocks the enzyme activity. J. Biol. Chem. 275 (29), 21981–21987. doi:10.1074/jbc.M001049200
Chen J. X., Lyu Z. H., Wang C. Y., Cheng J., Lin T. (2018). RNA interference of a trehalose-6-phosphate synthase gene reveals its roles in the biosynthesis of chitin and lipids in Heortia vitessoides (Lepidoptera: Crambidae). Insect Sci. 27, 212–223. doi:10.1111/1744-7917.12650
Dasika S. K., Vinnakota K. C., Beard D. A. (2015). Determination of the catalytic mechanism for mitochondrial malate dehydrogenase. Biophys. J. 108 (2), 408–419. doi:10.1016/j.bpj.2014.11.3467
Denzel M. S., Antebi A. (2015). Hexosamine pathway and (ER) protein quality control. Curr. Opin. Cell. Biol. 33, 14–18. doi:10.1016/j.ceb.2014.10.001
Eguchi S., Oshiro N., Miyamoto T., Yoshino K., Okamoto S., Ono T., et al. (2009). AMP-Activated protein kinase phosphorylates glutamine: fructose-6-phosphate amidotransferase 1 at Ser243 to modulate its enzymatic activity. Genes. cells. 14 (2), 179–189. doi:10.1111/j.1365-2443.2008.01260.x
Elbein A. D., Pan Y. T., Pastuszak I., Carroll D. (2003). New insights on trehalose: A multifunctional molecule. Glycobiology 13 (4), 17R–27R. doi:10.1093/glycob/cwg047
Farkas R., Danis P., Medved'ová L., Mechler B. M., Knopp J. (2002). Regulation of cytosolic malate dehydrogenase by juvenile hormone in Drosophila melanogaster. Cell. Biochem. Biophys. 37 (1), 37–52. doi:10.1385/CBB:37:1:37
Gaster M., Nehlin J. O., Minet A. D. (2012). Impaired TCA cycle flux in mitochondria in skeletal muscle from type 2 diabetic subjects: Marker or maker of the diabetic phenotype? Arch. Physiol. Biochem. 118 (3), 156–189. doi:10.3109/13813455.2012.656653
Gibb A. A., Lorkiewicz P. K., Zheng Y. T., Zhang X., Bhatnagar A., Jones S. P., et al. (2017). Integration of flux measurements to resolve changes in anabolic and catabolic metabolism in cardiac myocytes. Biochem. J. 474 (16), 2785–2801. doi:10.1042/BCJ20170474
Graack H. R., Cinque U., Kress H. (2001). Functional regulation of glutamine: fructose-6-phosphate aminotransferase 1 (GFAT1) of Drosophila melanogaster in a UDP-N-acetylglucosamine and cAMP-dependent manner. Biochem. J. 360 (Pt 2), 401–412. doi:10.1042/0264-6021:3600401
Gu J., Shao Y., Zhang C., Liu Z., Zhang Y. (2009). Characterization of putative soluble and membrane-bound trehalases in a hemipteran insect, Nilaparvata lugens. J. Insect Physiol. 55 (11), 997–1002. doi:10.1016/j.jinsphys.2009.07.003
Hassan A., Mehmood N., Xu H., Usman H. M., Wu J., Liu L., et al. (2022). Phosphofructokinase downregulation disturbed termite social behaviors and immunity against fungal infections. Entomol. Gen. 42 (5), 799–808. doi:10.1127/entomologia/2022/1444
Hornbuckle L. A., Edgerton D. S., Ayala J. E., Svitek C. A., Oeser J. K., Neal D. W., et al. (2001). Selective tonic inhibition of G-6-pase catalytic subunit, but not G-6-P transporter, gene expression by insulin in vivo. Am. J. Physiol. Endocrinol. Metab. 281 (4), E713–E725. doi:10.1152/ajpendo.2001.281.4.E713
Hu D., Luo W., Fan L. F., Liu F. L., Gu J., Deng H. M., et al. (2016). Dynamics and regulation of glycolysis-tricarboxylic acid metabolism in the midgut of Spodoptera litura during metamorphosis. Insect Mol. Biol. 25 (2), 153–162. doi:10.1111/imb.12208
Huang X., Tsuji N., Miyoshi T., Motobu M., Islam M. K., Alim M. A., et al. (2007). Characterization of glutamine: fructose-6-phosphate aminotransferase from the ixodid tick, Haemaphysalis longicornis, and its critical role in host blood feeding. Int. J. Parasitol. 37 (3-4), 383–392. doi:10.1016/j.ijpara.2006.11.012
Israelsen W. J., Vander Heiden M. G. (2015). Pyruvate kinase: Function, regulation and role in cancer. Semin. Cell. Dev. Biol. 43, 43–51. doi:10.1016/j.semcdb.2015.08.004
Jojima T., Inui M. (2015). Engineering the glycolytic pathway: A potential approach for improvement of biocatalyst performance. Bioengineered 6 (6), 328–334. doi:10.1080/21655979.2015.1111493
Kato N., Dasgupta R., Smartt C. T., Christensen B. M. (2002). Glucosamine: fructose-6-phosphate aminotransferase: Gene characterization, chitin biosynthesis and peritrophic matrix formation in Aedes aegypti. Insect Mol. Biol. 11 (3), 207–216. doi:10.1046/j.1365-2583.2002.00326.x
Li Y., Gao H., Zhang Y. W., Lin X. D. (2021). Role of the transcription factor Taiman in moulting and ovarian development of Nilaparvata lugens. Nil. lugens Entomol. Gen. 41 (2), 169–177. doi:10.1127/entomologia/2021/0976
Liu H., Su X. Y., Sun Z., Wang C., Shi J. H., Foba C. N., et al. (2022). Nitrogen and plant pathogens alter rice plant volatiles mediating host location behavior of Nilaparvata lugens and its parasitoid Anagrus nilaparvatae. Entomol. Gen. 42 (4), 549–557. doi:10.1127/entomologia/2022/1281
Liu X., Cooper A. M. W., Zhang J., Zhu K. Y. (2019). Biosynthesis, modifications and degradation of chitin in the formation and turnover of peritrophic matrix in insects. J. Insect Physiol. 114, 109–115. doi:10.1016/j.jinsphys.2019.03.006
Liu Z., Gong P., Heckel D. G., Wei W., Sun J., Li D. (2009). Effects of larval host plants on over-wintering physiological dynamics and survival of the cotton bollworm, Helicoverpa armigera (Hübner) (Lepidoptera: Noctuidae). J. Insect Physiol. 55, 1–9. doi:10.1016/j.jinsphys.2008.07.017
Livak K. J., Schmittgen T. D. (2001). Analysis of relative gene expression data using real-time quantitative PCR and the 2−ΔΔCT method. Methods 25, 402–408. doi:10.1006/meth.2001.1262
Luo Y. J., Chen Y., Wang X. J., Wang S. T., Yang Y. Y., Xu H. X., et al. (2022). Validamycin affects the development and chitin metabolism in Spodoptera frugiperda by inhibiting trehalase activity. Entomol. Gen. 42 (6), 931–939. doi:10.1127/entomologia/2022/1608
Marsin A. S., Bertrand L., Rider M. H., Deprez J., Beauloye C., Vincent M. F., et al. (2000). Phosphorylation and activation of heart PFK-2 by AMPK has a role in the stimulation of glycolysis during ischaemia. Curr. Biol. 10 (20), 1247–1255. doi:10.1016/s0960-9822(00)00742-9
Martínez-Costa O. H., Hermida C., Sánchez-Martínez C., Santamaría B., Aragón J. J. (2004). Identification of C-terminal motifs responsible for transmission of inhibition by ATP of mammalian phosphofructokinase, and their contribution to other allosteric effects. Biochem. J. 377 (Pt 1), 77–84. doi:10.1042/BJ20031032
Merzendorfer H., Zimoch L. (2003). Chitin metabolism in insects: Structure, function and regulation of chitin synthases and chitinases. J. Exp. Biol. 206 (Pt 24), 4393–4412. doi:10.1242/jeb.00709
Mu X. C., Zhang W., Wang L. X., Zhang S., Zhang K., Gao C. F., et al. (2016). Resistance monitoring and cross-resistance patterns of three rice planthoppers, Nilaparvata lugens, Sogatella furcifera and Laodelphax striatellus to dinotefuran in China. Pestic. Biochem. Physiol. 134, 8–13. doi:10.1016/j.pestbp.2016.05.004
Newsholme B. E. A., Sugden P. H., Williams T. (1977). Effect of citrate on the activities of 6-phosphofructokinase from nervous and muscle tissues from different animals and its relationships to the regulation of glycolysis. Biochem. J. 166, 123–129. doi:10.1042/bj1660123
Nunes R. D., Romeiro N. C., De Carvalho H. T., Moreira J. R., Sola-Penna M., Silva-Neto M. A., et al. (2016). Unique PFK regulatory property from some mosquito vectors of disease, and from Drosophila melanogaster. Parasit. Vectors 9, 107. doi:10.1186/s13071-016-1391-y
Prats C., Cadefau J. A., Cusso R., Qvortrup K., Nielsen J. N., Wojtaszewki J. F. P., et al. (2005). Phosphorylation-dependent translocation of glycogen synthase to a novel structure during glycogen resynthesis. J. Biol. Chem. 280, 23165–23172. doi:10.1074/jbc.M502713200
Qian Y., Ahmad M., Chen S., Gillespie P., Le N., Mennona F., et al. (2011). Discovery of 1-arylcarbonyl-6,7-dimethoxyisoquinoline derivatives as glutamine fructose-6-phosphate amidotransferase (GFAT) inhibitors. Bioorg. Med. Chem. Lett. 21 (21), 6264–6269.
Ren X. Y., Zhang L. S., Qi X. Y., An T., Han Y. H., Chen H. Y. (2015). Metabolic adaption and evaluation of cold hardiness on diapausing ladybird, Coccinella septempunctata L. J. Environ. Entomol. 37, 1195–1202. doi:10.3969/j.issn.1674-0858.2015.06.11
Ruegenberg S., Mayr F. A. M. C., Atanassov I., Baumann U., Denzel M. S. (2021). Protein kinase A controls the hexosamine pathway by tuning the feedback inhibition of GFAT-1. Nat. Commun. 12 (1), 2176. doi:10.1038/s41467-021-22320-y
Seo Y., Kingsley S., Walker G., Mondoux M. A., Tissenbaum H. A. (2018). Metabolic shift from glycogen to trehalose promotes lifespan and healthspan in Caenorhabditis elegans. Proc. Natl. Acad. Sci. U. S. A. 115 (12), E2791-E2800–E2800. doi:10.1073/pnas.1714178115
Shukla E., Thorat L. J., Nath B. B., Gaikwad S. M. (2015). Insect trehalase: Physiological significance and potential applications. Glycobiology 25 (4), 357–367. doi:10.1093/glycob/cwu125
Srinivasan V., Sandhya N., Sampathkumar R., Farooq S., Mohan V., Balasubramanyam M. (2007). Glutamine fructose-6-phosphate amidotransferase (GFAT) gene expression and activity in patients with type 2 diabetes: Inter-relationships with hyperglycaemia and oxidative stress. Clin. Biochem. 40 (13-14), 952–957. doi:10.1016/j.clinbiochem.2007.05.002
Sun H., Yang B., Zhang Y., Liu Z. (2017). Metabolic resistance in Nilaparvata lugens to etofenprox, a non-ester pyrethroid insecticide. Pestic. Biochem. Physiol. 136, 23–28. doi:10.1016/j.pestbp.2016.08.009
Tamang A. M., Kalra B., Parkash R. (2017). Cold and desiccation stress induced changes in the accumulation and utilization of proline and trehalose in seasonal populations of Drosophila immigrans. Comp. Biochem. Physiol. a Mol. Integr. Physiol. 203, 304–313. doi:10.1016/j.cbpa.2016.10.011
Tang B., Wei P., Chen J., Wang S. G., Zhang W. Q. (2012). Progress in gene features and functions of insect trehalases. Acta Entomol. Sin. 55, 1315–1321. doi:10.16380/j.kcxb.2012.11.008
Tang B., Yang M., Shen Q1, Xu Y., Wang H., Wang S. (2017). Suppressing the activity of trehalase with validamycin disrupts the trehalose and chitin biosynthesis pathways in the rice Brown planthopper, Nilaparvata lugens. Pestic. Biochem. Physiol. 137, 81–90. doi:10.1016/j.pestbp.2016.10.003
Tatun N., Singtripop T., Sakurai S. (2008a). Dual control of midgut trehalase activity by 20-hydroxyecdysone and an inhibitory factor in the bamboo borer Omhisa fuscidentalis Hampson. J. Insect Physiol. 54, 351–357. doi:10.1016/j.jinsphys.2007.10.006
Tatun N., Singtripop T., Tungjitwitayakul J., Sakurai S. (2008b). Regulation of soluble and membrane-bound trehalase activity and expression of the enzyme in the larval midgut of the bamboo borer Omphisa fuscidentalis. Omphisa fuscidentalis Insect Biochem. Mol. Biol. 38, 788–795. doi:10.1016/j.ibmb.2008.05.003
Terol J., Soler G., Talon M., Cercos M. (2010). The aconitate hydratase family from Citrus. BMC Plant Biol. 10, 222. doi:10.1186/1471-2229-10-222
Tharanathan R. N., Kittur F. S. (2003). Chitin-the undisputed biomolecule of great potential. Crit. Rev. Food Sci. Nutr. 43, 61–87. doi:10.1080/10408690390826455
Tian L., Guo E., Wang S., Liu S., Jiang R. J., Cao Y., et al. (2010). Developmental regulation of glycolysis by 20-hydroxyecdysone and juvenile hormone in fat body tissues of the silkworm, Bombyx mori. J. Mol. Cell. Biol. 2, 255–263. doi:10.1093/jmcb/mjq020
Tolmasky D. S., RabossiQuesada-Allue L. A. (2001). Synthesis and mobilization of glycogen during metamorphosis of the medfly Ceratitis capitata. Arch. Biochem. Biophys. 392, 38–47. doi:10.1006/abbi.2001.2394
Ullah F., Gul H., Tariq K., Hafeez M., Desneux N., Gao X. W., et al. (2022). RNA interference-mediated silencing of ecdysone receptor (EcR) gene causes lethal and sublethal effects on melon aphid, Aphis gossypii. Entomol. Gen. 42 (5), 791–797. doi:10.1127/entomologia/2022/1434
Vacanti N. M., Divakaruni A. S., Green C. R., Parker S. J., Henry R. R., Ciaraldi T. P., et al. (2014). Regulation of substrate utilization by the mitochondrial pyruvate carrier. Mol. Cell. 56 (3), 425–435. doi:10.1016/j.molcel.2014.09.024
van Schaftingen E., Gerin I. (2002). The glucose-6-phosphatase system. Biochem. J. 362 (Pt 3), 513–532. doi:10.1042/0264-6021:3620513
Vasconcelos-Dos-Santos A., Loponte H. F., Mantuano N. R., Oliveira I. A., de Paula I. F., Teixeira L. K., et al. (2017). Hyperglycemia exacerbates colon cancer malignancy through hexosamine biosynthetic pathway. Oncogenesis 6 (3), e306. doi:10.1038/oncsis.2017.2
Wang S. S., Chen X., Li Y., Pan B. Y., Wang S. G., Dai H. J., et al. (2020). Effects of changing temperature on the physiological and biochemical properties of Harmonia axyridis larvae. Entomol. Gen. 40 (3), 229–241. doi:10.1127/entomologia/2020/0917
Wen X., Wang S., Duman J. G., Arifin J. F., Juwita V., Goddard W. A., et al. (2016). Antifreeze proteins govern the precipitation of trehalose in a freezing-avoiding insect at low temperature. Proc. Natl. Acad. Sci. U. S. A. 113 (24), 6683–6688. doi:10.1073/pnas.1601519113
White K. P., Rifkin S. A., Hurban P., Hogness D. S. (1999). Microarray analysis of Drosophila development during metamorphosis. Science 286, 2179–2184. doi:10.1126/science.286.5447.2179
Wipking W., Viebahn M., Neumann D. (1995). Oxygen consumption, water, lipid and glycogen content of early and late diapause and non-diapause larvae of the burnet moth Zygaena trifolii. J. Insect Physiol. 41, 47–56. doi:10.1016/0022-1910(94)00079-v
Xi Y., Pan P. L., Ye Y. X., Yu B., Xu H. J., Zhang C. X. (2015). Chitinase-like gene family in the Brown planthopper, Nilaparvata lugens. Insect Mol. Biol. 24 (1), 29–40. doi:10.1111/imb.12133
Xu C. D., Liu Y. K., Qiu L. Y., Wang S. S., Pan B. Y., Li Y., et al. (2021). GFAT and PFK genes show contrasting regulation of chitin metabolism in Nilaparvata lugens. Sci. Rep. 11 (1), 5246. doi:10.1038/s41598-021-84760-2
Yang M., Zhao L., Shen Q., Xie G., Wang S., Tang B. (2017). Knockdown of two trehalose-6-phosphate synthases severely affects chitin metabolism gene expression in the Brown planthopper Nilaparvata lugens. Pest Manag. Sci. 73 (1), 206–216. doi:10.1002/ps.4287
Yasugi T., Yamada T., Nishimura T. (2017). Adaptation to dietary conditions by trehalose metabolism in Drosophila. Sci. Rep. 7 (1), 1619. doi:10.1038/s41598-017-01754-9
Yu C. H., Lu Lin R. H., Wang X. J., Jiang H., Zhao F. (2008). Trehalose-Insect blood sugar. Chin. Bull. Entomol. 45, 832–837. doi:10.3969/j.issn.0452-8255.2008.05.035
Zhang L., Qiu L. Y., Yang H. L., Wang H. J., Zhou M., Wang S. G., et al. (2017). Study on the effect of wing bud chitin metabolism and its developmental network genes in the Brown planthopper, Nilaparvata lugens, by knockdown of TRE gene. Front. Physiol. 8, 750. doi:10.3389/fphys.2017.00750
Zhang W., Bouchard G., Yu A., Shafiq M., Jamali M., Shrager J. B., et al. (2018). GFPT2-expressing cancer-associated fibroblasts mediate metabolic reprogramming in Human lung adenocarcinoma. Cancer Res. 78 (13), 3445–3457. doi:10.1158/0008-5472.CAN-17-2928
Zhao L. N., Yang M. M., Shen Q. D., Shi Z. K., Wang S. G., Tang B., et al. (2016). Functional characterization of three trehalase genes regulating the chitin metabolism pathway in rice Brown planthopper using RNA interference. Sci. Rep. 6, 27841–27854. doi:10.1038/srep27841
Zhou C., Yang X. B., Yang H., Gong M. F., Long G. Y., Jin D. C. (2022). Role of insecticide-mediated transcription of the TOR and JH signaling pathway-related genes in the regulation of reproduction in Sogatella furcifera. Entomol. Gen. 42 (5), 771–779. doi:10.1127/entomologia/2022/1496
Zhou J., Yan J., You K., Chen X., Yuan Z., Zhou Q., et al. (2018). Characterization of a Nilaparvata lugens (Stål) brummer gene and analysis of its role in lipid metabolism. Arch. Insect Biochem. Physiol. 97(3), e21442. doi:10.1002/arch.21442
Zhu K. Y., Merzendorfer H., Zhang W., Zhang J., Muthukrishnan S. (2016). Biosynthesis, turnover, and functions of chitin in insects. Annu. Rev. Entomol. 61, 177–196. doi:10.1146/annurev-ento-010715-023933
Zibrova D., Vandermoere F., Göransson O., Peggie M., Mariño K. V., Knierim A., et al. (2017). GFAT1 phosphorylation by AMPK promotes VEGF-induced angiogenesis. Biochem. J. 474 (6), 983–1001. doi:10.1042/BCJ20160980
Keywords: Nilaparvata lugens, RNA interference, glutamine: fructose-6-phosphate aminotransferase, phosphofructokinase, energy metabolism
Citation: Si H-R, Sun S-S, Liu Y-K, Qiu L-Y, Tang B, Liu F, Fu Q, Xu C-D and Wan P-J (2023) Roles of GFAT and PFK genes in energy metabolism of brown planthopper, Nilaparvata lugens. Front. Physiol. 14:1213654. doi: 10.3389/fphys.2023.1213654
Received: 28 April 2023; Accepted: 13 June 2023;
Published: 21 June 2023.
Edited by:
Ya-Nan Zhang, Huaibei Normal University, ChinaReviewed by:
Hu Kui, Yangzhou University, ChinaCopyright © 2023 Si, Sun, Liu, Qiu, Tang, Liu, Fu, Xu and Wan. This is an open-access article distributed under the terms of the Creative Commons Attribution License (CC BY). The use, distribution or reproduction in other forums is permitted, provided the original author(s) and the copyright owner(s) are credited and that the original publication in this journal is cited, in accordance with accepted academic practice. No use, distribution or reproduction is permitted which does not comply with these terms.
*Correspondence: Cai-Di Xu, eHVjYWlkaTAwMUAxNjMuY29t; Pin-Jun Wan, d2FucGluanVuQGNhYXMuY24=
†These authors have contributed equally to this work
Disclaimer: All claims expressed in this article are solely those of the authors and do not necessarily represent those of their affiliated organizations, or those of the publisher, the editors and the reviewers. Any product that may be evaluated in this article or claim that may be made by its manufacturer is not guaranteed or endorsed by the publisher.
Research integrity at Frontiers
Learn more about the work of our research integrity team to safeguard the quality of each article we publish.