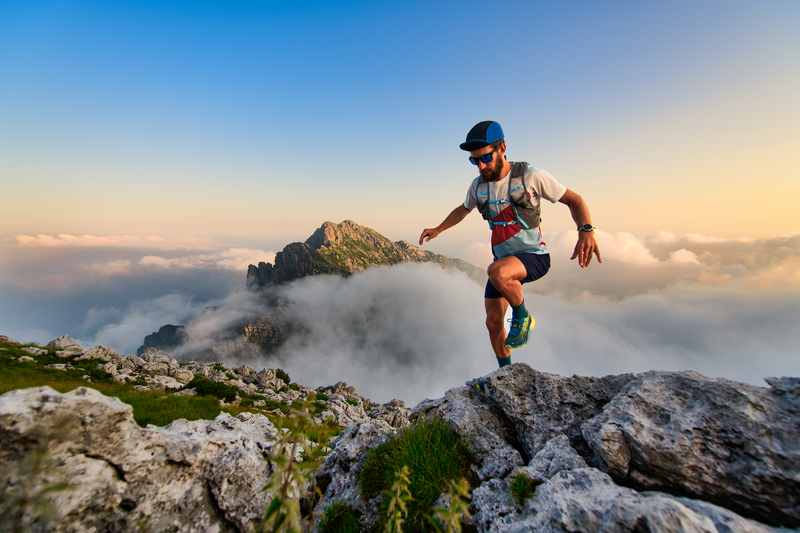
95% of researchers rate our articles as excellent or good
Learn more about the work of our research integrity team to safeguard the quality of each article we publish.
Find out more
SYSTEMATIC REVIEW article
Front. Physiol. , 07 June 2023
Sec. Vascular Physiology
Volume 14 - 2023 | https://doi.org/10.3389/fphys.2023.1204874
Background: Burst suppression (BS) is an electroencephalography (EEG) pattern in which there are isoelectric periods interspersed with bursts of cortical activity. Targeting BS through anaesthetic administration is used as a tool in the neuro-intensive care unit but its relationship with cerebral blood flow (CBF) and cerebral autoregulation (CA) is unclear. We performed a systematic scoping review investigating the effect of BS on CBF and CA in animals and humans.
Methods: We searched MEDLINE, BIOSIS, EMBASE, SCOPUS and Cochrane library from inception to August 2022. The data that were collected included study population, methods to induce and measure BS, and the effect on CBF and CA.
Results: Overall, there were 66 studies that were included in the final results, 41 of which examined animals, 24 of which examined humans, and 1 of which examined both. In almost all the studies, BS was induced using an anaesthetic. In most of the animal and human studies, BS was associated with a decrease in CBF and cerebral metabolism, even if the mean arterial pressure remained constant. The effect on CA during periods of stress (hypercapnia, hypothermia, etc.) was variable.
Discussion: BS is associated with a reduction in cerebral metabolic demand and CBF, which may explain its usefulness in patients with brain injury. More evidence is needed to elucidate the connection between BS and CA.
“Burst suppression” (BS) is an electroencephalography (EEG) phenomenon in which there is continuous alternation between epochs of suppressed activity, evidenced by “flattening” or slowing of the wave form, and high-voltage sharp waves, or “spikes” on the wave form (Amzica, 2009; Amzica, 2015; Shanker et al., 2021). One method of identifying BS is purely by visualization (see Figure 1). According to the American Clinical Neurophysiological Society, when an EEG shows 50%–99% suppression of the waveform, it can be termed BS (Ma and Bebawy, 2022). Another, more accurate method is through identification of the burst-suppression ratio (BSR). The BSR can be obtained by dividing the total duration of suppression by the total epoch time and then multiplying the result by 100; in other words, if a waveform was completely suppressed or “isoelectric,” the BSR would be 100% and if there was absolutely no suppression the BSR would be 0%. Yet another method is through power spectral analysis (PSA) in which frequency-domain signal analytic approaches are used to convert EEG signals in which amplitude is plotted against frequency. This method quantifies the amplitude of each frequency component and is a more objective measure of the EEG and BS (Liu et al., 2021). By performing PSA, spectrograms can be generated which can provide visually striking displays of deep sedation (Shanker et al., 2021).
FIGURE 1. Electroencephalographic burst suppression. The blue arrows indicate the spikes or “bursts” and the red arrows indicate the epochs of “suppression.” Taken and adapted with permission from Ng et al.,2015.
BS has been seen as a harbinger of severe neurological injury as in coma, cerebral anoxia, or infantile encephalopathy (Amzica, 2015; Shanker et al., 2021; Ma and Bebawy, 2022). In one retrospective review of 101 patients in a coma, BS with identical bursts/spikes was associated with poor outcome with a specificity and positive predictive value of 100% (Hofmeijer et al., 2014). However, interestingly, BS has also been used as a method of neuroprotection, usually using anaesthetic agents. It is hypothesized that BS leads to a state of cortical inactivation, decreasing cerebral metabolism and oxygen demand (CMRO2) and thereby can be protective during times of cerebral stress such as during cardiac surgery, cerebral aneurysm surgery, and carotid surgery (Ma and Bebawy, 2022).
BS may also be protective for patients with traumatic brain injury (TBI) (Frohlich et al., 2021). As cerebral perfusion pressure (CPP) is equal to the difference between the mean arterial pressure (MAP) and intracranial pressure (ICP), it is possible that BS can be neuroprotective through decreasing ICP in patients with brain injury (Mount and Das, 2022). In a systematic review, Zeiler et al., demonstrated Oxford level 2b and GRADE C evidence to support that BS reduces ICP but does not have any effect on ICP in severe TBI (Zeiler et al., 2017). Given that ICP reduction happened no matter which anaesthetic was used, the authors hypothesized that it was BS that caused the ICP reduction and not the anaesthetic itself. One possibility is that BS leads to the reduction of ICP by reducing cerebral blood flow (CBF). The normal CBF ranges from 45 to 50 mL/100 g/min, being higher in grey matter and lower in white matter (Tameem and Krovvidi, 2013). When cerebral blood vessels dilate (due to hypercapnia, drugs, or a variety of other triggers) the resulting CBF increases, increasing the amount of cerebral blood volume, and concurrently, the ICP.
CBF is tightly controlled through a variety of mechanisms (Tameem and Krovvidi, 2013). The term “cerebral autoregulation” (CA) applies to the capacity of the cerebral blood vessels to alter their resistance to maintain constant CBF in the face of a constantly changing MAP. When there are changes in MAP, there are changes in the smooth muscle of the cerebral vasculature through nitric oxide and endothelium-derived relaxing factors (Tameem and Krovvidi, 2013). Classically, autoregulation occurs between a MAP of 50 and 150 mmHg and outside of this range, CBF becomes significantly dependent on MAP and changes accordingly; this is known as the “Lassen Curve” (11). Different disease states, from hypertension to hydrocephalus can shift this curve, thereby increasing the risk of pressure-passive neural injury, even at a higher MAP (12). CBF is also highly coupled to neuronal activity, a process known as “flow-metabolism coupling”. An increase in neuronal activity leads to an increase in CMRO2 and cerebral metabolic rate for glucose (CMRglu) and there are proportional increases in CBF (Tameem and Krovvidi, 2013). CMRO2 can be calculated by multiplying the CBF and the difference in oxygen concentration in the cerebral veins and arteries (arteriovenous difference in oxygen). To measure autoregulation, it is essential to measure changes in CBF while keeping all other factors such as partial pressure of CO2 and cerebral metabolic rate (CMR) constant.
Many anaesthetics decrease CBF, but it remains unclear if it is the anaesthetic itself or the BS that causes a reduction in CBF; that is to say, is the depression of CMRO2 responsible for the decrease in CBF through flow-metabolism coupling or the direct effect of the anaesthetic on the cerebral vasculature? Further, it is unclear whether anaesthetics effect autoregulation in addition to the CBF. We sought to conduct a systematic scoping review on the effect that BS, induced primarily by anaesthesia, has on CBF and autoregulation in animals and humans.
Using the methodology outlined in the Cochrane Handbook for Systematic Reviews, we performed a systematically conducted scoping review of the available literature (Cochrane Handbook, 2023). Reporting was done in accordance with the Preferred Reporting Items for Systematic Reviews and Meta-Analysis (PRISMA) (Tricco et al., 2018). The methodology and search strategy followed are similar to previous systematic scoping reviews conducted by our group (Froese et al., 2020a; Sainbhi et al., 2021; Batson et al., 2022; Gomez et al., 2022). Review objectives and search strategy were conceptualized by the primary (AS, LF) and senior (FZ.) authors.
The question evaluated for this systematic review was as follows: What is the effect of BS on CBF and CA in animals and humans?
BS was defined as an EEG pattern characterized by alternating periods of isoelectricity and active oscillations or “spikes” (2). Note, for the present study, “isoelectricity” was considered synonymous with BS as they both indicate a state of profound cerebral inactivity. BS could be induced by anaesthesia or by brain injury.
The measures of CBF that were included in the search were the following: measuring blood flow in the superior sagittal sinus, Xenon133 clearance, MS techniques (radiolabelled, florescent, or coloured), laser Doppler flowmetry, thermal diffusion flowmetry, positron emission tomography (PET) imaging, perfusion weighted magnetic resonance imaging (PW-MRI), arterial spin labeling magnetic resonance imaging (ASL-MRI), functional magnetic resonance imaging (fMRI) and blood-oxygen-level-dependent (BOLD), perfusion computed tomography (CTP), xenon-enhanced perfusion computed tomography (Xe-CT), single-photon emission computed tomography (SPECT), near infrared spectroscopy (NIRS), and transcranial doppler (TCD). The primary outcome of interest was the quantifiable effect that the induction of BS has on these measures of CBF. Animal studies included all studies performed in non-human animals including but not limited to rats, canines, swine, and non-human primates. Human studies included adults as well as pediatric populations.
Both continuous and intermittent methods of measures of CA were included in the search, a complete list of which can be found in Supplementary Appendix S1A.
Inclusion criteria for this study were as follows: BS measured by EEG in some form (visualized, bispectral index, power analysis); animal subjects, human participants, original full-length papers, use of some form of CBF measurement including the above, and/or a measurement of CA. Exclusion criteria included: non-English language and non-original studies.
MEDLINE, BIOSIS, Cochrane library, EMBASE, Global Health, and SCOPUS were all searched from their inceptions to August 2022 using relevant search terms. An example of the used search strategy can be found in Supplementary Appendix S1B. Search results were then compiled and deduplicated before being filtered for inclusion.
A two-step review of all articles returned by our search was conducted using two reviewers (A.Z.S. and L.F.). In the first filtering phase, reviewers independently screened all titles and abstracts for possible inclusion. Next, full texts of articles that passed the first filtering phase were assessed to confirm that they met all inclusion criteria. Any discrepancies between the two reviewers were resolved by the senior author (F.A.Z.). Finally, the reference lists for each included article were screened for any missed articles.
The following data was extracted from each of the final included articles and is presented in Supplementary Tables S1, S2: Study subject, experimental conditions, number of subjects, method of BS, method of BS determination, the measure of CBF, study results and conclusions, and study limitations.
Due to the heterogenous nature of the study designs and results of the relevant literature, no formal meta-analysis was conducted.
Given the overall goal of this review, to perform a comprehensive systematic review of the literature, a formal bias assessment was not conducted.
The overall search and filtration results have been summarized in Figure 2 using a PRISMA flow-diagram. The total results of the search, which was carried out over all five databases, yielded 35,673 articles. After deduplication, 20,401 unique articles were identified. Based on their title and abstract, 20,218 articles were found to not meet the inclusion/exclusion criteria during the first filter phase. The full text of the remaining 183 articles was reviewed in the second filter phase. After reviewing the full text, 118 articles were found to not meet inclusion/exclusion criteria leaving 65 articles. Examination of the reference sections of those texts yielded one additional article resulting in 66 studies being included in this scoping review.
FIGURE 2. PRISMA flow diagram. PRISMA, preferred reporting items for systematic reviews and meta analyses.
Overall, there were 66 studies that were included in the final results, 41 of which examined animals (Michenfelder, 1974; Harper and MacKenzie, 1977; Kassell et al., 1980; Gronert et al., 1981; Newberg et al., 1983; Milde et al., 1985; Maekawa et al., 1986; Scheller et al., 1986; Baughman et al., 1989; Warner et al., 1989; Lutz et al., 1990; Zornow et al., 1990; Roald et al., 1991; Artru et al., 1992; Ramani et al., 1992; Werner et al., 1992; Kochs et al., 1993; Golanov and Reis, 1995; Werner et al., 1995; Nemoto et al., 1996a; Nemoto et al., 1996b; Klementavicius et al., 1996; Young et al., 1997; Zarchin et al., 1998; Schmid-Elsaesser et al., 1999; Westermaier et al., 2000; Mäkiranta et al., 2002; Joshi et al., 2004; Joshi et al., 2005; Hungerhuber et al., 2006; Joshi et al., 2006; Liu et al., 2011; Liu et al., 2013a; Liu et al., 2013b; Walter et al., 2013; Sutin et al., 2014; Choi et al., 2016; Benveniste et al., 2017; Yang et al., 2018; Zhang et al., 2019; Berndt et al., 2021), 24 of which examined humans (Bendtsen et al., 1985; Woodcock et al., 1987; Young et al., 1991; Matta et al., 1995a; Matta et al., 1995b; Lam et al., 1995; Matta and Lam, 1995; Newman et al., 1995; Artru et al., 1997; Hoffman et al., 1997; Doyle and Matta, 1999; Matta et al., 1999; Ludbrook et al., 2002; Johnston et al., 2003; Reinsfelt et al., 2003; Reinsfelt et al., 2011; Zanatta et al., 2013; Du et al., 2014; Connolly et al., 2015; Chalia et al., 2016; Golkowski et al., 2017; Sahinovic et al., 2018; Akbik et al., 2020; Chaix et al., 2020; Kassab et al., 2021), and 1 of which examined both (Sirmpilatze et al., 2022). The study (Sirmpilatze et al., 2022) that examined both humans and animals will be included in both the animal and human sections. The animal studies are described in Supplementary Table S1 and the human studies are described in Supplementary Table S2. A brief overview of the studies can be seen in Table 1.
TABLE 1. Summary of the effect of different methods of BS on CBE and Autortgalation. GABA = a γ- aminobutyric acid; CMRO2 = Cerebral metabolic rate for oxygen ; AVDO2 = Arterio-venous different in oxygen.
Of the 42 studies that examined animals, six used propofol (Artru et al., 1992; Ramani et al., 1992; Werner et al., 1992; Joshi et al., 2006; Wang et al., 2011; Liu et al., 2013a), 13 used barbiturates (thiopental) (Michenfelder, 1974; Kassell et al., 1980; Gronert et al., 1981; Milde et al., 1985; Nemoto et al., 1996a; Nemoto et al., 1996b; Klementavicius et al., 1996; Zarchin et al., 1998; Schmid-Elsaesser et al., 1999; Westermaier et al., 2000; Mäkiranta et al., 2002; Joshi et al., 2005; Hungerhuber et al., 2006), 18 used inhalational anaesthetics (isoflurane) (Newberg et al., 1983; Maekawa et al., 1986; Scheller et al., 1986; Baughman et al., 1989; Lutz et al., 1990; Zornow et al., 1990; Roald et al., 1991; Kochs et al., 1993; Golanov and Reis, 1995; Liu et al., 2011; Walter et al., 2013; Sutin et al., 2014; Choi et al., 2016; Benveniste et al., 2017; Yang et al., 2018; Zhang et al., 2019; Berndt et al., 2021; Sirmpilatze et al., 2022), four used a combination of methods (Harper and MacKenzie, 1977; Warner et al., 1989; Young et al., 1997; Joshi et al., 2004), and one induced BS through hypotension (Werner et al., 1995).
In terms of the subjects that were used in the studies, 12 used different species of dogs (beagle, mongrel) (Michenfelder, 1974; Kassell et al., 1980; Gronert et al., 1981; Newberg et al., 1983; Milde et al., 1985; Lutz et al., 1990; Zornow et al., 1990; Roald et al., 1991; Artru et al., 1992; Werner et al., 1992; Kochs et al., 1993; Werner et al., 1995), 18 used different species of rodents (Sprague Dawley rats, wistar rats, gerbils) (Maekawa et al., 1986; Baughman et al., 1989; Warner et al., 1989; Golanov and Reis, 1995; Nemoto et al., 1996a; Nemoto et al., 1996b; Klementavicius et al., 1996; Young et al., 1997; Zarchin et al., 1998; Schmid-Elsaesser et al., 1999; Westermaier et al., 2000; Hungerhuber et al., 2006; Liu et al., 2011; Liu et al., 2013a; Sutin et al., 2014; Choi et al., 2016; Benveniste et al., 2017; Berndt et al., 2021), six used different species of rabbits (Scheller et al., 1986; Ramani et al., 1992; Joshi et al., 2004; Joshi et al., 2005; Joshi et al., 2006; Wang et al., 2011), two used different species of swine (Mäkiranta et al., 2002; Walter et al., 2013), three used different species of non-human primates (Harper and MacKenzie, 1977; Yang et al., 2018; Zhang et al., 2019), and one used both rodents and non-human primates (Sirmpilatze et al., 2022).
Out of the six studies that used propofol to induce BS, two measured CBF by using the Laser Doppler Flowmetry Method (Joshi et al., 2006; Wang et al., 2011), one by measuring blood flow in the superior sagittal sinus (Artru et al., 1992), one by functional MRI and BOLD signal analysis (Liu et al., 2013a), one by hydrogen clearance method (Ramani et al., 1992), and one by both quantitative autoradiography and TCD (Werner et al., 1992). Overall, four of the studies showed a significant change in CBF with BS, all of them showing a significant decrease (Artru et al., 1992; Ramani et al., 1992; Werner et al., 1992; Joshi et al., 2006). The lowest decrease that was seen was 16% (Joshi et al., 2006) and the highest was 70% (Artru et al., 1992; Werner et al., 1992). Further, the authors found that there was a significant correlation between cortical CBF and CBF velocity (r = 0.86; p < 0.05), and that cortical cerebral vascular resistance (CVR) and PI were significantly correlated (r = 0.79; p < 0.05).
Overall, there was only one paper that was found that examined an established measure of CA (Werner et al., 1992). Werner et al., found that, when BS was achieved, CBF decreased by 70% and that this decrease was paralleled by a 210% increase in CVR and a 50% increase in the PI (Werner et al., 1992). While the other studies did not look at direct measures of CA, the effect on CA can be inferred from the effect of BS on MAP and CBF concurrently. In all of the studies that showed a significant change in CBF under BS, there was a disproportionately larger decrease in CBF compared with the MAP (Artru et al., 1992; Ramani et al., 1992; Werner et al., 1992; Joshi et al., 2006) and in one study, CBF fell by 70% while MAP remained unchanged (Werner et al., 1992). One study investigated CBF under normocapnia and hypocapnia finding similar decreases in CBF with normocapnia and BS (76%) and with hypocapnia and BS (77%) (Artru et al., 1992). However, interestingly, Wang et al. did not find a significant effect of BS on CBF (Wang et al., 2011). This may be due to the fact that rabbits were already sedated with propofol in the baseline condition, confounding the results although this would suggest CBF is related to sedation instead of BS. Liu et al. used fMRI BOLD signal analysis and found increased subcortical connectivity under BS but the implications on CBF remain unclear (Liu et al., 2013b).
Out of the propofol studies, two examined CMRO2 in addition to CBF (Artru et al., 1992; Werner et al., 1992). Artru et al., found a 30% significant reduction in CMRO2 to parallel the 70% decrease in CBF(21) and Werner et al., found a 70% decrease in CMRO2 to match the 70% decrease in CBF(24). In summary, most of the evidence suggests that BS induced by propofol reduces CBF, but it remains unclear if the decrease in CBF induced by BS is associated with a concurrent decrease in CMRO2. Overall, there is a lack of evidence regarding the effect of BS induced by propofol on CA as assessed by well-established methods of CA measurement. However, given that CBF is disproportionately affected compared with MAP, there is some evidence to suggest that CA impairs autoregulation and the Lassen Curve (Lassen, 1959; Ruesch et al., 2017).
Out of the 13 studies that used barbiturates to induce BS, five measured CBF using the Laser Doppler Flowmetry Method (Zarchin et al., 1998; Schmid-Elsaesser et al., 1999; Westermaier et al., 2000; Joshi et al., 2005; Hungerhuber et al., 2006), three by measuring blood flow in the superior sagittal sinus (Michenfelder, 1974; Gronert et al., 1981; Milde et al., 1985), one by functional MRI and BOLD signal analysis (Mäkiranta et al., 2002), three by hydrogen clearance method (Nemoto et al., 1996a; Nemoto et al., 1996b; Klementavicius et al., 1996), one by quantitative autoradiography (Kassell et al., 1980). All of the studies used thiopental except for one that used pentobarbital (Gronert et al., 1981), one that used methohexital (Westermaier et al., 2000), and one that used etomidate (Milde et al., 1985). While etomidate is not technically a barbiturate, it is considered in this section as it is more like the barbiturate medications compared with propofol or inhalational anaesthetics.
Overall, 11 studies showed a significant reduction in CBF with induction of BS(25–28,30,33–37,87). The amount of reduction in CBF ranged from 23% (Zarchin et al., 1998) to 80% (Hungerhuber et al., 2006) in the studies in which the values are reported. Three of the studies did not measure or report change in MAP (Zarchin et al., 1998; Schmid-Elsaesser et al., 1999; Hungerhuber et al., 2006). Similar to the pattern seen in the propofol studies, in all of the studies in which MAP was recorded (Michenfelder, 1974; Kassell et al., 1980; Gronert et al., 1981; Milde et al., 1985; Nemoto et al., 1996a; Nemoto et al., 1996b; Klementavicius et al., 1996; Westermaier et al., 2000), the decrease in CBF was disproportionately greater than the decrease in MAP. Westermaier et al., showed that there was a 30% greater reduction in CBF in hypothermic rats that were burst suppressed compared with normothermic rats that were burst suppressed (p < 0.05) (Westermaier et al., 2000). However, this was not seen in another study where BS caused an equal 50% reduction in CBF in the normothermic and hypothermic rats (Nemoto et al., 1996a).
Out of the seven studies that examined CMRO2 (Michenfelder, 1974; Kassell et al., 1980; Gronert et al., 1981; Milde et al., 1985; Nemoto et al., 1996a; Nemoto et al., 1996b; Klementavicius et al., 1996), six showed a decrease that ranged from 42% to 54% (Michenfelder, 1974; Kassell et al., 1980; Milde et al., 1985; Nemoto et al., 1996a; Nemoto et al., 1996b; Klementavicius et al., 1996). The remaining study used pentobarbital and showed a 25% increase in CMRO2 in canines (Gronert et al., 1981). One noteworthy study found a strong positive correlation between CBF and CMRO2 (R = 0.77; p < 0.05) and a strong negative correlation between CVR and CMRO2 (R = −0.77; p < 0.05) (Kassell et al., 1980). One notable study demonstrated that CVR was inversely related to CBF, increasing to 214% of baseline when CBF was at 50% of baseline, and increasing to 300% of baseline when CBF reached its trough (Milde et al., 1985).
There were two studies that did not demonstrate a reduction in CBF with induction of BS (Mäkiranta et al., 2002; Joshi et al., 2005). One used fMRI and demonstrated that there was an increase as well as decrease in the BOLD signal depending on the specific region of interest in pigs (Mäkiranta et al., 2002). Joshi et al. measured the effect that BS induced by thiopental has on CBF using laser doppler flowmetry in rabbits but did not find any significant change (Joshi et al., 2005).
In summary, like the propofol studies, almost all of the studies demonstrated that BS causes a significant reduction in CBF. The reduction in CBF is paralleled by a decrease in CMRO2 implying that it may be due to reduced brain metabolism. Overall, there is a lack of evidence regarding the effect of BS induced by propofol on CA as assessed by well-established methods of CA measurement. However, again given the connection between CBF and CA, there may be some impairment of autoregulation.
Out of the 18 studies that used inhalational anaesthetics to induce BS, four measured blood flow in the superior sagittal sinus (Newberg et al., 1983; Lutz et al., 1990; Zornow et al., 1990; Roald et al., 1991), two used the Laser Doppler Flowmetry Method (Golanov and Reis, 1995; Berndt et al., 2021), two used quantitative autoradiography (Maekawa et al., 1986; Baughman et al., 1989), three used near infrared spectroscopy (Sutin et al., 2014; Choi et al., 2016; Yang et al., 2018), two used functional MRI and BOLD signal analysis (Zhang et al., 2019; Sirmpilatze et al., 2022), two used FDG-PET (Walter et al., 2013), two used hydrogen clearance method (Scheller et al., 1986), one analyzed glymphatic transport and used magnetic resonance time of flight (Liu et al., 2013b), one used laser doppler flowmetry and fMRI (Liu et al., 2011), and one used quantitative autoradiography and TCD (Kochs et al., 1993).
Almost all of the studies that used inhalational anaesthetic used isoflurane except for one that used desflurane (Lutz et al., 1990) and one that used nitrous oxide in addition to isoflurane (Baughman et al., 1989). There was more variability in the results of the isoflurane studies which may be in part be due to the variability in the techniques used. Of the studies that looked at overall CBF, four studies showed that BS led to a decrease in CBF (Baughman et al., 1989; Zornow et al., 1990; Walter et al., 2013; Berndt et al., 2021), two showed an increase in CBF (Ramani et al., 1992; Gomez et al., 2022), three showed no change (Newberg et al., 1983; Scheller et al., 1986; Roald et al., 1991), and two showed an increase or decrease depending on the region of the brain and the concentration of anaesthetic used (Maekawa et al., 1986; Lutz et al., 1990). In the remainder, conclusions could not be drawn on the average effect of BS on CBF but did shed light on neurovascular coupling (Golanov and Reis, 1995; Liu et al., 2011; Sutin et al., 2014; Zhang et al., 2019; Sirmpilatze et al., 2022). For example, several studies showed strong coupling between BS spikes and spikes in CBF (Golanov and Reis, 1995; Liu et al., 2011; Sutin et al., 2014; Zhang et al., 2019; Berndt et al., 2021). In one notable study that used FDG-PET, during BS there was a 48% reduction in CBF post onset of BS which was paralleled by a 42% reduction in CMRO2 (Walter et al., 2013). Further, there was a significant 31% reduction of metabolism for glucose in the neocortex, 25% in the basal ganglia, 20% reduction in the thalamus in neonatal pigs lending support to the concept that BS reduces CBF by reducing cerebral metabolic demand. In another study that used near infrared spectroscopy, as the duration of BS increased, total hemoglobin (Hgb) (R2 = 0.53, p < 0.05), and oxygenated hemoglobin (oxyHgb) significantly increased (R2 = 0.72, p < 0.05), and the amount of deoxygenated hemoglobin (deoxyHgb) significantly decreased (R2 = −0.92; p < 0.05) (Choi et al., 2016).
In summary, there still exists heterogeneity in the literature regarding the effects that BS induced by inhalational anaesthetics has on CBF and autoregulation. However, there is evidence to suggest that there is tight neurovascular coupling in the brain and spikes on EEG during the BS state are associated with concurrent spikes in CBF. Like the other anaesthetics, there is a paucity of literature on the effect of BS on CA measures.
Four studies used multiple methods to induce BS. Two used a barbiturate and inhalational anaesthetic (Harper and MacKenzie, 1977; Warner et al., 1989) but in one (Harper and MacKenzie, 1977), there was no baseline measurement of CBF prior to induction of BS so the conclusions that can be drawn are limited. In the other (Warner et al., 1989), compared to the sham condition, there was 67% lower CBF in the pentobarbital condition but no difference in the CBF in the isoflurane condition. Interestingly, there was no difference in MAP or in CMRglu with pentobarbital or isoflurane. Another study used a barbiturate and propofol (Joshi et al., 2004) to induce BS in rabbits but neither induced a significant change in CBF. Finally, one used an inhalational anaesthetic and propofol (Young et al., 1997) in an ischemia reperfusion model in rats and while CBF was not directly measured, the infarct volume in the propofol group was 21% significantly lower than in the isoflurane group (p < 0.001). This may suggest greater decrease in cerebral metabolism with propofol compared with isoflurane.
One study also used hemorrhagic hypotension to induce BS in dogs (Werner et al., 1995) but the experimental design itself examined the effect of CBF on BS instead of vice versa, limiting the conclusions that can be drawn.
In summary, there is mild evidence to suggest that barbiturates and propofol may have stronger effects on CBF compared with inhalational anaesthetics, but more studies are needed comparing the different anaesthetics. The effect on CA remains unclear.
Of the 24 studies that examined humans, six used propofol (Matta et al., 1995a; Newman et al., 1995; Doyle and Matta, 1999; Ludbrook et al., 2002; Klein et al., 2011; Chaix et al., 2020), four used barbiturates (thiopental) (Bendtsen et al., 1985; Young et al., 1991; Hoffman et al., 1997; Connolly et al., 2015), six used inhalational anaesthetics (isoflurane) (Lam et al., 1995; Artru et al., 1997; Reinsfelt et al., 2003; Reinsfelt et al., 2011; Golkowski et al., 2017; Sirmpilatze et al., 2022), six used a combination of methods (Woodcock et al., 1987; Matta et al., 1995b; Matta et al., 1999; Zanatta et al., 2013; Akbik et al., 2020), and three looked at the effect of brain injury on BS (Du et al., 2014; Chalia et al., 2016; Kassab et al., 2021).
In terms of the participants that were used in the studies, five involved adults undergoing cardiac surgery (coronary bypass graft or valve) (Woodcock et al., 1987; Newman et al., 1995; Reinsfelt et al., 2003; Reinsfelt et al., 2011; Zanatta et al., 2013), five involved adults undergoing open neurosurgical procedures (Bendtsen et al., 1985; Artru et al., 1997; Hoffman et al., 1997; Doyle and Matta, 1999; Klein et al., 2011), six involved adults undergoing orthopedic and other surgeries (Matta et al., 1995a; Matta et al., 1995b; Lam et al., 1995; Matta and Lam, 1995; Matta et al., 1999; Ludbrook et al., 2002), one involved patients undergoing carotid endarterectomy (Young et al., 1991), one involved patients undergoing minimally invasive neuroradiological procedures (Chaix et al., 2020), five involved critically ill and/or brain injured patients (Johnston et al., 2003; Connolly et al., 2015; Sahinovic et al., 2018; Akbik et al., 2020; Kassab et al., 2021), one involved infant patients with hypoxic ischemic encephalopathy (Chalia et al., 2016), and two involved healthy adults (Golkowski et al., 2017; Sirmpilatze et al., 2022).
Out of the six studies that used propofol to induce BS, one measured CBF using the Laser Doppler Flowmetry Method (Du et al., 2014), one by the Xe clearance (Newman et al., 1995), three by TCD (Lam et al., 1995; Doyle and Matta, 1999; Ludbrook et al., 2002), one by TCD and NIRS (Chaix et al., 2020).
Out of the six studies that used propofol to induce BS, five directly looked at CBF and all of them found a significant decrease in CBF (Matta et al., 1995a; Newman et al., 1995; Doyle and Matta, 1999; Ludbrook et al., 2002; Chaix et al., 2020). The highest reduction seen in CBF velocity was 42% (p < 0.0001) and it was achieved in adult patients undergoing orthopedic procedures (Ludbrook et al., 2002) and the highest decrease in CBF was 44% (p < 0.05), seen in adult patients undergoing cardiac surgery (note that in this study, the CBF was compared between groups rather than within groups) (Newman et al., 1995). In one notable study which looked at BS in addition to hypothermia, CBF was significantly lower in burst suppressed patients compared to controls at normothermia (44%; p < 0.05), hypothermia (41%; p < 0.05), and during rewarming (37%; p < 0.05); CMRO2 followed the same pattern (Newman et al., 1995). In all the studies, MAP either remained the same or did not decrease as much as CBF. In another study, patients receiving propofol were divided into two groups: one that included patients with more cardiovascular risk factors (“high risk”) and another with fewer cardiovascular risk factors (“low risk”) (Chaix et al., 2020). The “high risk” group had a higher drop in CBF with BS (34%) compared with the “low risk patients” (17%; p < 0.001) suggesting that patients with risk factors may already have altered CA. Overall, there is a lack of evidence regarding the effect of BS induced by propofol on CA as assessed by well-established methods of CA measurement.
In the one remaining study, venous capillary blood flow was measured in lieu of arterial CBF and there was no significant change found (Klein et al., 2011).
There were four studies that examined AVDO2 (Newman et al., 1995; Doyle and Matta, 1999; Ludbrook et al., 2002; Klein et al., 2011); all of the studies found no significant change in AVDO2 except for one which examined patients undergoing elective craniotomy and found that there was a 25% significant decrease and 58% significant decrease in AVDO2 in the group with lower bispectral index compared with the group with higher bispectral index (p = 0.022) (Klein et al., 2011).
Out of the 4 studies that used barbiturates, one uses althesin (Bendtsen et al., 1985), one used pentobarbital (Connolly et al., 2015), one used thiopental (Young et al., 1991), and one used etomidate (Hoffman et al., 1997).
In two of the studies, BS led to a change in CBF (Young et al., 1991; Hoffman et al., 1997); in Hoffman et al., there was a significant decrease of 40% in CBF (p < 0.05) which was also accompanied by a decrease in CMRO2 and in Young et al., there was a 48% significant decrease in CBF (p < 0.01). In the former, there was a 10% decrease in MAP and in the latter, phenylephrine was used to maintain MAP. In the other two studies, CBF was either not measured at baseline without BS, limiting the conclusions that can be drawn (Bendtsen et al., 1985) or the sample size was extremely small at 2 participants (Connolly et al., 2015). This evidence suggests that barbiturates decrease CBF and may impact CA but there is a dearth of literature regarding barbiturates compared with well-established measures of CA.
Out of the six studies that used inhalational anaesthetics, three used sevoflurane (Reinsfelt et al., 2011; Golkowski et al., 2017; Sirmpilatze et al., 2022), one used isoflurane (Reinsfelt et al., 2003), and the others used a combination of sevoflurane, isoflurane, and/or desflurane (Lam et al., 1995; Artru et al., 1997). There were four studies that showed a consistent effect on CBF or CBF velocity (Lam et al., 1995; Artru et al., 1997; Reinsfelt et al., 2003; Reinsfelt et al., 2011), all of which showed a consistent decrease after induction of BS. Notably, Artru et al, (1997) showed a 30% reduction in CBF velocity accompanied by a 36% reduction in CPP (p < 0.05) but no change in CVR with isoflurane, and 32% reduction in CBF velocity and 41% increase in CVR (p < 0.05) without a significant reduction in CPP with sevoflurane. There was only a 10% reduction in MAP in the isoflurane group and no reduction in MAP in the sevoflurane group. Reinsfelt et al, (2011) showed that BS led to a 27% reduction in CBF velocity (p < 0.05) but more interestingly, that this was also accompanied by a 13% decrease in cerebral oxygen extraction (COE) (p < 0.05). Further, under BS, there was a steeper positive slope of relationship between CBF velocity and CPP and a steeper negative slope between CPP and COE (p < 0.05), which may suggest the effect of sevoflurane was through vasodilation.
The remaining two studies performed BOLD signal analysis and showed that there was more widespread fluctuation in BOLD signal and significant correlation with cortical sensory areas and the thalamus during BS (p < 0.05) (Sirmpilatze et al., 2022) or a lower amplitude of the BOLD signal and significant correlation of the BOLD signal with BS in frontal, parietal, and temporal lobes and in basal ganglia (p < 0.001) (Golkowski et al., 2017).
In summary, inhalational anaesthetics may reduce CBF but more evidence is needed to determine what effect that these anaesthetics have on CVR and cerebral metabolism. Further, much like the studies with the other anaesthetics, there is a lack of evidence regarding the evidence regarding BS induced by inhalational anaesthetics and CA.
There were four studies that investigated the use of propofol in addition to inhalational anaesthetics (Matta et al., 1995b; Matta and Lam, 1995; Matta et al., 1999; Zanatta et al., 2013). Interestingly, all the studies showed that when sevoflurane, isoflurane, halothane, desflurane or nitric oxide are added to propofol, there is a corresponding increase in CBF velocity. There is also evidence to suggest that when nitric oxide is added to propofol, there is a corresponding 16% decrease in CVR and 14% increase in CMRO2 (p < 0.05) (Matta and Lam, 1995).
Woodcock et al. (1987) compared the effect that thiopental has on CBF with the effect of isoflurane under normothermia and hypothermia. Under both conditions, CBF was significantly lower in the thiopental burst suppressed group compared to control but there was no significant difference in CBF between isoflurane and control. Surprisingly, in the normothermic condition, CMRO2 was 21% lower in the thiopental group and 34% lower in the isoflurane group compared to control (p < 0.05). This difference was 34% and 29% respectively for thiopental and isoflurane in the hypothermic condition (p < 0.05). Taken together, this may mean that inhalational anaesthetics, while having a significant effect on cerebral metabolic demand, do not have as significant effect on CBF and autoregulation but more comparative studies are needed.
Finally, one study used FDG-PET with a smaller and more heterogenous sample size did not look at CBF directly (Akbik et al., 2020). However, it did show that BS led to reduced FDG uptake in patients with status epilepticus, stroke-like migraine attacks after radiotherapy, and epilepsy secondary to cerebral amyloid angiopathy but led to increased FDG uptake in patients with viral or autoimmune encephalitis. This may reflect the effect that underlying disease processes have on cerebral metabolism and the subsequent consequences that anaesthesia can have.
Overall, more research is needed regarding the differential effects of anaesthetics on CBF as well as how different anesthetics effect CA.
There were three studies that investigated the effect that brain injury has on EEG, CBF, and neurovascular coupling (Chalia et al., 2016; Sahinovic et al., 2018; Kassab et al., 2021). Kassab and others showed that, in a group of patients in status epilepticus, there was a significant positive correlation between seizure duration on EEG and hemodynamic response function (oxygenated hemoglobin p = 0.21 R2 = 0.569, total hemoglobin p = 0.034 R2 = 0.403); when BS was induce there was an increase in oxygenated hemoglobin and total hemoglobin following onset of bursts in representative hemodynamic response functions (Kassab et al., 2021). Chalia and others showed that there was a pronounced decrease in oxygenated hemoglobin just before or during EEG burst activity followed by large increase reaching peak 20s after burst onset (Chalia et al., 2016). Taken together, these studies suggest tight neurovascular coupling during BS.
In this systematically conducted scoping review, we identified 66 studies, most of which involved animals. This should be expected as manipulation of CBF using anaesthetics poses significant risk to humans and can only be investigated in participants who are receiving anaesthetics for some other purpose. This is also why most human studies involved patients who were injured or receiving a therapeutic surgery.
By far, most of the studies involved small animal models (rodents and rabbits), which are not as applicable to humans and is a weakness of the literature. The largest sample sized used in the small-animal models was 61 (Maekawa et al., 1986) and involved Sprague-Dawley rats. The largest sample size seen in the large animal models was 16 and involved non-human primates, but only two of the primates achieved BS (Zhang et al., 2019).
Six animal and seven human studies investigated propofol. Propofol is a γ-aminobutyric acid (GABA) agonist with a rapid terminal half life that readily crosses the blood brain barrier (Sahinovic et al., 2018). The most common adverse effects are bradycardia, hypotension, and very rarely, the life-threatening propofol infusion syndrome. In both animals and humans, propofol consistently showed a decrease in CBF in most studies of up to 70% in animals and up to a 44% decrease in humans. There were only two animal studies amongst the studies that showed a change in CBF that examined CMRO2 (Artru et al., 1992; Werner et al., 1992). One found that the CBF decreased to the same proportion as the CMRO2 (Werner et al., 1992) and another found that the CBF decreased disproportionately much greater compared to the decrease in CMRO2 (Artru et al., 1992). The former result would be expected if the decrease in CBF was purely due to flow-metabolism coupling whereas the latter would imply that propofol may have also had direct effects on the smooth muscle of the cerebral blood vessels themselves in addition to causing a reduction in CMR. Interestingly, in the human studies, while five studies demonstrated a significant decrease in CBF (Matta et al., 1995a; Newman et al., 1995; Doyle and Matta, 1999; Ludbrook et al., 2002; Klein et al., 2011; Chaix et al., 2020), all but one (Klein et al., 2011) showed no significant change in the AVDO2, supporting the conclusion that propofol decreases CBF through mechanisms other than flow-metabolism coupling. In the one study that investigated patients with TBI, even though CBF was not measured, there was no significant effect found on ICP or AVDO2 (Johnston et al., 2003). Interestingly, in one animal study, propofol reduced CBF by similar amounts under both normocapnia and hypocapnia (Artru et al., 1992) and in one human study, decreased CBF by similar amounts under both hypothermia and normothermia (Newman et al., 1995). Both hypothermia and hypercapnia are known to reduce CBF through vasoconstriction (Tameem and Krovvidi, 2013) and interestingly, it seems that when propofol is added, despite the fact that it reduces CBF on its own, the effect does not seem to be synergistic. Interestingly, it seems that patients who have cardiovascular risk factors have a higher reduction in CBF compared with those who have fewer risk factors (Chaix et al., 2020). In patients with hypertension, due to factors such as arterial remodelling and renin-angiotensin system, the CA curve is shifted to the right and cerebral ischemia can occur at higher MAPs compared to patients without hypertension (Tameem and Krovvidi, 2013; Machado et al., 2021).
Barbiturates have a similar mechanism of action to propofol but have longer half-lives and have higher risk of inducing hypotension (Shin et al., 2018). The number of animal studies that investigated barbiturates was comparable to the other anaesthetics; this contrasted with the human studies of which only four investigated barbiturates. Eleven of the animal students demonstrated that barbiturates had a significant reduction in CBF to a maximum of 80% (Michenfelder, 1974; Kassell et al., 1980; Gronert et al., 1981; Milde et al., 1985; Nemoto et al., 1996a; Nemoto et al., 1996b; Klementavicius et al., 1996; Zarchin et al., 1998; Schmid-Elsaesser et al., 1999; Westermaier et al., 2000; Hungerhuber et al., 2006). Six animal studies showed a decrease in CMRO2 (Michenfelder, 1974; Kassell et al., 1980; Milde et al., 1985; Nemoto et al., 1996a; Nemoto et al., 1996b; Klementavicius et al., 1996) and out of those six studies, five showed a decrease proportional to the decrease in CBF (Michenfelder, 1974; Kassell et al., 1980; Nemoto et al., 1996a; Nemoto et al., 1996b; Klementavicius et al., 1996). One study even found a strong positive correlation between CBF and CMRO2 (R = 0.77; p < 0.05) (Kassell et al., 1980). In another study in which CBF decreased disproportionately more than CMRO2 (75% vs. 54%), the anaesthetic used to induce BS was etomidate and CBF plateaued before BS was even reached (Milde et al., 1985). As mentioned earlier, etomidate is not technically a barbiturate and is structurally unrelated to other anaesthetics (Williams et al., 2022). Etomidate has been shown to cause depression in certain parts of the brain and cause hyperexcitability in others such as the basal ganglia. However, in the one human study that used etomidate for anaesthesia, the CBF and CMRO2 decreased proportionally by 40% (Hoffman et al., 1997). Therefore, it remains unclear that is the effect etomidate has on CBF is due to its decrease in CMR alone or also due to other mechanisms. Interestingly, the only animal study in which CMRO2 was increased used pentobarbital (Gronert et al., 1981). This was presumably due to tolerance as there is evidence to suggest that tolerance develops much quicker with pentobarbital compared to the other barbarities (Saunders et al., 1990). There was a significant lack of human studies that investigated barbiturates; out of the four, one used etomidate (Hoffman et al., 1997) and one only had a sample size of 2 (Connolly et al., 2015). The lack of studies investigating barbiturates on humans may be due to its unpopularity as an anaesthetic given their tendency to cause hypotension (Shin et al., 2018).
It has been well-described that inhalational anaesthetics produce deep sedation by reducing activation in the thalamus and midbrain reticular formations (Campagna et al., 2003). However, while there is a reduction in brain activation in several cortical areas, activation of the visual, motor, and subcortical regions seems to be unaffected. While inhalational anaesthetics primarily target the GABA receptor, they have effects on a plethora of other channels including glycine receptors, nicotinic acetylcholine receptors, and glutamate receptors (Campagna et al., 2003). There were a total of 18 animal and 6 human studies that used inhalational anaesthetics. The animal literature was extremely variable in terms of the effect that inhalational anaesthetics have on CBF and only four showed a decrease in CBF (Baughman et al., 1989; Zornow et al., 1990; Walter et al., 2013; Berndt et al., 2021). This may have been because, as previously mentioned, inhalational anaesthetics have different effects on the activity of different parts of the brain. In addition, the studies used a variety of concentrations of isoflurane, ranging from 1%–3%. In one study, at a concentration of 1.0 minimum alveolar concentration (MAC), there was no change in CBF but at 1.5 MAC the auditory cortex saw a 4.9% significant decrease in CBF (p < 0.05), the visual cortex did not see any significant change, and the extrapyramidal system (140%–170%) and limbic system (87%–120%) saw a significant increase, and finally, with 2.0 MAC, all regions saw a significant increase in CBF (Maekawa et al., 1986). Further, in one fMRI study, there was more widespread fluctuation in BOLD signal and significant correlation with cortical sensory areas and the thalamus during BS (Sirmpilatze et al., 2022) and in another a lower amplitude of the BOLD signal and significant correlation of the BOLD signal with BS in frontal, parietal, and temporal lobes and in basal ganglia (Golkowski et al., 2017). While CMRglu decreased across the brain, it did not decrease in the hippocampus or corpus callosum at any concentrations (Maekawa et al., 1986). Several of the animal inhalational anaesthetic studies (Golanov and Reis, 1995; Liu et al., 2011; Sutin et al., 2014; Zhang et al., 2019; Berndt et al., 2021) demonstrated strong correlation between EEG bursts/spikes and spikes in CBF, lending support to the mechanisms behind flow-metabolism coupling in the control of CBF. Out of the six human studies that used inhalational anaesthetics, four showed a consistent decrease in CBF or CBF velocity (Roald et al., 1991; Kochs et al., 1993; Liu et al., 2013b; Yang et al., 2018). While sevoflurane did reduce CBF velocity and CBF (Artru et al., 1997; Reinsfelt et al., 2011), but it did not show any significant reduction in CPP (Artru et al., 1997). This was in contrast to isoflurane which showed a 36% reduction in CPP in the same study (Artru et al., 1997). None of the human studies examined the effect of the anaesthetics on CMRO2 or AVDO2 and therefore, many questions remain unanswered regarding the effect that isoflurane and other inhalational drugs have on CBF and to what extent the effect is mediated by a change in CMR.
In terms of the studies that investigated multiple anaesthetics, there was evidence to suggest that when inhalational anaesthetics are added to propofol, there is an increase in CBF velocity compared to the use of propofol alone. This may be because inhalational anaesthetics increase CBF in certain parts of the brain, thereby decreasing the effect that propofol has. Further, inhalational anaesthetics affect a variety of receptor sites including glutamate receptors and by doing so may nullify the effect of propofol (e.g., inhibition of inhibition). Compared to inhalational anaesthetics, barbiturates seem to have a higher effect on reducing CBF, which was seen in an animal (Warner et al., 1989) as well as a human study (Woodcock et al., 1987). This may be due in part to the higher likelihood of barbiturates to cause hypotension but may also be because inhalational anaesthetics have different effects depending on the region in the brain (Campagna et al., 2003; Golkowski et al., 2017; Sirmpilatze et al., 2022).
Overall, there was a significant lack of discussion of autoregulation in the studies that were examined in the present review, as will be discussed in the limitations section. Previous work has shown that, at lower doses, propofol, barbiturates, and inhalational anaesthetics do not affect CBF and autoregulation (Veselis et al., 2005; Farag et al., 2017; Slupe and Kirsch, 2018) at low doses but at higher doses CBF and autoregulation are impaired (Slupe and Kirsch, 2018). It was unclear why higher doses of anaesthetics cause this change. Given that most of the evidence pointed toward BS causing a reduction in CBF that was disproportionately greater than the reduction in MAP (Michenfelder, 1974; Kassell et al., 1980; Gronert et al., 1981; Milde et al., 1985; Artru et al., 1992; Ramani et al., 1992; Werner et al., 1992; Matta et al., 1995b; Newman et al., 1995; Nemoto et al., 1996a; Doyle and Matta, 1999; Westermaier et al., 2000; Ludbrook et al., 2002; Joshi et al., 2006; Chaix et al., 2020), it can possibly be inferred that BS has at least some effect on autoregulation impairment. While there was some evidence that CBF changes in proportion to CMRO2 (Michenfelder, 1974; Kassell et al., 1980; Milde et al., 1985; Werner et al., 1992; Nemoto et al., 1996a; Klementavicius et al., 1996), there was also evidence that CBF changed disproportionately (Milde et al., 1985; Baughman et al., 1989; Artru et al., 1992), making it challenging to know what effect exactly BS has on autoregulation. It is also possible that different depths of sedation have a significant effect on animal and human cerebral autoregulation. For example, previous work looked at the relationship between objectively measured depth of sedation (through the bispectrality index) and cerebrovascular reactivity (surrogate measure of cerebral autoregulation) in patients with TBI (Froese et al., 2022a; Froese et al., 2022b). From this work it was seen in almost all patients that there was a depth of sedation that optimized cerebrovascular reactivity (achieve the most intact cerebral reactivity value), indicating that too much/little sedation results in non-optimal cerebral states.
In most of the studies, it was clear that BS decreases CBF, and this effect is most pronounced with propofol and barbiturates. However, it remains unclear why BS has this effect. CBF is believed to be controlled by four primary mechanisms: myogenic, endothelial, neurogenic, and flow-metabolism coupling (Tameem and Krovvidi, 2013). In the myogenic mechanisms, the smooth muscle cells themselves are responsible to modulating cerebral vessel diameter. The endothelial theory suggests that shear-stress induced by blood flow changes on the endothelial lining leading to induction of vasoactive mediators, such as nitric oxide or endothelin, precipitating changes in vascular tone. In the neurogenic mechanism, vasoconstriction is caused by norepinephrine and neuropeptide Y released from postganglionic sympathetic from the superior cervical ganglion. Finally, in flow-metabolic coupling, CBF is closely matched to the demands of the neuronal firing. Flow-metabolic coupling is thought to be mediated by astrocytes, a type of glial cell in the brain. While the process is not entirely understood, it is believed that astrocytes communicate the activity of neurons to blood vessels and coordinate the energy demand with oxygen and glucose supply (Venkat et al., 2016). Astrocytes may mediate the synthesis of vasoactive substances, thereby inducing vasodilation when neuronal activity increases. Reasonably, it is unlikely that the anaesthetics have much of an effect on the smooth muscle cells or on the sympathetic ganglion as much as they do on the neuronal activity. However, given that many studies did not investigate CMRO2 and a significant few looked at CMRglu, it is challenging to know if this is indeed the mechanism. One of the main confounders in the human studies was the maintenance of MAP. In some of the studies, MAP was maintained with pressors above a given level with pressors while in others, it was allowed to drop. First, if MAP is allowed to drop, it is difficult to state whether CBF dropped due to a drop in systemic hemodynamics or due to the BS induced by the anaesthetic. Interestingly, in previous papers our team has shown that, while pressors cause vasoconstriction when applied to cerebral blood vessels directly, there is no effect when applied systemically (Froese et al., 2020b; Froese et al., 2020c). Therefore, vasopressors do not appear to have a significant effect on CBF or autoregulation. Without MAP being maintained throughout the experiment, it is challenging to know if CBF dropped due to alteration of cerebral hemodynamics and therefore a myogenic mechanism or due to a flow-metabolic coupling method. Further, without estimating the amount of sedation that is given to the subjects, no firm conclusions can be drawn in relation to the effects that BS induced by anaesthetics has on CBF. More animal but especially more human studies are needed to clarify how and why BS causes a decrease in CBF.
It remains unclear what effect inhalational anaesthetics have on CBF as there were mixed results from the studies. These anaesthetics are especially interesting as they seem to not have as much hemodynamic depression (Campagna et al., 2003) as other agents and are especially useful for disease processes such as refractory status epilepticus (Mirsattari et al., 2004). Further, if inhalational anaesthetics are used in combination with other anaesthetics in the neurocritical care unit, could the profiles of both agents be used in a synergistic fashion? For example, adding isoflurane to propofol in a patient in whom it would be beneficial to reduce the CMR but not the CBF as much. There still exists a gap in the literature regarding inhalational anaesthetics as well as the use of a combination of anaesthetics in patients. There was a significant lack of studies that investigated the effect of BS on CBF in patients with critical neurological illness or traumatic brain injury. Given that these patients may have altered autoregulation (Armstead, 2016), it would be pertinent to see how these patients respond to the different anaesthetics.
Finally, most of the studies that were examined in the present review did not measure the effect of BS on autoregulation. From the results of the present review, we can conclude that BS leads to a decrease in CBF but given that autoregulation links CBF and CMRO2 by flow-metabolism coupling, the drop in CBF may be an appropriate response of autoregulation (Tameem and Krovvidi, 2013). There are two overarching methods of measuring autoregulation: a “static” method and a “dynamic” method (Tiecks et al., 1995). The older, static method involves measuring CBF at a constant baseline MAP and constant CBF. After manipulation of the MAP is undertaken, another measure of CBF is taken and if this measure changes significantly, there is an impairment in CA (Tiecks et al., 1995). The major disadvantage with this method is that there is no assessment of the time in which the change in CVR occurs. The dynamic method involves using a thigh blood pressure cuff (Obrist et al., 1975); the cuff is inflated and rapidly released which causes rapid drops in MAP. During this process, MAP and CBF velocity, measured using TCD, and the latency of the change in CVR can be measured. In using both methods with BS, there exists a confound. One possible method of inferring a loss of autoregulation is if CBF decreases disproportionately more than CMRO2 as they should otherwise be tightly coupled. More studies are needed to measure the effect of BS on CBF and CMRO2 using both static and dynamic methods.
One of the main limitations to the present review was its breadth. By design, the review’s purpose was to examine the effect of BS on overall cerebral physiology, hence, we were not selective about the methods used to measure CBF or to induce BS. The advantage to this was that we were able to assess the effect of BS in a wide variety of scenarios and in different species. However, by including a vast array of methodologies, we compromised on accuracy. For example, we included studies that used TCD in the present review even though TCD measures CBF velocity which is not completely analogous to CBF and under conditions in which the vessel diameter is variable, can under or overestimate CBF (Coverdale et al., 2014). In addition, we included studies that measured CBF using the BOLD signal, which only measures changes in oxygenated blood (Logothetis, 2002). Nevertheless, inclusion of these studies allowed us to examine the effect of BS on cerebral physiology in a variety of experimental conditions.
One limitation of the present review is that we did not compare the different techniques used to measure CBF. A number of different techniques were used in the studies mentioned in this review and some are known to be more accurate than others. However, the primary goal of the review was to take the techniques as a whole to determine what the consensus was on the effect of BS and CBF, and despite the variety of methods used, there was agreement amongst most of the studies. In future work, it may be beneficial to examine the different techniques used and their accuracy for measuring CBF.
Another limitation was that we focused on the quantitative effect that BS has on CBF but did not look at as much on the clinical implications of this in animals, in healthy controls or in patients with brain injury. For example, if certain anaesthetics are superior at reducing CMRO2, they might be more neuroprotective or if others have a high effect on CBF, they may not be the optimal choice for patients at risk for brain ischemia. In future work, we hope to look at the clinical significance of different anaesthetics and how this relates to their specific action on CBF.
Finally, in the present review, we were not able to conduct a meta-analysis of the data due to extremely heterogenous populations and data sets. In future work, it would be pertinent to narrow our search to studies in which there are more homogenous populations (e.g., patients with status epilepticus) and determine the effect size that BS has on CBF or even on clinical outcomes.
Future work should use in vivo methods of monitoring CBF such as NIRS in neurologically ill patients to determine the effect of BS in this population. The NIRS technique uses the infrared light, which can penetrate into deep tissues, and be absorbed by oxygenated and deoxygenated hemoglobin (Chen et al., 2020). The technique can determine the regional concentration of oxyhemoglobin and deoxyhemoglobin and thereby, the oxygenation of the tissue. In other words, NIRS is a cost-effective, portable, and efficient means of measuring neurovascular coupling. The advantage of being able to monitor cerebral physiology continuously and non-invasively in critically ill patients make it a favored technique for further investigating the questions left unanswered by the present review. A previous scoping review of 47 studies by our team showed evidence that continuous-wave NIRS metrics were linearly correlated with changes in CBF (Gomez et al., 2022). Cerebral oxygenation can be obtained from NIRS, and by correlating this value to MAP, one can obtain the cerebral oximetry index (COx) which is a measure of CA (Chen et al., 2020). When COx is close to zero or negative, autoregulation is being maintained but when the value is closer to one, autoregulation is impaired. Using NIRS, EEG, and BS, the effect of the above-discussed anaesthetics could be investigated in patients admitted to the neurological ICU in real time. Other evidence has shown that NIRS can predict epileptic spikes even before the spikes are seen on EEG (Chen et al., 2020). In patients with status epilepticus, the technique could be used to investigate CBF, autoregulation, and metabolism in this critically ill group of patients to determine how best to use BS to improve morbidity and mortality. Concurrent work by our group aims to answer these questions using continuous in-vivo methods of neuromonitoring such as NIRS.
In this systematic review of the effect of BS on CBF and autoregulation in animals and humans, we identified 67 studies. Most of the literature involved animal models, which largely involved small animals. In the human studies, most were conducted on patients undergoing surgery or who were critically ill, as expected. There was significant evidence to suggest that propofol and barbiturates decrease CBF in both animals and humans. However, the evidence regarding inhalational anaesthetics, comparative effects, and clinical implications is lacking. More large animal models as well as clinically relevant human studies are needed to further elucidate the mechanisms behind BS and CBF and what implications this has on the future of care in the neurological intensive care unit.
The original contributions presented in the study are included in the article/Supplementary Material, further inquiries can be directed to the corresponding author.
AZS conducted the search, extracted the data, created the tables, and wrote the first draft of the manuscript. LF helped in conducting the search, contributed to the conception and design of the study, and edited the final manuscript. AG, AZS, KS, KP, and NV proofread and edited the final manuscript. FZ conceptualized and designed the study, edited the manuscript, and supervised the project. All authors contributed to the article and approved the submitted version.
This work was directly supported through the Manitoba Public Insurance (MPI) Professorship in Neuroscience, and the Natural Sciences and Engineering Research Council of Canada (NSERC) (DGECR-2022-00260, RGPIN-2022-03621, ALLRP-576386-22, and ALLRP-578524-22).
We would like to acknowledge the contribution of Dr. Marcus Ng who generously allowed us to use Figure 1, adapted from one of his previous publications. FAZ receives research support from the Manitoba Public Insurance (MPI) Professorship in Neuroscience/TBI Research Endowment, NSERC, CIHR, the MPI Neuroscience Research Operating Fund, the Health Sciences Centre Foundation Winnipeg, the Canada Foundation for Innovation (CFI) (Project #: 38583), Research Manitoba (Grant #: 3906 and 5429), and the University of Manitoba VPRI Research Investment Fund (RIF). AG is supported through the University of Manitoba Clinician Investigator Program and a Canadian Institutes of Health Research (CIHR) Fellowship (Grant #: 472286). ASS is supported through the University of Manitoba Graduate Fellowship (Doctoral)—Biomedical Engineering, NSERC (RGPIN-2022-03621) and the University of Manitoba Graduate Enhancement of Tri-Agency Stipend (GETS) program. LF is supported through the University of Manitoba—Department of Surgery GFT Research Grant, the University of Manitoba—University Research Grant Program (URGP), the Biomedical Engineering (BME) Fellowship Grant at the University of Manitoba, the Research Manitoba PhD Studentship, NSERC (ALLRP-576386-22) and the University of Manitoba Graduate Enhancement of Tri-Agency Stipend (GETS) program. KS is supported by the University of Manitoba MD/PhD program and the University of Manitoba Graduate Fellowship program. NV is supported by NSERC (RGPIN-2022-03621).
The authors declare that the research was conducted in the absence of any commercial or financial relationships that could be construed as a potential conflict of interest.
All claims expressed in this article are solely those of the authors and do not necessarily represent those of their affiliated organizations, or those of the publisher, the editors and the reviewers. Any product that may be evaluated in this article, or claim that may be made by its manufacturer, is not guaranteed or endorsed by the publisher.
The Supplementary Material for this article can be found online at: https://www.frontiersin.org/articles/10.3389/fphys.2023.1204874/full#supplementary-material
Akbik, F., Robertson, M., Das, A. S., Singhal, T., Lee, J. W., and Vaitkevicius, H. (2020). The PET sandwich: Using serial FDG-PET scans with interval burst suppression to assess ictal components of disease. Neurocrit Care 33 (3), 657–669. doi:10.1007/s12028-020-00956-w
Amzica, F. (2009). Basic physiology of burst-suppression. Epilepsia 50 (12), 38–39. doi:10.1111/j.1528-1167.2009.02345.x
Amzica, F. (2015). What does burst suppression really mean? Epilepsy Behav. 49, 234–237. doi:10.1016/j.yebeh.2015.06.012
Armstead, W. M. (2016). Cerebral blood flow autoregulation and dysautoregulation. Anesthesiol. Clin. 34 (3), 465–477. doi:10.1016/j.anclin.2016.04.002
Artru, A. A., Lam, A. M., Johnson, J. O., and Sperry, R. J. (1997). Intracranial pressure, middle cerebral artery flow velocity, and plasma inorganic fluoride concentrations in neurosurgical patients receiving sevoflurane or isoflurane. Anesth. Analg. 85 (3), 587–592. doi:10.1097/00000539-199709000-00019
Artru, A. A., Shapira, Y., and Bowdle, T. A. (1992). Electroencephalogram, cerebral metabolic, and vascular responses to propofol anesthesia in dogs. J. Neurosurg. Anesthesiol. 4 (2), 99–109. doi:10.1097/00008506-199204000-00005
Batson, C., Stein, K. Y., Gomez, A., Sainbhi, A. S., Froese, L., Alizadeh, A., et al. (2022). Intracranial pressure–derived cerebrovascular reactivity indices, chronological age, and biological sex in traumatic brain injury: A scoping review. Neurotrauma Rep. 3 (1), 44–56. doi:10.1089/neur.2021.0054
Baughman, V. L., Hoffman, W. E., Thomas, C., Albrecht, R. F., and Miletich, D. J. (1989). The interaction of nitrous oxide and isoflurane with incomplete cerebral ischemia in the rat. Anesthesiology 70 (5), 767–774. doi:10.1097/00000542-198905000-00011
Bendtsen, A., Kruse, A., Madsen, J. B., Astrup, J., Rosenørn, J., Blatt-Lyon, B., et al. (1985). Use of a continuous infusion of althesin in neuroanaesthesia. Changes in cerebral blood flow, cerebral metabolism, the EEG and plasma alphaxalone concentration. Br. J. Anaesth. 57 (4), 369–374. doi:10.1093/bja/57.4.369
Benveniste, H., Lee, H., Ding, F., Sun, Q., Al-Bizri, E., Makaryus, R., et al. (2017). Anesthesia with dexmedetomidine and low-dose isoflurane increases solute transport via the glymphatic pathway in rat brain when compared with high-dose isoflurane. Anesthesiol. Hagerst. 127, 976–988. doi:10.1097/ALN.0000000000001888
Berndt, N., Kovács, R., Schoknecht, K., Rösner, J., Reiffurth, C., Maechler, M., et al. (2021). Low neuronal metabolism during isoflurane-induced burst suppression is related to synaptic inhibition while neurovascular coupling and mitochondrial function remain intact. J. Cereb. Blood Flow. Metab. Off. J. Int. Soc. Cereb. Blood Flow. Metab. 41 (10), 2640–2655. doi:10.1177/0271678X211010353
Campagna, J. A., Miller, K. W., and Forman, S. A. (2003). Mechanisms of actions of inhaled anesthetics. N. Engl. J. Med. 348 (21), 2110–2124. doi:10.1056/NEJMra021261
Chaix, I., Manquat, E., Liu, N., Casadio, M. C., Ludes, P. O., Tantot, A., et al. (2020). Impact of hypotension on cerebral perfusion during general anesthesia induction: A prospective observational study in adults. Acta Anaesthesiol. Scand. 64 (5), 592–601. doi:10.1111/aas.13537
Chalia, M., Lee, C. W., Dempsey, L. A., Edwards, A. D., Singh, H., Michell, A. W., et al. (2016). Hemodynamic response to burst-suppressed and discontinuous electroencephalography activity in infants with hypoxic ischemic encephalopathy. Neurophotonics 3 (3), 031408. doi:10.1117/1.NPh.3.3.031408
Chen, W. L., Wagner, J., Heugel, N., Sugar, J., Lee, Y. W., Conant, L., et al. (2020). Functional near-infrared spectroscopy and its clinical application in the field of neuroscience: Advances and future directions. Front. Neurosci. 14, 724. doi:10.3389/fnins.2020.00724
Choi, D. H., Shin, T. J., Kim, S., Bae, J., Cho, D., Ham, J., et al. (2016). Monitoring cerebral oxygenation and local field potential with a variation of isoflurane concentration in a rat model. Biomed. Opt. Express 7 (10), 4114–4124. doi:10.1364/BOE.7.004114
Cochrane Handbook (2023). Cochrane Handbook for systematic reviews of interventions. [Internet][cited 2023 Mar 12]. Available from: https://training.cochrane.org/handbook.
Connolly, M., Vespa, P., Pouratian, N., Gonzalez, N. R., and Hu, X. (2015). Characterization of the relationship between intracranial pressure and electroencephalographic monitoring in burst-suppressed patients. Neurocrit Care 22 (2), 212–220. doi:10.1007/s12028-014-0059-8
Coverdale, N. S., Gati, J. S., Opalevych, O., Perrotta, A., and Shoemaker, J. K. (2014). Cerebral blood flow velocity underestimates cerebral blood flow during modest hypercapnia and hypocapnia. J. Appl. Physiol. 117 (10), 1090–1096. doi:10.1152/japplphysiol.00285.2014
Doyle, P. W., and Matta, B. F. (1999). Burst suppression or isoelectric encephalogram for cerebral protection: Evidence from metabolic suppression studies. Br. J. Anaesth. 83 (4), 580–584. doi:10.1093/bja/83.4.580
Du, B., Shan, A., Zhang, Y., Zhong, X., Chen, D., and Cai, K. (2014). Zolpidem arouses patients in vegetative state after brain injury: Quantitative evaluation and indications. Am. J. Med. Sci. 347, 178–182. doi:10.1097/MAJ.0b013e318287c79c
Farag, E., Kot, M., Podolyak, A., Argalious, M., Deogaonkar, M., Mascha, E. J., et al. (2017). The relative effects of dexmedetomidine and propofol on cerebral blood flow velocity and regional brain oxygenation: A randomised noninferiority trial. Eur. J. Anaesthesiol. 34 (11), 732–739. doi:10.1097/EJA.0000000000000662
Froese, L., Dian, J., Batson, C., Gomez, A., Unger, B., and Zeiler, F. A. (2020). Cerebrovascular response to propofol, fentanyl, and midazolam in moderate/severe traumatic brain injury: A scoping systematic review of the human and animal literature. Neurotrauma Rep. 1 (1), 100–112. doi:10.1089/neur.2020.0040
Froese, L., Dian, J., Gomez, A., Unger, B., and Zeiler, F. A. (2020). Cerebrovascular response to phenylephrine in traumatic brain injury: A scoping systematic review of the human and animal literature. Neurotrauma Rep. 1 (1), 46–62. doi:10.1089/neur.2020.0008
Froese, L., Dian, J., Gomez, A., Unger, B., and Zeiler, F. A. (2020). The cerebrovascular response to norepinephrine: A scoping systematic review of the animal and human literature. Pharmacol. Res. Perspect. 8 (5), e00655. doi:10.1002/prp2.655
Froese, L., Gomez, A., Sainbhi, A. S., Batson, C., Slack, T., Stein, K. Y., et al. (2022). Optimal bispectral index level of sedation and cerebral oximetry in traumatic brain injury: A non-invasive individualized approach in critical care? Intensive Care Med. Exp. 10 (1), 33. doi:10.1186/s40635-022-00460-9
Froese, L., Gomez, A., Sainbhi, A. S., Batson, C., Stein, K., Alizadeh, A., et al. (2022). Continuous determination of the optimal bispectral index value based on cerebrovascular reactivity in moderate/severe traumatic brain injury: A retrospective observational cohort study of a novel individualized sedation target. Crit. Care Explor 4 (3), e0656. doi:10.1097/CCE.0000000000000656
Frohlich, J., Johnson, M. A., McArthur, D. L., Lutkenhoff, E. S., Dell’Italia, J., Real, C., et al. (2021). Sedation-induced burst suppression predicts positive outcome following traumatic brain injury. Front. Neurol. 12, 750667. doi:10.3389/fneur.2021.750667
Golanov, E. V., and Reis, D. J. (1995). Vasodilation evoked from medulla and cerebellum is coupled to bursts of cortical EEG activity in rats. Am. J. Physiol. 268 (2), R454–R467. doi:10.1152/ajpregu.1995.268.2.R454
Golkowski, D., Ranft, A., Kiel, T., Riedl, V., Kohl, P., Rohrer, G., et al. (2017). Coherence of BOLD signal and electrical activity in the human brain during deep sevoflurane anesthesia. Brain Behav. 7 (7), e00679. doi:10.1002/brb3.679
Gomez, A., Sainbhi, A. S., Froese, L., Batson, C., Slack, T., Stein, K. Y., et al. (2022). The quantitative associations between near infrared spectroscopic cerebrovascular metrics and cerebral blood flow: A scoping review of the human and animal literature. Front. Physiol. 13, 934731. doi:10.3389/fphys.2022.934731
Gronert, G. A., Michenfelder, J. D., Sharbrough, F. W., and Milde, J. H. (1981). Canine cerebral metabolic tolerance during 24 hours deep pentobarbital anesthesia. Anesthesiology 55 (2), 110–113. doi:10.1097/00000542-198108000-00005
Harper, M. A., and MacKenzie, E. T. (1977). Cerebral circulatory and metabolic effects of 5-hydroxytryptamine in anesthetized baboons. J. Physiol. 271 (3), 721–733. doi:10.1113/jphysiol.1977.sp012022
Hoffman, W. E., Charbel, F. T., and Ausman, J. I. (1997). Cerebral blood flow and metabolic response to etomidate and ischemia. Neurol. Res. 19 (1), 41–44. doi:10.1080/01616412.1997.11740770
Hofmeijer, J., Tjepkema-Cloostermans, M. C., and van Putten, M. J. A. M. (2014). Burst-suppression with identical bursts: A distinct EEG pattern with poor outcome in postanoxic coma. Clin. Neurophysiol. Off. J. Int. Fed. Clin. Neurophysiol. 125 (5), 947–954. doi:10.1016/j.clinph.2013.10.017
Hungerhuber, E., Zausinger, S., Westermaier, T., Plesnila, N., and Schmid-Elsaesser, R. (2006). Simultaneous bilateral laser Doppler fluxmetry and electrophysiological recording during middle cerebral artery occlusion in rats. J. Neurosci. Methods 154 (1–2), 109–115. doi:10.1016/j.jneumeth.2005.12.004
Johnston, A. J., Steiner, L. A., Chatfield, D. A., Coleman, M. R., Coles, J. P., Al-Rawi, P. G., et al. (2003). Effects of propofol on cerebral oxygenation and metabolism after head injury. Br. J. Anaesth. 91 (6), 781–786. doi:10.1093/bja/aeg256
Joshi, S., Wang, M., Etu, J. J., Nishanian, E. V., and Pile-Spellman, J. (2006). Cerebral blood flow affects dose requirements of intracarotid propofol for electrocerebral silence. Anesthesiology 104 (2), 290–298. discussion 5A. doi:10.1097/00000542-200602000-00014
Joshi, S., Wang, M., Etu, J. J., and Pile-Spellman, J. (2005). Reducing cerebral blood flow increases the duration of electroencephalographic silence by intracarotid thiopental. Anesth. Analg. 101 (3), 851–858. doi:10.1213/01.ANE.0000160583.42078.B2
Joshi, S., Wang, M., Nishanian, E. V., and Emerson, R. G. (2004). Electrocerebral silence by intracarotid anesthetics does not affect early hyperemia after transient cerebral ischemia in rabbits. Anesth. Analg. 98 (5), 1454–1459. table of contents. doi:10.1213/01.ane.0000112320.77816.fa
Kassab, A., Hinnoutondji Toffa, D., Robert, M., Lesage, F., Peng, K., and Khoa Nguyen, D. (2021). Hemodynamic changes associated with common EEG patterns in critically ill patients: Pilot results from continuous EEG-fNIRS study. NeuroImage Clin. 32, 102880. doi:10.1016/j.nicl.2021.102880
Kassell, N. F., Hitchon, P. W., Gerk, M. K., Sokoll, M. D., and Hill, T. R. (1980). Alterations in cerebral blood flow, oxygen metabolism, and electrical activity produced by high dose sodium thiopental. Neurosurgery 7 (6), 598–603. doi:10.1227/00006123-198012000-00011
Klein, K. U., Fukui, K., Schramm, P., Stadie, A., Fischer, G., Werner, C., et al. (2011). Human cerebral microcirculation and oxygen saturation during propofol-induced reduction of bispectral index. Br. J. Anaesth. 107, 735–741. doi:10.1093/bja/aer227
Klementavicius, R., Nemoto, E. M., and Yonas, H. (1996). The Q10 ratio for basal cerebral metabolic rate for oxygen in rats. J. Neurosurg. 85 (3), 482–487. doi:10.3171/jns.1996.85.3.0482
Kochs, E., Hoffman, W. E., Werner, C., and Albrecht, R. F. (1993). Cerebral blood flow velocity in relation to cerebral blood flow, cerebral metabolic rate for oxygen, and electroencephalogram analysis during isoflurane anesthesia in dogs. Anesth. Analg. 76 (6), 1222–1226. doi:10.1213/00000539-199376060-00007
Lam, A. M., Matta, B. F., Mayberg, T. S., and Strebel, S. (1995). Change in cerebral blood flow velocity with onset of EEG silence during inhalation anesthesia in humans: Evidence of flow-metabolism coupling? J. Cereb. Blood Flow. Metab. Off. J. Int. Soc. Cereb. Blood Flow. Metab. 15 (4), 714–717. doi:10.1038/jcbfm.1995.88
Lassen, N. A. (1959). Cerebral blood flow and oxygen consumption in man. Physiol. Rev. 39 (2), 183–238. doi:10.1152/physrev.1959.39.2.183
Liu, S., Shen, J., Li, Y., Wang, J., Wang, J., Xu, J., et al. (2021). EEG power spectral analysis of abnormal cortical activations during REM/NREM sleep in obstructive sleep apnea. Front. Neurol. 12, 643855. doi:10.3389/fneur.2021.643855
Liu, X., Lauer, K. K., Ward, B. D., Li, S. J., and Hudetz, A. G. (2013). Differential effects of deep sedation with propofol on the specific and nonspecific thalamocortical systems A functional magnetic resonance imaging study. Anesthesiol. Hagerst. 118, 59–69. doi:10.1097/ALN.0b013e318277a801
Liu, X., Pillay, S., Li, R., Vizuete, J. A., Pechman, K. R., Schmainda, K. M., et al. (2013). Multiphasic modification of intrinsic functional connectivity of the rat brain during increasing levels of propofol. NeuroImage 83, 581–592. doi:10.1016/j.neuroimage.2013.07.003
Liu, X., Zhu, X. H., Zhang, Y., and Chen, W. (2011). Neural origin of spontaneous hemodynamic fluctuations in rats under burst-suppression anesthesia condition. Cereb. Cortex N. Y. N. 21 (2), 374–384. doi:10.1093/cercor/bhq105
Logothetis, N. K. (2002). The neural basis of the blood-oxygen-level-dependent functional magnetic resonance imaging signal. Philos. Trans. R. Soc. B Biol. Sci. 357 (1424), 1003–1037. doi:10.1098/rstb.2002.1114
Ludbrook, G. L., Visco, E., and Lam, A. M. (2002). Propofol: Relation between brain concentrations, electroencephalogram, middle cerebral artery blood flow velocity, and cerebral oxygen extraction during induction of anesthesia. Anesthesiology 97 (6), 1363–1370. doi:10.1097/00000542-200212000-00006
Lutz, L. J., Milde, J. H., and Milde, L. N. (1990). The cerebral functional, metabolic, and hemodynamic effects of desflurane in dogs. Anesthesiology 73 (1), 125–131. doi:10.1097/00000542-199007000-00018
Ma, K., and Bebawy, J. F. (2022). Electroencephalographic burst-suppression, perioperative neuroprotection, postoperative cognitive function, and mortality: A focused narrative review of the literature. Anesth. Analg. 135 (1), 79–90. doi:10.1213/ANE.0000000000005806
Machado, M. F., Muela, H. C. S., Costa-Hong, V. A., Yassuda, M. S., Moraes, N. C., Memoria, C. M., et al. (2021). Cerebral autoregulation in hypertensive patients: A review. Interv. Cardiol. 0 (0), 87.
Maekawa, T., Tommasino, C., Shapiro, H. M., Keifer-Goodman, J., and Kohlenberger, R. W. (1986). Local cerebral blood flow and glucose utilization during isoflurane anesthesia in the rat. Anesthesiology 65 (2), 144–151. doi:10.1097/00000542-198608000-00003
Mäkiranta, M. J., Jauhiainen, J. P. T., Oikarinen, J. T., Suominen, K., Tervonen, O., Alahuhta, S., et al. (2002). Functional magnetic resonance imaging of swine brain during change in thiopental anesthesia into EEG burst-suppression level--a preliminary study. Magma N. Y. N. 15 (1–3), 27–35. doi:10.1007/BF02693841
Matta, B. F., Heath, K. J., Tipping, K., and Summors, A. C. (1999). Direct cerebral vasodilatory effects of sevoflurane and isoflurane. Anesthesiology 91, 677–680. doi:10.1097/00000542-199909000-00019
Matta, B. F., and Lam, A. M. (1995). Nitrous oxide increases cerebral blood flow velocity during pharmacologically induced EEG silence in humans. J. Neurosurg. Anesthesiol. 7 (2), 89–93. doi:10.1097/00008506-199504000-00003
Matta, B. F., Lam, A. M., Strebel, S., and Mayberg, T. S. (1995). Cerebral pressure autoregulation and carbon dioxide reactivity during propofol-induced EEG suppression. Br. J. Anaesth. 74 (2), 159–163. doi:10.1093/bja/74.2.159
Matta, B. F., Mayberg, T. S., and Lam, A. M. (1995). Direct cerebrovasodilatory effects of halothane, isoflurane, and desflurane during propofol-induced isoelectric electroencephalogram in humans. Anesthesiology 83 (5), 980–985. discussion 27A. doi:10.1097/00000542-199511000-00011
Michenfelder, J. D. (1974). The interdependency of cerebral functional and metabolic effects following massive doses of thiopental in the dog. Anesthesiology 41 (3), 231–236. doi:10.1097/00000542-197409000-00004
Milde, L. N., Milde, J. H., and Michenfelder, J. D. (1985). Cerebral functional, metabolic, and hemodynamic effects of etomidate in dogs. Anesthesiology 63 (4), 371–377. doi:10.1097/00000542-198510000-00005
Mirsattari, S. M., Sharpe, M. D., and Young, G. B. (2004). Treatment of refractory status epilepticus with inhalational anesthetic agents isoflurane and desflurane. Arch. Neurol. 61 (8), 1254–1259. doi:10.1001/archneur.61.8.1254
Mount, C. A., and Das, M. J. (2022). “Cerebral perfusion pressure,” in StatPearls (Treasure Island (FL): StatPearls Publishing). [Internet]. [cited 2023 Mar 18]. Available from: http://www.ncbi.nlm.nih.gov/books/NBK537271/.
Nemoto, E. M., Klementavicius, R., Melick, J. A., and Yonas, H. (1996). Suppression of cerebral metabolic rate for oxygen (CMRO2) by mild hypothermia compared with thiopental. J. Neurosurg. Anesthesiol. 8 (1), 52–59. doi:10.1097/00008506-199601000-00012
Nemoto, E. M. K. R., Klementavicius, R., Melick, J. A., and Yonas, H. (1996). Norepinephrine activation of basal cerebral metabolic rate for oxygen (CMRO2) during hypothermia in rats. Anesth. Analg. 83, 1262–1267. doi:10.1097/00000539-199612000-00023
Newberg, L. A., Milde, J. H., and Michenfelder, J. D. (1983). The cerebral metabolic effects of isoflurane at and above concentrations that suppress cortical electrical activity. Anesthesiology 59 (1), 23–28. doi:10.1097/00000542-198307000-00005
Newman, M. F., Murkin, J. M., Roach, G., Croughwell, N. D., White, W. D., Clements, F. M., et al. (1995). Cerebral physiologic effects of burst suppression doses of propofol during nonpulsatile cardiopulmonary bypass. CNS Subgroup of McSPI. Anesth. Analg. 81 (3), 452–457. doi:10.1097/00000539-199509000-00004
Obrist, W. D., Thompson, H. K., Wang, H. S., and Wilkinson, W. E. (1975). Regional cerebral blood flow estimated by 133-xenon inhalation. Stroke 6 (3), 245–256. doi:10.1161/01.str.6.3.245
Ramani, R., Todd, M. M., and Warner, D. S. (1992). A dose-response study of the influence of propofol on cerebral blood flow, metabolism and the electroencephalogram in the rabbit. J. Neurosurg. Anesthesiol. 4 (2), 110–119. doi:10.1097/00008506-199204000-00006
Reinsfelt, B., Westerlind, A., Houltz, E., Ederberg, S., Elam, M., and Ricksten, S. E. (2003). The effects of isoflurane-induced electroencephalographic burst suppression on cerebral blood flow velocity and cerebral oxygen extraction during cardiopulmonary bypass. Anesth. Analg. 97 (5), 1246–1250. doi:10.1213/01.ane.0000086732.97924.be
Reinsfelt, B., Westerlind, A., and Ricksten, S. E. (2011). The effects of sevoflurane on cerebral blood flow autoregulation and flow-metabolism coupling during cardiopulmonary bypass. Acta Anaesthesiol. Scand. 55 (1), 118–123. doi:10.1111/j.1399-6576.2010.02324.x
Roald, O. K., Forsman, M., Heier, M. S., and Steen, P. A. (1991). Cerebral effects of nitrous oxide when added to low and high concentrations of isoflurane in the dog. Anesth. analgesia 72, 75–79. doi:10.1213/00000539-199101000-00013
Ruesch, A., Smith, M. A., Wollstein, G., Sigal, I. A., Nelson, S., and Kainerstorfer, J. M. (2017). Correlation between cerebral hemodynamic and perfusion pressure changes in non-human primates. Proc. SPIE-- Int. Soc. Opt. Eng. 10059, 100591P. doi:10.1117/12.2252550
Sahinovic, M. M., Struys, M. M. R. F., and Absalom, A. R. (2018). Clinical pharmacokinetics and pharmacodynamics of propofol. Clin. Pharmacokinet. 57 (12), 1539–1558. doi:10.1007/s40262-018-0672-3
Sainbhi, A. S., Froese, L., Gomez, A., Batson, C., Stein, K. Y., Alizadeh, A., et al. (2021). Continuous time-domain cerebrovascular reactivity metrics and discriminate capacity for the upper and lower limits of autoregulation: A scoping review of the animal literature. Neurotrauma Rep. 2 (1), 639–659. doi:10.1089/neur.2021.0043
Saunders, P. A., Ito, Y., Baker, M. L., Hume, A. S., and Ho, I. K. (1990). Pentobarbital tolerance and withdrawal: Correlation with effects on the GABAA receptor. Pharmacol. Biochem. Behav. 37 (2), 343–348. doi:10.1016/0091-3057(90)90346-j
Scheller, M. S., Todd, M. M., and Drummond, J. C. (1986). Isoflurane, halothane, and regional cerebral blood flow at various levels of PaCO2 in rabbits. Anesthesiology 64 (5), 598–604. doi:10.1097/00000542-198605000-00009
Schmid-Elsaesser, R., Schröder, M., Zausinger, S., Hungerhuber, E., Baethmann, A., and Reulen, H. J. (1999). EEG burst suppression is not necessary for maximum barbiturate protection in transient focal cerebral ischemia in the rat. J. Neurol. Sci. 162 (1), 14–19. doi:10.1016/s0022-510x(98)00300-1
Shanker, A., Abel, J. H., Schamberg, G., and Brown, E. N. (2021). Etiology of burst suppression EEG patterns. Front. Psychol. 12, 673529. doi:10.3389/fpsyg.2021.673529
Shin, H. J., Yang, G. Y., and Kim, Y. Z. (2018). Clinical advantage of propofol compared with barbiturate for the coma therapy in the patients with severe traumatic brain injury. J. Neurointensive Care 1 (1), 32–39. doi:10.32587/jnic.2018.00010
Sirmpilatze, N., Mylius, J., Ortiz-Rios, M., Baudewig, J., Paasonen, J., Golkowski, D., et al. (2022). “Spatial signatures of anesthesia-induced burst-suppression differ between primates and rodents,” in eLife. Editors M. Meng, T. R. Makin, S. Sasai, and C. Faber, 11, e74813.
Slupe, A. M., and Kirsch, J. R. (2018). Effects of anesthesia on cerebral blood flow, metabolism, and neuroprotection. J. Cereb. Blood Flow. Metab. 38 (12), 2192–2208. doi:10.1177/0271678X18789273
Sutin, J., Chang, C., Boas, D., Brown, E., and Franceschini, M. A. (2014). “Diffuse optical spectroscopy measurement of cerebral hemodynamics and oxygen metabolism during anesthesia-induced burst suppression in rats,” in Biomedical optics (Optica Publishing Group). paper BT5B3 [Internet] [cited 2023 Mar 9]. p. BT5B.3. Available from: https://opg.optica.org/abstract.cfm?uri=BIOMED-2014-BT5B.3.
Tameem, A., and Krovvidi, H. (2013). Cerebral physiology. Contin. Educ. Anaesth. Crit. Care Pain 13 (4), 113–118. doi:10.1093/bjaceaccp/mkt001
Tiecks, F. P., Lam, A. M., Aaslid, R., and Newell, D. W. (1995). Comparison of static and dynamic cerebral autoregulation measurements. Stroke 26 (6), 1014–1019. doi:10.1161/01.str.26.6.1014
Tricco, A. C., Lillie, E., Zarin, W., O’Brien, K. K., Colquhoun, H., Levac, D., et al. (2018). PRISMA extension for scoping reviews (PRISMA-ScR): Checklist and explanation. Ann. Intern Med. 169 (7), 467–473. doi:10.7326/M18-0850
Venkat, P., Chopp, M., and Chen, J. (2016). New insights into coupling and uncoupling of cerebral blood flow and metabolism in the brain. Croat. Med. J. 57 (3), 223–228. doi:10.3325/cmj.2016.57.223
Veselis, R. A., Feshchenko, V. A., Reinsel, R. A., Beattie, B., and Akhurst, T. J. (2005). Propofol and thiopental do not interfere with regional cerebral blood flow response at sedative concentrations. Anesthesiology 102 (1), 26–34. doi:10.1097/00000542-200501000-00008
Walter, B., Eiselt, M., Cumming, P., Xiong, G., Hinz, R., Uthe, S., et al. (2013). Resistance of brain glucose metabolism to thiopental-induced CNS depression in newborn piglets. Int. J. Dev. Neurosci. Off. J. Int. Soc. Dev. Neurosci. 31 (3), 157–164. doi:10.1016/j.ijdevneu.2012.12.008
Wang, M., Agarwal, S., Mayevsky, A., and Joshi, S. (2011). Optically measured NADH concentrations are unaffected by propofol induced EEG silence during transient cerebral hypoperfusion in anesthetized rabbits. Brain Res. 1396, 69–76. doi:10.1016/j.brainres.2011.04.002
Warner, D. S., Hansen, T. D., Vust, L., and Todd, M. M. (1989). Distribution of cerebral blood flow during deep isoflurane vs. pentobarbital anesthesia in rats with middle cerebral artery occlusion. J. Neurosurg. Anesthesiol. 1 (3), 219–226. doi:10.1097/00008506-198909000-00003
Werner, C., Hoffman, W. E., Kochs, E., Albrecht, R. F., and Am Esch, J. S. (1992). The effects of propofol on cerebral blood flow in correlation to cerebral blood flow velocity in dogs. J. Neurosurg. Anesthesiol. 4 (1), 41–46. doi:10.1097/00008506-199201000-00008
Werner, C., Hoffman, W. E., Kochs, E., and Albrecht, R. F. (1995). Transcranial Doppler sonography indicates critical brain perfusion during hemorrhagic hypotension in dogs. Anesth. Analg. 81 (6), 1203–1207. doi:10.1097/00000539-199512000-00015
Westermaier, T., Zausinger, S., Baethmann, A., Steiger, H. J., and Schmid-Elsaesser, R. (2000). No additional neuroprotection provided by barbiturate-induced burst suppression under mild hypothermic conditions in rats subjected to reversible focal ischemia. J. Neurosurg. 93 (5), 835–844. doi:10.3171/jns.2000.93.5.0835
Williams, L. M., Boyd, K. L., and Fitzgerald, B. M. (2022). “Etomidate,” in StatPearls (Treasure Island (FL): StatPearls Publishing). [cited 2023 Mar 18]. Available from: http://www.ncbi.nlm.nih.gov/books/NBK535364/.
Woodcock, T. E., Murkin, J. M., Farrar, J. K., Tweed, W. A., Guiraudon, G. M., and McKenzie, F. N. (1987). Pharmacologic EEG suppression during cardiopulmonary bypass: Cerebral hemodynamic and metabolic effects of thiopental or isoflurane during hypothermia and normothermia. Anesthesiology 67 (2), 218–224. doi:10.1097/00000542-198708000-00011
Yang, J., Ruesch, A., Schmitt, S., Smith, M. A., and Kainerstorfer, J. M. (2018). “Correlation of EEG with intercranial pressure and cerebral hemodynamics during burst-supression,” in Biophotonics congress: Biomedical optics congress 2018 (Optica Publishing Group). Microscopy/Translational/Brain/OTS) (2018), paper JTu3A40 [Internet] [cited 2023 Mar 9]. p. JTu3A.40. Available from: https://opg.optica.org/abstract.cfm?uri=Translational-2018-JTu3A.40.
Young, W. L., Prohovnik, I., Correll, J. W., Ostapkovich, N., and Ornstein, E. (1991). Thiopental effect on cerebral blood flow during carotid endarterectomy. J. Neurosurg. Anesthesiol. 3, 265–269. doi:10.1097/00008506-199112000-00004
Young, Y., Menon, D. K., Tisavipat, N., Matta, B. F., and Jones, J. G. (1997). Propofol neuroprotection in a rat model of ischaemia reperfusion injury. Eur. J. Anaesthesiol. 14 (3), 320–326. doi:10.1046/j.1365-2346.1997.00130.x
Zanatta, P., Toffolo, G. M., Sartori, E., Bet, A., Baldanzi, F., Agarwal, N., et al. (2013). The human brain pacemaker: Synchronized infra-slow neurovascular coupling in patients undergoing non-pulsatile cardiopulmonary bypass. NeuroImage 72, 10–19. doi:10.1016/j.neuroimage.2013.01.033
Zarchin, N., Guggenheimer-Furman, E., Meilin, S., Ornstein, E., and Mayevsky, A. (1998). Thiopental induced cerebral protection during ischemia in gerbils. Brain Res. 780 (2), 230–236. doi:10.1016/s0006-8993(97)01188-8
Zeiler, F. A., Akoth, E., Gillman, L. M., and West, M. (2017). Burst suppression for ICP control. J. Intensive Care Med. 32 (2), 130–139. doi:10.1177/0885066615593939
Zhang, Z., Cai, D. C., Wang, Z., Zeljic, K., Wang, Z., and Wang, Y. (2019). Isoflurane-induced burst suppression increases intrinsic functional connectivity of the monkey brain. Front. Neurosci. 13, 296. doi:10.3389/fnins.2019.00296
Keywords: cerebral blood flow (CBF), cerebrovascular physiology, cerebral autoregulation (CA), neuroanaesthesia, systematic review, burst suppression
Citation: Siddiqi AZ, Froese L, Gomez A, Sainbhi AS, Stein K, Park K, Vakitbilir N and Zeiler FA (2023) The effect of burst suppression on cerebral blood flow and autoregulation: a scoping review of the human and animal literature. Front. Physiol. 14:1204874. doi: 10.3389/fphys.2023.1204874
Received: 12 April 2023; Accepted: 25 May 2023;
Published: 07 June 2023.
Edited by:
Ye Zeng, Sichuan University, ChinaReviewed by:
Vladislav Toronov, Ryerson University, CanadaCopyright © 2023 Siddiqi, Froese, Gomez, Sainbhi, Stein, Park, Vakitbilir and Zeiler. This is an open-access article distributed under the terms of the Creative Commons Attribution License (CC BY). The use, distribution or reproduction in other forums is permitted, provided the original author(s) and the copyright owner(s) are credited and that the original publication in this journal is cited, in accordance with accepted academic practice. No use, distribution or reproduction is permitted which does not comply with these terms.
*Correspondence: A. Zohaib Siddiqi, c2lkZGlxaWFAbXl1bWFuaXRvYmEuY2E=
†ORCID: A. Zohaib Siddiqi, https://orcid.org/0000-0002-5228-6376; Logan Froese, https://orcid.org/0000-0002-6076-0189; Alwyn Gomez, https://orcid.org/0000-0002-3737-2065; Amanjyot Singh Sainbhi, https://orcid.org/0000-0003-3231-5683; Nuray Vakitbilir, https://orcid.org/0000-0003-2764-145X; Frederick A. Zeiler, https://orcid.org/0000-0003-1737-0510
Disclaimer: All claims expressed in this article are solely those of the authors and do not necessarily represent those of their affiliated organizations, or those of the publisher, the editors and the reviewers. Any product that may be evaluated in this article or claim that may be made by its manufacturer is not guaranteed or endorsed by the publisher.
Research integrity at Frontiers
Learn more about the work of our research integrity team to safeguard the quality of each article we publish.