- 1Department of Anaesthesiology, Pain and Respiratory Support, Neuroscience Centre, Copenhagen University Hospital–Rigshospitalet, Glostrup, Denmark
- 2Functional Imaging Unit, Department of Clinical Physiology and Nuclear Medicine, Copenhagen University Hospital—Rigshospitalet, Copenhagen, Denmark
- 3Anesthesiology and Intensive Care, Department of Clinical Sciences, Faculty of Medicine, Lund University, Lund, Sweden
- 4Department of Neuroanaesthesiology, Neuroscience Centre, Copenhagen University Hospital–Rigshospitalet, Copenhagen, Denmark
- 5Department of Clinical Medicine, Faculty of Health and Medical Sciences, University of Copenhagen, Copenhagen, Denmark
In humans, resting cerebral perfusion, oxygen consumption and energy metabolism demonstrate large intersubject variation regardless of methodology. Whether a similar large variation is also present longitudinally in individual subjects is much less studied, but knowing the time variance in reproducibility is important when designing and interpreting longitudinal follow-up studies examining brain physiology. Therefore, we examined the reproducibility of cerebral blood flow (CBF), global cerebral metabolic rate of oxygen (CMRO2), global arteriovenous oxygen saturation difference (A-V.O2), and cerebral lactate and N-acetyl-aspartate (NAA) concentrations measured using magnetic resonance imaging (MRI) and spectroscopy (MRS) techniques through repeated measurements at 6 h, 24 h, 7 days and several weeks after initial baseline measurements in young healthy adults (N = 26, 13 females, age range 18–35 years). Using this setup, we calculated the correlation, limit of agreement (LoA) and within-subject coefficient of variation (CoVWS) between baseline values and the subsequent repeated measurements to examine the longitudinal variation in individual cerebral physiology. CBF and CMRO2 correlated significantly between baseline and all subsequent measurements. The strength of the correlations (R2) and reproducibility metrics (LoA and CoVWS) demonstrated the best reproducibility for the within-day measurements and generally declined with longer time between measurements. Cerebral lactate and NAA concentrations also correlated significantly for all measurements, except between baseline and the 7-day measurement for lactate. Similar to CBF and CMRO2, lactate and NAA demonstrated the best reproducibility for within-day repeated measurements. The gradual decline in reproducibility over time should be considered when designing and interpreting studies on brain physiology, for example, in the evaluation of treatment efficacy.
1 Introduction
Measurements of cerebral blood flow (CBF), oxygen consumption and energy metabolism are of considerable interest when studying brain physiology and pathophysiology. Numerous research studies have examined the effects of disease or certain interventions on cerebral physiology (Powers et al., 1985; Liddle et al., 1992; Oshima et al., 2003; Peng et al., 2014; Snyder et al., 2015; Wolters et al., 2017; Younis et al., 2021; Vestergaard et al., 2022). However, cerebral physiology, both CBF and energy metabolism, demonstrates very large intersubject variability, which makes it difficult to observe small effects of intervention or disease. Multiple factors modulate CBF both acutely and over longer time periods (Kety and Schmidt, 1948; Lassen, 1959; Iida et al., 2004; Coles et al., 2006; Henriksen et al., 2013); for example, changes in arterial carbon dioxide or oxygen tension as well as oxygen saturation affect CBF (Paulson et al., 1990; Vestergaard et al., 2016; Vestergaard and Larsson, 2019). The cerebral metabolic rate of oxygen (CMRO2) has similarly a large intersubject variability but demonstrates smaller acute fluctuations than CBF (Iida et al., 2004; Coles et al., 2006; Peng et al., 2014; Vestergaard et al., 2020) A key role of the regulation of CBF is to maintain CMRO2 at constant levels.
Studies have examined intersubject variation in cerebral physiology, but normal longitudinal intrasubject variation is much less examined and has mainly focused only on CBF. However, longitudinal studies on cerebral physiology, for example, in terms of disease progression or intervention, require knowledge of normal longitudinal intrasubject variation for correct scaling of the project and interpretation of the results. Studies have examined the reproducibility of CBF using various techniques, but typically only two measurements were acquired, often with only a short time between scans (hours or a few days).
In the present study, we aimed to measure intrasubject variation in cerebral physiology by performing multiple repeated measurements in the same subjects with 6 h, 1 day, 7 days and several weeks between examinations. We used MRI techniques to acquire brain physiological parameters. Due to the noninvasiveness of MRI, it is possible to obtain multiple repeated measurements with only minor considerations related to patient safety. For example, exposure to radiation is not a concern, as would be the case if using positron emission tomography (PET) imaging. MRI techniques were therefore chosen as the most suitable examination method. Global average CBF and CMRO2 were acquired using phase contrast mapping (PCM) and susceptibility-based oximetry (SBO) MRI. CBF maps were obtained using the arterial spin labelling (ASL) MRI technique.
In addition to CBF and CMRO2, we also measured the concentrations of cerebral lactate and N-acetyl-aspartate (NAA) by magnetic resonance spectroscopy (MRS). In the healthy brain, most of the glucose for energy production is fully oxidized, resulting in an oxygen-to-glucose ratio close to 6. However, in the young healthy brain, approximately 10% of glucose goes through oxygen-free glycolysis with lactate as the end-product (Goyal et al., 2014; Hyder et al., 2016). By measuring the cerebral lactate concentration, we can examine the consistency of this glycolytic activity over time. NAA is predominantly synthesized in neurons and is primarily a marker of neuronal density. The NAA concentration is lower in patients with dementia and is reduced after stroke where it correlates with neuron loss. However, NAA is also affected by neuronal metabolic activity. For example, during disease activity in multiple sclerosis and after traumatic brain injury, the NAA concentration is reduced. Yet, this reduction is reversed during recovery. This suggests that NAA concentration is also affected by metabolic integrity, in addition to neuronal density. (Bitsch et al., 1999; Moffett et al., 2007). In healthy subjects, as examined in the current study, the NAA concentration is expected to be stable, however knowing the normal variation is important to correctly use NAA as a marker of neuronal function.
Overall, we examined intrasubject longitudinal variation in CBF, CMRO2, arteriovenous oxygen saturation difference (A-V.O2), cerebral lactate concentration, and cerebral NAA concentration in healthy humans through repeated MRI scan sessions. By measuring all these parameters, we obtained a comprehensive examination of longitudinal intrasubject variation in several markers of cerebral physiology.
2 Materials and methods
2.1 Subjects
Two groups of young healthy subjects participated in the study. All participants were healthy, right-handed, non-smokers, with a body mass index of 18–30 kg/m2 and normal physical and neurological examinations. Female participants were non-pregnant. The participants were instructed to have no intake or use of caffeine, alcohol, medication or other substances known to influence the parameters measured in the study prior to study participation. Detailed description regarding the inclusion criteria of the participants are provided in Madsen et al. (2020). In the first group (Group A), ten young healthy subjects (5 females) with a mean age of 25.4 years (range 18–35 years) were included and examined in four MRI sessions with repeated measurements acquired at 6 h, 1 day, and 7 days after an initial baseline measurement. All participants adhered to the same schedule, aligning the weekdays and timing of all scans and measurements. All participants were fasting 2 h for clear liquids and 6 h for all other intake before baseline scans. Between baseline and the second scan on the same day, the participants were served a light meal and drinks adhering to protocol (e.g., no caffeine, alcohol or medication). Next, we examined a second group (Group B), which included sixteen young healthy subjects (8 females) with a mean age of 23.9 years (range 18–32 years) who were examined twice with 28–49 days (mean 31.9 days) between examinations. Thus, we investigated short-term repeatability (up to 7 days) in Group A and repeatability after several weeks in Group B. Group B was included as a follow-up analysis due to the highly stable correlations observed in Group A, and we wanted to examine whether correlations were maintained after several weeks. All participants in Group B also adhered to a strict schedule, aligning the weekdays and timing of all scans and measurements, and following the same fasting regime for all scans used in this study. The participants from Group B were part of a study on the effect of general anaesthesia on brain structure and physiology; none of these subjects underwent anaesthesia during the present study (Madsen et al., 2020). Data from Group A was acquired in 2019, and data from Group B was acquired subsequently, in 2019–2021.
In all sessions, we measured global CBF and CMRO2 twice (run 1 and run 2) at the beginning and end of the session to determine within-session reproducibility of these measurements. The remaining parameters were measured once in each session. The study setup is depicted in Figure 1.
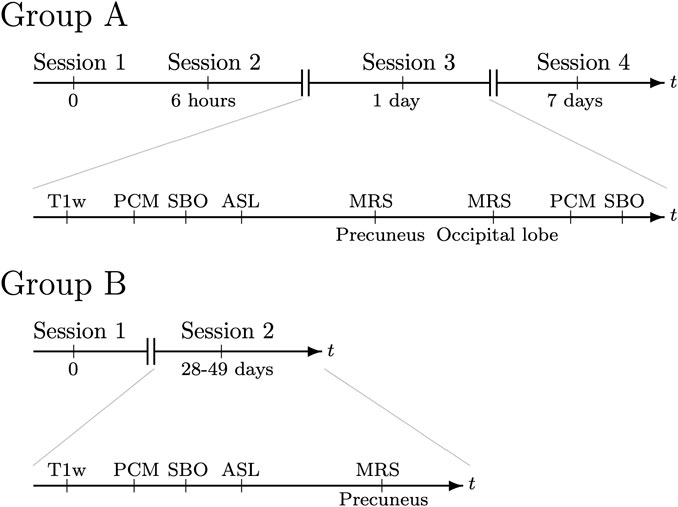
FIGURE 1. Outline of the MRI sessions and acquisition of cerebral physiology parameters allowing for examination of reproducibility. Group A underwent MRI scans four times, with 6 h, 1 day and 7 days between reacquisitions after an initial baseline measurement. Group B underwent MRI scans two times, with the second scan 28–49 days after the initial baseline scan. In each session, structural brain images were acquired using a T1-weighted high-resolution anatomical MRI sequence (T1w). Cerebral blood flow (CBF) was measured using phase contrast mapping (PCM) and arterial spin labelling (ASL) MRI techniques. Susceptibility-based oximetry (SBO) was used to measure the venous oxygen saturation of the blood leaving the brain, from which the cerebral metabolic rate of oxygen (CMRO2) was calculated. Cerebral NAA and lactate concentrations were measured using magnetic resonance spectroscopy (MRS).
Participants were recruited through advertisement on a Danish recruitment website (www.forsøgsperson.dk). Exclusion criteria were known neurological diseases and contraindications for MRI, such as metal implants and recent operations or pregnancy. The study was approved by the scientific ethical committee of the Capital Region of Denmark (H-18020364 and H-18028925) and was carried out according to the Declaration of Helsinki.
2.2 Magnetic resonance imaging
All scans were acquired on a 3 T Philips (Philips Medical Systems, Best, Netherlands) Achieva dStream (software release 5.4.1) equipped with a 32-channel phased-array receive head coil. The scanner was subjected to ongoing maintenance from the manufacture (Philips Healthcare); and we performed quality control and monitored the scanner and coil performance every week. There were no major software updates or hardware changes during data collection for this study.
The heart rate, arterial oxygen saturation and end tidal CO2 partial pressure (PetCO2) were measured continuously throughout the MRI scans using a Veris Monitor system (MEDRAD, Pittsburgh, Pennsylvania, USA). The blood pressure was measured with regular intervals during the examinations. The average values of these measurements throughout the scans are presented in Table 1. A venous blood sample was drawn before each scan session and analysed for haemoglobin (Hgb) concentration using a blood gas analyser XN 9000 (Sysmex, Kobe, Japan).
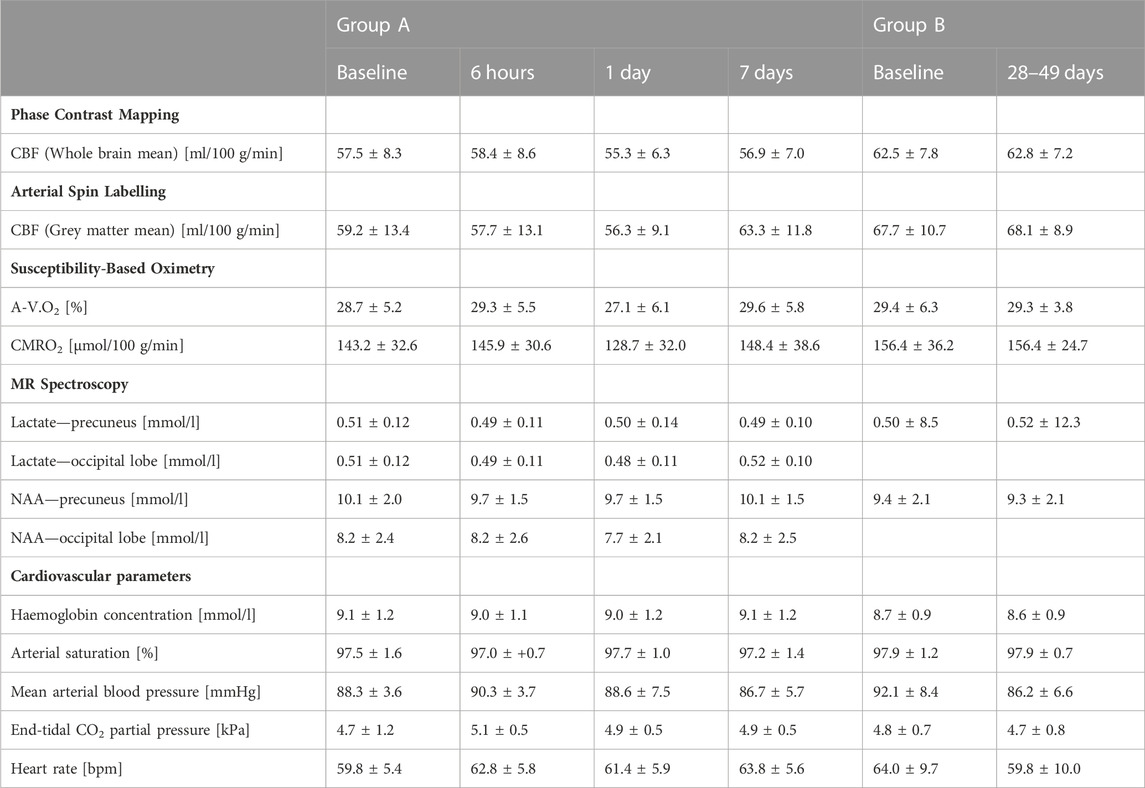
TABLE 1. Summary of the acquired parameters from each MRI session. The means ± standard deviations are noted. Abbreviations: A-V.O2, arteriovenous oxygen saturation difference; CBF, cerebral blood flow; CMRO2, cerebral metabolic rate of oxygen; NAA, N-acetyl-aspartate.
2.2.1 Structural images
Structural brain images were acquired using a sagittal three-dimensional T1-weighted high-resolution Magnetization Prepared Rapid Gradient Echo (MPRAGE) scan (echo time (TE) = 2.8 ms, repetition time (TR) = 6.9 ms, inversion time (TI) = 900 ms; flip angle = 9°, 137 slices, field-of-view (FOV) = 262 × 280 × 150 mm3; voxel size = 1.1 × 1.1 × 1.1 mm3). Bias field correction and segmentation of the brain into grey matter, white matter and cerebrospinal fluid (CSF) was carried out using FAST (FSL 5.0.11, FMRIB, Oxford, UK) (Jenkinson et al., 2012) to estimate brain volume. The anatomical images were additionally used for structural normalisation for the ASL analysis.
2.2.2 Arterial spin labelling (ASL)
Cerebral blood flow maps were acquired using arterial spin labelling (ASL). A dual-echo two-dimensional echo planar imaging pseudo-continuous arterial spin labelling (pCASL) sequence scan with 2 background suppression pulses was used (16 transverse slices (6 mm thick); TE1/TE2 = 12.56/31.66 ms; TR = 4550 ms (for one image); flip angle = 90°; FOV = 240 × 240 mm2; matrix size = 88 × 88 (acquired), 128 × 128 (reconstructed); labelling duration = 1800 ms; postlabelling delay = 1800 ms with an additional slice acquisition time of 32 ms; background suppression (BS) pulses = BS1/BS2: 1813/3135 ms; 60 dynamics, 30 label/control pairs). A calibration scan was acquired with the same imaging parameters using a 16-phase look-locker readout after saturation (saturation delay (TD) = 50 ms; TR = 520 ms; slice timing difference = 32 ms; flip angle = 30°; 2 dynamics). R1 (relaxation rate R1 = 1/T1) was fitted from the saturation recovery signal using Eq. 1 by in-house developed MATLAB (version 9.10.0 (R2021a), The MathWorks Inc, Natick, Massachusetts, United States) programs utilizing a nonlinear least squares Levenberg‒Marquardt method.
TD is the time since saturation, corrected for individual slice timing; TR is the time interval between two consecutive alpha pulses; and n is the index of the alpha pulses, which for this sequence took the values n = [1:16]. The R1 value was used to calculate M0 from the initial six pCASL control measurements using Eq. 2.
BS1/BS2 are the timing of the background suppression pulses and Mctr is the control part of the ASL data pairs. TD is the read-out time after saturation, corrected for slice timing differences.
The perfusion weighted maps were converted to quantitative CBF maps using the voxel-wise M0 signal from Eq. 2 in the BASIL tool (FSL) (Chappell et al., 2009) with additional correction of tissue T1-and R2*-decay. T1 of blood was corrected for each subject’s session-specific haemoglobin concentration using the arterial equation from Lu et al. (Lu et al., 2004). Grey matter mean CBF values were extracted from the grey matter segmentations of the structural images resliced into ASL space using the oxford_asl (part of FSL) tool with the default threshold parameter of 0.8.
2.2.3 Phase contrast mapping
Global mean CBF was acquired using the PCM technique by measuring the blood flow supplying the brain through the carotid and basilary arteries (Bakker et al., 1995; Vestergaard et al., 2017). Velocity maps were acquired by a velocity-encoding turbo field echo sequence (1 slice, FOV = 240 × 240 mm2; voxel size = 0.75 × 0.75 × 8 mm3; TE/TR = 7.33/27.63 ms; flip angle = 10°; velocity encoding = 100 cm/s, without cardiac gating; 10 dynamics). The sequence was recorded twice to obtain optimal perpendicular slice positions on both internal carotid arteries (first scan) and the basilar artery (second scan). Each feeding artery was manually delineated using in-house developed MATLAB scripts. The blood flow to the brain was then calculated as the mean blood velocity times the cross-sectional area of the delineated cerebral arteries. The resulting flow was normalised to brain weight to obtain values in ml/100 g/min. Brain weight was estimated from the segmentation of the structural MRI image, including grey matter and white matter but excluding CSF and assuming a brain density of 1.05 g/ml (Torack et al., 1976).
2.2.4 Susceptibility-based oximetry (SBO)
Cerebral arteriovenous oxygen saturation differences (A-V.O2) and CMRO2 were acquired using susceptibility-based oximetry (SBO) MRI (Jain et al., 2010). Using the SBO technique, the oxygen saturation of the venous blood (SvO2) leaving the brain in the sagittal sinus can be measured. The technique utilizes the magnetic properties of deoxyhaemoglobin in venous blood changes the intravascular magnetic susceptibility, which can be measured by MRI phase images. Susceptibility-weighted phase maps were acquired using a dual-echo gradient-echo sequence (1 slice, FOV = 220 × 190 mm2; voxel size = 0.69 × 0.69 × 8 mm3; TR = 23.1 ms; TE1/TE2 = 8.16/17.83 ms; flip angle = 30°; SENSE factor = 2; 10 dynamics, velocity encoding = 100 cm/s). The imaging plane was placed orthogonal to the sagittal sinus. By manual delineation of the sagittal sinus and the surrounding tissue, SvO2 was calculated. An in-depth description of the postprocessing has been previously published (Vestergaard and Larsson, 2019). A-V.O2 was calculated by subtracting SvO2 from arterial saturation (SaO2) measured using pulse oximetry. CMRO2 was then calculated using the Fick principle (Eq. 3). Haemoglobin (Hgb) concentrations were measured from venous blood sampling.
From this sequence, we simultaneously also acquired phase information from which we could calculate the blood flow in the sagittal sinus by a similar approach as that used for calculating the flow in the feeding cerebral arteries by manual delineation of the sagittal sinus.
2.2.5 Magnetic resonance spectroscopy (MRS)
Cerebral NAA and lactate concentrations were measured using a single-voxel water-suppressed point-resolved 1H-spectroscopy (PRESS) sequence (TE/TR = 288/2000 ms; voxel size = 30 × 35 × 30 mm3; 176 averages, 1,024 complex data points). Precuneus is a part of the default mode network and relatively metabolic stable, whereas the occipital cortex is a region which possibly could demonstrate more metabolic variation. These two regions could therefore possibly demonstrate different reproducibilities. For Group A, two measurements were acquired, one in the precuneus and a second in the occipital lobe. For Group B, only measurements in the precuneus were acquired due to the similar concentrations in the precuneus and occipital lobe observed in Group A.
Postprocessing and quantification of the spectra were performed using LCModel (LCModel, Version 6.3-1F, Toronto, Canada). The water peak acquired in the spectrum was used as reference to the measured metabolites. The water concentration in the spectroscopy voxel was estimated from the content of grey matter, white matter, and CSF within the voxel using the tissue segmentations from the structural images (Quadrelli et al., 2016). NAA and lactate concentrations were corrected for T2 decay using literature values (T2,H2O = 95 ms; T2,NAA = 247 ms; T2,Lac = 240 ms) (Wansapura et al., 1999; Träber et al., 2004).
2.3 Statistics
All values are reported as means ± standard deviations. p-values less than 0.05 were considered significant. Reproducibility between the baseline measurement and subsequent repeated measurements was assessed by linear regression models and Bland‒Altman analysis. R2 values from the regression models and limit of agreement (LoA) metrics and within-subject coefficient of variation (CoVws) from Bland‒Altman analysis were used to assess the reproducibility of the measurements. LoA was calculated as 1.96 times the mean standard deviation of the pairwise differences between baseline and each subsequent measurement.
2.4 Data availability
The parameters derived from the MRI-images and supporting data are available upon reasonable request. The MRI images are not publicly available due to privacy restrictions.
2.5 Code availability
Software used for calculating CBF by the PCM technique is available at https://github.com/MarkVestergaard/PCMCalculator/. Software used to calculate SvO2 from the SBO technique is available at https://github.com/MarkVestergaard/SBOCalculator/.
3 Results
For one subject in Group A, ASL analysis at the baseline measurement failed and this subject was removed from the further analysis of ASL reproducibility. Furthermore, the ASL analysis failed in one subject at the 6-h measurement and two subjects at the 7-day measurement in Group A. The failure to analyse these ASL measurements was due to technical issues with the calibration scan. For the other parameters, all data were satisfactorily acquired.
The average values of the acquired metrics in each MRI session are provided in Table 1. A summary of R2 values from the linear regression, LoA from Bland‒Altman analysis and CoVws for all parameters and correlations are summarized in Table 2. There were no significant differences in baseline values between group A and B.
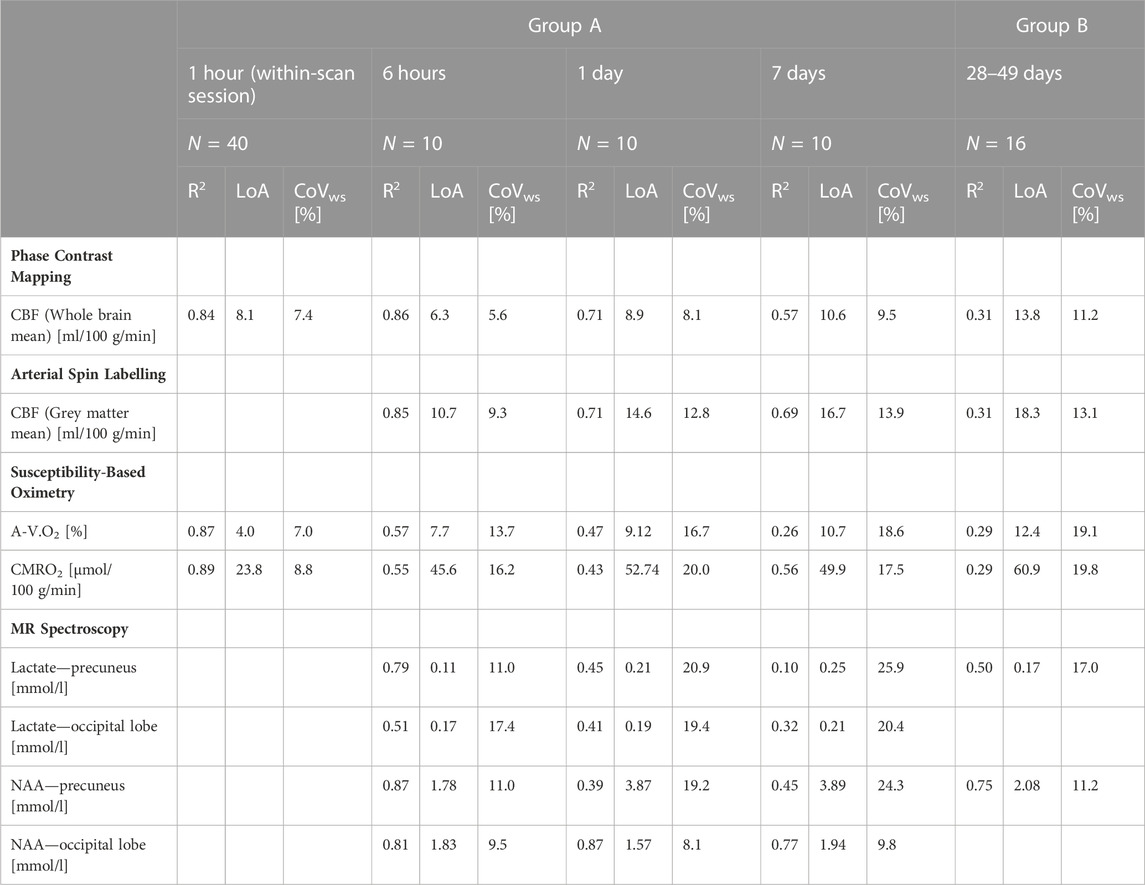
TABLE 2. Summary of R2, limit of agreement (LoA) and within-subject coefficient of variation (CoVws) for the correlations between baseline and the subsequent measurements. Limit of agreement is defined as 1.96 standard deviations from Bland–Altman analysis. Abbreviations: A-V.O2, arteriovenous oxygen saturation difference; CBF, cerebral blood flow; CMRO2, cerebral metabolic rate of oxygen; NAA, N-acetyl-aspartate.
The correlations and Bland‒Altman analysis for global CBF, CMRO2 and A-V.O2 are demonstrated in Figure 2. The correlations for CBF, CMRO2 and A-V.O2 were significant for all comparisons. Correlations and reproducibilities were best for within-day measurements for all parameters (LoA = 6.3 ml/100 g/min; CoVws = 5.6% for CBF and LoA = 45.6 µmol/100 g/min, CoVws = 16.2% for CMRO2) and gradually declined for longer time periods between measurements. The within-session reproducibility of CBF and CMRO2 (Figure 2) demonstrated good reproducibility with low LoA (8.1 ml/100 g/min and 23.8 µmol/100 g/min) and highly significant correlations (R2 = 0.84 and 0.89). However, CBF also demonstrated a significant bias towards lower values for the second measurement in the session compared to the first measurement (first measurement = 57.5 ± 8.3 ml/100 g/min; second measurement = 54.4 ± 7.2 ml/100 g/min, p = 0.0003).
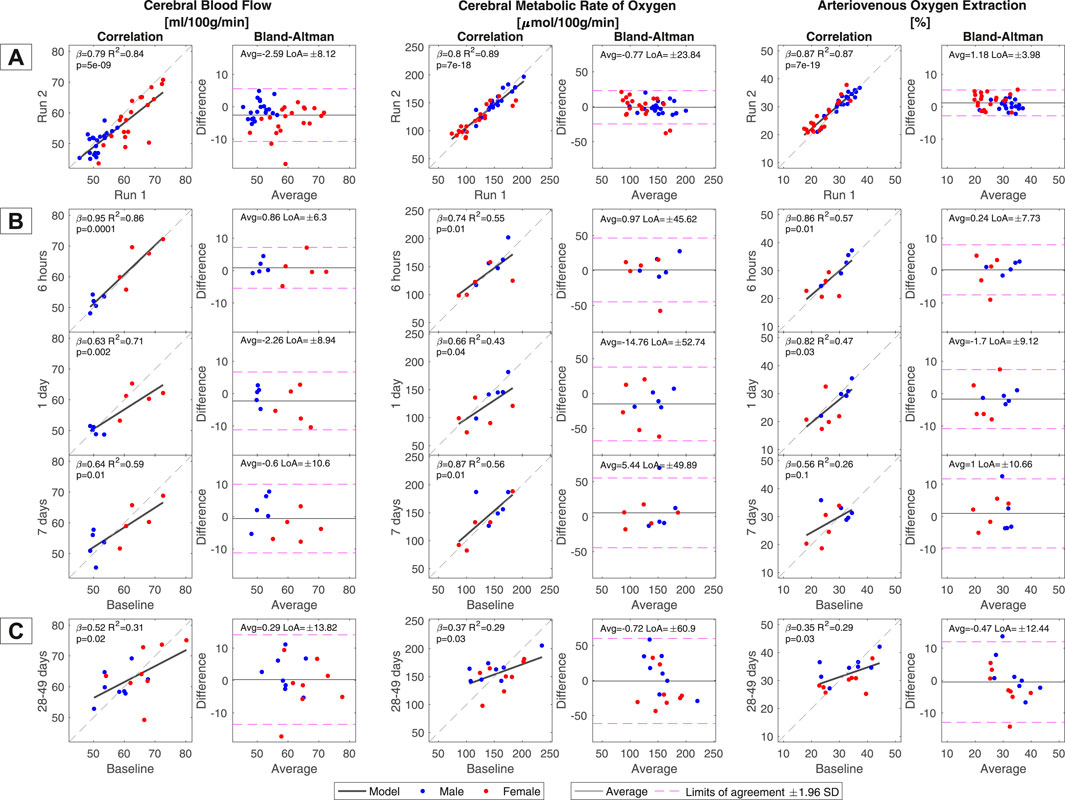
FIGURE 2. Correlation and reproducibility between baseline measurements and the subsequent acquisitions of global cerebral blood flow (CBF), cerebral metabolic rate of oxygen (CMRO2) and arteriovenous oxygen saturation difference (A-V.O2). (A) The within-session correlation was calculated from the duplicate measurements of CBF, A-V.O2 and CMRO2 that were acquired in each MRI session. The correlations between baseline and values from each subsequent MRI from Group A are shown in (B). The correlations between baseline and values remeasured 28–49 days after the initial examination in Group B are shown in (C). A general pattern of weaker correlation with increasing time between sessions is noted. Limits of agreement (LoAs) in the Bland–Altman analysis were calculated as 1.96 standard deviations of the pairwise subtracted values. Sex was not part of the regression model but is highlighted for visual interpretation. The regression slopes (β), R2 coefficients, p-values and LoAs are noted in each panel.
Global CBF values across all sessions were significantly correlated with both haemoglobin concentration (p < 10–4) and A-V.O2 (p = 0.006) (Supplementary Figure S1). Reproducibility of the venous blood flow leaving the brain in the sagittal sinus is presented in Supplementary Figure S2A–C. The blood flow in the sagittal sinus demonstrated similar reproducibility to CBF, including a bias towards lower values at the second compared to the first measurement during the baseline session. There was also a significant correlation between CBF and blood flow in the sagittal sinus (Supplementary Figure S2D).
The mean regional CBF maps from the ASL acquisition and the correlation between the grey matter mean CBF from each session are shown in Figure 3. No significant difference was observed between each session. The voxel-wise LoA maps also did not demonstrate any regional differences in reproducibility for measurements up to 7 days apart. For measurements taken 28–49 days apart, the LoA was generally higher in the cortex than in the rest of the brain. Grey matter mean CBF values from the ASL measurement demonstrated the best reproducibility for within-day measurements and worse reproducibility for the remaining sessions, similar to global CBF values obtained by the PCM method.
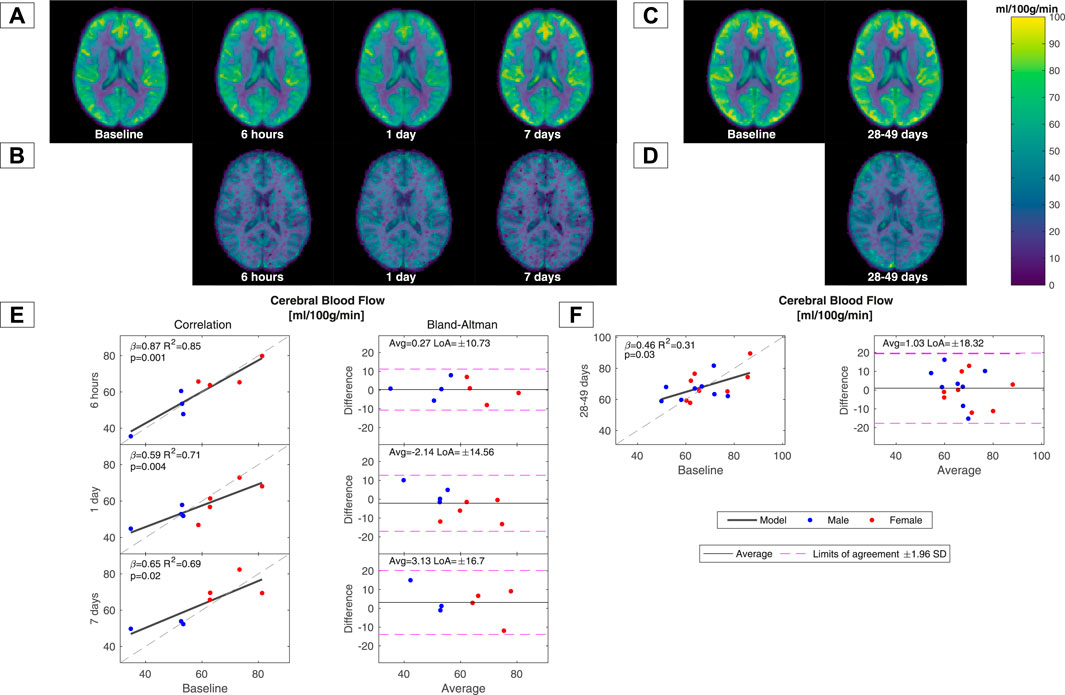
FIGURE 3. CBF maps acquired using arterial spin labelling and correlations of grey matter mean values between baseline and subsequent measurements. Average CBF maps of all subjects in Group A for each session in MNI-152 standard space are shown in (A). Maps demonstrating voxel-wise limits of agreement (LoAs) between the baseline and subsequent measurements in Group A are shown in (B). Average CBF maps from all subjects in Group B at the two MRI sessions are shown in (C). Maps demonstrating voxel-wise LoAs between the baseline measurement and subsequent measurements 28–49 days after the initial examination of Group B are shown in (D). Correlations and Bland‒Altman analysis between the grey matter mean CBF values from the ASL measurement at baseline and subsequent sessions are shown in (E) for Group A and in (F) for Group B. The regression slopes (β), R2 coefficients, p-values, and limits of agreement (LoAs) are noted in each panel.
Measurements of brain size and changes in haemoglobin concentration will affect the calculation of CBF and CMRO2, and we therefore examined the reproducibility of these measurements as well (Supplementary Figure S3). Both estimation of brain size and haemoglobin concentration demonstrated very high reproducibility and therefore only minorly affected the longitudinal variant in CBF and CMRO2 observed in this study. CBF was higher in women across all measurements as also demonstrated in multiple previous studies (Rodriguez et al., 1988; Esposito et al., 1996). There were no sex differences for the remaining parameters.
The correlations and Bland‒Altman analysis of lactate and NAA concentrations are shown in Figure 4. All comparisons demonstrated significant correlations between baseline and subsequent measurements, except for lactate measurements after 7 days, which were only near significant for the measurement in the occipital lobe (p = 0.09) and nonsignificant for the measurement in the precuneus (p = 0.40). The reproducibility was best for the within-day measurements for both lactate and NAA and similarly worse for the longer time periods between measurements. The quality parameters for the MRS data were signal-to-noise (SNR) = 38.8 ± 4.4, full width at half maximum (FWHM) = 0.05 ± 0.01, Cramer-Rao lower bounds (CRLB) for lactate = 25.9 ± 10.8%, CRLB for NAA = 2.9 ± 1.1% for the measurements in the occipital lobe and SNR = 42.5 ± 2.7, FWMH = 0.04 ± 0.01, CRLB for lactate = 20.2 ± 3.9% and CRLB for NAA = 2.1 ± 0.4% for the measurements in precuneus.
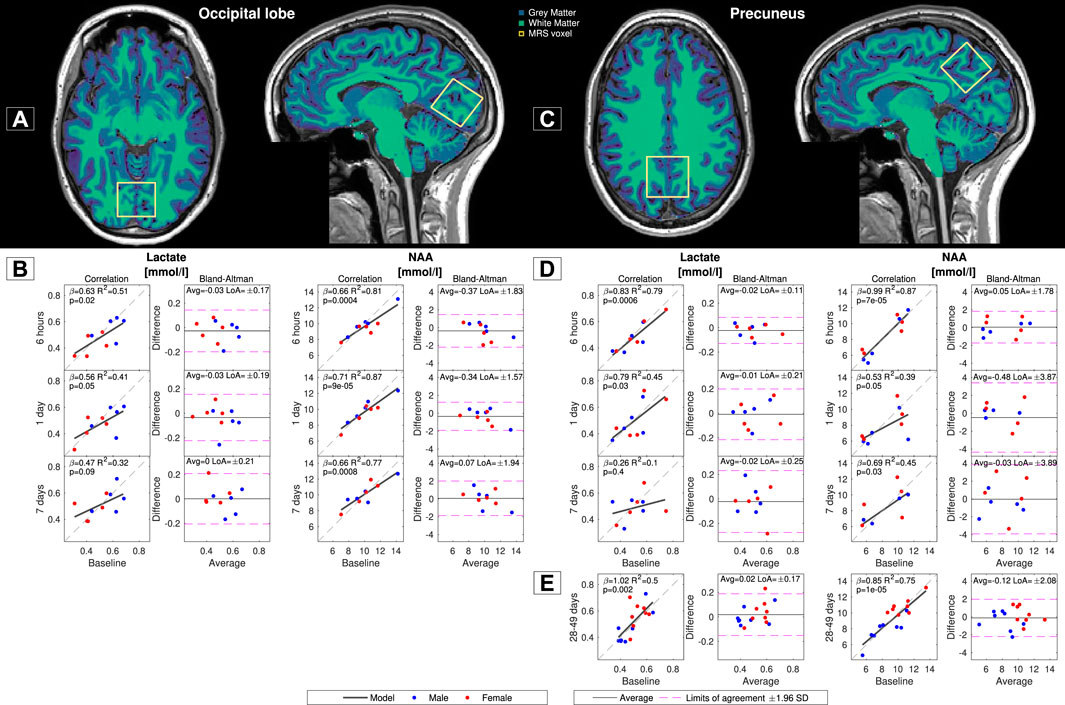
FIGURE 4. Correlation and reproducibility between baseline measurements and the subsequent acquisitions of cerebral lactate and N-acetyl-aspartate (NAA) concentrations. Lactate and NAA concentrations were measured in the occipital lobe (A) and precuneus (C). Correlations and Bland‒Altman analysis of the lactate and NAA concentrations in the occipital lobe between baseline and the subsequent acquisitions from Group A are shown in (B) and those from Group B in (E). Correlations and Bland‒Altman analysis of the lactate and NAA concentrations in the precuneus between baseline and the subsequent acquisitions from Group A are shown in (D) and those from Group B in (E). The regression slopes (β), R2 coefficients, p-values from the regressions and limits of agreement (LoAs) from Bland‒Altman analysis are noted in each panel.
4 Discussion
We observed that repeated measurements of CBF, A-V.O2, CMRO2, lactate and NAA correlated and demonstrated good reproducibility, including up to several weeks between measurements. For CBF and CMRO2, the reproducibility was best for within-day measurements and gradually declined for longer times between measurements. The reproducibility of lactate and NAA in the occipital lobe was similarly best for within-day measurements and gradually declined for the repeated measurements after 1 and 7 days. For lactate and NAA in the precuneus, the reproducibility was again best for within-day measurements and was equally worse for the remaining repeated measurements.
The variation in repeated measurements is caused by both measurement errors from the equipment and physiological changes. Modifications to the MRI scanner could influence the performance and affect the measurement error. Alterations to the MRI scanner, such as updating the scanner software or replacing the MRI coils, might also modify the reproducibility. However, there were no such modifications on the MRI scanner in the current study, and we do not anticipate the scanner to have significantly deteriorated during the project duration. Overall, we believe that the measurement error of the MRI scanner was similar in all MRI sessions, and we do not expect that any systematic changes occurred. Consequently, we think that the gradual decline in reproducibility we noticed with longer spans between measurements is due to physiological variation. The decreased reproducibility is therefore expected to be similar when using modalities other than MRI scans.
4.1 CBF
The techniques we used to measure CBF are noninvasive and widely available on MRI scanners, making them ideal for studies requiring multiple repeated measurements. Both PCM and ASL are therefore extensively used in research on brain physiology. We found CBF values similar to previously reported values using the same methods.
Cerebral perfusion is dynamically regulated to ensure sufficient delivery of nutrients, most notably oxygen, to the brain. Numerous factors affect CBF, for example, changes in blood gas tension, such as oxygen and CO2; haemoglobin concentration; and hormonal variations (Kety and Schmidt, 1948; Henriksen et al., 2013; Vestergaard and Larsson, 2019). Intake of certain compounds, such as caffeine or nicotine, will also affect CBF (Zubieta et al., 2005; Krause et al., 2006). Therefore, it is to be expected that CBF will also vary within subjects and knowing the reproducibility over different time periods is important when studying CBF. Generally, we observed better reproducibility for shorter periods between examinations, with the lowest LoA and best correlation for the within-session measurements. However, we also observed a small but significant bias towards lower CBF at the second measurement in the within-session examination, also resulting in a relatively high CoVws. There were approximately 60 min between these two measurements. Interestingly, this lower CBF did not reflect changes in CMRO2, suggesting that the effect on CBF from lying in the scanner is not due to changes in cerebral energy metabolism but rather an effect directly on CBF. An effect on CBF from lying for a prolonged time in an MRI scanner should therefore be considered when designing experiments on brain physiology with interventions in the scanner. Prior studies on the reproducibility of CBF have often included just two repeated examinations; therefore, the time variant effects could not be investigated. However, studies on within-day or within-session repeated measurements have generally shown good reproducibility, and studies using longer periods between examinations have similarly shown poorer reproducibility (Fan et al., 2016). A previous study on the reproducibility of CBF measurement by PCM demonstrated slightly worse reproducibility for within-session and within-day examinations compared to our study and similar reproducibility for between-day examinations (Spilt et al., 2002). Another study using 7 weeks between measurements and the ASL technique for the measurement of CBF found reproducibility for whole-brain values similar to our data (Hermes et al., 2007). A study using PET imaging with oxygen-15-labelled water [15O-H2O] as the radiotracer and a study using ASL MRI found slightly worse within-session reproducibility compared to our study (Coles et al., 2006; Gevers et al., 2009). A study comparing CBF values obtained from ASL MRI against values acquired by 15O-H2O PET found similar reproducibilities for measurements with 25–45 days apart compared to our reproducibility for measurements with 28–49 days apart but poorer within-session reproducibility (Heijtel et al., 2014). A study using the dynamic contrast-enhanced (DCE) MRI technique found similar reproducibility for the measurement of grey and white matter separately 1 week apart compared to our whole-brain measurement (Cramer et al., 2023). Overall, the results from our study suggest that the differences in reproducibility observed in different studies are likely, at least partly, a result of the various lengths of time between examinations used in the studies.
Most studies on CBF reproducibility examine homogenous groups of healthy young adults, as was the case for this study. For example, the subjects examined had similar ages, were non-smokers and did not have any diseases or take medicine that could affect vascular function. Investigations of less homogenous groups could likely demonstrate poorer reproducibility.
4.2 CMRO2
For CMRO2, we observed values similar to those acquired using invasive PET imaging (Bremmer et al., 2011; Kudomi et al., 2013) or blood sampling from jugular vein catheters (Ainslie et al., 2014). The SBO technique used to measure venous oxygen saturation in the sagittal sinus for calculation of CMRO2 has been validated against blood samples acquired by catheter from the jugular vein during MRI scanning (Miao et al., 2019). Reproducibility of CMRO2 has been far less studied than CBF, likely due to the technical difficulties in acquisition compared to CBF. A study using T2-Relaxation-Under-Spin-Tagging (TRUST) MRI for the measurement of venous oxygen saturation and PCM for CBF found reproducibility for within-session repeated measurements comparable to our study but better between-day (1–14 days) reproducibility (Liu et al., 2013). A study using calibrated BOLD imaging and a complicated calibration scheme involving inhalation of hypercapnic and hyperoxic air to quantify CMRO2 observed a slightly worse between day reproducibility of grey matter CMRO2 compared to our whole brain CMRO2 (Lajoie et al., 2016). The method relies on many parameters which are either fitted or derived from literature values and inaccuracies in these parameters could be the cause for the slightly poorer reproducibility.
Studies using PET imaging and inhalation of oxygen-15 as a radiotracer for CMRO2 measurements have demonstrated very high reproducibility for within-session repeated measurements (Coles et al., 2006). Another PET study examining CMRO2 using inhalation of oxygen-15 with an interval of 3 to 54 days between measurements found significantly better reproducibility than our results with 7 days or several weeks between measurements (Bremmer et al., 2011). Overall, this suggests that the PET technique for CMRO2 measurements has better reproducibility than using combined PCM and SBO, as in this study. However, using PET and oxygen-15 is also significantly more cumbersome, time-consuming, and invasive than MRI techniques. Generally, studies on the reproducibility of CMRO2 are few, and more studies using different techniques and with different timespans between examinations should be performed in the future.
4.3 Lactate and NAA
We observed relatively stable and reproducible concentrations for both lactate and NAA in both the occipital lobe and precuneus. Generally, we observed similar trends in correlations and reproducibility for lactate and CMRO2, both related to energy metabolism, suggesting that the variation in lactate and CMRO2 could be due to fluctuations in overall energy metabolism.
Studies on the reproducibility of MRS, similar to those on CBF and CMRO2, have often compared only two repeated examinations, and time variant effects were not considered. Furthermore, the various placements of the MRS voxels, the exact technique used, and the patient groups studied make it difficult to compare results.
It has been shown that NAA can be affected by brain diseases which impairs the brain metabolism, such as dementia or multiple sclerosis, however it would be expected that in healthy subjects the NAA concentration is relatively stable over time (Moffett et al., 2007). One study has demonstrated a very high intersession reproducibility of NAA with a voxel primarily located in white matter (Brooks et al., 1999). Another study using spectroscopic imaging with repeated measurements between a few days and several months apart found reasonable reproducibility of the NAA/creatine ratio in grey matter (Tedeschi et al., 1996). Studies using a 7 T MRI scanner found a reproducibility for NAA of 5.3% in the anterior cingulate cortex with 2-3 months between visits, which is better than the reproducibility we observed in the precuneus (Wijtenburg et al., 2019). The higher field strength of the MRI scanner in that study compared to ours could be a reason for the better reproducibility. Studies on lactate reproducibility are very limited; however, one study examined the reproducibility of lactate in the posterior cingulate using 7 T MRI with 1 week between measurements and found similar values to our measurement of lactate in the precuneus with 1 week between measurements (Terpstra et al., 2016).
4.4 Strengths and limitations
The main strength of the study is that we measured multiple parameters in the same subjects and acquired data from multiple repeated measurements at distinct time points after the initial baseline measurement. This enables us to examine time variant changes in reproducibility.
A limitation is that we only examined young healthy subjects. In cohorts of older individuals or subjects with certain diseases, the reproducibility might be lower than in young subjects. This should be considered when applying the results in projects conducting research on other subject groups. It should also be noted that all parameters in this study are measured on the same scanner with optimised MRI sequence parameters set for our purposes. Thus, this does not guarantee a direct translation to different scanners or sequence parameters, e.g., single echo pCASL instead of dual echo pCASL.
Another limitation is that we do not have a sufficient number of participants to examine which and to what extend the systemic factors explain the variation of the cerebral physiology. Systemic factors, such as SaO2 or arterial CO2 partial pressure, are known to impact CBF and changes in these parameters could account for some of the alterations in cerebral physiology over time. We observed, on average, stable values of SaO2, PetCO2, heart rate and blood pressure between the MRI sessions and a relatively narrow span of values across participants, suggesting limited effect from changes of these parameters. Nevertheless, individual natural fluctuations of systemic factors could likely still explain some of the variation in the cerebral physiology. Larger studies are needed to quantify the contributions arising from fluctuations in the various systemic factors on the cerebral physiology.
4.5 Conclusion
Overall, the results from the present study demonstrate satisfactorily good reproducibility of cerebral physiology measurements using non-invasive MRI techniques. We observed the best reproducibility for short timespans between the examinations and, generally, a gradual worsening of the reproducibility for longer times between measurements. Reproducibility in the context of evaluating an intervention or disease evolution should therefore be estimated based on the time scale of the study.
Data availability statement
The datasets presented in this article are not readily available because of privacy restrictions. The parameters derived from the MRI-images and supporting data are available upon reasonable request. Requests to access the datasets should be directed to bWFyay5iaXRzY2gudmVzdGVyZ2FhcmRAcmVnaW9uaC5kaw==.
Ethics statement
The studies involving humans were approved by the Scientific Ethical Committee of the Capital Region of Denmark. The studies were conducted in accordance with the local legislation and institutional requirements. The participants provided their written informed consent to participate in this study.
Author contributions
SM: conceptualisation, investigation, writing—original draft. UL: methodology, data curation, formal analysis, software, visualization, writing—original draft. SA: conceptualization, project administration, funding acquisition, resources, writing—review and editing. KO: project administration, writing—review and editing. KM: conceptualisation, project administration, resources, writing—review and editing. HL: conceptualisation, methodology, formal analysis, writing—review and editing. MV: conceptualisation, methodology, data curation, formal analysis, software, writing—original draft. All authors contributed to the article and approved the submitted version.
Funding
The study was supported by fundings from Læge Sofus Carl Emil Friis og Hustru Olga Doris Friis Legat and AP Møller Fonden. MV was funded by grant from the Lundbeck Foundation (R347-2020-217).
Acknowledgments
The authors thank Maria Højberg Knudsen for invaluable help executing MRI sessions, and research assistants Mathilde Aya Røen, Kübra Hidiroglu, Ingeborg By Raaum, Nanna Eriksen, Maryam Noory and Bardha Murtezaj for a steady team effort during data collection. Special thanks to all participants for making our research possible.
Conflict of interest
The authors declare that the research was conducted in the absence of any commercial or financial relationships that could be construed as a potential conflict of interest.
Publisher’s note
All claims expressed in this article are solely those of the authors and do not necessarily represent those of their affiliated organizations, or those of the publisher, the editors and the reviewers. Any product that may be evaluated in this article, or claim that may be made by its manufacturer, is not guaranteed or endorsed by the publisher.
Supplementary material
The Supplementary Material for this article can be found online at: https://www.frontiersin.org/articles/10.3389/fphys.2023.1213352/full#supplementary-material
Abbreviations
A-V.O2, Arteriovenous oxygen saturation difference; ASL, Arterial spin labelling; CBF, Cerebral blood flow; CMRO2, Cerebral metabolic rate of oxygen; CoVws, Within-subject coefficient of variation; CSF, Cerebrospinal fluid; FOV, Field of view; LoA, Limit of agreement; MRS, Magnetic resonance spectroscopy; NAA, N-acetyl aspartate; pCASL, Pseudo-continuous arterial spin labelling; PCM, Phase contrast mapping; PET, positron emission tomography; SBO, susceptibility-based oximetry; SaO2, oxygen saturation of the arterial blood; SvO2, oxygen saturation of the venous blood; TD, Saturation delay; TE, Echo time; TI, Inversion time; TR, Repetition time.
References
Ainslie, P. N., Shaw, A. D., Smith, K. J., Willie, C. K., Ikeda, K., Graham, J., et al. (2014). Stability of cerebral metabolism and substrate availability in humans during hypoxia and hyperoxia. Clin. Sci. 126, 661. doi:10.1042/CS20130343
Bakker, C. J. G., Kouwenhoven, M., Hartkamp, M. J., Hoogeveen, R. M., and Mali, W. P. T. M. (1995). Accuracy and precision of time-averaged flow as measured by nontriggered 2D phase-contrast MR angiography, a phantom evaluation. Magn. Reson. Imaging 13, 959–965. doi:10.1016/0730-725X(95)02005-E
Bitsch, A., Bruhn, H., Vougioukas, V., Stringaris, A., Lassmann, H., Frahm, J., et al. (1999). Inflammatory CNS demyelination: histopathologic correlation with in vivo quantitative proton MR spectroscopy. AJNR Am. J. Neuroradiol. 20, 1619–1627.
Bremmer, J. P., Van Berckel, B. N. M., Persoon, S., Kappelle, L. J., Lammertsma, A. A., Kloet, R., et al. (2011). Day-to-Day test–retest variability of CBF, CMRO2, and OEF measurements using dynamic 15O PET studies. Mol. Imaging Biol. 13, 759–768. doi:10.1007/s11307-010-0382-1
Brooks, W. M., Friedman, S. D., and Stidley, C. A. (1999). Reproducibility of1H-MRS in vivo. Magn. Reson. Med. 41, 193–197. doi:10.1002/(SICI)1522-2594
Chappell, M. A., Groves, A. R., Whitcher, B., and Woolrich, M. W. (2009). Variational bayesian inference for a nonlinear forward model. IEEE Trans. Signal Process. 57, 223–236. doi:10.1109/TSP.2008.2005752
Coles, J. P., Fryer, T. D., Bradley, P. G., Nortje, J., Smielewski, P., Rice, K., et al. (2006). Intersubject variability and reproducibility of 15 O PET studies. J. Cereb. Blood Flow. Metab. 26, 48–57. doi:10.1038/sj.jcbfm.9600179
Cramer, S. P., Larsson, H. B. W., Knudsen, M. H., Simonsen, H. J., Vestergaard, M. B., and Lindberg, U. (2023). Reproducibility and optimal arterial input function selection in dynamic contrast-enhanced perfusion MRI in the healthy brain. J. Magn. Reson. Imaging 57, 1229–1240. doi:10.1002/jmri.28380
Esposito, G., Van Horn, J. D., Weinberger, D. R., and Berman, K. F. (1996). Gender differences in cerebral blood flow as a function of cognitive state with PET. J. Nucl. Med. Off. Publ. Soc. Nucl. Med. 37, 559–564.
Fan, A. P., Jahanian, H., Holdsworth, S. J., and Zaharchuk, G. (2016). Comparison of cerebral blood flow measurement with [ 15 O]-water positron emission tomography and arterial spin labeling magnetic resonance imaging: A systematic review. J. Cereb. Blood Flow. Metab. 36, 842–861. doi:10.1177/0271678X16636393
Gevers, S., Majoie, C. B. L. M., Van Den Tweel, X. W., Lavini, C., and Nederveen, A. J. (2009). Acquisition time and reproducibility of continuous arterial spin-labeling perfusion imaging at 3T. Am. J. Neuroradiol. 30, 968–971. doi:10.3174/ajnr.A1454
Goyal, M. S., Hawrylycz, M., Miller, J. A., Snyder, A. Z., and Raichle, M. E. (2014). Aerobic glycolysis in the human brain is associated with development and neotenous gene expression. Cell. Metab. 19, 49–57. doi:10.1016/j.cmet.2013.11.020
Heijtel, D. F. R., Mutsaerts, H. J. M. M., Bakker, E., Schober, P., Stevens, M. F., Petersen, E. T., et al. (2014). Accuracy and precision of pseudo-continuous arterial spin labeling perfusion during baseline and hypercapnia: A head-to-head comparison with ¹⁵O H₂O positron emission tomography. NeuroImage 92, 182–192. doi:10.1016/j.neuroimage.2014.02.011
Henriksen, O. M., Kruuse, C., Olesen, J., Jensen, L. T., Larsson, H. B., Birk, S., et al. (2013). Sources of variability of resting cerebral blood flow in healthy subjects: A study using 133 Xe spect measurements. J. Cereb. Blood Flow. Metab. 33, 787–792. doi:10.1038/jcbfm.2013.17
Hermes, M., Hagemann, D., Britz, P., Lieser, S., Rock, J., Naumann, E., et al. (2007). Reproducibility of continuous arterial spin labeling perfusion MRI after 7 weeks. Magn. Reson. Mat. Phys. Biol. Med. 20, 103–115. doi:10.1007/s10334-007-0073-3
Hyder, F., Herman, P., Bailey, C. J., Møller, A., Globinsky, R., Fulbright, R. K., et al. (2016). Uniform distributions of glucose oxidation and oxygen extraction in gray matter of normal human brain: no evidence of regional differences of aerobic glycolysis. J. Cereb. Blood Flow. Metab. 36, 903–916. doi:10.1177/0271678X15625349
Iida, A., Okazawa, H., Hayashida, K., Tsuyuguchi, N., Ishii, K., Kuwabara, Y., et al. (2004). Database of normal human cerebral blood flow, cerebral blood volume, cerebral oxygen extraction fraction and cerebral metabolic rate of oxygen measured by positron emission tomography with 15 O-labelled carbon dioxide or water, carbon monoxide and oxygen: A multicentre study in Japan. Eur. J. Nucl. Med. Mol. Imaging 31, 635–643. doi:10.1007/s00259-003-1430-8
Jain, V., Langham, M. C., and Wehrli, F. W. (2010). MRI estimation of global brain oxygen consumption rate. J. Cereb. Blood Flow. Metab. 30, 1598–1607. doi:10.1038/jcbfm.2010.49
Jenkinson, M., Beckmann, C. F., Behrens, T. E. J., Woolrich, M. W., and Smith, S. M. (2012). FSL. NeuroImage 62, 782–790. doi:10.1016/j.neuroimage.2011.09.015
Kety, S. S., and Schmidt, C. F. (1948). The effects of altered arterial tensions of carbon dioxide and oxygen on cerebral blood flow and cerebral oxygen consumption of normal young men. J. Clin. Investig. 27, 484–492. doi:10.1172/JCI101995
Krause, D. N., Duckles, S. P., and Pelligrino, D. A. (2006). Influence of sex steroid hormones on cerebrovascular function. J. Appl. Physiol. 101, 1252–1261. doi:10.1152/japplphysiol.01095.2005
Kudomi, N., Hirano, Y., Koshino, K., Hayashi, T., Watabe, H., Fukushima, K., et al. (2013). Rapid quantitative CBF and CMRO2 measurements from a single PET scan with sequential administration of dual 15 O-labeled tracers. J. Cereb. Blood Flow. Metab. 33, 440–448. doi:10.1038/jcbfm.2012.188
Lajoie, I., Tancredi, F. B., and Hoge, R. D. (2016). Regional reproducibility of BOLD calibration parameter M, OEF and resting-state CMRO2 measurements with QUO2 MRI. PLoS ONE 11, 01630711–e163131. doi:10.1371/journal.pone.0163071
Lassen, N. A. (1959). Cerebral blood flow and oxygen consumption in man. Physiol. Rev. 39, 183–238. doi:10.1152/physrev.1959.39.2.183
Liddle, P. F., Friston, K. J., Frith, C. D., Hirsch, S. R., Jones, T., and Frackowiak, R. S. J. (1992). Patterns of cerebral blood flow in schizophrenia. Br. J. Psychiatry 160, 179–186. doi:10.1192/bjp.160.2.179
Liu, P., Xu, F., and Lu, H. (2013). Test-retest reproducibility of a rapid method to measure brain oxygen metabolism. Magn. Reson. Med. 69, 675–681. doi:10.1002/mrm.24295
Lu, H., Clingman, C., Golay, X., and Van Zijl, P. C. M. (2004). Determining the longitudinal relaxation time (T1) of blood at 3.0 Tesla. Magn. Reson. Med. 52, 679–682. doi:10.1002/mrm.20178
Madsen, S. S., Møller, K., Olsen, K. S., Vestergaard, M. B., Lindberg, U., Larsson, H. B. W., et al. (2020). Neuroplasticity induced by general anaesthesia: study protocol for a randomised cross-over clinical trial exploring the effects of sevoflurane and propofol on the brain – a 3-T magnetic resonance imaging study of healthy volunteers. Trials 21, 805. doi:10.1186/s13063-020-04468-y
Miao, X., Nayak, K. S., and Wood, J. C. (2019). In vivo validation of T2- and susceptibility-based S v O 2 measurements with jugular vein catheterization under hypoxia and hypercapnia. Magn. Reson. Med. 82, 2188–2198. doi:10.1002/mrm.27871
Moffett, J., Ross, B., Arun, P., Madhavarao, C., and Namboodiri, A. (2007). N-Acetylaspartate in the CNS: from neurodiagnostics to neurobiology. Prog. Neurobiol. 81, 89–131. doi:10.1016/j.pneurobio.2006.12.003
Oshima, T., Karasawa, F., Okazaki, Y., Wada, H., and Satoh, T. (2003). Effects of sevoflurane on cerebral blood flow and cerebral metabolic rate of oxygen in human beings: A comparison with isoflurane. Eur. J. Anaesthesiol. 20, 543–547. doi:10.1017/S0265021503000863
Paulson, O. B., Strandgaard, S., and Edvinsson, L. (1990). Cerebral autoregulation. Cerebrovasc. Brain Metab. Rev. 2, 161–192.
Peng, S.-L., Dumas, J. A., Park, D. C., Liu, P., Filbey, F. M., McAdams, C. J., et al. (2014). Age-related increase of resting metabolic rate in the human brain. NeuroImage 98, 176–183. doi:10.1016/j.neuroimage.2014.04.078
Powers, W. J., Grubb, R. L., Darriet, D., and Raichle, M. E. (1985). Cerebral blood flow and cerebral metabolic rate of oxygen requirements for cerebral function and viability in humans. J. Cereb. Blood Flow. Metab. 5, 600–608. doi:10.1038/jcbfm.1985.89
Quadrelli, S., Mountford, C., and Ramadan, S. (2016). Hitchhiker’S guide to voxel segmentation for partial volume correction of in vivo magnetic resonance spectroscopy. Magn. Reson. Insights 9, 1–8. MRI. doi:10.4137/MRI.S32903
Rodriguez, G., Warkentin, S., Risberg, J., and Rosadini, G. (1988). Sex differences in regional cerebral blood flow. J. Cereb. Blood Flow. Metab. 8, 783–789. doi:10.1038/jcbfm.1988.133
Snyder, H. M., Corriveau, R. A., Craft, S., Faber, J. E., Greenberg, S. M., Knopman, D., et al. (2015). Vascular contributions to cognitive impairment and dementia including Alzheimer’s disease. Alzheimers Dement. 11, 710–717. doi:10.1016/j.jalz.2014.10.008
Spilt, A., Box, F. M. A., Van Der Geest, R. J., Reiber, J. H. C., Kunz, P., Kamper, A. M., et al. (2002). Reproducibility of total cerebral blood flow measurements using phase contrast magnetic resonance imaging. J. Magn. Reson. Imaging 16, 1–5. doi:10.1002/jmri.10133
Tedeschi, G., Bertolino, A., Campbell, G., Barnett, A. S., Duyn, J. H., Jacob, P. K., et al. (1996). Reproducibility of proton MR spectroscopic imaging findings. AJNR Am. J. Neuroradiol. 17, 1871–1879.
Terpstra, M., Cheong, I., Lyu, T., Deelchand, D. K., Emir, U. E., Bednařík, P., et al. (2016). Test-retest reproducibility of neurochemical profiles with short-echo, single-voxel MR spectroscopy at 3T and 7T: test-Retest Reproducibility of Neurochemical Profiles at 3T and 7T. Magn. Reson. Med. 76, 1083–1091. doi:10.1002/mrm.26022
Torack, R. M., Alcala, H., Gado, M., and Burton, R. (1976). Correlative assay of computerized cranial tomography (CCT), water content and specific gravity in normal and pathological postmortem brain. J. Neuropathol. Exp. Neurol. 35, 385–392. doi:10.1097/00005072-197607000-00001
Träber, F., Block, W., Lamerichs, R., Gieseke, J., and Schild, H. H. (2004). 1 H metabolite relaxation times at 3.0 tesla: measurements of T1 and T2 values in normal brain and determination of regional differences in transverse relaxation: 1 h metabolite relaxation times at 3.0 T. J. Magn. Reson. Imaging 19, 537–545. doi:10.1002/jmri.20053
Vestergaard, M. B., Ghanizada, H., Lindberg, U., Arngrim, N., Paulson, O. B., Gjedde, A., et al. (2022). Human cerebral perfusion, oxygen consumption, and lactate production in response to hypoxic exposure. Cereb. Cortex 32, 1295–1306. doi:10.1093/cercor/bhab294
Vestergaard, M. B., Jensen, M. L., Arngrim, N., Lindberg, U., and Larsson, H. B. (2020). Higher physiological vulnerability to hypoxic exposure with advancing age in the human brain. J. Cereb. Blood Flow. Metab. 40, 341–353. doi:10.1177/0271678X18818291
Vestergaard, M. B., and Larsson, H. B. (2019). Cerebral metabolism and vascular reactivity during breath-hold and hypoxic challenge in freedivers and healthy controls. J. Cereb. Blood Flow. Metab. 39, 834–848. doi:10.1177/0271678X17737909
Vestergaard, M. B., Lindberg, U., Aachmann-Andersen, N. J., Lisbjerg, K., Christensen, S. J., Law, I., et al. (2016). Acute hypoxia increases the cerebral metabolic rate – A magnetic resonance imaging study. J. Cereb. Blood Flow. Metab. 36, 1046–1058. doi:10.1177/0271678X15606460
Vestergaard, M. B., Lindberg, U., Aachmann-Andersen, N. J., Lisbjerg, K., Christensen, S. J., Rasmussen, P., et al. (2017). Comparison of global cerebral blood flow measured by phase-contrast mapping MRI with 15 O-H 2 O positron emission tomography: CBF measured by PCM MRI and 15 O-H 2 O PET. J. Magn. Reson. Imaging 45, 692–699. doi:10.1002/jmri.25442
Wansapura, J. P., Holland, S. K., Dunn, R. S., and Ball, W. S. (1999). NMR relaxation times in the human brain at 3.0 tesla. J. Magn. Reson. Imaging 9, 531–538. doi:10.1002/(SICI)1522-2586
Wijtenburg, S. A., Rowland, L. M., Oeltzschner, G., Barker, P. B., Workman, C. I., and Smith, G. S. (2019). Reproducibility of brain MRS in older healthy adults at 7T. NMR Biomed. 32, e4040. doi:10.1002/nbm.4040
Wolters, F. J., Zonneveld, H. I., Hofman, A., Van Der Lugt, A., Koudstaal, P. J., Vernooij, M. W., et al. (2017). Cerebral perfusion and the risk of dementia: A population-based study. Circulation 136, 719–728. doi:10.1161/CIRCULATIONAHA.117.027448
Younis, S., Christensen, C. E., Vestergaard, M. B., Lindberg, U., Tolnai, D., Paulson, O. B., et al. (2021). Glutamate levels and perfusion in pons during migraine attacks: A 3T MRI study using proton spectroscopy and arterial spin labeling. J. Cereb. Blood Flow. Metab. 41, 604–616. doi:10.1177/0271678X20906902
Keywords: cerebral blood flow, cerebral metabolic rate of oxygen, cerebral lactate, phase contrast mapping, arterial spin labelling, reproducibility, N-acetyl-aspartate
Citation: Madsen SS, Lindberg U, Asghar S, Olsen KS, Møller K, Larsson HBW and Vestergaard MB (2023) Reproducibility of cerebral blood flow, oxygen metabolism, and lactate and N-acetyl-aspartate concentrations measured using magnetic resonance imaging and spectroscopy. Front. Physiol. 14:1213352. doi: 10.3389/fphys.2023.1213352
Received: 27 April 2023; Accepted: 21 August 2023;
Published: 05 September 2023.
Edited by:
Alex Bhogal, Utrecht University, NetherlandsReviewed by:
Binu P. Thomas, University of Texas Southwestern Medical Center, United StatesHenk J. M. M. Mutsaerts, VU Medical Center, Netherlands
Copyright © 2023 Madsen, Lindberg, Asghar, Olsen, Møller, Larsson and Vestergaard. This is an open-access article distributed under the terms of the Creative Commons Attribution License (CC BY). The use, distribution or reproduction in other forums is permitted, provided the original author(s) and the copyright owner(s) are credited and that the original publication in this journal is cited, in accordance with accepted academic practice. No use, distribution or reproduction is permitted which does not comply with these terms.
*Correspondence: Mark Bitsch Vestergaard, bWFyay5iaXRzY2gudmVzdGVyZ2FhcmRAcmVnaW9uaC5kaw==
†These authors have contributed equally to this work and share first authorship