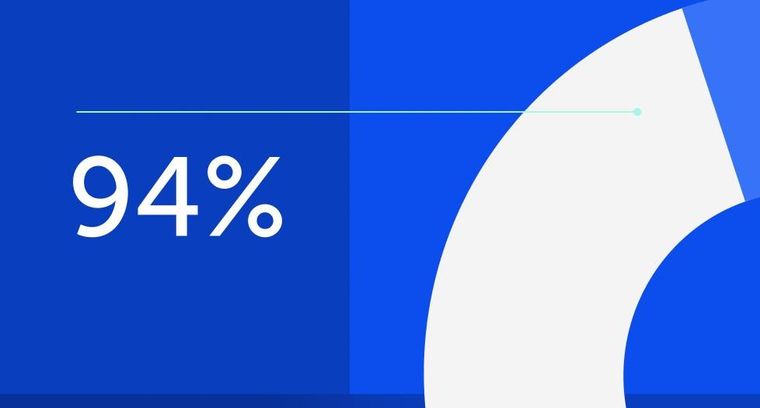
94% of researchers rate our articles as excellent or good
Learn more about the work of our research integrity team to safeguard the quality of each article we publish.
Find out more
ORIGINAL RESEARCH article
Front. Physiol., 20 July 2023
Sec. Developmental Physiology
Volume 14 - 2023 | https://doi.org/10.3389/fphys.2023.1201699
This article is part of the Research TopicWomen in Developmental Physiology: 2022View all 7 articles
Introduction: Novel therapeutics are emerging to mitigate damage from perinatal brain injury (PBI). Few newborns with PBI suffer from a singular etiology. Most experience cumulative insults from prenatal inflammation, genetic and epigenetic vulnerability, toxins (opioids, other drug exposures, environmental exposure), hypoxia-ischemia, and postnatal stressors such as sepsis and seizures. Accordingly, tailoring of emerging therapeutic regimens with endogenous repair or neuro-immunomodulatory agents for individuals requires a more precise understanding of ligand, receptor-, and non-receptor-mediated regulation of essential developmental hormones. Given the recent clinical focus on neurorepair for PBI, we hypothesized that there would be injury-induced changes in erythropoietin (EPO), erythropoietin receptor (EPOR), melatonin receptor (MLTR), NAD-dependent deacetylase sirtuin-1 (SIRT1) signaling, and hypoxia inducible factors (HIF1α, HIF2α). Specifically, we predicted that EPO, EPOR, MLTR1, SIRT1, HIF1α and HIF2α alterations after chorioamnionitis (CHORIO) would reflect relative changes observed in human preterm infants. Similarly, we expected unique developmental regulation after injury that would reveal potential clues to mechanisms and timing of inflammatory and oxidative injury after CHORIO that could inform future therapeutic development to treat PBI.
Methods: To induce CHORIO, a laparotomy was performed on embryonic day 18 (E18) in rats with transient uterine artery occlusion plus intra-amniotic injection of lipopolysaccharide (LPS). Placentae and fetal brains were collected at 24 h. Brains were also collected on postnatal day 2 (P2), P7, and P21. EPO, EPOR, MLTR1, SIRT1, HIF1α and HIF2α levels were quantified using a clinical electrochemiluminescent biomarker platform, qPCR, and/or RNAscope. MLT levels were quantified with liquid chromatography mass spectrometry.
Results: Examination of EPO, EPOR, and MLTR1 at 24 h showed that while placental levels of EPO and MLTR1 mRNA were decreased acutely after CHORIO, cerebral levels of EPO, EPOR and MLTR1 mRNA were increased compared to control. Notably, CHORIO brains at P2 were SIRT1 mRNA deficient with increased HIF1α and HIF2α despite normalized levels of EPO, EPOR and MLTR1, and in the presence of elevated serum EPO levels. Uniquely, brain levels of EPO, EPOR and MLTR1 shifted at P7 and P21, with prominent CHORIO-induced changes in mRNA expression. Reductions at P21 were concomitant with increased serum EPO levels in CHORIO rats compared to controls and variable MLT levels.
Discussion: These data reveal that commensurate with robust inflammation through the maternal placental-fetal axis, CHORIO impacts EPO, MLT, SIRT1, and HIF signal transduction defined by dynamic changes in EPO, EPOR, MLTR1, SIRT1, HIF1α and HIF2α mRNA, and EPO protein. Notably, ligand-receptor mismatch, tissue compartment differential regulation, and non-receptor-mediated signaling highlight the importance, complexity and nuance of neural and immune cell development and provide essential clues to mechanisms of injury in PBI. As the placenta, immune cells, and neural cells share many common, developmentally regulated signal transduction pathways, further studies are needed to clarify the perinatal dynamics of EPO and MLT signaling and to capitalize on therapies that target endogenous neurorepair mechanisms.
Perinatal brain injury (PBI) is a leading cause of long-term morbidity and neurodevelopmental impairment in children (Kochanek et al., 2012; Novak et al., 2018). In recent years, modern medical advances in neonatal and perinatal critical care have improved the survival rate of preterm infants at earlier gestational ages (Larroque et al., 2008; Kyser et al., 2012). Despite this, concomitant advances in neuroscience have failed to effectively reduce the burden of disability associated with brain injury in this patient population. Indeed, more than half of those infants surviving PBI have chronic neurological conditions such as cerebral palsy, cognitive delay, epilepsy, sensory-motor disability, and deficits of attention (Volpe, 2009a; Nosarti et al., 2012; Anderson, 2014; Fant et al., 2014). Thus, the continued refinement of our understanding of the pathophysiological mechanisms of brain and neural-immune injury in infants is essential. Similarly, the identification of new strategies for neural repair and restoration, including therapies directed at improving developmental homeostasis and immunomodulation, is required. (Hodnett et al., 2010; Chen et al., 2016).
For a large proportion of infants with PBI, central nervous system (CNS) injury begins in utero with inflammation/infection (chorioamnionitis/CHORIO) and/or hypoxia-ischemia. CHORIO is the most common abnormality found in placentae from very preterm infants (Lee J. et al., 2013; Lee SM. et al., 2013; Chau et al., 2014). The placental dysfunction caused by CHORIO affects the entire maternal-placental-fetal axis (Redline, 2009). CHORIO affects placental permeability and blood flow, facilitating hypoxia-ischemia and transmission of inflammation to fetuses of all gestational ages. Indeed, the placental-fetal-brain axis plays an important role in neural development, and alterations or disruptions lead to chronic neurological deficits (Dammann and Leviton, 1997; Dammann and Leviton, 2014; Fant et al., 2014; Williams et al., 2017). In preterm infants, PBI can be diffuse and is known as encephalopathy of prematurity (Volpe, 2009b). PBI leads to impaired structural and functional connectivity in developing neural networks, and changes in brain network topology resulting in multiple motor, cognitive, and neuropsychiatric disabilities (Counsell et al., 2008) and lasting neural-immune injury (Lin et al., 2010; Huggard et al., 2020; Kitase et al., 2021).
Inflammation throughout the placental-fetal-brain axis and neural-immune aberrations after CHORIO are prominent factors in the pathophysiology of PBI. The associated signal transduction and specific molecules have been identified as therapeutic targets (Robinson et al., 2018a; Jantzie et al., 2018; Yellowhair et al., 2018; Yellowhair et al., 2019a; Yellowhair et al., 2019b; Kitase et al., 2021; Gall et al., 2022). For example, therapies such as erythropoietin (EPO) and melatonin (MLT) have been studied individually, and as a cocktail, in preterm infants (Yawno et al., 2017; Robinson et al., 2018a; Robinson et al., 2018b; Lee et al., 2019; Juul et al., 2020; Song et al., 2021; Wood et al., 2021; Jantzie et al., 2022). As monotherapy, both have been investigated in clinical trials to ameliorate CNS injury (Juul et al., 2008; Ohls et al., 2014; Fauchère et al., 2015; Ohls et al., 2016; Wu et al., 2016; Carloni et al., 2017). Like EPO, MLT has favorable pharmacokinetics and an acceptable safety profile (Buscemi et al., 2006; Gitto et al., 2013; Merchant et al., 2013; Andersen et al., 2016; Carloni et al., 2017). In clinical trials for preterm infants, MLT has been used for neuroprotection and neonatal sepsis (El-Kabbany et al., 2020; Garofoli et al., 2021).
EPO is an important molecule for neurorepair and immunomodulation as it has anti-apoptotic, antioxidant, and anti-inflammatory properties (Villa et al., 2003; Kumral et al., 2004; Maiese et al., 2008; Juul et al., 2009), alongside cytoprotective effects on endothelial cells, glial cells, and neurons (Mazur et al., 2010; Xiong et al., 2011; Gonzalez et al., 2013; Jantzie et al., 2015a; Ott et al., 2015). EPO affects neuronal differentiation (Park et al., 2006), neuroblast migration (Tsai et al., 2006), oligodendrogenesis (Iwai et al., 2010; Mazur et al., 2010; Jantzie et al., 2013), oligodendrocyte maturation (Sugawa et al., 2002; Mazur et al., 2010; Jantzie et al., 2013), myelination (Mazur et al., 2010; Jantzie et al., 2013; Jantzie et al., 2014a; Jantzie et al., 2016; Jantzie et al., 2018), and axon health (Hellewell et al., 2013; Jantzie et al., 2014b). EPO receptors (EPOR) play an important and critical role in development and tissue repair after injury (Mazur et al., 2010; Jantzie et al., 2019). Present in the brain early in gestation, EPO and EPOR are localized to neurons, oligodendrocytes, astrocytes, and choroid plexus. Both ligand and receptor expression persist after birth (Juul et al., 1999). After PBI, endogenous EPO levels transiently increase (Teramo and Widness, 2009; Logan et al., 2014; Sweetman et al., 2017; Teramo et al., 2018). Similarly, there can be a sustained upregulation of EPOR on many cells (Assaraf et al., 2007; Mazur et al., 2010; Messier and Ohls, 2014; Robinson et al., 2016). However, brain injury during development can persistently increase EPOR, without a sustained increase in EPO ligand production (Mazur et al., 2010). This mismatch can lead to cell death. MLT similarly has protective properties with antioxidant, anti-inflammatory, free radical scavenging and anti-apoptotic effects through receptor-mediated signaling, and non-receptor-mediated actions in brain, placenta, and immune cells. (Reiter et al., 2010; Tosini et al., 2014; Tarocco et al., 2019). Preterm infants, however, are at a developmental disadvantage in terms of its supply (Kennaway et al., 1992; Kennaway et al., 1996; Acuna-Castroviejo et al., 2014; Biran et al., 2019). MLT is synthesized at higher concentrations in the placenta than the pineal gland (Okatani et al., 1998; Man et al., 2017), and infants born before 34 weeks have lower blood levels of melatonin than those of mature infants, in part due to loss of placental sources. (Kennaway et al., 1992; Kennaway et al., 1996; Acuna-Castroviejo et al., 2014; Biran et al., 2019). Indeed, the rhythmic secretion of pineal MLT usually occurs around 2–3 months of age in full-term newborns (Commentz et al., 1996; Jan et al., 2007), but MLT secretion in preterm infants remains delayed and persists until 8–9 months of age (Biran et al., 2014; Biran et al., 2019; Ejaz et al., 2020)
In the present study, we evaluated EPO and MLT signaling throughout the placental-fetal-brain axis and modulation by CHORIO. We also evaluated SIRT1, HIF1α and HIF2α as upstream signaling partners and molecular convergence points between EPO and MLT pathways. (Chavez et al., 2006; Hou et al., 2011; Chen et al., 2012; Jantzie et al., 2022). We aimed to define changes in the expression of these important developmental regulators in the placenta-fetal-brain axis following injury. To accomplish this, we used an established model of CHORIO in rats (Jantzie et al., 2014a; Maxwell et al., 2015; Jantzie et al., 2018; Yellowhair et al., 2018; Yellowhair et al., 2019a; Yellowhair et al., 2019b; Kitase et al., 2021; Jantzie et al., 2022). This model yields cerebral palsy-like deficits in the mature CNS that mimic those of preterm survivors, with white matter injury, gliosis, spastic-like gait, poor social interaction, and cognitive impairment (Jantzie et al., 2014a; Maxwell et al., 2015; Jantzie et al., 2018; Yellowhair et al., 2018; Yellowhair et al., 2019a; Yellowhair et al., 2019b; Kitase et al., 2021; Jantzie et al., 2022). This model encompasses the fetal and systemic inflammatory response syndromes and placental pathology common in preterm infants with CHORIO, including acutely elevated IL-1β, TNF-α, IL-6, IL-10, and CXCL1 in serum and placental neutrophilia (Jantzie et al., 2014a; Maxwell et al., 2015; Yellowhair et al., 2018; Yellowhair et al., 2019a; Yellowhair et al., 2019b). The systemic inflammation associated with CHORIO causes PBMC hyper-reactivity at P7 (term-equivalent human age), P21 (toddler-equivalent human age), and throughout adulthood concomitant with profound immune dysregulation and changes in immune population dynamics throughout the lifespan (Yellowhair et al., 2018; Yellowhair et al., 2019a; Kitase et al., 2021). We hypothesized that there would be relative EPO and MLT expression changes after CHORIO that would mirror EPO and MLT fluctuations and alterations observed in human preterm infants including increases in EPO after injury, and relative melatonin and HIF2α deficiency. Similarly, we expected unique developmental regulation after injury that would reveal potential clues to the mechanisms, timing, and treatment of PBI.
Sprague Dawley rat dams and litters were maintained in a temperature- and humidity-controlled facility with food and water available ad libitum. Animals were subject to a 12-h dark/light cycle, with lights on at 0800 h. All experiments were performed in strict accordance with protocols approved by the institutional Animal Care and Use Committee (ACUC) at the Johns Hopkins University School of Medicine. Protocols were developed and performed consistent with National Research Council and ARRIVE guidelines (Kilkenny et al., 2010).
Pregnant Sprague Dawley rats underwent laparotomy on embryonic day 18 (E18) as previously reported (Jantzie et al., 2013; Jantzie et al., 2014a; Jantzie et al., 2014b; Jantzie et al., 2015a; Jantzie et al., 2015b; Maxwell et al., 2015; Jantzie et al., 2018). Under isoflurane anesthesia, uterine arteries were transiently occluded for 60 min to induce temporary placental insufficiency. Subsequently, an intra-amniotic injection of lipopolysaccharide (LPS 0111: B4, 4 μg/sac; Sigma-Aldrich, St. Louis, MO, United states) was administered to each amniotic sac, and the laparotomy was closed. The dam was allowed to recover and rat pups were born on embryonic day 22 (E22). Sham animals underwent a laparotomy (no uterine artery occlusion and no intra-amniotic LPS injection) with an equivalent duration of anesthesia. For each experiment described, the data represents true n (individual rats) from at least 4 different dams per condition. Balanced numbers of male and female offspring were used in every outcome measure.
Blood was collected on postnatal day (P) 2, P7, and P21 as a terminal procedure and centrifuged at 6,000 g for 15 min at 4°C. Serum was then removed and stored at −80°C for later analysis. Care was taken to avoid freeze-thaw cycles (Mitchell et al., 2005; Yellowhair et al., 2018).
Placentae and forebrain were harvested at E19 (24 h following induction of CHORIO). Forebrain was also collected at P2, P7, and P21. Gene-of-interest primers and cDNA synthesized from 0.9 μg RNA were added to PowerUp™ SYBR™ Green Master Mix (Thermo Fisher Scientific, Waltham, MA), and run in triplicate on a Quant Studio™ 3 Real-Time PCR System (Thermo Fisher Scientific, Waltham, MA). To standardize transcripts from samples between experiments, cycle thresholds from samples of interest were compared to cycle threshold values from pooled E19 naïve samples, with gene-of-interest transcription normalized to ribosomal 18s endogenous control as previously published (Jantzie et al., 2013; Jantzie et al., 2014a; Jantzie et al., 2014b; Jantzie et al., 2015a). Primer sequences were verified with the Basic Local Alignment Search Tool (BLAST) for Nucleotides on the U.S. National Center for Biotechnology Information (NCBI) website. Primer sequences for EPO, EPO receptor (EPOR), Melatonin receptor 1 (MLTR1), Sirtuin 1 (SIRT1), HIF1α, HIF2α and 18S are listed in Table 1 (all from IDT Technologies, Coralville, Iowa). To ensure rigor, experimental replicates were run in triplicate and those cycle threshold (CT) values varying by greater than 0.25 standard deviations were excluded from all analyses.
To localize single mRNA molecules of EPO, HIF1α, and HIF2α, RNAscope was performed on 12 μm paraformaldehyde-fixed frozen brain slices at the time points listed above. For each experiment, we used one positive (Rat Polr2a), and one negative (E. coli DapB) control probe, and probes against the mRNA of interest. In Situ hybridization (ISH) was performed according to manufacturer’s specifications and the RNAscope Multiplex Fluorescent Reagent Kit V2 protocol (ACD Biosciences, Newark, CA). Signal was detected with Opal 570. Confocal z stacks were acquired by blinded observers in the somatosensory cortex at the level of the dorsal hippocampus using a Nikon ECLIPSE Ti2 microscope (Tokyo, Japan) with a C2 system (variable emission confocal system coupled to a GaAsp detector). Gain parameters, zoom, pinhole size, step size, scan speed and resolution were uniform across scans.
Immunostaining for EPO was performed as previously described. (Mazur et al., 2010; Jantzie et al., 2013; Jantzie et al., 2014a; Jantzie et al., 2016; Jantzie et al., 2019). Specifically, rats were deeply anesthetized with ketamine and xylazine and perfused with 4% paraformaldehyde. Brains were then collected, and post-fixed in paraformaldehyde. After immersion in 30% sucrose solution, 50 μm, frozen, slide mounted, coronal sections were obtained and collected using a cryostat (Leica, Buffalo Grove, IL, United States). Slides were then washed and incubated with 0.5% Triton X, followed by blocking solution containing 10% normal goat serum in phosphate buffered solution (PBS). Primary antibodies against EPO protein (Epo clone B-4 sc-5290, Santa Cruz Biotechnology, 1:50, CA, United States), in blocking solution containing 0.5% Trition-X100 were incubated on sections overnight at 4°C. The next day, sections were rinsed, and incubated with species-appropriate biotinylated secondary antibodies for 1 h. This was followed by incubation in Fluorescein Avidin D (Vector Labolatories, CA, United States) for 1 h. Slides were then washed and coverslipped with ProlongTM Gold antifade reagent containing DAPI (Thermo Fisher Scientific, MA, United States).
Serum EPO analyses were performed using an R-PLEX assay for the detection of rat EPO (R-PLEX Rat F231G, Meso Scale Diagnostics, Rockville, MD, United States). In accordance with the manufacturer’s specifications and as previously described (Maxwell et al., 2015; Robinson et al., 2016; Robinson et al., 2018b; Newville et al., 2020), serum was diluted to 1:2 and then loaded in duplicate with prepared standard onto a blocked and washed small-spot 96-well plate. EPO levels in brain and placentae were measured in the same manner after being adjusted to 300 µg tissue load (Yellowhair et al., 2019a; Robinson et al., 2018b; Robinson et al., 2016; Maxwell et al., 2015; Dammann et al., 2016; Kuban et al., 2015; Leviton et al., 2011; O'Shea et al., 2012). Plates were analyzed with a Quickplex SQ 120.
Proteins from serum were precipitated by the addition of 200 μL of LCMS grade acetonitrile to 50 μL of serum followed by vigorous vortexing for 30 s and centrifugation at 13,000 x g for 15 min at 4°C. The supernatant was pipetted off and dried with vacuum centrifugation (SpeedVac). The dried extract was resuspended in 20 μL of LCMS grade methanol followed by water bath sonication for 5 min at room temperature. The solution was again centrifuged at 13,000 x g for 15 min at 4°C and the supernatant was transferred to tapered autosampler vials for LCMS analysis.
Absolute quantification of melatonin was performed using a Dionex U3000 UHPLC system coupled to a Q Exactive “Classic” Mass Spectrometer using a 7-min gradient of 0.1% formic acid in LCMS grade water (Buffer A) and 0.1% formic acid in LCMS grade acetonitrile (Buffer B). All buffers were purchased from Fisher Scientific. A Poroshell 120 EC-18 2.1 × 50 mm column with 1.9 µm particles (Agilent) was used to separation with a consistent flowrate of 400 μL/min across the column maintained at 40°C. The gradient ramped from 5% B to 45% B in 3 min with a rapid ramp to 98% B in 0.5 min where it remained for 0.5 min before returning to baseline conditions for the remainder of the gradient. Eluting molecules were directly ionized into the Q Exactive at 3,500 V with a capillary temperature of 262°C and an accessory probe temperature of 425°C. Sheath gas and auxiliary gas pressures were set at 50 and 12.5 arbitrary units, respectively. A two-step method utilizing one full scan and one parallel reaction monitoring (PRM) scan were performed with the latter centered on the mass 233.1 with an isolation window of 2 Th. For the MS1 scan, all ions from 150 to 500 m/z were collected with a target of 3 × 106 charges or a maximum injection time of 50 milliseconds at a resolution of 17,500 at m/z 200. For the PRM 2 × 105 charges were acquired or a maximum of 50 milliseconds attempting to reach that charge number. All collected ions were fragmented at 20 eV.
The Thermo.RAW files were processed in the freely available Xcalibur QuanBrowser software (Thermo Fisher) with the area under the curve extracted for the PRM fragment ion of 233.1, 174.0915 using a 10 part per million (PPM) mass extraction window and a 15 s extraction window around the target elution time of 2.15 min. For concentration calculation, the area under the curve was compared to a serial dilution of melatonin obtained from the Johns Hopkins Hospital pharmacy diluted in methanol to an approximate concentration range of 1 picogram–60 picogram on column. A linear regression was automatically performed by the program and peaks were manually adjusted only in the case of obvious failures of the program to correctly integrate peak baselines.
Data are represented as mean ± standard error of the mean (SEM). Data were tested for normality with the Shapiro-Wilk Test. Parametric statistical differences between two groups were established with a t-test, and non-parametric differences between two groups were established with a Mann-Whitney U-test. GraphPad Prism 9.3.1 software was used for statistical analysis.
The first stage of our investigation involved the assessment of EPO, EPOR, and MLTR1 mRNA, along with EPO protein expression in the placenta. In placentae at E19, 24 h following CHORIO, EPO mRNA was significantly decreased compared to sham (sham: 0.26 ± 0.10, n = 11, CHORIO: 0.10 ± 0.05, n = 10, p < 0.05, Mann-Whitney U-test) (Figure 1A). Interestingly, there was no companion alteration in EPO protein expression (sham: 1.19 ± 0.31 pg/mL, n = 6, CHORIO: 1.47 ± 0.34 pg/mL, n = 6, n.s., t-test) or EPOR mRNA expression (Figure 1B). Like EPO mRNA, assessment of MLTR1 mRNA expression in the placenta revealed a significant decrease following CHORIO compared to sham (sham: 1.87 ± 0.75, n = 10, CHORIO: 0.08 ± 0.02, n = 8, p < 0.001; Figure 1C, Mann-Whitney U-test). Acute reductions in these key signaling molecules is commensurate with profound inflammation from CHORIO (Jantzie et al., 2014a; Maxwell et al., 2015; Yellowhair et al., 2018; Yellowhair et al., 2019a; Yellowhair et al., 2019b; Kitase et al., 2021; Gall et al., 2022; Jantzie et al., 2022).
FIGURE 1. CHORIO decreases EPO and MLTR1 mRNA in placentae at E19. (A) In CHORIO placentae, EPO mRNA was significantly decreased compared to sham (Mann-Whitney U-test). (B) There was no significant difference between sham and CHORIO in EPOR mRNA (Mann-Whitney U-test). (C) MLTR1 mRNA levels were significantly lower in CHORIO placentae compared to sham (Mann-Whitney U-test). (*p < 0.05, ***p < 0.001).
After assessing the placenta 24 h after the induction of CHORIO, we next examined the fetal brain at the same timepoint. Evaluation of E19 brains revealed significant increases in EPO and EPOR mRNA in CHORIO compared to sham (EPO sham: 0.65 ± 0.36, n = 6, CHORIO: 3.73 ± 0.46, n = 10, p < 0.001, Mann-Whitney U-test, Figure 2A; EPOR, sham: 0.70 ± 0.32, n = 6, CHORIO: 3.38 ± 0.44, n = 10, p < 0.001, Mann-Whitney U-test; Figure 2B). Notably, no cerebral increase in EPO protein was observed (sham: 0.43 ± 0.10 pg/mL, n = 9, CHORIO: 0.41 ± 0.19 pg/mL, n = 7, n.s., t-test). However, cerebral MLTR1 levels were also significantly increased in CHORIO brains compared to sham controls (sham: 0.64 ± 0.28, n = 6, CHORIO: 3.98 ± 0.49, n = 9, p < 0.001; Figure 2C, t-test). Unlike the increases in EPO, EPOR and MLTR1 mRNA, no significant change in SIRT1, HIF1α, HIF2α mRNA were observed in CHORIO brains compared to sham (Figures 2D–F). These data highlight the tissue-specific changes that occur in the acute period after CHORIO, with differential expression between the placenta and the fetal brain.
FIGURE 2. CHORIO markedly increases of EPO, EPOR, and MTLR1 mRNA in the fetal brain at E19. (A, B) EPO and EPOR mRNA levels were significantly elevated in CHORIO animals compared to sham (Mann-Whitney U-test). (C) MLTR1 mRNA levels in CHORIO brains was also significantly elevated compared to sham (t-test). (D–F) There was no significant differences in SIRT1, HIF1α, and HIF2α mRNA between sham and CHORIO. (***p < 0.001).
After assessing acute changes in EPO, EPOR, MLTR1, SIRT1, HIF1α and HIF2α in the fetal brain, we expanded our time course to the postnatal period from P2-P21 to assess cerebral injury-induced mRNA expression dynamics. At P2, a preterm equivalent time point approximately 5 days after CHORIO, EPO, EPOR, and MLTR1 mRNA expression were all stable and unchanged between CHORIO and sham groups. SIRT1 mRNA expression in the brain, however, was significantly decreased (sham: 0.82 ± 0.05, n = 6, CHORIO: 0.64 ± 0.02, n = 7, p < 0.01, Figure 3D, t-test) with increased HIF1α (sham: 1.18 ± 0.13, n = 8, CHORIO: 1.58 ± 0.08, n = 7, p < 0.05, Figure 3E, t-test) and HIF2α mRNA (sham: 1.45 ± 0.09, n = 8, CHORIO: 1.85 ± 0.09, n = 7, p < 0.05, Figure 3F, t-test) in CHORIO brains compared to sham.
FIGURE 3. CHORIO Induces SIRT-1 Deficiency, and increases HIF1α and HIF2α at P2 but does not induce changes of EPO, EPOR, or MTLR1 mRNA. (A–C) CHORIO does not induce significant alterations in EPO, EPOR, nor MLTR1 mRNA in the P2 CHORIO brain compared to sham (Mann-Whitney U-test). (D) SIRT1 mRNA expression is reduced in CHORIO brains compared to sham controls (t-test). (E, F) HIF1α and HIF2α mRNA levels is elevated in CHORIO brains compared to sham (t-test) (*p < 0.05, **p < 0.01).
Extension of the time course to P7, a term equivalent time point, revealed increased EPO mRNA in the brains of CHORIO rats compared to sham (sham: 1.64 ± 0.57, n = 13, CHORIO: 3.59 ± 0.48, n = 10, p < 0.05, Figure 4A, Mann-Whitney U-test). This occurred without changes in EPOR, MLTR1, SIRT1, and HIF1α mRNA expression (Figures 4B–E). However, HIF2α mRNA expression was reduced in CHORIO brains compared to sham controls (sham: 4.82 ± 0.77, n = 6, CHORIO: 1.93 ± 0.30, n = 8, p < 0.01, Figure 4F, t-test). To add rigor, localize regional differences, and to examine dynamic cell-specific expression of the mRNAs, we performed RNAscope. Assessment of individual mRNAs revealed widespread increases in EPO with CHORIO, in the presence of decreased HIF2α throughout the somatosensory cortex (Figure 5). Consistent with previous publications, EPO protein was similarly expressed on neurons and oligodendrocytes with changes in expression level dependent on brain region and injury response (Figure 6).
FIGURE 4. CHORIO differentially changes cerebral EPO and HIF2α levels at P7. (A) EPO mRNA was significantly increased in CHORIO brains compared to sham (Mann-Whitney U-test). (B–E) There was no significant difference between sham and CHORIO in EPOR, MLTR1, SIRT1 and HIF1α mRNA (B) Mann-Whitney U-test, (C–E) t-test). (F) CHORIO decreased HIF2α mRNA compared to sham (t-test) (*p < 0.05, **p < 0.01).
FIGURE 5. CHORIO increases EPO and decreases HIF2α mRNA spatial and temporal expression. Consistent with qPCR results at P7, a term-equivalent time point, RNAscope assays confirm changes in spatial and termporal localization of EPO mRNA with injury. Specifically, EPO mRNA is increased in the cortex of CHORIO brains compared to sham (Top Row). On the other hand, temporal and spatial localization of HIF1α mRNA does not differ between sham and CHORIO brains (Middle Row). However, HIF2α mRNA expression is deficient CHORIO brains compared to sham (Bottom Row). (Scale bars 100 μm and 10 μm inset).
FIGURE 6. CHORIO increases EPO protein expression in the P7 brain. At P7, a term equivalent time point, EPO protein is increased in the hippocampus of CHORIO animals compared to sham consistent with expression level changes dependent on brain region, neural cell and injury response (Scale bars are 50 μm and 10 μm inset).
Notably at P21, a toddler equivalent time point, the pattern shifted considerably. Compared to sham, EPO mRNA (sham: 4.48 ± 0.64, n = 5, CHORIO: 2.77 ± 0.34, n = 7, p < 0.05, Mann-Whitney U-test, Figure 7A) and EPOR mRNA (sham: 4.88 ± 0.62, n = 5, CHORIO: 2.53 ± 0.22, n = 7, p < 0.01, t-test, Figure 7B) were significantly decreased in CHORIO brains. These changes were concomitant with decreased cerebral MLTR1 mRNA expression in CHORIO pups compared to sham (sham: 6.28 ± 0.70, n = 5, CHORIO: 3.03 ± 0.21, n = 7, p < 0.001, Figure 7C, t-test) and persistently reduced HIF2α mRNA expression (sham:12.34 ± 1.79, n = 7, CHORIO: 8.19 ± 0.58, n = 7, p < 0.05, Figure 7F, t-test). Thus, over the developmental time course from the third trimester through infancy and toddler stages of development, brain mRNA expression increases and then declines in the setting of CHORIO, emphasizing the complex pattern of changes in the injured, developing brain.
FIGURE 7. CHORIO causes profound decreases in cerebral EPO, EPOR, MLTR1, and HIF2α mRNA at P21. (A, B) EPO and EPOR mRNA levels were significantly decreased in CHORIO brains compared to sham (A) t-test, (B) Mann-Whitney U-test). (C) These changes were concomitant with decreased MLTR1 levels (t-test). (D, E) SIRT1 and mRNA showed no significant difference between sham and CHORIO (t-test). (D, E) SIRT1 and HIF1α mRNA showed no significant difference between sham and CHORIO (t-test). (F) HIF2α mRNA levels in CHORIO brains was significantly reduced compared to sham (t-test) (*p < 0.05, **p < 0.01 ***p < 0.001).
Given the compartment-specific, and tissue-specific regulation of these key hormones, we next evaluated EPO and MLT protein expression in the serum. Unlike what was observed in placenta and brain tissue, serum EPO protein levels were markedly elevated across the developmental time course. Specifically, serum EPO protein was significantly higher in CHORIO animals at P2 compared to sham (sham: 22.3 ± 4.5 pg/mL, n = 13, CHORIO: 51.1 ± 10.9 pg/mL, n = 6, p < 0.01, Figure 8A). At P7, serum EPO levels in controls were elevated compared to P2 (P2: 22.3 ± 4.49 pg/mL, n = 13, P7: 74.33 ± 13.7 pg/mL, n = 20, p < 0.01, Mann-Whitney U-test), despite no observed significant difference between sham and CHORIO (Figure 8B). Interestingly, serum EPO levels were significantly higher in CHORIO pups at P21 compared to sham (sham: 40.0 ± 6.7 pg/mL, n = 7, CHORIO: 68.1 ± 9.4 pg/mL, n = 8, p < 0.05, Mann-Whitney U-test, Figure 8C) mirroring the CHORIO-induced increase at P2 and uniquely opposite to brain EPO mRNA expression at the same time point. Consistent with the clinical literature (Kennaway et al., 1992; Kennaway et al., 1996; Biran et al., 2019; Perrone et al., 2023), MLT levels were variable among individual animals independent of injury (161.5 ± 70.2 pg/mL, n = 12 vs. 87.4 ± 25.8 pg/mL, n = 21, p = ns, Mann-Whitney U-test, Figure 9). In summary, serum EPO and MLT levels do not necessarily reflect the complex pattern of mRNA expression noted in end organ brain tissue.
FIGURE 8. CHORIO Changes Serum EPO Levels. (A) Serum levels of EPO protein was significantly higher in CHORIO animals at P2 compared to sham (Mann-Whitney U-test). (B) By P7, there were no differences between sham and CHORIO groups apparent (Mann-Whitney U-test). (C) At P21, the EPO serum protein in CHORIO was again significantly higher than sham (Mann-Whitney U-test). (*p < 0.05, **p < 0.01).
FIGURE 9. Melatonin levels in serum are variable between animals and treatment groups. As measured by liquid chromatography mass spectrometry, MLT levels were variable among individual animals independent of injury status.
Melatonin and erythropoietin are unique, developmentally regulated endogenous hormones essential to many body systems. Here, we studied changes throughout the placental-fetal-brain axis along an extended developmental time course. Our results delineate the complex, developmental, ligand-receptor-mediated, and tissue-specific regulation of these neuroimmunomodulatory molecules. Using an established preclinical model of PBI secondary to CHORIO with robust inflammation through the placenta-fetal brain axis (Maxwell et al., 2015; Robinson et al., 2018a; Jantzie et al., 2018; Yellowhair et al., 2019a; Kitase et al., 2021; Gall et al., 2022), we found that CHORIO also confers a sustained impact on EPO, EPOR, MLTR1, and HIF2α mRNA and protein from late gestation through early childhood. Notably, EPO and MLT signaling is multifaceted, and context dependent. We have previously shown that EPO/EPOR signaling is dependent on cell type and defined by developmental timing and injury response (Mazur et al., 2010; Jantzie et al., 2013; Jantzie et al., 2014a; Jantzie et al., 2015a; Jantzie et al., 2016). During neurodevelopment, EPO has multiple critical roles for most lineages including neurons (Tsai et al., 2006; Fernandez Garcia-Agudo et al., 2021), oligodendrocytes (Iwai et al., 2010; Jantzie et al., 2013), astrocytes (Tang et al., 2013), microglia and pericytes (Mazur et al., 2010; Fernandez Garcia-Agudo et al., 2021). Importantly, EPO’s protective properties require adequate SIRT1 expression (Hou et al., 2011; Wang et al., 2013; Li et al., 2019). Specifically, conditional mutants with SIRT1 knock-down fail to respond to exogenous EPO as wild-type animals do after injury. (Wang et al., 2013; Robinson and Jantzie, 2022).
MLT also acts on SIRT1 to increase its levels and shifts hypoxia-inducible factor (HIF) from HIF1α to HIF2α (Kudová et al., 2016). SIRT1 deficiency contributes to dysregulated HIF2α (hypoxia-inducible factor-2 alpha) (Chen et al., 2012), and HIF2α drives astrocytic production of EPO during neurodevelopment and immune maturation (Chavez et al., 2006; Semenza, 2019). During an acute hypoxic challenge, HIF1α and HIF2α functions overlap (Semenza, 2019; Packer, 2020; Packer, 2021). In contrast, in the setting of chronic inflammation, as reported in children with cerebral palsy (Lin et al., 2010; Zareen et al., 2020a; Zareen et al., 2020b), HIF1α and HIF2α functions diverge markedly. The shift to HIF2α contributes to immune cell health because HIF1α promotes toxicity, fibrosis, and inflammatory responses, while HIF2α provides nutritional and anti-inflammatory support (Packer, 2020; Robinson and Jantzie, 2022). Our data support that CHORIO induces a SIRT-1 deficiency at a preterm equivalent age concomitant with distinct, acuity-dependent EPO ligand- EPO receptor mRNA mismatch after CHORIO with accompanying changes in HIF2α. Specifically, we report deficient HIF2α mRNA expression at term equivalent (P7) and toddler equivalent (P21) developmental timepoints supporting longer term alterations in the setting of ongoing inflammation. We also report differential circulating levels of EPO and MLT in the serum compared to brain emphasizing the importance of both tissue levels and serum expression when evaluating injury and therapeutic response (Juul et al., 2020; Wood et al., 2021). Interestingly, MLT-SIRT1-HIF2α deficiency may contribute to the attenuated effect of exogenous EPO seen in preterm infants, and it is possible that increasing SIRT1 levels could allow for exogenous MLT to synergize with exogenous EPO to promote recovery after early brain damage (Robinson et al., 2018a; Jantzie et al., 2018; Robinson and Jantzie, 2022).
The data presented herein emphasizes the importance of EPO ligand-receptor mismatch and the increased demand for MLT following CHORIO. In addition to the changes in EPO, EPOR, SIRT1, HIF1α, and HIF2α observed, we also found that CHORIO induced placental reductions in EPO and MLTR1 mRNA expression. This was distinct from the time-dependent changes in cerebral MLTR1 mRNA expression. Maternal MLT plays a key role in fetal growth and in the early stages of neurodevelopment (Conway et al., 1997; Yu et al., 2017). Typical receptors for MLT regulation of circadian rhythm include MLTR1 and MLTR2 (Dubocovich, 2007; Zlotos et al., 2014), which are classical G-protein-linked receptors that inhibit adenylate cyclase (Zlotos et al., 2014). Placental trophoblasts synthesize melatonin and express its receptors (Lanoix et al., 2008). In placenta, MLTR1 receptors are involved in the regulation of apoptosis (Soliman et al., 2015). In the fetal brain, MLTR1 has been found in the leptomeninges, cerebellum, thalamus, hypothalamus, and brainstem (Thomas et al., 2002). Moreover, MLTR1 is found in the hippocampus, frontal cortex, and other brain regions that are important for cognition and memory (Ekmekcioglu, 2006). Although melatonin plays a key role in development, preterm infants are at a developmental disadvantage in terms of its supply (Muñoz-Hoyos et al., 2007; Merchant et al., 2013; Biran et al., 2019). MLT is synthesized in higher concentrations within the placenta and numerous other locations compared to the pineal gland (Okatani et al., 1998; Man et al., 2017; McCarthy et al., 2019). The rhythmic secretion of endogenous melatonin usually appears around 2–3 months of age after full-term birth (Commentz et al., 1996; Jan et al., 2007). However, infants born before 34 weeks have lower blood levels of melatonin (Biran et al., 2019), and melatonin secretion in preterm infants is delayed (Biran et al., 2014). We found CHORIO increases MLTR1 mRNA expression at E19, with subsequent decreases at P21, corresponding to human toddler age. This lag in recovery of MLTR1 levels suggests that MLT, when used for neurorepair in the developing CNS, is likely needed for an extended period to cover periods of developmental fluctuation, the multiple phases of PBI pathophysiology and innate recovery. Indeed, therapeutic strategies that maintain brain EPO, EPOR, and MLT homeostasis together may be needed as part of an extended regimen to achieve lasting brain protection. Notably, white matter injury in preterm infants progresses over an extended time course with concomitant gliosis and sustained elevations in key systemic inflammatory proteins throughout the lifespan with tertiary pathophysiology and permanently altered neural-immune developmental trajectory (Lin et al., 2010; Chau et al., 2014; Yellowhair et al., 2019a; Kitase et al., 2021).
This study is not without its limitations. Melatonin levels are difficult to measure, and LCMS -based evaluation of serum melatonin was used here. Future studies should include expanded time courses and direct brain melatonin measurements. MLT signaling also occurs independent of membrane bound MLTR1, and likely involves nuclear receptors. Since both EPO and MLT signaling occur in the placenta, immune cells, and brain, further studies are needed to elucidate the perinatal immune dynamics of EPO, MLT, SIRT1, and HIF2α signaling and to exploit the potential of endogenous therapeutic agents. The present study was conducted in a genetically homogeneous rodent background after a single stimulus and highlights the need to tailor diagnostic and therapeutic approaches to human infants on an individual basis with attention to initiating insults. In addition, since mRNA may not directly reflect actual protein expression or activity, degradation, phosphorylation, acetylation or other post-translational modification in the context of brain and placental injury, more detailed interpretation of homeostasis after CHORIO would be possible with additional studies of protein dynamics and function.
In conclusion, EPO and MLT drive signaling pathways that enhance sustained neuroimmunomodulation and promote microenvironmental homeostasis in the developing brain. CHORIO induces lasting effects on EPO, MLTR1, SIRT1, and HIF2α mRNA and protein expression suggestive of differential effects on EPO and MLT neurorepair capacity dependent on injury and developmental stage. Maintenance of MLT, SIRT1, and HIF2α homeostasis could modulate endogenous EPO levels and may drive signal transduction that maximizes neurorepair compared to EPO dosing in restricted, acute, and isolated regimens. Given the ligand-receptor mismatch found in preterm infants, and the supplementation of endogenous MLT signaling, the combination of EPO and MLT could be a reasonable and indispensable therapeutic strategy for infants with brain injury, as could therapies that support HIF2α.
The raw data supporting the conclusion of this article will be made available by the authors, without undue reservation.
The animal study was reviewed and approved by the Johns Hopkins University Animal Care and Use Committee.
Conceptualization and design, ShR and LJ; methodology, ShR, YK, BO, and LJ; investigation, NM, SH, SiR, SM, VV, RS, AG, TH, SK, OO, RH, MO, BO, and YK; formal analysis, ShR, YK, BO, and LJ; writing–original draft preparation, YK and LJ; writing–review and editing, All Authors; supervision, LJ; project administration, LJ; funding acquisition, ShR and LJ; approval of the final manuscript, All Authors; correspondence and material requests, LJ. All authors contributed to the article and approved the submitted version.
This work was supported by grants from the National Institutes of Health (NIH), the Cerebral Palsy Alliance Research Foundation (CPARF), and the Rudi Schulte Research Institute (RSRI). Specifically, this work was supported by R01HL139492 to LJ, R56NS060765 to ShR, a CPARF award to LJ and ShR and an RSRI award to LJ and ShR.
The authors declare that the research was conducted in the absence of any commercial or financial relationships that could be construed as a potential conflict of interest.
All claims expressed in this article are solely those of the authors and do not necessarily represent those of their affiliated organizations, or those of the publisher, the editors and the reviewers. Any product that may be evaluated in this article, or claim that may be made by its manufacturer, is not guaranteed or endorsed by the publisher.
Acuna-Castroviejo, D., Escames, G., Venegas, C., Díaz-Casado, M. E., Lima-Cabello, E., López, L. C., et al. (2014). Extrapineal melatonin: Sources, regulation, and potential functions. Cell Mol. Life Sci. 71 (16), 2997–3025. doi:10.1007/s00018-014-1579-2
Andersen, L. P., Gögenur, I., Rosenberg, J., and Reiter, R. J. (2016). The safety of melatonin in humans. Clin. Drug Investig. 36 (3), 169–175. doi:10.1007/s40261-015-0368-5
Anderson, P. J. (2014). Neuropsychological outcomes of children born very preterm. Seminars fetal & neonatal Med. 19 (2), 90–96. doi:10.1016/j.siny.2013.11.012
Assaraf, M. I., Diaz, Z., Liberman, A., Miller, W. H., Arvanitakis, Z., Li, Y., et al. (2007). Brain erythropoietin receptor expression in Alzheimer disease and mild cognitive impairment. J. neuropathology Exp. neurology 66 (5), 389–398. doi:10.1097/nen.0b013e3180517b28
Biran, V., Decobert, F., Bednarek, N., Boizeau, P., Benoist, J. F., Claustrat, B., et al. (2019). Melatonin levels in preterm and term infants and their mothers. Int. J. Mol. Sci. 20 (9), 2077. doi:10.3390/ijms20092077
Biran, V., Phan Duy, A., Decobert, F., Bednarek, N., Alberti, C., and Baud, O. (2014). Is melatonin ready to be used in preterm infants as a neuroprotectant? Dev. Med. child neurology 56 (8), 717–723. doi:10.1111/dmcn.12415
Buscemi, N., Vandermeer, B., Hooton, N., Pandya, R., Tjosvold, L., Hartling, L., et al. (2006). Efficacy and safety of exogenous melatonin for secondary sleep disorders and sleep disorders accompanying sleep restriction: meta-analysis. Bmj 332 (7538), 385–393. doi:10.1136/bmj.38731.532766.F6
Carloni, S., Proietti, F., Rocchi, M., Longini, M., Marseglia, L., D'Angelo, G., et al. (2017). Melatonin pharmacokinetics following oral administration in preterm neonates. Molecules 22 (12), 2115. doi:10.3390/molecules22122115
Chau, V., McFadden, D. E., Poskitt, K. J., and Miller, S. P. (2014). Chorioamnionitis in the pathogenesis of brain injury in preterm infants. Clin. perinatology 41 (1), 83–103. doi:10.1016/j.clp.2013.10.009
Chavez, J. C., Baranova, O., Lin, J., and Pichiule, P. (2006). The transcriptional activator hypoxia inducible factor 2 (HIF-2/EPAS-1) regulates the oxygen-dependent expression of erythropoietin in cortical astrocytes. J. Neurosci. 26 (37), 9471–9481. doi:10.1523/JNEUROSCI.2838-06.2006
Chen, A., Oster, E., and Williams, H. (2016). Why is infant mortality higher in the United States than in europe? Am. Econ. J. Econ. policy 8 (2), 89–124. doi:10.1257/pol.20140224
Chen, R., Xu, M., Hogg, R. T., Li, J., Little, B., Gerard, R. D., et al. (2012). The acetylase/deacetylase couple CREB-binding protein/Sirtuin 1 controls hypoxia-inducible factor 2 signaling. J. Biol. Chem. 287 (36), 30800–30811. doi:10.1074/jbc.M111.244780
Commentz, J. C., Henke, A., Dammann, O., Hellwege, H. H., and Willig, R. P. (1996). Decreasing melatonin and 6-hydroxymelatonin sulfate excretion with advancing gestational age in preterm and term newborn male infants. Eur. J. Endocrinol. 135 (2), 184–187. doi:10.1530/eje.0.1350184
Conway, S., Drew, J. E., Canning, S. J., Barrett, P., Jockers, R., Strosberg, A. D., et al. (1997). Identification of Mel1a melatonin receptors in the human embryonic kidney cell line HEK293: evidence of G protein-coupled melatonin receptors which do not mediate the inhibition of stimulated cyclic AMP levels. FEBS Lett. 407 (1), 121–126. doi:10.1016/s0014-5793(97)00315-3
Counsell, S. J., Edwards, A. D., Chew, A. T., Anjari, M., Dyet, L. E., Srinivasan, L., et al. (2008). Specific relations between neurodevelopmental abilities and white matter microstructure in children born preterm. Brain a J. neurology 131 (Pt 12), 3201–3208. doi:10.1093/brain/awn268
Dammann, O., Allred, E. N., Fichorova, R. N., Kuban, K., O'Shea, T. M., Leviton, A., et al. (2016). Duration of systemic inflammation in the first postnatal month among infants born before the 28th week of gestation. Inflammation 39 (2), 672–677. doi:10.1007/s10753-015-0293-z
Dammann, O., and Leviton, A. (2014). Intermittent or sustained systemic inflammation and the preterm brain. Pediatr. Res. 75 (3), 376–380. doi:10.1038/pr.2013.238
Dammann, O., and Leviton, A. (1997). Maternal intrauterine infection, cytokines, and brain damage in the preterm newborn. Pediatr. Res. 42 (1), 1–8. doi:10.1203/00006450-199707000-00001
Dubocovich, M. L. (2007). Melatonin receptors: Role on sleep and circadian rhythm regulation. Sleep. Med. 8 (Suppl. 3), 34–42. doi:10.1016/j.sleep.2007.10.007
Ejaz, H., Figaro, J. K., Woolner, A. M. F., Thottakam, B. M. V., and Galley, H. F. (2020). Maternal serum melatonin increases during pregnancy and falls immediately after delivery implicating the placenta as a major source of melatonin. Front. Endocrinol. (Lausanne) 11, 623038. doi:10.3389/fendo.2020.623038
Ekmekcioglu, C. (2006). Melatonin receptors in humans: Biological role and clinical relevance. Biomed. Pharmacother. 60 (3), 97–108. doi:10.1016/j.biopha.2006.01.002
El-Kabbany, Z. A., El-Farghali, O. G., Khafagy, S. M., Shaaban, H. A., Osman, H. H. A., and Metwally, M. H. (2020). Melatonin as an adjuvant therapy in preterm infants with neonatal sepsis, randomized trial. Egypt. Pediatr. Assoc. Gaz. 68 (1), 2. doi:10.1186/s43054-019-0013-7
Fant, M. E., Fuentes, J., Kong, X., and Jackman, S. (2014). The nexus of prematurity, birth defects, and intrauterine growth restriction: A role for plac1-regulated pathways. Front. Pediatr. 2, 8. doi:10.3389/fped.2014.00008
Fauchère, J. C., Koller, B. M., Tschopp, A., Dame, C., Ruegger, C., Bucher, H. U., et al. (2015). Safety of early high-dose recombinant erythropoietin for neuroprotection in very preterm infants. J. Pediatr. 167 (1), 52–57.e1-3-3. doi:10.1016/j.jpeds.2015.02.052
Fernandez Garcia-Agudo, L., Steixner-Kumar, A. A., Curto, Y., Barnkothe, N., Hassouna, I., Jähne, S., et al. (2021). Brain erythropoietin fine-tunes a counterbalance between neurodifferentiation and microglia in the adult hippocampus. Cell Rep. 36 (8), 109548. doi:10.1016/j.celrep.2021.109548
Gall, A. R., Amoah, S., Kitase, Y., and Jantzie, L. L. (2022). Placental mediated mechanisms of perinatal brain injury: Evolving inflammation and exosomes. Exp. Neurol. 347, 113914. doi:10.1016/j.expneurol.2021.113914
Garofoli, F., Longo, S., Pisoni, C., Accorsi, P., Angelini, M., Aversa, S., et al. (2021). Oral melatonin as a new tool for neuroprotection in preterm newborns: Study protocol for a randomized controlled trial. Trials 22 (1), 82. doi:10.1186/s13063-021-05034-w
Gitto, E., Marseglia, L., Manti, S., D'Angelo, G., Barberi, I., Salpietro, C., et al. (2013). Protective role of melatonin in neonatal diseases. Oxid. Med. Cell Longev. 2013, 980374. doi:10.1155/2013/980374
Gonzalez, F. F., Larpthaveesarp, A., McQuillen, P., Derugin, N., Wendland, M., Spadafora, R., et al. (2013). Erythropoietin increases neurogenesis and oligodendrogliosis of subventricular zone precursor cells after neonatal stroke. Stroke 44 (3), 753–758. doi:10.1161/strokeaha.111.000104
Hellewell, S. C., Yan, E. B., Alwis, D. S., Bye, N., and Morganti-Kossmann, M. C. (2013). Erythropoietin improves motor and cognitive deficit, axonal pathology, and neuroinflammation in a combined model of diffuse traumatic brain injury and hypoxia, in association with upregulation of the erythropoietin receptor. J. Neuroinflammation 10, 156. doi:10.1186/1742-2094-10-156
Hodnett, E. D., Fredericks, S., and Weston, J. (2010). Support during pregnancy for women at increased risk of low birthweight babies. Cochrane database Syst. Rev. (6), Cd000198. doi:10.1002/14651858.CD000198.pub2
Hou, J., Wang, S., Shang, Y. C., Chong, Z. Z., and Maiese, K. (2011). Erythropoietin employs cell longevity pathways of SIRT1 to foster endothelial vascular integrity during oxidant stress. Curr. Neurovasc Res. 8 (3), 220–235. doi:10.2174/156720211796558069
Hsu, T. S., Lin, Y. L., Wang, Y. A., Mo, S. T., Chi, P. Y., Lai, A. C. Y., et al. (2020). HIF-2α is indispensable for regulatory T cell function. Nat. Commun. 11 (1), 5005. doi:10.1038/s41467-020-18731-y
Huggard, D., Kelly, L., Ryan, E., McGrane, F., Lagan, N., Roche, E., et al. (2020). Increased systemic inflammation in children with Down syndrome. Cytokine 127, 154938. doi:10.1016/j.cyto.2019.154938
Iwai, M., Stetler, R. A., Xing, J., Hu, X., Gao, Y., Zhang, W., et al. (2010). Enhanced oligodendrogenesis and recovery of neurological function by erythropoietin after neonatal hypoxic/ischemic brain injury. Stroke 41 (5), 1032–1037. doi:10.1161/strokeaha.109.570325
Jan, J. E., Wasdell, M. B., Freeman, R. D., and Bax, M. (2007). Evidence supporting the use of melatonin in short gestation infants. J. Pineal Res. 42 (1), 22–27. doi:10.1111/j.1600-079X.2006.00398.x
Jantzie, L., El Demerdash, N., Newville, J. C., and Robinson, S. (2019). Time to reconsider extended erythropoietin treatment for infantile traumatic brain injury? Exp. Neurol. 318, 205–215. doi:10.1016/j.expneurol.2019.05.004
Jantzie, L., Muthukumar, S., Kitase, Y., Vasan, V., Fouda, M. A., Hamimi, S., et al. (2022). Infantile cocktail of erythropoietin and melatonin restores gait in adult rats with preterm brain injury. Dev. Neurosci. 44, 266–276. doi:10.1159/000524394
Jantzie, L. L., Corbett, C. J., Berglass, J., Firl, D. J., Flores, J., Mannix, R., et al. (2014a). Complex pattern of interaction between in utero hypoxia-ischemia and intra-amniotic inflammation disrupts brain development and motor function. J. Neuroinflammation 11, 131. doi:10.1186/1742-2094-11-131
Jantzie, L. L., Corbett, C. J., Firl, D. J., and Robinson, S. (2015a). Postnatal erythropoietin mitigates impaired cerebral cortical development following subplate loss from prenatal hypoxia-ischemia. Cereb. cortex (New York, N. Y. 1991) 25 (9), 2683–2695. doi:10.1093/cercor/bhu066
Jantzie, L. L., Getsy, P. M., Denson, J. L., Firl, D. J., Maxwell, J. R., Rogers, D. A., et al. (2015b). Prenatal hypoxia-ischemia induces abnormalities in CA3 microstructure, potassium chloride Co-transporter 2 expression and inhibitory tone. Front. Cell Neurosci. 9, 347. doi:10.3389/fncel.2015.00347
Jantzie, L. L., Getsy, P. M., Firl, D. J., Wilson, C. G., Miller, R. H., and Robinson, S. (2014b). Erythropoietin attenuates loss of potassium chloride co-transporters following prenatal brain injury. Mol. Cell Neurosci. 61, 152–162. doi:10.1016/j.mcn.2014.06.009
Jantzie, L. L., Miller, R. H., and Robinson, S. (2013). Erythropoietin signaling promotes oligodendrocyte development following prenatal systemic hypoxic-ischemic brain injury. Pediatr. Res. 74 (6), 658–667. doi:10.1038/pr.2013.155
Jantzie, L. L., Oppong, A. Y., Conteh, F. S., Yellowhair, T. R., Kim, J., Fink, G., et al. (2018). Repetitive neonatal erythropoietin and melatonin combinatorial treatment provides sustained repair of functional deficits in a rat model of cerebral palsy. Front. neurology 9, 233. doi:10.3389/fneur.2018.00233
Jantzie, L. L., Winer, J. L., Corbett, C. J., and Robinson, S. (2016). Erythropoietin modulates cerebral and serum degradation products from excess calpain activation following prenatal hypoxia-ischemia. Dev. Neurosci. 38 (1), 15–26. doi:10.1159/000441024
Juul, S. E., Beyer, R. P., Bammler, T. K., McPherson, R. J., Wilkerson, J., and Farin, F. M. (2009). Microarray analysis of high-dose recombinant erythropoietin treatment of unilateral brain injury in neonatal mouse hippocampus. Pediatr. Res. 65 (5), 485–492. doi:10.1203/PDR.0b013e31819d90c8
Juul, S. E., Comstock, B. A., Wadhawan, R., Mayock, D. E., Courtney, S. E., Robinson, T., et al. (2020). A randomized trial of erythropoietin for neuroprotection in preterm infants. N. Engl. J. Med. 382 (3), 233–243. doi:10.1056/NEJMoa1907423
Juul, S. E., McPherson, R. J., Bauer, L. A., Ledbetter, K. J., Gleason, C. A., and Mayock, D. E. (2008). A phase I/II trial of high-dose erythropoietin in extremely low birth weight infants: Pharmacokinetics and safety. Pediatrics 122 (2), 383–391. doi:10.1542/peds.2007-2711
Juul, S. E., Yachnis, A. T., Rojiani, A. M., and Christensen, R. D. (1999). Immunohistochemical localization of erythropoietin and its receptor in the developing human brain. Pediatr. Dev. Pathol. 2 (2), 148–158. doi:10.1007/s100249900103
Kennaway, D. J., Goble, F. C., and Stamp, G. E. (1996). Factors influencing the development of melatonin rhythmicity in humans. J. Clin. Endocrinol. Metab. 81 (4), 1525–1532. doi:10.1210/jcem.81.4.8636362
Kennaway, D. J., Stamp, G. E., and Goble, F. C. (1992). Development of melatonin production in infants and the impact of prematurity. J. Clin. Endocrinol. Metab. 75 (2), 367–369. doi:10.1210/jcem.75.2.1639937
Kilkenny, C., Browne, W. J., Cuthill, I. C., Emerson, M., and Altman, D. G. (2010). Improving bioscience research reporting: The ARRIVE guidelines for reporting animal research. PLoS Biol. 8 (6), e1000412. doi:10.1371/journal.pbio.1000412
Kitase, Y., Chin, E. M., Ramachandra, S., Burkhardt, C., Madurai, N. K., Lenz, C., et al. (2021). Sustained peripheral immune hyper-reactivity (SPIHR): An enduring biomarker of altered inflammatory responses in adult rats after perinatal brain injury. J. Neuroinflammation 18 (1), 242. doi:10.1186/s12974-021-02291-z
Kochanek, K. D., Kirmeyer, S. E., Martin, J. A., Strobino, D. M., and Guyer, B. (2012). Annual summary of vital statistics: 2009. Pediatrics 129 (2), 338–348. doi:10.1542/peds.2011-3435
Kuban, K. C., O'Shea, T. M., Allred, E. N., Fichorova, R. N., Heeren, T., Paneth, N., et al. (2015). The breadth and type of systemic inflammation and the risk of adverse neurological outcomes in extremely low gestation newborns. Pediatr. Neurol. 52 (1), 42–48. doi:10.1016/j.pediatrneurol.2014.10.005
Kudová, J., Vašíček, O., Číž, M., and Kubala, L. (2016). Melatonin promotes cardiomyogenesis of embryonic stem cells via inhibition of HIF-1α stabilization. J. Pineal Res. 61 (4), 493–503. doi:10.1111/jpi.12366
Kumral, A., Baskin, H., Gokmen, N., Yilmaz, O., Genc, K., Genc, S., et al. (2004). Selective inhibition of nitric oxide in hypoxic-ischemic brain model in newborn rats: Is it an explanation for the protective role of erythropoietin? Biol. Neonate 85 (1), 51–54. doi:10.1159/000074958
Kyser, K. L., Morriss, F. H., Bell, E. F., Klein, J. M., and Dagle, J. M. (2012). Improving survival of extremely preterm infants born between 22 and 25 weeks of gestation. Obstetrics Gynecol. 119 (4), 795–800. doi:10.1097/AOG.0b013e31824b1a03
Lanoix, D., Beghdadi, H., Lafond, J., and Vaillancourt, C. (2008). Human placental trophoblasts synthesize melatonin and express its receptors. J. Pineal Res. 45 (1), 50–60. doi:10.1111/j.1600-079X.2008.00555.x
Larroque, B., Ancel, P. Y., Marret, S., Marchand, L., André, M., Arnaud, C., et al. (2008). Neurodevelopmental disabilities and special care of 5-year-old children born before 33 weeks of gestation (the EPIPAGE study): A longitudinal cohort study. Lancet (London, Engl. 371 (9615), 813–820. doi:10.1016/s0140-6736(08)60380-3
Lee, J., Kim, J.-S., Park, J. W., Park, C.-W., Park, J. S., Jun, J. K., et al. (2013a). Chronic chorioamnionitis is the most common placental lesion in late preterm birth. Placenta 34 (8), 681–689. doi:10.1016/j.placenta.2013.04.014
Lee, S. M., Park, J. W., Kim, B. J., Park, C.-W., Park, J. S., Jun, J. K., et al. (2013b). Acute histologic chorioamnionitis is a risk factor for adverse neonatal outcome in late preterm birth after preterm premature rupture of membranes. PloS one 8 (12), e79941. doi:10.1371/journal.pone.0079941
Lee, J. Y., Song, H., Dash, O., Park, M., Shin, N. E., McLane, M. W., et al. (2019). Administration of melatonin for prevention of preterm birth and fetal brain injury associated with premature birth in a mouse model. Am. J. Reprod. Immunol. 82 (3), e13151. doi:10.1111/aji.13151
Leviton, A., Kuban, K. C., Allred, E. N., Fichorova, R. N., O'Shea, T. M., Paneth, N., et al. (2011). Early postnatal blood concentrations of inflammation-related proteins and microcephaly two years later in infants born before the 28th post-menstrual week. Early Hum. Dev. 87 (5), 325–330. doi:10.1016/j.earlhumdev.2011.01.043
Li, K., Liu, T. X., Li, J. F., Ma, Y. R., Liu, M. L., Wang, Y. Q., et al. (2019). rhEPO inhibited cell apoptosis to alleviate acute kidney injury in sepsis by AMPK/SIRT1 activated autophagy. Biochem. Biophys. Res. Commun. 517 (4), 557–565. doi:10.1016/j.bbrc.2019.07.027
Lin, C. Y., Chang, Y. C., Wang, S. T., Lee, T. Y., Lin, C. F., and Huang, C. C. (2010). Altered inflammatory responses in preterm children with cerebral palsy. Ann. neurology 68 (2), 204–212. doi:10.1002/ana.22049
Logan, J. W., Allred, E. N., Fichorova, R. N., Engelke, S., Dammann, O., Leviton, A., et al. (2014). Endogenous erythropoietin varies significantly with inflammation-related proteins in extremely premature newborns. Cytokine 69 (1), 22–28. doi:10.1016/j.cyto.2014.04.009
Maiese, K., Chong, Z. Z., Hou, J., and Shang, Y. C. (2008). Erythropoietin and oxidative stress. Curr. Neurovasc Res. 5 (2), 125–142. doi:10.2174/156720208784310231
Man, G. C. W., Zhang, T., Chen, X., Wang, J., Wu, F., Liu, Y., et al. (2017). The regulations and role of circadian clock and melatonin in uterine receptivity and pregnancy-An immunological perspective. Am. J. Reprod. Immunol. 78 (2), e12715. doi:10.1111/aji.12715
Maxwell, J. R., Denson, J. L., Joste, N. E., Robinson, S., and Jantzie, L. L. (2015). Combined in utero hypoxia-ischemia and lipopolysaccharide administration in rats induces chorioamnionitis and a fetal inflammatory response syndrome. Placenta 36 (12), 1378–1384. doi:10.1016/j.placenta.2015.10.009
Mazur, M., Miller, R. H., and Robinson, S. (2010). Postnatal erythropoietin treatment mitigates neural cell loss after systemic prenatal hypoxic-ischemic injury. J. Neurosurg. Pediatr. 6 (3), 206–221. doi:10.3171/2010.5.Peds1032
McCarthy, R., Jungheim, E. S., Fay, J. C., Bates, K., Herzog, E. D., and England, S. K. (2019). Riding the rhythm of melatonin through pregnancy to deliver on time. Front. Endocrinol. (Lausanne) 10, 616. doi:10.3389/fendo.2019.00616
Merchant, N. M., Azzopardi, D. V., Hawwa, A. F., McElnay, J. C., Middleton, B., Arendt, J., et al. (2013). Pharmacokinetics of melatonin in preterm infants. Br. J. Clin. Pharmacol. 76 (5), 725–733. doi:10.1111/bcp.12092
Messier, A. M., and Ohls, R. K. (2014). Neuroprotective effects of erythropoiesis-stimulating agents in term and preterm neonates. Curr. Opin. Pediatr. 26 (2), 139–145. doi:10.1097/mop.0000000000000077
Mitchell, B. L., Yasui, Y., Li, C. I., Fitzpatrick, A. L., and Lampe, P. D. (2005). Impact of freeze-thaw cycles and storage time on plasma samples used in mass spectrometry based biomarker discovery projects. Cancer Inf. 1 (1), 117693510500100–117693510500104. (In eng). doi:10.1177/117693510500100110
Muñoz-Hoyos, A., Bonillo-Perales, A., Avila-Villegas, R., González-Ripoll, M., Uberos, J., Florido-Navío, J., et al. (2007). Melatonin levels during the first week of life and their relation with the antioxidant response in the perinatal period. Neonatology 92 (3), 209–216. doi:10.1159/000102957
Newville, J., Maxwell, J. R., Kitase, Y., Robinson, S., and Jantzie, L. L. (2020). Perinatal opioid exposure primes the peripheral immune system toward hyperreactivity. Front. Pediatr. 8, 272. doi:10.3389/fped.2020.00272
Nosarti, C., Reichenberg, A., Murray, R. M., Cnattingius, S., Lambe, M. P., Yin, L., et al. (2012). Preterm birth and psychiatric disorders in young adult life. Archives general psychiatry 69 (6), E1–E8. doi:10.1001/archgenpsychiatry.2011.1374
Novak, C. M., Ozen, M., and Burd, I. (2018). Perinatal brain injury: Mechanisms, prevention, and outcomes. Clin. perinatology 45 (2), 357–375. doi:10.1016/j.clp.2018.01.015
O'Shea, T. M., Allred, E. N., Kuban, K. C., Dammann, O., Paneth, N., Fichorova, R., et al. (2012). Elevated concentrations of inflammation-related proteins in postnatal blood predict severe developmental delay at 2 years of age in extremely preterm infants. J. Pediatr. 160 (3), 395–401.e4. doi:10.1016/j.jpeds.2011.08.069
Ohls, R. K., Cannon, D. C., Phillips, J., Caprihan, A., Patel, S., Winter, S., et al. (2016). Preschool assessment of preterm infants treated with darbepoetin and erythropoietin. Pediatrics 137 (3), e20153859. doi:10.1542/peds.2015-3859
Ohls, R. K., Kamath-Rayne, B. D., Christensen, R. D., Wiedmeier, S. E., Rosenberg, A., Fuller, J., et al. (2014). Cognitive outcomes of preterm infants randomized to darbepoetin, erythropoietin, or placebo. Pediatrics 133 (6), 1023–1030. doi:10.1542/peds.2013-4307
Okatani, Y., Okamoto, K., Hayashi, K., Wakatsuki, A., Tamura, S., and Sagara, Y. (1998). Maternal-fetal transfer of melatonin in pregnant women near term. J. Pineal Res. 25 (3), 129–134. doi:10.1111/j.1600-079x.1998.tb00550.x
Ott, C., Martens, H., Hassouna, I., Oliveira, B., Erck, C., Zafeiriou, M. P., et al. (2015). Widespread expression of erythropoietin receptor in brain and its induction by injury. Mol. Med. 21 (1), 803–815. doi:10.2119/molmed.2015.00192
Packer, M. (2021). Mechanisms leading to differential hypoxia-inducible factor signaling in the diabetic kidney: Modulation by SGLT2 inhibitors and hypoxia mimetics. Am. J. Kidney Dis. 77 (2), 280–286. doi:10.1053/j.ajkd.2020.04.016
Packer, M. (2020). Mutual antagonism of hypoxia-inducible factor isoforms in cardiac, vascular, and renal disorders. JACC Basic Transl. Sci. 5 (9), 961–968. doi:10.1016/j.jacbts.2020.05.006
Park, M. H., Lee, S. M., Lee, J. W., Son, D. J., Moon, D. C., Yoon, D. Y., et al. (2006). ERK-mediated production of neurotrophic factors by astrocytes promotes neuronal stem cell differentiation by erythropoietin. Biochem. Biophys. Res. Commun. 339 (4), 1021–1028. doi:10.1016/j.bbrc.2005.10.218
Perrone, S., Romeo, C., Marseglia, L., Manti, S., Rizzo, C., Carloni, S., et al. (2023). Melatonin in newborn infants undergoing surgery: A pilot study on its effects on postoperative oxidative stress. Antioxidants (Basel) 12 (3), 563. doi:10.3390/antiox12030563
Redline, R. W. (2009). Disorders of placental circulation and the fetal brain. Clin. perinatology 36 (3), 549–559. doi:10.1016/j.clp.2009.06.003
Reiter, R. J., Tan, D. X., and Fuentes-Broto, L. (2010). Melatonin: A multitasking molecule. Prog. Brain Res. 181, 127–151. doi:10.1016/s0079-6123(08)81008-4
Robinson, S., Conteh, F. S., Oppong, A. Y., Yellowhair, T. R., Newville, J. C., Demerdash, N. E., et al. (2018a). Extended combined neonatal treatment with erythropoietin plus melatonin prevents posthemorrhagic hydrocephalus of prematurity in rats. Front. Cell Neurosci. 12, 322. doi:10.3389/fncel.2018.00322
Robinson, S., Corbett, C. J., Winer, J. L., Chan, L. A. S., Maxwell, J. R., Anstine, C. V., et al. (2018b). Neonatal erythropoietin mitigates impaired gait, social interaction and diffusion tensor imaging abnormalities in a rat model of prenatal brain injury. Exp. Neurol. 302, 1–13. doi:10.1016/j.expneurol.2017.12.010
Robinson, S., and Jantzie, L. L. (2022). Pathogenesis of posthemorrhagic hydrocephalus of prematurity: New horizons. Semin. Perinatol. 46 (5), :151596. doi:10.1016/j.semperi.2022.151596
Robinson, S., Winer, J. L., Berkner, J., Chan, L. A. S., Denson, J. L., Maxwell, J. R., et al. (2016). Imaging and serum biomarkers reflecting the functional efficacy of extended erythropoietin treatment in rats following infantile traumatic brain injury. J. Neurosurg. Pediatr. 17 (6), 739–755. doi:10.3171/2015.10.Peds15554
Semenza, G. L. (2019). Pharmacologic targeting of hypoxia-inducible factors. Annu. Rev. Pharmacol. Toxicol. 59, 379–403. doi:10.1146/annurev-pharmtox-010818-021637
Soliman, A., Lacasse, A. A., Lanoix, D., Sagrillo-Fagundes, L., Boulard, V., and Vaillancourt, C. (2015). Placental melatonin system is present throughout pregnancy and regulates villous trophoblast differentiation. J. Pineal Res. 59 (1), 38–46. doi:10.1111/jpi.12236
Song, J., Wang, Y., Xu, F., Sun, H., Zhang, X., Xia, L., et al. (2021). Erythropoietin improves poor outcomes in preterm infants with intraventricular hemorrhage. CNS Drugs 35 (6), 681–690. doi:10.1007/s40263-021-00817-w
Sugawa, M., Sakurai, Y., Ishikawa-Ieda, Y., Suzuki, H., and Asou, H. (2002). Effects of erythropoietin on glial cell development; oligodendrocyte maturation and astrocyte proliferation. Neurosci. Res. 44 (4), 391–403. doi:10.1016/s0168-0102(02)00161-x
Sweetman, D. U., Onwuneme, C., Watson, W. R., Murphy, J. F., and Molloy, E. J. (2017). Perinatal asphyxia and erythropoietin and VEGF: Serial serum and cerebrospinal fluid responses. Neonatology 111 (3), 253–259. doi:10.1159/000448702
Tang, Z., Sun, X., Huo, G., Xie, Y., Shi, Q., Chen, S., et al. (2013). Protective effects of erythropoietin on astrocytic swelling after oxygen-glucose deprivation and reoxygenation: Mediation through AQP4 expression and MAPK pathway. Neuropharmacology 67, 8–15. doi:10.1016/j.neuropharm.2012.10.017
Tarocco, A., Caroccia, N., Morciano, G., Wieckowski, M. R., Ancora, G., Garani, G., et al. (2019). Melatonin as a master regulator of cell death and inflammation: Molecular mechanisms and clinical implications for newborn care. Cell Death Dis. 10 (4), 317. doi:10.1038/s41419-019-1556-7
Teramo, K. A., Klemetti, M. M., and Widness, J. A. (2018). Robust increases in erythropoietin production by the hypoxic fetus is a response to protect the brain and other vital organs. Pediatr. Res. 84 (6), 807–812. doi:10.1038/s41390-018-0054-4
Teramo, K. A., and Widness, J. A. (2009). Increased fetal plasma and amniotic fluid erythropoietin concentrations: Markers of intrauterine hypoxia. Neonatology 95 (2), 105–116. doi:10.1159/000153094
Thomas, L., Purvis, C. C., Drew, J. E., Abramovich, D. R., and Williams, L. M. (2002). Melatonin receptors in human fetal brain: 2-[(125)I]iodomelatonin binding and MT1 gene expression. J. Pineal Res. 33 (4), 218–224. doi:10.1034/j.1600-079x.2002.02921.x
Tosini, G., Owino, S., Guillaume, J. L., and Jockers, R. (2014). Understanding melatonin receptor pharmacology: Latest insights from mouse models, and their relevance to human disease. Bioessays 36 (8), 778–787. doi:10.1002/bies.201400017
Tsai, P. T., Ohab, J. J., Kertesz, N., Groszer, M., Matter, C., Gao, J., et al. (2006). A critical role of erythropoietin receptor in neurogenesis and post-stroke recovery. J. Neurosci. 26 (4), 1269–1274. doi:10.1523/jneurosci.4480-05.2006
Villa, P., Bigini, P., Mennini, T., Agnello, D., Laragione, T., Cagnotto, A., et al. (2003). Erythropoietin selectively attenuates cytokine production and inflammation in cerebral ischemia by targeting neuronal apoptosis. J. Exp. Med. 198 (6), 971–975. doi:10.1084/jem.20021067
Volpe, J. J. (2009a). Brain injury in premature infants: A complex amalgam of destructive and developmental disturbances. Lancet Neurology 8 (1), 110–124. doi:10.1016/s1474-4422(08)70294-1
Volpe, J. J. (2009b). The encephalopathy of prematurity--brain injury and impaired brain development inextricably intertwined. Semin. Pediatr. Neurol. 16 (4), 167–178. doi:10.1016/j.spen.2009.09.005
Wang, L., Teng, R., Di, L., Rogers, H., Wu, H., Kopp, J. B., et al. (2013). PPARα and Sirt1 mediate erythropoietin action in increasing metabolic activity and browning of white adipocytes to protect against obesity and metabolic disorders. Diabetes 62 (12), 4122–4131. doi:10.2337/db13-0518
Williams, M., Zhang, Z., Nance, E., Drewes, J. L., Lesniak, W. G., Singh, S., et al. (2017). Maternal inflammation results in altered tryptophan metabolism in rabbit placenta and fetal brain. Dev. Neurosci. 39 (5), 399–412. doi:10.1159/000471509
Wood, T. R., Parikh, P., Comstock, B. A., Law, J. B., Bammler, T. K., Kuban, K. C., et al. (2021). Early biomarkers of hypoxia and inflammation and two-year neurodevelopmental outcomes in the preterm erythropoietin neuroprotection (PENUT) trial. EBioMedicine 72, 103605. doi:10.1016/j.ebiom.2021.103605
Wu, Y. W., Mathur, A. M., Chang, T., McKinstry, R. C., Mulkey, S. B., Mayock, D. E., et al. (2016). High-Dose erythropoietin and hypothermia for hypoxic-ischemic encephalopathy: A phase II trial. Pediatrics 137 (6), e20160191. doi:10.1542/peds.2016-0191
Xiong, Y., Mahmood, A., Zhang, Y., Meng, Y., Zhang, Z. G., Qu, C., et al. (2011). Effects of posttraumatic carbamylated erythropoietin therapy on reducing lesion volume and hippocampal cell loss, enhancing angiogenesis and neurogenesis, and improving functional outcome in rats following traumatic brain injury. J. Neurosurg. 114 (2), 549–559. doi:10.3171/2010.10.Jns10925
Yawno, T., Mahen, M., Li, J., Fahey, M. C., Jenkin, G., and Miller, S. L. (2017). The beneficial effects of melatonin administration following hypoxia-ischemia in preterm fetal sheep. Front. Cell Neurosci. 11, 296. doi:10.3389/fncel.2017.00296
Yellowhair, T. R., Newville, J. C., Noor, S., Maxwell, J. R., Milligan, E. D., Robinson, S., et al. (2019b). CXCR2 blockade mitigates neural cell injury following preclinical chorioamnionitis. Front. Physiol. 10, 324. doi:10.3389/fphys.2019.00324
Yellowhair, T. R., Noor, S., Mares, B., Jose, C., Newville, J. C., Maxwell, J. R., et al. (2019a). Chorioamnionitis in rats precipitates extended postnatal inflammatory lymphocyte hyperreactivity. Dev. Neurosci. 40, 523–533. doi:10.1159/000497273
Yellowhair, T. R., Noor, S., Maxwell, J. R., Anstine, C. V., Oppong, A. Y., Robinson, S., et al. (2018). Preclinical chorioamnionitis dysregulates CXCL1/CXCR2 signaling throughout the placental-fetal-brain axis. Exp. Neurol. 301 (Pt B), 110–119. doi:10.1016/j.expneurol.2017.11.002
Yu, X., Li, Z., Zheng, H., Ho, J., Chan, M. T., and Wu, W. K. (2017). Protective roles of melatonin in central nervous system diseases by regulation of neural stem cells. Cell Prolif. 50 (2), e12323. doi:10.1111/cpr.12323
Zareen, Z., Strickland, T., Eneaney, V. M., Kelly, L. A., McDonald, D., Sweetman, D., et al. (2020a). Cytokine dysregulation persists in childhood post Neonatal Encephalopathy. BMC Neurol. 20 (1), 115. doi:10.1186/s12883-020-01656-w
Zareen, Z., Strickland, T., Fallah, L., McEneaney, V., Kelly, L., McDonald, D., et al. (2020b). Cytokine dysregulation in children with cerebral palsy. Dev. Med. child neurology 63, 407–412. doi:10.1111/dmcn.14724
Keywords: inflammation, perinatal brain injury, neurorepair, placenta, neural-immune, neuroimmunomodulation
Citation: Kitase Y, Madurai NK, Hamimi S, Hellinger RL, Odukoya OA, Ramachandra S, Muthukumar S, Vasan V, Sevensky R, Kirk SE, Gall A, Heck T, Ozen M, Orsburn BC, Robinson S and Jantzie LL (2023) Chorioamnionitis disrupts erythropoietin and melatonin homeostasis through the placental-fetal-brain axis during critical developmental periods. Front. Physiol. 14:1201699. doi: 10.3389/fphys.2023.1201699
Received: 06 April 2023; Accepted: 06 July 2023;
Published: 20 July 2023.
Edited by:
Casey A. Mueller, California State University San Marcos, United StatesReviewed by:
Robert Roghair, The University of Iowa, United StatesCopyright © 2023 Kitase, Madurai, Hamimi, Hellinger, Odukoya, Ramachandra, Muthukumar, Vasan, Sevensky, Kirk, Gall, Heck, Ozen, Orsburn, Robinson and Jantzie. This is an open-access article distributed under the terms of the Creative Commons Attribution License (CC BY). The use, distribution or reproduction in other forums is permitted, provided the original author(s) and the copyright owner(s) are credited and that the original publication in this journal is cited, in accordance with accepted academic practice. No use, distribution or reproduction is permitted which does not comply with these terms.
*Correspondence: Lauren L. Jantzie, bGphbnR6aWVAamhtaS5lZHU=
Disclaimer: All claims expressed in this article are solely those of the authors and do not necessarily represent those of their affiliated organizations, or those of the publisher, the editors and the reviewers. Any product that may be evaluated in this article or claim that may be made by its manufacturer is not guaranteed or endorsed by the publisher.
Research integrity at Frontiers
Learn more about the work of our research integrity team to safeguard the quality of each article we publish.