- 1School of Life Sciences, Jiangsu Normal University, Xuzhou, China
- 2Hangzhou Institute of Test and Calibration for Quality and Technology Supervision, Hangzhou, China
- 3Jiangsu Provincial Key Laboratory for Organic Solid Waste Utilization, National Engineering Research Center for Organic-Based Fertilizers, Jiangsu Collaborative Innovation Center for Solid Organic Waste Resource Utilization, Nanjing Agricultural University, Nanjing, China
- 4Jiangsu Keybio Co., Ltd, Xuzhou, China
Despite the physiological significance of effective CO2 diffusion across biological membranes, the underlying mechanism behind this process is not yet resolved. Particularly debatable is the existence of CO2-permeable aquaporins. The lipophilic characteristic of CO2 should, according to Overton’s rule, result in a rapid flux across lipid bilayers. However, experimental evidence of limited membrane permeability poses a challenge to this idea of free diffusion. In this review, we summarized recent progress with regard to CO2 diffusion, and discussed the physiological effects of altered aquaporin expression, the molecular mechanisms of CO2 transport via aquaporins, and the function of sterols and other membrane proteins in CO2 permeability. In addition, we highlight the existing limits in measuring CO2 permeability and end up with perspectives on resolving such argument either by determining the atomic resolution structure of CO2 permeable aquaporins or by developing new methods for measuring permeability.
Introduction
More than 30 years ago, aquaporin was found to be a highly specialized water channel protein in erythrocytes (Preston et al., 1992; Agre, 2004). The discovery of aquaporins changed our perspective on the highly controlled permeability of biological membranes, which had previously been explained by the paradigm of free diffusion of water transport across membranes (Edidin, 2003). Aquaporins are a class of structurally conserved proteins that have been shown to function as channels for a wide range of neutral chemicals since their discovery. These molecules include glycerol (Jensen et al., 2001; Nollert et al., 2001), urea (Ishibashi et al., 1994; Ma et al., 1997), hydrogen peroxide (Almasalmeh et al., 2014), ammonia (Jahn et al., 2004; Kirscht et al., 2016), and even the gas molecule-carbon dioxide (Uehlein et al., 2003). Such a wide range of substrate selectivity suggests that biological membrane permeability is tightly controlled and is not just based on passive diffusion across the lipid bilayer. More than a century ago, Meyer and Overton proposed that the membrane permeability of a given solute is closely associated with its lipid solubility [also known as Overton’s rule (Missner and Pohl, 2009)]. For many molecules, experimental evidence confirmed this rule (Missner et al., 2008a), but some did not follow the prediction. The appearance of these molecules begs the question of whether or not Overton’s rule alone can account for the passage of molecules through biological membranes. Among those molecules that deviated from the prediction by Overton’s rule, CO2 was intensively investigated due to its role as a physiological component for essential process, i.e., respiration and photosynthesis. Despite the predicted high permeability of CO2, accumulated evidences from biologists found that certain cell membranes are remarkably resistant to CO2, which could not be explained solely by Overton’s rule due to its high lipophilic property. To address this challenge, Pohl’s team has introduced the effect of unstirred layers (USLs) and buffer, which account for a significant portion of the diffusion barrier of the lipid bilayer (Missner and Pohl, 2009). While research directed by Kaldenhoff and Boron independently demonstrated the existence of aquaporin-mediated CO2 transport in regulating the CO2 diffusion across biological membranes (Nakhoul et al., 1998; Uehlein et al., 2003). Since then, discussion has been continued with regard to the potential biological significance of CO2 channels in regulating CO2 transport across biological membranes. As more relevant results continue to uncover the complexities of CO2 movement across biological membranes, several questions have emerged: Why do some biological membranes have such low intrinsic CO2 permeability? How do biological systems deal with the conflict between the need for fast gas exchange and the low intrinsic permeability of the CO2 membrane? Is there a CO2 channel protein that exists in addition to free diffusion?
Baring the above open questions, we focused in this review on recent updates since our last systematic review article in 2014 (Kaldenhoff et al., 2014). We began with discussing the classical theory of CO2 solubility-diffusion of CO2 transport across lipid bilayers and the physiological influence of altered aquaporin expression. We then delved into the molecular details of aquaporin-mediated CO2 transport, as well as the role of sterols and nonrelevant membrane proteins on the overall CO2 permeability of biological membranes. Finally, we summarized the current limitations of the different methods used to measure CO2 permeability and offered a perspective on the current understanding of CO2 diffusion across biological membranes.
Meyer overton’s rule and the CO2 solubility-diffusion model
As the basic principle for mass transportation through diffusive means, Adolf Fick described that the diffusive flux (J) was related to the diffusion coefficient (D) and gradient of the substrate (in case of two phases, rewritten as the concentration difference between the two phases
Later, Meyer and Overton established the rule of spontaneous permeation of solutes and solvents across membranes, stating that the flux of the substance across a membrane, J, was linearly dependent on the permeability of the membrane, Pm, with a concentration difference
Eq. 1 can be rewritten as following:
Where
As indicated by the rule, the permeability of a given molecule is related to the partition coefficient
Physiological roles for aquaporin-mediated CO2 membrane diffusion
CO2 and O2 are gas molecules that play crucial roles in respiration by providing energy through oxidative phosphorylation reactions. Both gases need to be exchanged efficiently between the cellular organelles and the atmosphere, guided by their osmotic gradients. Unlike animals, plant cells or other photosynthesis microorganisms take up CO2 as a substrate for photosynthesis, and the concentration gradient is less significant compared to animals (Uehlein et al., 2017). Therefore, a higher efficient diffusion of CO2 from the atmosphere to the chloroplast stroma, where photosynthesis occurred, would be more beneficial for photosynthesis-active organisms (Kaldenhoff et al., 2014).
For quite a long time, the resistance of the mesophyll to CO2 was overlooked for green-leaf plants. Instead, the regulation of stroma and CO2 interconversion to bicarbonate and protons catalyzed by carbonic anhydrases (CA) was considered to be the limiting factor in CO2 availability (Kaldenhoff, 2012). However, even with complete deletion of CA activity in the chloroplast stroma, photosynthesis decreased by only about 7% (Price et al., 1994; Kaldenhoff, 2012). Furthermore, the mesophyll CO2 conductance varied rapidly in response to temperature, light, or water stress, instead of having a relatively constant value. This contradicted the pure physical model of mesophyll CO2 diffusion. Together, these shreds of evidence pointed to the existence of other major factors that regulated CO2 diffusion, such as aquaporin-mediated transportation.
The physiological influence of altered expression of potential permeable CO2 aquaporins was recently systematically evaluated and reviewed by Evans‘ group (Groszmann et al., 2017). To understand the role of certain putative permeable aquaporins with CO2, transgenic plants were generated and the impact on parameters relevant to photosynthesis was determined. In general, the change of mesophyII conductance was correlated with the tuned expression level of corresponding aquaporins. However, the mesophyII drawdown should be negatively correlated with the mesophyII conductance and the CO2 assimilation rate, where causal links between AQP and mesophyII conductance can be established. To provide the general ranges of photosynthetic-related parameters under varied mesophyII conductance, they performed simulations by changing the mesophyII conductance, when either stomatal conductance or Ci was set to be constant. They gave an estimated range of mesophyII drawdown, CO2 assimilation rates, transpiration rate, and transpiration efficiency based on consistent literature data (Groszmann et al., 2017). Furthermore, mesophyII conductance is a combined feature that could be influenced by many factors other than membrane permeability, such as the chloroplast surface area, adjacent to the intercellular air space per unit of leaf area and cell wall thickness (Evans, 2021). Since 2014, more direct or indirect evidence accumulated, supporting the aquaporin-facilitated CO2 transportation, i.e., AtPIP2;5, SlPIP1;2 (from tomato), OsPIP1;2 and OsPIP1;3 (from rice), HvPIP2;1, 2;2, 2;3, 2;5 (from Barley), ZmPIP1;5, 1;6 (from Maize), as well as SiPIP2;7 from C4 plant-foxtail millet (see Table 1).
Recent studies have shown that the influence of altered expression of potential CO2 permeable aquaporins on the mesophyII conductance and photosynthesis rate should be calibrated by growth and environmental conditions, as well as the oligomeric/phosphorylation status of the corresponding aquaporins. Although there are accumulated evidences for aquaporin-mediated CO2 transportation, there have also been studies that have shown that simple manipulation of these aquaporins did not lead to changes in mesophyII conductance or photosynthetic efficiency. In one study, the knockout of three aquaporin genes-AtPIP1;2, AtPIP1;3, AtPIP2;6 from Arabidopsis thaliana did not result in changes in mesophyII conductance nor photosynthetic efficiency. The authors discussed possible reasons for these results: i) functional redundancy within aquaporin families; ii) the possible change in hydraulic conductance together with the higher light intensities (200 μmol m−2 s−1) altered the photosynthetic capacity, which would be sufficient to remove the effect on both gm and gs; iii) altered the hydraulic conductance of mutant lines through functional stimulation by colocalization of PIP1s and PIP2s on the plasma membrane (Kromdijk et al., 2020). However, the hydraulic conductance of mutant lines was not measured in the above study, which left this question to be further investigated. In another case, the ectopic expression of either AtPIP1;2 or AtPIP1;4 in tobacco did not further increase mesophyII conductance nor the rate of assimilation of CO2. Similarly, the authors pointed out the influence of plant growth and environmental conditions on the ability of certain CO2 permeable aquaporins to alter mesophyII conductance, particularly, when a high basal gm was observed in control wild-type control plants (Clarke et al., 2022). This effect was also observed from rice PIPs (Huang et al., 2021) and tomato SiPIP1;2 knockout mutants (Kelly et al., 2014), where gm was affected only when grown in a CO2 enriched environment. Other studies have pointed out that the oligomeric or phosphorylation state of overexpressed CO2 permeable aquaporins can directly impact their function (Otto et al., 2010; Maurel et al., 2015; Groszmann et al., 2017). Additionally, aquaporins can act as signaling molecules, responding to different environmental stimuli and regulating stomatal dynamics in response to changes in ambient CO2 concentration (Ding and Chaumont, 2020) or ABA-mediated biotic stress (Fang et al., 2019). Finally, one important aspect to consider is the relative humidity within the substomatal cavity, which was assumed to be saturated when calculating the intercellular CO2 concentration determined by the gas exchange experiment (Cernusak et al., 2018). As recently investigated by Farquhar’s group, the relative humidity within the substomatal cavity could drop down to around 80%, with the saturation edge retreating to the mesophyII cell walls. Surprisingly, the mesophyII conductance to CO2 remained less affected when alter the Δw (the difference between saturated humidity and the humidity in the air) if compared to uncorrected data, which might be controlled by the aquaporins within the mesophyII cell membranes (Wong et al., 2022). Although there are several aquaporins reported to function as both water and CO2 channels, the detailed mechanism of such potential dual functions still needs to be investigated, which could be investigated with new methods such as in situ measurement of water potentials within leaves using the fluorescent powder-hydrogel nanoreporters (Jain et al., 2021), as well as cell specific overexpress experiment to avoid functional redundancy from endogenous aquaporins using plant leave single cell RNA sequence data base (Kim et al., 2021).
To conclude, the impact of changes in CO2 permeable aquaporins on mesophyll conductance and photosynthesis rate should be considered, with respect to growth and environmental conditions, in particular the relative humidity within the substomatal cavity, as well as the oligomeric and phosphorylation status of the corresponding aquaporins.
Molecular mechanism of aquaporin-mediated CO2 diffusion
Since the discovery of the CO2 channel protein: aquaporin-1 from humans and NtAQP1 from tobacco, many aquaporins from different organisms were reported to mediate CO2 transport, covering many members from mammals, plants, microalgae, and fish (see Table 1). The family of aquaporins has a relatively conserved structure, with six membrane-spanning helices, two reentrant short helices with NPA motifs, and flexible N-/C-termini heading towards the cytosol. The six bundle-like membrane-spanning alpha helices were tightly arranged in a circle, constituting the solute conduction pore/channel. Although aquaporins function as the water channel in monomers, they often form a quaternary tetramer assembly in native membranes and even large orthogonal arrays in the case of AQP4 (Ho et al., 2009). Until now, the physiological relevance of such a tetrameric assembly is not completely clear; however, a few cases showed that the central pore formed by the aquaporin tetramer was likely to be the CO2 channel. Early work based on X. laevis oocytes with low intrinsic CO2 permeabilities provided experimental evidence that AQP1 acts as a permeable CO2 channel (Nakhoul et al., 1998). Later, a molecular simulation based on the high-resolution structure of AQP1 gave the atomic level of details that the central pore of the AQP1 tetramer could mediate fast CO2 diffusion in low intrinsic CO2-permeable membranes (Hub and de Groot, 2006). This hypothesis was further demonstrated by the yeast protoplast system to determine the altered permeability of CO2 when the assembly of the artificial tetramer with a fixed ratio of NtPIP1;2 and NtPIP2;1, connected by a short linker (Otto et al., 2010). The results demonstrated that the homo-tetrameric assembly of CO2 permeable NtPIP1;2 was necessary for CO2 channel activity. However, such a relationship between the oligomeric state and CO2 permeability was not further investigated in other model plants, except for tobacco.
In 2021, Tyerman et al. gave a systematic review on multifunctional aquaporins, describing the dynamic regulation of the central pore with the high-resolution crystal structures of AQP1 and SoPIP2;1 (Tyerman et al., 2021). Furthermore, the MOLEonline MOLEonline channel radii analysis (Berka et al., 2012) showed a diameter of 3.6 Å at the Leu200 constriction residue, modelled by the closed water channel conformation of SoPIP2;1 (PDB: 1Z98) (Tornroth-Horsefield et al., 2006). According to the analysis, the constriction side would allow the CO2 to pass when considering a kinetic diameter of 3.3 Å for CO2. Additionally, Tyerman et al. (2021) proposed that both post-translational modification and protein-protein interactions could contribute to dynamic regulation of central pore permeability via local conformational changes, allowing a wide range of molecules, including K+, Na+, as well as CO2 passing through the central pore. In early studies, aquaporins from the PIP1 family were found to be permeable to CO2. While, later on, members from the PIP2 family were also reported to function as CO2 channels. Despite the relative conservation of transmembrane helices in all PIP aquaporins, it was difficult to identify the crucial residues creating the selective filter of the central pore, which was not surprising given the very variable pore environment generated by tetrameric assembly. As seen in Figure1, the major part of the central pore lining area is composed of transmembrane helices 2 and 5 and loop D, which were dynamically influenced by other neighboring motifs as well. However, due to the lack of high-resolution structure of PIP1 aquaporin, it remains unresolved how the sequence difference between PIP1s and PIP2s could contribute to CO2 permeability, especially the long N-terminal flexible loop that only exists in PIP1s. Although it is difficult to obtain structural details for the flexible loop region, a detailed biochemical assay might answer this question, such as domain switch or truncated variants in the case of an N-terminal loop. As indicated in Figure 1., conserved residues Leu in helix 5 and Ile at the end of loop E were reported to be essential to allow the passage of a CO2 molecule based on either simulation or biochemical assays (Mori et al., 2014; Tyerman et al., 2021). However, other residues alone the channel might also be the restriction site, depending on the arrangement of the helixes structures that form the central channel.
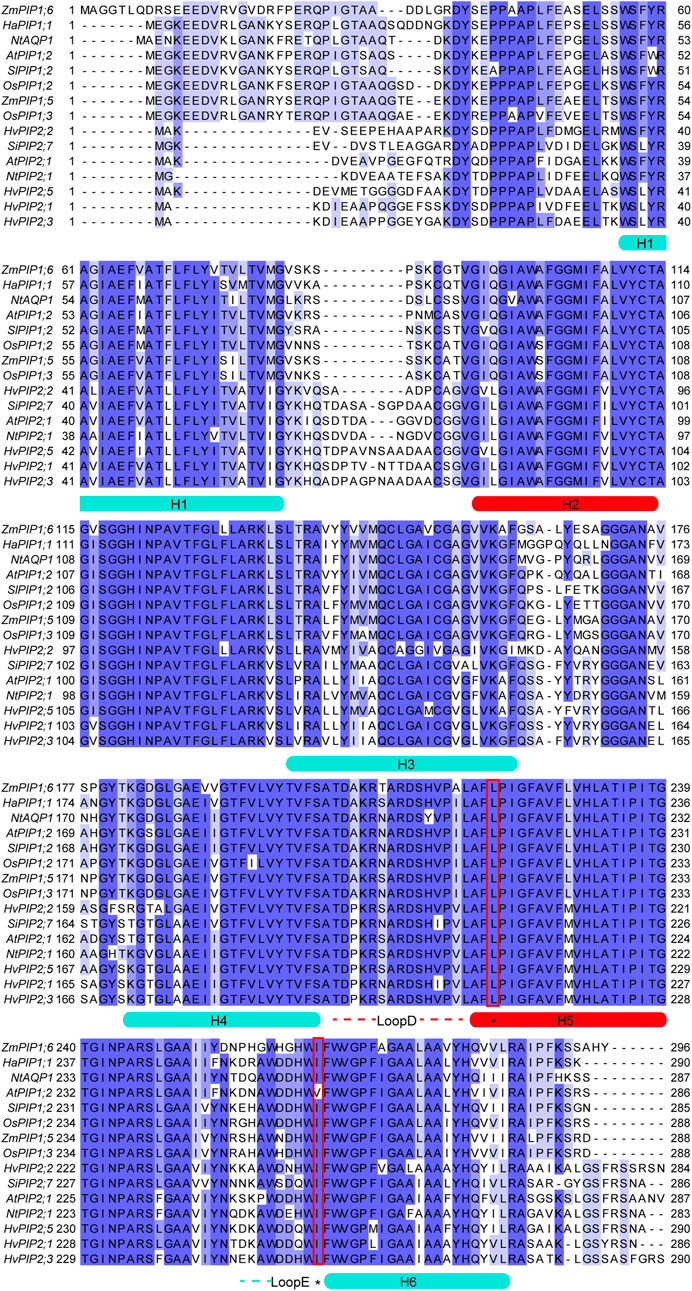
FIGURE 1. Sequence alignment of CO2 permeable aquaporins from plants. Alignment was performed by the online tool Claustral Omega (https://www.ebi.ac.uk/Tools/msa/clustalo/) using default settings (Madeira et al., 2022). Blue shades indicated the percentage of identity. Helical regions were highlighted and denoted H1-H6. The central pore lining region were highlighted in red, including H2, H5 and LoopD. The key residues for CO2 permeability were highlighted with red rectangles.
Role of sterols and non-CO2 permeable proteins and technical challenges in measuring CO2 permeability
The Singer-Nicolson fluid-mosaic model was widely recognized as the fundamental model for the structure and molecular dynamics of the plasma membrane (Singer and Nicolson, 1972). Many basic properties of biological membranes were characterized on the basis of this two-dimensional fluid model. Among the basic properties, the permeability was also intensively investigated using such a lipid bilayer model both theoretically and experimentally. However, other factors, such as sterols or integrated membrane proteins were not considered, which could influence the overall permeability (Suzuki and Kusumi, 2023). Therefore, lack of such factors could be the potential source for the inconsistency of measured membrane permeability. This inconsistency became non-trivial when determining CO2 permeability. Due to the higher lipophilic properties of CO2, the phospholipid-formed lipid bilayer exhibits very low resistance to CO2, while the plasma membrane of X. laevis oocytes, Madin-Darby canine kidney (MDCK) cells, the transformed human embryonal kidney SV40 cell line (tsA201), as well as the apical membrane of the gastric glands, showed extremely low CO2 permeability (Endeward et al., 2006a; Endeward et al., 2006b; Endeward et al., 2008; Itel et al., 2012). In a recent review, Gros et al. proposed that the cholesterol content in the majority biological membranes dominates its CO2 permeability, regulating the CO2 permeability by at least 2 orders of magnitude with a cholesterol content between 0%–70% (Arias-Hidalgo et al., 2018). However, an exception of normal native human red cells showed aquaporin-dependent CO2 permeability instead of cholesterol content, indicating the existence of unidentified factors (Endeward et al., 2008). Kaldenhoff’s group suggested a possibility, pointing out the role of non-channel proteins on the CO2 permeability of the phospholipid bilayer (Kai and Kaldenhoff, 2014). Finally, the existence of lipid rafts, which are rich in both sterols and proteins, could further contribute to the overall permeability [see review by Kai Simons and Elina Ikonen (Simons and Ikonen, 1997)].
One possible reason for the inconsistent permeability of CO2 reported in many previous reports could be the limitations of different techniques in determining permeability of CO2, due to the high permeability of the phospholipid bilayer (Endeward et al., 2014). Both stop flow-based and mass spectrometry-based methods were questioned for their inability to quantify dynamic fast CO2 across the membrane (Boron et al., 2011; Hannesschlaeger et al., 2019). On the other hand, the scanning pH electrode could provide an alternative that was not limited by the fast dynamics of CO2 passing through the black lipid membrane. However, the formation of a black lipid membrane with the solvent-containing method was challenged by the presence of organic solvent n-decan, as well as whether aquaporins still survive as a functional form during the formation of the corresponding black lipid membrane (Hannesschlaeger et al., 2019). Therefore, new techniques that can determine the fast transportation of CO2 across the membrane and avoid the influence of solvents may be necessary to improve the accuracy of the CO2 permeability measurements.
Conclusion
Despite the numerous structural and functional studies of aquaporins in the past several decades, our understanding of the detailed mechanism of functional and structural diversity of these relatively conserved channel proteins is still in its infancy. The debate over whether aquaporins are permeable to CO2 continues, with accumulating both supportive and contradictory evidence. However, the challenges in directly measuring CO2 permeability across native or artificial membranes make it difficult to fully interpret the results and understand their physiological implications. More attention should be paid to the interpretation of the data and investigating the potential effects of aquaporin overexpression on plant cultivars and photosynthesis-related parameters. Ultimately, a technical breakthrough for the direct measurement of CO2 transportation through aquaporins would be needed to fully clarify the molecular details and bring an end to the ongoing debate.
Author contributions
All authors listed have made a substantial, direct, and intellectual contribution to the work and approved it for publication.
Funding
LK is grateful for the support of the Jiangsu Higher Education Institutions Natural Science Foundation (Grant No. 17KJB180003), the Jiangsu Normal University Natural Science Foundation (Grant No. 17XLR037), and the Jiangsu Specially Appointed Professor program. LS acknowledges funding from Postgraduate Research and Practice Innovation Program of Jiangsu Province (Grant No. KYCX21_2576).
Conflicts of interest
Author KH is employed by Jiangsu Keybio Co. Ltd.
The remaining authors declare that the research was conducted in the absence of any commercial or financial relationships that could be construed as a potential conflict of interest.
Publisher’s note
All claims expressed in this article are solely those of the authors and do not necessarily represent those of their affiliated organizations, or those of the publisher, the editors and the reviewers. Any product that may be evaluated in this article, or claim that may be made by its manufacturer, is not guaranteed or endorsed by the publisher.
References
Agre, P. (2004). Aquaporin water channels (nobel lecture). Angew. Chem. Int. Ed. Engl. 43 (33), 4278–4290. doi:10.1002/anie.200460804
Almasalmeh, A., Krenc, D., Wu, B., and Beitz, E. (2014). Structural determinants of the hydrogen peroxide permeability of aquaporins. Febs. J. 281 (3), 647–656. doi:10.1111/febs.12653
Arias-Hidalgo, M., Al-Samir, S., Gros, G., and Endeward, V. (2018). Cholesterol is the main regulator of the carbon dioxide permeability of biological membranes. Am. J. Physiol. Cell Physiol. 315 (2), C137–C140. doi:10.1152/ajpcell.00139.2018
Berka, K., Hanak, O., Sehnal, D., Banas, P., Navratilova, V., Jaiswal, D., et al. (2012). MOLEonline 2.0: Interactive web-based analysis of biomacromolecular channels. Nucleic Acids Res. 40 (Web Server issue), W222–W227. doi:10.1093/nar/gks363
Boron, W. F., Endeward, V., Gros, G., Musa-Aziz, R., and Pohl, P. (2011). Intrinsic CO2 permeability of cell membranes and potential biological relevance of CO2 channels. Chemphyschem 12 (5), 1017–1019. doi:10.1002/cphc.201100034
Cernusak, L. A., Ubierna, N., Jenkins, M. W., Garrity, S. R., Rahn, T., Powers, H. H., et al. (2018). Unsaturation of vapour pressure inside leaves of two conifer species. Sci. Rep. 8 (1), 7667. doi:10.1038/s41598-018-25838-2
Chen, X., Ma, J., Wang, X., Lu, K., Liu, Y., Zhang, L., et al. (2021). Functional modulation of an aquaporin to intensify photosynthesis and abrogate bacterial virulence in rice. Plant J. 108 (2), 330–346. doi:10.1111/tpj.15427
Clarke, V. C., De Rosa, A., Massey, B., George, A. M., Evans, J. R., von Caemmerer, S., et al. (2022). Mesophyll conductance is unaffected by expression of Arabidopsis PIP1 aquaporins in the plasmalemma of Nicotiana. J. Exp. Bot. 73 (11), 3625–3636. doi:10.1093/jxb/erac065
Cooper, G. J., Occhipinti, R., and Boron, W. F. (2015). CrossTalk proposal: Physiological CO2 exchange can depend on membrane channels. J. Physiol. 593 (23), 5025–5028. doi:10.1113/JP270059
Ding, L., and Chaumont, F. (2020). Are aquaporins expressed in stomatal complexes promising targets to enhance stomatal dynamics? Front. Plant. Sci. 11, 458. doi:10.3389/fpls.2020.00458
Ding, X., Matsumoto, T., Gena, P., Liu, C., Pellegrini-Calace, M., Zhong, S., et al. (2013). Water and CO2 permeability of SsAqpZ, the cyanobacterium Synechococcus sp. PCC7942 aquaporin. Biol. Cell 105 (3), 118–128. doi:10.1111/boc.201200057
Edidin, M. (2003). Lipids on the frontier: A century of cell-membrane bilayers. Nat. Rev. Mol. Cell Biol. 4 (5), 414–418. doi:10.1038/nrm1102
Endeward, V., Cartron, J. P., Ripoche, P., and Gros, G. (2006a). Red cell membrane CO2 permeability in normal human blood and in blood deficient in various blood groups, and effect of DIDS. Transfus. Clin. Biol. 13 (1-2), 123–127. doi:10.1016/j.tracli.2006.02.007
Endeward, V., Musa-Aziz, R., Cooper, G. J., Chen, L. M., Pelletier, M. F., Virkki, L. V., et al. (2006b). Evidence that aquaporin 1 is a major pathway for CO2 transport across the human erythrocyte membrane. FASEB J. 20 (12), 1974–1981. doi:10.1096/fj.04-3300com
Endeward, V., Cartron, J. P., Ripoche, P., and Gros, G. (2008). RhAG protein of the Rhesus complex is a CO2 channel in the human red cell membrane. FASEB J. 22 (1), 64–73. doi:10.1096/fj.07-9097com
Endeward, V., Al-Samir, S., Itel, F., and Gros, G. (2014). How does carbon dioxide permeate cell membranes? A discussion of concepts, results and methods. Front. Physiol. 4, 382. doi:10.3389/fphys.2013.00382
Ermakova, M., Osborn, H., Groszmann, M., Bala, S., Bowerman, A., McGaughey, S., et al. (2021). Expression of a CO2-permeable aquaporin enhances mesophyll conductance in the C4 species Setaria viridis. Elife 10, e70095. doi:10.7554/eLife.70095
Evans, J. R. (2021). Mesophyll conductance: Walls, membranes and spatial complexity. New Phytol. 229 (4), 1864–1876. doi:10.1111/nph.16968
Fang, L., Abdelhakim, L. O. A., Hegelund, J. N., Li, S., Liu, J., Peng, X., et al. (2019). ABA-mediated regulation of leaf and root hydraulic conductance in tomato grown at elevated CO2 is associated with altered gene expression of aquaporins. Hortic. Res. 6, 104. doi:10.1038/s41438-019-0187-6
Geyer, R. R., Musa-Aziz, R., Qin, X., and Boron, W. F. (2013). Relative CO2/NH3 selectivities of mammalian aquaporins 0-9. Am. J. Physiol. Cell Physiol. 304 (10), C985–C994. doi:10.1152/ajpcell.00033.2013
Groszmann, M., Osborn, H. L., and Evans, J. R. (2017). Carbon dioxide and water transport through plant aquaporins. Plant Cell Environ. 40 (6), 938–961. doi:10.1111/pce.12844
Hannesschlaeger, C., Horner, A., and Pohl, P. (2019). Intrinsic membrane permeability to small molecules. Chem. Rev. 119 (9), 5922–5953. doi:10.1021/acs.chemrev.8b00560
Heckwolf, M., Pater, D., Hanson, D. T., and Kaldenhoff, R. (2011). The Arabidopsis thaliana aquaporin AtPIP1;2 is a physiologically relevant CO2 transport facilitator. Plant J. 67 (5), 795–804. doi:10.1111/j.1365-313X.2011.04634.x
Heinen, R. B., Bienert, G. P., Cohen, D., Chevalier, A. S., Uehlein, N., Hachez, C., et al. (2014). Expression and characterization of plasma membrane aquaporins in stomatal complexes of Zea mays. Plant Mol. Biol. 86 (3), 335–350. doi:10.1007/s11103-014-0232-7
Ho, J. D., Yeh, R., Sandstrom, A., Chorny, I., Harries, W. E., Robbins, R. A., et al. (2009). Crystal structure of human aquaporin 4 at 1.8 Å and its mechanism of conductance. Proc. Natl. Acad. Sci. U. S. A. 106 (18), 7437–7442. doi:10.1073/pnas.0902725106
Huang, X., Wang, Z., Huang, J., Peng, S., and Xiong, D. (2021). Mesophyll conductance variability of rice aquaporin knockout lines at different growth stages and growing environments. Plant J. 107 (5), 1503–1512. doi:10.1111/tpj.15397
Hub, J. S., and de Groot, B. L. (2006). Does CO2 permeate through aquaporin-1? Biophys. J. 91 (3), 842–848. doi:10.1529/biophysj.106.081406
Ishibashi, K., Sasaki, S., Fushimi, K., Uchida, S., Kuwahara, M., Saito, H., et al. (1994). Molecular cloning and expression of a member of the aquaporin family with permeability to glycerol and urea in addition to water expressed at the basolateral membrane of kidney collecting duct cells. Proc. Natl. Acad. Sci. U. S. A. 91 (14), 6269–6273. doi:10.1073/pnas.91.14.6269
Israel, D., Khan, S., Warren, C. R., Zwiazek, J. J., and Robson, T. M. (2021). The contribution of PIP2-type aquaporins to photosynthetic response to increased vapour pressure deficit. J. Exp. Bot. 72 (13), 5066–5078. doi:10.1093/jxb/erab187
Itel, F., Al-Samir, S., Oberg, F., Chami, M., Kumar, M., Supuran, C. T., et al. (2012). CO2 permeability of cell membranes is regulated by membrane cholesterol and protein gas channels. FASEB J. 26 (12), 5182–5191. doi:10.1096/fj.12-209916
Jahn, T. P., Moller, A. L., Zeuthen, T., Holm, L. M., Klaerke, D. A., Mohsin, B., et al. (2004). Aquaporin homologues in plants and mammals transport ammonia. FEBS Lett. 574 (1-3), 31–36. doi:10.1016/j.febslet.2004.08.004
Jain, P., Liu, W., Zhu, S., Chang, C. Y., Melkonian, J., Rockwell, F. E., et al. (2021). A minimally disruptive method for measuring water potential in planta using hydrogel nanoreporters. Proc. Natl. Acad. Sci. U. S. A. 118 (23), e2008276118. doi:10.1073/pnas.2008276118
Jensen, M. O., Tajkhorshid, E., and Schulten, K. (2001). The mechanism of glycerol conduction in aquaglyceroporins. Structure 9 (11), 1083–1093. doi:10.1016/s0969-2126(01)00668-2
Kai, L., and Kaldenhoff, R. (2014). A refined model of water and CO2 membrane diffusion: Effects and contribution of sterols and proteins. Sci. Rep. 4, 6665. doi:10.1038/srep06665
Kaldenhoff, R., Kai, L., and Uehlein, N. (2014). Aquaporins and membrane diffusion of CO2 in living organisms. Biochim. Biophys. Acta. 1840 (5), 1592–1595. doi:10.1016/j.bbagen.2013.09.037
Kaldenhoff, R. (2012). Mechanisms underlying CO2 diffusion in leaves. Curr. Opin. Plant Biol. 15 (3), 276–281. doi:10.1016/j.pbi.2012.01.011
Kelly, G., Sade, N., Attia, Z., Secchi, F., Zwieniecki, M., Holbrook, N. M., et al. (2014). Relationship between hexokinase and the aquaporin PIP1 in the regulation of photosynthesis and plant growth. PLoS One 9 (2), e87888. doi:10.1371/journal.pone.0087888
Kim, J. Y., Symeonidi, E., Pang, T. Y., Denyer, T., Weidauer, D., Bezrutczyk, M., et al. (2021). Distinct identities of leaf phloem cells revealed by single cell transcriptomics. Plant Cell 33 (3), 511–530. doi:10.1093/plcell/koaa060
Kirscht, A., Kaptan, S. S., Bienert, G. P., Chaumont, F., Nissen, P., de Groot, B. L., et al. (2016). Crystal structure of an ammonia-permeable aquaporin. PLoS Biol. 14 (3), e1002411. doi:10.1371/journal.pbio.1002411
Kromdijk, J., Glowacka, K., and Long, S. P. (2020). Photosynthetic efficiency and mesophyll conductance are unaffected in Arabidopsis thaliana aquaporin knock-out lines. J. Exp. Bot. 71 (1), 318–329. doi:10.1093/jxb/erz442
Ma, T., Yang, B., and Verkman, A. S. (1997). Cloning of a novel water and urea-permeable aquaporin from mouse expressed strongly in colon, placenta, liver, and heart. Biochem. Biophys. Res. Commun. 240 (2), 324–328. doi:10.1006/bbrc.1997.7664
Madeira, F., Pearce, M., Tivey, A. R. N., Basutkar, P., Lee, J., Edbali, O., et al. (2022). Search and sequence analysis tools services from EMBL-EBI in 2022. Nucleic Acids Res. 50 (W1), W276–W279. doi:10.1093/nar/gkac240
Matsui, H., Hopkinson, B. M., Nakajima, K., and Matsuda, Y. (2018). Plasma membrane-type aquaporins from marine diatoms function as CO2/NH3 channels and provide photoprotection. Plant Physiol. 178 (1), 345–357. doi:10.1104/pp.18.00453
Maurel, C., Boursiac, Y., Luu, D. T., Santoni, V., Shahzad, Z., and Verdoucq, L. (2015). Aquaporins in plants. Physiol. Rev. 95 (4), 1321–1358. doi:10.1152/physrev.00008.2015
Missner, A., and Pohl, P. (2009). 110 years of the meyer-overton rule: Predicting membrane permeability of gases and other small compounds. Chemphyschem 10 (9-10), 1405–1414. doi:10.1002/cphc.200900270
Missner, A., Kugler, P., Antonenko, Y. N., and Pohl, P. (2008a). Passive transport across bilayer lipid membranes: Overton continues to rule. Proc. Natl. Acad. Sci. U. S. A. 105 (52), E123; author reply E124. doi:10.1073/pnas.0809606106
Missner, A., Kugler, P., Saparov, S. M., Sommer, K., Mathai, J. C., Zeidel, M. L., et al. (2008b). Carbon dioxide transport through membranes. J. Biol. Chem. 283 (37), 25340–25347. doi:10.1074/jbc.M800096200
Mori, I. C., Rhee, J., Shibasaka, M., Sasano, S., Kaneko, T., Horie, T., et al. (2014). CO2 transport by PIP2 aquaporins of barley. Plant Cell Physiol. 55 (2), 251–257. doi:10.1093/pcp/pcu003
Musa-Aziz, R., Chen, L. M., Pelletier, M. F., and Boron, W. F. (2009). Relative CO2/NH3 selectivities of AQP1, AQP4, AQP5, AmtB, and RhAG. Proc. Natl. Acad. Sci. U. S. A. 106 (13), 5406–5411. doi:10.1073/pnas.0813231106
Nakhoul, N. L., Davis, B. A., Romero, M. F., and Boron, W. F. (1998). Effect of expressing the water channel aquaporin-1 on the CO2 permeability of Xenopus oocytes. Am. J. Physiol. 274 (2), C543–C548. doi:10.1152/ajpcell.1998.274.2.C543
Navarro-Rodenas, A., Barzana, G., Nicolas, E., Carra, A., Schubert, A., and Morte, A. (2013). Expression analysis of aquaporins from desert truffle mycorrhizal symbiosis reveals a fine-tuned regulation under drought. Mol. Plant Microbe. Interact. 26 (9), 1068–1078. doi:10.1094/MPMI-07-12-0178-R
Nollert, P., Harries, W. E., Fu, D., Miercke, L. J., and Stroud, R. M. (2001). Atomic structure of a glycerol channel and implications for substrate permeation in aqua(glycero)porins. FEBS Lett. 504 (3), 112–117. doi:10.1016/s0014-5793(01)02710-7
Otto, B., Uehlein, N., Sdorra, S., Fischer, M., Ayaz, M., Belastegui-Macadam, X., et al. (2010). Aquaporin tetramer composition modifies the function of tobacco aquaporins. J. Biol. Chem. 285 (41), 31253–31260. doi:10.1074/jbc.M110.115881
Prasad, G. V., Coury, L. A., Finn, F., and Zeidel, M. L. (1998). Reconstituted aquaporin 1 water channels transport CO2 across membranes. J. Biol. Chem. 273 (50), 33123–33126. doi:10.1074/jbc.273.50.33123
Preston, G. M., Carroll, T. P., Guggino, W. B., and Agre, P. (1992). Appearance of water channels in Xenopus oocytes expressing red cell CHIP28 protein. Science 256 (5055), 385–387. doi:10.1126/science.256.5055.385
Price, G. D., von Caemmerer, S., Evans, J. R., Yu, J.-W., Lloyd, J., Oja, V., et al. (1994). Specific reduction of chloroplast carbonic anhydrase activity by antisense RNA in transgenic tobacco plants has a minor effect on photosynthetic CO2 assimilation. Planta 193, 331–340. doi:10.1007/BF00201810
Simons, K., and Ikonen, E. (1997). Functional rafts in cell membranes. Nature 387 (6633), 569–572. doi:10.1038/42408
Singer, S. J., and Nicolson, G. L. (1972). The fluid mosaic model of the structure of cell membranes. Science 175 (4023), 720–731. doi:10.1126/science.175.4023.720
Suzuki, K. G. N., and Kusumi, A. (2023). Refinement of Singer-Nicolson fluid-mosaic model by microscopy imaging: Lipid rafts and actin-induced membrane compartmentalization. Biochim. Biophys. Acta. Biomembr. 1865 (2), 184093. doi:10.1016/j.bbamem.2022.184093
Talbot, K., Kwong, R. W., Gilmour, K. M., and Perry, S. F. (2015). The water channel aquaporin-1a1 facilitates movement of CO2 and ammonia in zebrafish (Danio rerio) larvae. J. Exp. Biol. 218 (Pt 24), 3931–3940. doi:10.1242/jeb.129759
Tornroth-Horsefield, S., Wang, Y., Hedfalk, K., Johanson, U., Karlsson, M., Tajkhorshid, E., et al. (2006). Structural mechanism of plant aquaporin gating. Nature 439 (7077), 688–694. doi:10.1038/nature04316
Tyerman, S. D., McGaughey, S. A., Qiu, J., Yool, A. J., and Byrt, C. S. (2021). Adaptable and multifunctional ion-conducting aquaporins. Annu. Rev. Plant Biol. 72, 703–736. doi:10.1146/annurev-arplant-081720-013608
Uehlein, N., Lovisolo, C., Siefritz, F., and Kaldenhoff, R. (2003). The tobacco aquaporin NtAQP1 is a membrane CO2 pore with physiological functions. Nature 425 (6959), 734–737. doi:10.1038/nature02027
Uehlein, N., Otto, B., Eilingsfeld, A., Itel, F., Meier, W., and Kaldenhoff, R. (2012a). Gas-tight triblock-copolymer membranes are converted to CO2 permeable by insertion of plant aquaporins. Sci. Rep. 2, 538. doi:10.1038/srep00538
Uehlein, N., Sperling, H., Heckwolf, M., and Kaldenhoff, R. (2012b). The Arabidopsis aquaporin PIP1;2 rules cellular CO2 uptake. Plant Cell Environ. 35 (6), 1077–1083. doi:10.1111/j.1365-3040.2011.02473.x
Uehlein, N., Kai, L., and Kaldenhoff, R. (2017). “Plant aquaporins and CO2,” in Plant aquaporins: From transport to signaling (cham, Germany, 255–265. doi:10.1007/978-3-319-49395-4_12
Wang, D., Boron, W. F., Yan, C., Allcock, N. S., Craigon, J., Blanco-Ulate, B., et al. (2019). Effect of aquaporin 5 and carbonic anhydrase II on the diffusion of CO2 in Xenopus oocytes. FASEB J. 33 (S1), 544–557. doi:10.1104/pp.18.01187
Wang, C., Hu, H., Qin, X., Zeise, B., Xu, D., Rappel, W. J., et al. (2016). Reconstitution of CO2 regulation of SLAC1 anion channel and function of CO2-permeable PIP2;1 aquaporin as CARBONIC ANHYDRASE4 interactor. Plant Cell 28 (2), 568–582. doi:10.1105/tpc.15.00637
Wong, S. C., Canny, M. J., Holloway-Phillips, M., Stuart-Williams, H., Cernusak, L. A., Márquez, D. A., et al. (2022). Humidity gradients in the air spaces of leaves. Nat. Plants 8 (8), 971–978. doi:10.1038/s41477-022-01202-1
Xu, F., Wang, K., Yuan, W., Xu, W., Shuang, L., Kronzucker, H. J., et al. (2019). Overexpression of rice aquaporin OsPIP1;2 improves yield by enhancing mesophyll CO2 conductance and phloem sucrose transport. J. Exp. Bot. 70 (2), 671–681. doi:10.1093/jxb/ery386
Zhang, D., Li, Y., and Li, Y. (2021). The potential implications of a plasma membrane aquaporin in improving CO2 transport capacity, photosynthetic potential and water use efficiency under contrasting CO2 source in Solanum lycopersicum (tomato). Sci. Hortic. 283, 110122. doi:10.1016/j.scienta.2021.110122
Keywords: carbon dioxide, aquaporin, biological membranes, diffusion, physiological relevance
Citation: Chen J, Yue K, Shen L, Zheng C, Zhu Y, Han K and Kai L (2023) Aquaporins and CO2 diffusion across biological membrane. Front. Physiol. 14:1205290. doi: 10.3389/fphys.2023.1205290
Received: 18 April 2023; Accepted: 05 June 2023;
Published: 13 June 2023.
Edited by:
Wangbiao Seven Guo, Yale University, United StatesReviewed by:
Jiaen Qiu, University of Adelaide, AustraliaCopyright © 2023 Chen, Yue, Shen, Zheng, Zhu, Han and Kai. This is an open-access article distributed under the terms of the Creative Commons Attribution License (CC BY). The use, distribution or reproduction in other forums is permitted, provided the original author(s) and the copyright owner(s) are credited and that the original publication in this journal is cited, in accordance with accepted academic practice. No use, distribution or reproduction is permitted which does not comply with these terms.
*Correspondence: Lei Kai, bGthaUBqc251LmVkdS5jbg==
†These authors have contributed equally to this work and shared the first authorship