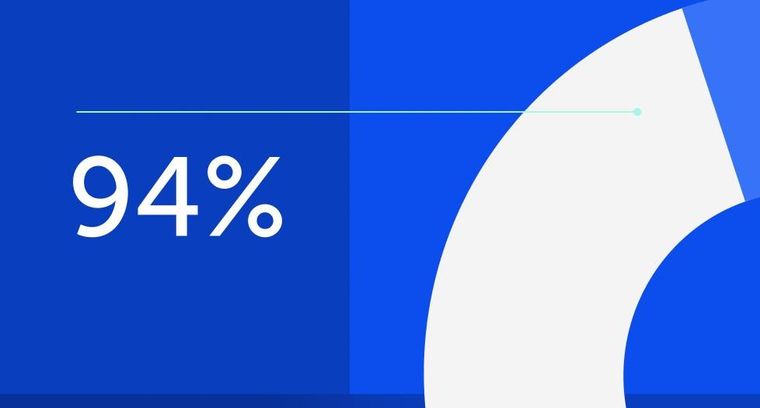
94% of researchers rate our articles as excellent or good
Learn more about the work of our research integrity team to safeguard the quality of each article we publish.
Find out more
MINI REVIEW article
Front. Physiol., 10 May 2023
Sec. Skin Physiology
Volume 14 - 2023 | https://doi.org/10.3389/fphys.2023.1195272
This article is part of the Research TopicAging Skin Physiology: Spotlight on Prevention and ManagementView all 8 articles
Skin aging is a multifaceted process that involves intrinsic and extrinsic mechanisms that lead to various structural and physiological changes in the skin. Intrinsic aging is associated with programmed aging and cellular senescence, which are caused by endogenous oxidative stress and cellular damage. Extrinsic aging is the result of environmental factors, such as ultraviolet (UV) radiation and pollution, and leads to the production of reactive oxygen species, ultimately causing DNA damage and cellular dysfunction. In aged skin, senescent cells accumulate and contribute to the degradation of the extracellular matrix, which further contributes to the aging process. To combat the symptoms of aging, various topical agents and clinical procedures such as chemical peels, injectables, and energy-based devices have been developed. These procedures address different symptoms of aging, but to devise an effective anti-aging treatment protocol, it is essential to thoroughly understand the mechanisms of skin aging. This review provides an overview of the mechanisms of skin aging and their significance in the development of anti-aging treatments.
Skin aging is a complex process that involves numerous biological and biochemical changes as well as secondary structural changes of the skin, underlying muscles, subcutaneous fat tissue, and bony structures. Common aesthetic procedures performed in clinical practice, such as chemical peels, energy-based treatments, injectable treatments, and threads, may share similar mechanisms; however, they often address the symptoms and signs of skin aging in distinct ways. Furthermore, as research on the mechanism of skin aging continues to expand, existing theories are replaced with new concepts, such as cellular senescence of dermal fibroblasts (Fang et al., 2022; Shvedova et al., 2022). Thus, clinicians must possess a thorough comprehension of skin aging physiology to devise a treatment plan that entails selecting anti-aging procedures that target specific mechanisms of skin aging while simultaneously reducing side effects. It is hoped that this narrative review will provide new avenues to comprehensively describe the complex skin aging process and help clinicians to establish anti-aging treatment protocols.
The skin is the body’s largest organ and is continuously exposed to various environmental factors, including ultraviolet (UV) rays, smoking, heat, and air pollution. Therefore, the skin undergoes extrinsic aging as well as intrinsic aging, which is also referred to as chronological aging. The process of intrinsic aging can be considered alongside programmed aging, and it results from continuous chromatic damage by various factors, of which the most representative is oxidative stress caused by reactive oxygen species (ROS). The cells have an endogenous defense system against oxidative stress including superoxide dismutase (SOD), tripeptide glutathione, and catalase (Steenvoorden and van Henegouwen, 1997). Age-related impairment in its redox capacity results in the accumulation of ROS, thereby causing a detrimental effect on cellular components including proteins, lipids, and DNA, consequently leading to cellular dysfunction (Gniadecka et al., 1998; Gu et al., 2020). ROS generated by exogenous factors such as UV rays and air pollution also play a significant role in extrinsic aging.
In response to stress factors including DNA damage, cells enter a state of irreversible growth arrest, which is called cellular senescence (Hayflick, 1965). Recent research has uncovered that cellular senescence plays a major role in the skin aging process (Fitsiou et al., 2021; Wlaschek et al., 2021; Kim et al., 2022a; Kim et al., 2022b; Gerasymchuk et al., 2022; Papaccio et al., 2022). Senescent cells exhibit several biomarkers: 1) increased activity of the cell cycle arrest proteins p21WAF1 and p16INK4A, 2) lysosomal enzyme senescence-associated β galactosidase (SA-β-gal), and 3) decreased expression of nuclear high mobility group box-1 (HMGB1) and lamin B1, a structural component of the nuclear lamina (Ho and Dreesen, 2021). They also release humoral factors known as senescence-associated secretory phenotype (SASP), which includes various inflammatory cytokines, chemokines, matrix proteases, and microRNAs (Coppe et al., 2010; Kim et al., 2016). The temporary cellular senescence signals that physiologically occur during wound healing promote the formation of granulation tissue and skin regeneration while inhibiting excessive cell growth that can progress to precancerous or cancerous lesions (Demaria et al., 2014; Wang and Dreesen, 2018). As age increases, the accumulation of senescent keratinocytes, melanocytes, and, most importantly, fibroblasts can cause various age-related diseases and disrupt the homeostasis of the skin (Wlaschek et al., 2021).
Moreover, the degradation of the extracellular matrix (ECM) is observed as a result of altered senescent cells and excessive ROS production. Excessive ROS activate the mitogen-activated protein kinase (MAPK)/activator protein 1 (AP-1) pathway, which consequently induces the expression of matrix metalloproteinase (MMP), resulting in collagen breakdown (Chung et al., 2000). It also downregulates the collagen production via the TGF-β/Smad signaling pathway (Quan et al., 2004; Quan et al., 2010; He et al., 2014). In addition, tissue inhibitors of metalloproteinases (TIMPs) are downregulated during the aging process. Furthermore, the presence of senescent cells contributes to ECM degradation by promoting chronic inflammatory responses and collagen breakdown. In particular, the senescent fibroblasts express SASP containing MMP-2, MMP-9, and proinflammatory cytokines such as interleukin (IL)-6 and IL-8 (Kuilman et al., 2008; Wang and Dreesen, 2018; Wlaschek et al., 2021). The migration of neutrophils after inflammation or UV exposure further accelerates the collagen and elastin fragmentation via production of neutrophil-derived proteolytic enzymes (Li et al., 2013; Sharma et al., 2020).
These two types of skin aging have several overlapping molecular mechanisms including ROS generation, DNA damage, and structural deterioration of ECM components. Therefore, the clinical phenotypes of skin aging are similar in some respects; however, some facets differ based on the aging process (Park, 2022). Intrinsic aging results in overall thinning of the skin, dry and pale skin, fine wrinkles, and skin sagging with decreased elasticity (Walker, 2022). The functions of the sweat and sebaceous glands also decrease, with less sebum secretion caused by decreased peroxisome proliferator-activated receptor gamma (PPAR-γ) expression ultimately leading to dry skin, while sebaceous gland hyperplasia can occur due to increased gland size (Zouboulis and Boschnakow, 2001; Kim et al., 2014). Furthermore, extrinsic aging manifests as relatively coarse wrinkles, severe loss of elasticity, and dyspigmentation (Walker, 2022).
Despite these differences, biochemical and biophysical changes in the dermis are common to both aging processes and are major contributing factors to the aging phenotypes such as wrinkles and loss of skin elasticity. The dermis of the skin consists of connective tissue that is rich in collagen, which provides mechanical support and structure. Recently, the changes in the dermal components in skin aging and treatments to reverse or combat them to reduce the signs of aging have become the focus of many dermatologists. Therefore, in this part, we will discuss these dermal aging processes in more detail.
The fibroblasts within the dermis are responsible for the synthesis, organization, and remodeling of collagen and thus play a major role in maintaining the integrity of the ECM. As aforementioned, aging causes the accumulation of senescent fibroblasts in the dermis, which causes gradual degradation and dysfunction of the ECM via release of proteolytic, matrix-degrading SASPs (Ressler et al., 2006).
In particular, the matricellular protein CCN1, also known as cysteine-rich protein 61, has been suggested to be a contributor to the age-associated dermal microenvironment. CCN1 is markedly elevated in the human dermal fibroblasts in aged skin, and Quan et al. demonstrated that elevated expression of CCN1 accelerates dermal aging by dysregulating the production and homeostasis of collagen using a transgenic mouse model (Quan et al., 2011; Quan et al., 2021). Their results showed that the fibroblasts of COL1A2-CCN1 mice had increased MMP expression and impaired TGF-β/Smad signaling, resulting in reduced COL-1 expression and fragmentation of ECM. Furthermore, CCN1 induces increased expression of proinflammatory cytokines, thus further promoting dermal aging (Quan et al., 2011). In addition, Ezure et al. found that complement factor D secreted from senescent dermal fibroblasts induces increased MMP-1 expression and negatively impacts matrix production in surrounding young dermal fibroblasts in vitro (Ezure et al., 2019). Collectively, these changes disrupt the complex interaction of dermal fibroblasts with the ECM, including by reducing the mechanical forces exerted on the fibroblasts, which negatively affects their morphology and function (Qin et al., 2014; Fisher et al., 2016).
In addition, Solé-Boldo et al. (2020) found that there is decrease in the number and heterogeneity of dermal fibroblasts with age. These skin aging-associated changes were mainly observed in the papillary dermis rather than in the reticular dermis with decreased papillary dermal fibroblasts (Mine et al., 2008). Collectively, these changes impair the structure and function of the skin and create a microenvironment that is conducive to age-related skin pathologies, including delayed wound healing and skin cancer (Woodley, 2017; Blair et al., 2020; Fane and Weeraratna, 2020; Xue et al., 2022).
Furthermore, senescent fibroblasts contribute to skin aging by interacting with other neighboring cells, including keratinocytes and melanocytes, through paracrine signaling. Insulin-like growth factor (IGF)-1, which is mainly released by dermal fibroblasts, is known to be necessary for mesenchymal stem cell niches and the modulation of epidermal cell proliferation and differentiation (Hodak et al., 1996; Youssef et al., 2017; Muraguchi et al., 2019). In addition, IGF-1 signaling is essential for the appropriate protective responses (DNA damage response, DDR) of keratinocytes to UV-induced DNA damage by inducing favorable cellular senescence or DNA damage repair (Lewis et al., 2009; Loesch et al., 2016; Alkawar et al., 2020). Aged skin exhibits decreased synthesis of IGF-1, which results in the epidermal atrophy and proliferation of keratinocytes with unrepaired DNA, leading to the development of age-related non-melanoma skin cancers (Stachelscheid et al., 2008; Lewis et al., 2009; Alkawar et al., 2020; Wlaschek et al., 2021). More recently, Terlecki-Zaniewicz et al. (2019) showed that extracellular vesicles derived from the senescent fibroblasts affect the terminal differentiation of keratinocytes with decreased expression levels of involucrin in a 2D cell culture model, which is reported to be a major initiator of cornification. Senescent fibroblasts have also been suggested to contribute to age-related pigmentation by inducing activation of melanocytes through several factors such as secreted frizzled-related protein 2, growth differentiation factor 15, and stromal-derived factor 1 (Kim et al., 2016; Yoon et al., 2018; Kim Y. et al., 2020; Kim J. C. et al., 2022). This was also supported by reduced epidermal pigmentation after radiofrequency treatment with reduced p16INK4A-positive senescent fibroblasts in a pilot study by (Kim et al., 2019).
There are also age-related structural changes in the dermal elastic fibers. Elastic fiber networks are composed of elastin and fibrillin, forming a unique arrangement within the dermis. In the upper papillary dermis, oxytalan fibers, which are microfibrillar bundles abundant in fibrillin, play a role in preventing the epidermis from easily detaching from the dermo-epidermal junction (DEJ) by forming a candlestick-shaped organic bond with the DEJ (Heinz, 2021). In photoaged skin, the oxytalan fibers undergo degeneration, and the elastic fibers of upper dermis are degraded by elastolytic enzymes including MMPs and neutrophil elastases (Bernstein et al., 1996; Naylor et al., 2011; Bonta et al., 2013). In addition, the altered, disorganized elastic fibers gradually accumulate in the reticular dermis, appearing as solar elastosis. In contrast, intrinsic skin aging is characterized by overall depletion of the elastic fiber network (El-Domyati et al., 2002).
Recently, it has been recognized that the basement membrane not only provides physical support for keratinocytes but also plays a major role in the regulation of signaling and communication between epidermal and dermal cells (Tsutsui et al., 2021). With age, the protein components of the basement membrane zone, including collagen 7 and 17, nidogen, integrins, and laminin 332, decrease, and the papillary pattern of the DEJ flattens (Iriyama et al., 2011a; Amano, 2016; Roig-Rosello and Rousselle, 2020). It has been postulated that disrupted basement membranes allow soluble melanogenic regulators from senescent fibroblasts to more easily stimulate melanocyte activity and accelerate age-related pigmentation (Goyarts et al., 2007; Amano, 2009; Iriyama et al., 2011b; Bastonini et al., 2016). Iriyama et al. (2022) have shown that the inhibition of basement membrane degradation with MMP inhibitors and heparinase inhibitors promotes the deposition of laminin-511 at the DEJ, which in turn promotes the secretion of platelet-derived growth factor consisting of 2 B subunits (PDGF-BB). Expression of COL5A1 and COL1A1 genes was increased in the fibroblasts stimulated with PDGF-BB, suggesting increased collagen expression in the papillary dermis (Iriyama et al., 2022). Therefore, strengthening the damaged basement membrane and restoring epidermal-dermal integrity have been proposed as new anti-ageing targets, but the actual clinical significance of the DEJ and its role in aging requires much further research.
Age-related changes in proteoglycans (PGs) and glycosaminoglycans (GAGs) are very complex, and there are still many unknown aspects (Oh et al., 2011b; Lee et al., 2016). Although previous research has often reported conflicting results in the changes of PGs and GAGs, they have received attention as promising targets for skin rejuvenation (Oh et al., 2011a; Oh et al., 2011b; Lee et al., 2016; Wang et al., 2021). Unlike collagen, which has a relatively long half-life, GAGs, such as hyaluronic acid (HA), have a much shorter half-life ranging from 24 to 36 h in human skin (Jiang et al., 2007; Fallacara et al., 2018). While the regulation of collagen metabolism takes a long time to show any visible changes, GAGs have the advantage that a treatment effect can be observed within a short period of time. However, further follow-up studies are needed to understand the role of PGs and GAGs in skin aging.
Table 1 summarizes the differences between intrinsic and extrinsic aging of the skin that have been generally recognized to date. However, recent research suggests that this distinction is not as clear-cut as textbooks describe and can often be confusing. It would be more clinically appropriate to understand that middle-aged adults visiting dermatologic clinics for skin rejuvenation undergo concomitant intrinsic and extrinsic aging. Thus, the histological and molecular changes related to skin aging that have been acknowledged to date should be organized to establish an appropriate treatment plan.
As mentioned above, UV rays play a critical role in cellular aging and skin aging; thus, Sun protection—using sunscreen or protective clothing and staying in the shade—is the most basic and essential option for preventing skin aging and slowing the rate of aging-related changes.
Various energy-based devices, such as lasers, high-intensity focused ultrasound (HFU), and radiofrequency (RF) devices, have grown increasingly common to address aging phenotypes. These devices deliver thermal energy to the reticular dermis and subcutaneous tissue, which subsequently causes tissue contraction and stimulates neocollagenesis, leading to improvement in skin laxity and rhytides (Orringer et al., 2012; Majidian et al., 2021; Chen et al., 2022).
An ablative laser, such as a CO2 laser or an Erbium:YAG laser, which requires re-epithelialization, has been used in the past, but recently, a non-ablative fractional laser has been used mainly to reduce the downtime and risk of adverse events including postinflammatory hyperpigmentation or scarring (Nanni and Alster, 1998; Chen et al., 2022). In contrast, fractional picosecond lasers produce nonthermal, photomechanical stress in the dermis and promote fibroblast proliferation (Tanghetti, 2016; K et al., 2021). Recent ex vivo animal and clinical studies also support that 532-nm and 1,064-nm picosecond Nd:YAG lasers may improve photoaged skin (Yim et al., 2020; Connor et al., 2021; Han et al., 2023). In addition, various lasers including low fluence Q-switched Nd:YAG lasers, Q-switched ruby lasers, and Q-switched alexandrite lasers are effective for treating aging-related pigmentation through selective photothermolysis of melanosomes (Anderson and Parrish, 1983; Sadighha et al., 2008; Vachiramon et al., 2016).
HFU is a noninvasive and safe treatment that focuses ultrasound waves on a localized area, much like a magnifying glass focuses light, causing thermal coagulation of the subcutaneous tissue and rearranging the collagen and elastic fibers of the subcutaneous tissue without affecting the skin surface. In contrast, RF devices deliver relatively diffuse thermal energy throughout the dermis (Suh et al., 2015). Kwon et al. (2021) showed that bipolar RF device treatment reduces the number of p16INK4A-positive senescent fibroblasts and increases the expression of HSP70 and HSP90 in melasma skin. More recently, fractional RF microneedling devices that deliver targeted bipolar RF energy directly to the reticular dermis via microneedles have been developed. Fractional RF microneedling devices have also shown to be effective in treating UV-induced hyperpigmentation by upregulating the anti-senescence pathways (Rangarajan et al., 2013; Yoon et al., 2018; Lee et al., 2021).
Furthermore, recent evidence suggests that a light emitting diode (LED) can also ameliorate UV-induced changes in dermal fibroblasts and promote collagen synthesis by photobiomodulation (Baez and Reilly, 2007; Kim et al., 2015; Mamalis and Jagdeo, 2018; Hong et al., 2022). The mechanisms underlying the effects of LEDs aren’t fully understood, and clinical data are insufficient; therefore, further studies are needed.
A variety of topical agents have been used to improve the signs of skin aging, but retinoids are currently considered the most effective option (Samuel et al., 2005). Retinoids have been shown to increase types Ⅰ, III, and VII collagen and GAG deposition and to normalize elastic tissue organization (Woodley et al., 1990). In addition, topical tretinoin treatment also induces thickening of the granular layer and compaction of the stratum corneum, resulting in smooth skin (Berardesca et al., 1990). Clinical evidence also supports the role of topical retinoids in the reversal of skin aging phenotypes including fine wrinkling, dyschromia, and skin elasticity (Weinstein et al., 1991; Olsen et al., 1992; Darlenski et al., 2010; Milosheska and Roskar, 2022). Topical antioxidants, such as ascorbic acid (vitamin C), have also been shown to be effective in reducing skin aging. Ascorbic acid reduces ROS and is required for collagen synthesis in human skin fibroblasts. However, its poor skin penetration and chemical instability can reduce its clinical efficacy. In addition, chemical peeling using topical alpha-hydroxy acids, such as glycolic or lactic acid, have been shown to improve the quality of elastic fibers, stimulate GAG and collagen production in the dermis, and increase the epidermal thickness (Bernstein et al., 2001; Hussein et al., 2008). Tricholoroacetic acid peels also have been shown to promote neocollagenesis and improve benign pigmented lesions (Kitzmiller et al., 2003; Chun et al., 2004).
The development of new formulations through advances in nanotechnology and drug delivery systems is expected to further increase the use of topical agents as well as cosmeceuticals. Microneedling with a dermaroller has also been used to enhance drug delivery by creating pores in the stratum corneum, promoting neocollagenesis through release of various growth factors during the micro-wound healing process (Hou et al., 2017). Similarly, microdermabrasion using aluminum oxide crystals has been shown to be effective in improving the drug delivery and promoting dermal collagen synthesis. Recently, there is increasing evidence that stem cell-derived exosomes can ameliorate aging-related changes including UV-induced DNA damage and ROS generation and MMP-1 expression in senescent fibroblasts, and promote the expression of ECM proteins (Oh et al., 2018; Gao et al., 2021). The autologous stromal vascular fraction extracted from adipose tissue-derived stem cells has also shown to be effective in dermal rejuvenation due to its regenerative capacity (Charles-de-Sa et al., 2015; Rigotti et al., 2016). Still, clinical data are still insufficient, and further studies are needed to elucidate the anti-aging effects of exosomes and the stromal vascular fraction.
The use of injectables in the dermatologic field has been increasing to improve rhytides and restore the soft tissue volume in aged skin. HA is one of the most commonly used injectables available due to its biocompatibility, ease of use, and reversibility. The injection of HA causes the dermis to stretch mechanically and enhances the structural support of the ECM, which activates dermal fibroblasts and leads to the production of type I collagen by activating the TGF-β signaling pathway (Wang et al., 2007; Turlier et al., 2013; Landau and Fagien, 2015). In addition, HA directly activates fibroblasts through its hyaluronan receptors, CD44 and CD168, causing them to migrate and proliferate (Mast et al., 1993; Turley et al., 2002). HA injection is also effective in improving skin hydration and texture (Ayatollahi et al., 2020). Recently, a novel EGF-containing HA filler was shown to induce types I and III collagen production and downregulate the expression of MMP-9 (Shin et al., 2022). In addition to HA, biocompatible polymers such as poly-L-lactic acid, polycaprolactone, and polynucleotide have also been found to stimulate fibroblasts and induce neocollagenesis and are thus increasingly used as injectables (Park et al., 2016; Kim J. H. et al., 2020; Oh et al., 2021). Furthermore, botulinum toxin injection not only overcomes hyperkinetic rhytides but also improves skin elasticity, skin hydration level and decrease skin erythema via suppression of neurogenic inflammation (Gazerani et al., 2009; Zhu et al., 2017). Table 2 summarizes the mechanisms of skin aging and corresponding dermatological interventions.
In very recent decades, researchers have attempted to counteract aging using senotherapeutics that selectively target senescent cells. Senotherapeutics are categorized into two groups. Senolytic drugs selectively eliminate senescent cells, and senomorphic drugs inhibit the negative effects of their SASPs. Since the combination of dasatinib and quercetin was proposed as the first senolytic drug to suppress genes that are increased in senescent cells, many studies have shown that various substances such as ABT-737, ABT-263, A1155463, and fiestin have anti-aging properties (Zhu et al., 2015; Thompson et al., 2022).
In particular, ABT-263 and ABT-737, which are Bcl-2 inhibitors, have been found to selectively eliminate SA β-gal-positive senescent cells in skin both in vitro and ex vivo (Victorelli et al., 2019; Kim et al., 2022a; Kim et al., 2022b; Park et al., 2022). Kim and his colleagues demonstrated that either ABT-263 or ABT-737 treatment selectively eliminated dermal fibroblasts in an intrinsic skin aging mouse model (Kim et al., 2022a). They also showed that the treatment increased the collagen density, epidermal thickness, and keratinocyte proliferation while reducing SASPs including MMP-1 and IL-6. After, this team revealed that treatment with ABT-263 and ABT-737 also attenuated the induction of MMPs and decreased collagen density in the photoaging mouse model (Kim et al., 2022b). In addition, ABT-263 showed potential in reducing pigmentation caused by photoaging in human skin inducing apoptosis of p16INK4A-positive fibroblasts with its senolytic activity, resulting in decreased levels of melanin and tyrosinase activity (Park et al., 2022).
One of the most notable targets of senomorphic agents is the mechanistic/mammalian target of rapamycin (mTOR) pathway, which regulates cellular metabolism and is linked to cellular growth, proliferation, and autophagy (Papadopoli et al., 2019). The mTOR pathway is also involved in the synthesis of SASPs (Cayo et al., 2021). Rapamycin, an mTOR inhibitor, exhibited significant reduction in senescence markers and SASPs as well as oxidative cellular stress in UV-induced photoaged human dermal fibroblasts (Bai et al., 2021). Moreover, Chung et al. revealed the potential anti-aging effect of topical application of rapamycin (an mTOR inhibitor) (Chung et al., 2019). A total of 17 subjects over the age of 40 years with age-related photoaging of the skin applied a rapamycin-containing hand cream to the dorsum of one hand and a placebo hand cream to the other hand daily for 8 months and found that the rapamycin-treated hand had a decrease in p16 and an increase in collagen VII protein.
Taken together, these promising results suggest that senotherapeutics may be a novel therapeutic option for skin aging; however, the limitations of these drugs, such as their specificity, selectivity, and efficiency, still need to be addressed, and their mechanisms of action and side effects must be better understood.
In conclusion, skin aging is a complex process that involves numerous biological and biochemical changes, and clinicians must have a thorough comprehension of skin aging physiology to devise an effective treatment plan. It is hoped that this narrative review will aid medical professionals in developing treatment plans to combat aging and gain a more complete understanding of the intricate process of skin aging.
KP and N-KR contributed to the conception. SS, YL, KP, and N-KR analyzed the data. SS and YL wrote the manuscript. KP and N-KR revised the manuscript. All authors listed have made a substantial, direct, and intellectual contribution to the work and approved it for publication. All authors contributed to the article and approved the submitted version.
The authors declare that the research was conducted in the absence of any commercial or financial relationships that could be construed as a potential conflict of interest.
All claims expressed in this article are solely those of the authors and do not necessarily represent those of their affiliated organizations, or those of the publisher, the editors and the reviewers. Any product that may be evaluated in this article, or claim that may be made by its manufacturer, is not guaranteed or endorsed by the publisher.
Alkawar, A. M. M., Castellanos, A. J., Carpenter, M. A., Hutcherson, R. J., Madkhali, M. . a. O., Johnson, R. M., et al. (2020). Insulin-like growth factor-1 impacts p53 target gene induction in UVB-irradiated keratinocytes and human skin. Photochem Photobiol. 96, 1332–1341. doi:10.1111/php.13279
Amano, S. (2016). Characterization and mechanisms of photoageing-related changes in skin. Damages of basement membrane and dermal structures. Exp. Dermatol 25 (3), 14–19. doi:10.1111/exd.13085
Amano, S. (2009). Possible involvement of basement membrane damage in skin photoaging. J. Investig. Dermatol Symp. Proc. 14, 2–7. doi:10.1038/jidsymp.2009.5
Anderson, R. R., and Parrish, J. A. (1983). Selective photothermolysis: Precise microsurgery by selective absorption of pulsed radiation. Science 220, 524–527. doi:10.1126/science.6836297
Ayatollahi, A., Firooz, A., and Samadi, A. (2020). Evaluation of safety and efficacy of booster injections of hyaluronic acid in improving the facial skin quality. J. Cosmet. Dermatol 19, 2267–2272. doi:10.1111/jocd.13493
Baez, F., and Reilly, L. R. (2007). The use of light-emitting diode therapy in the treatment of photoaged skin. J. Cosmet. Dermatol 6, 189–194. doi:10.1111/j.1473-2165.2007.00329.x
Bai, G. L., Wang, P., Huang, X., Wang, Z. Y., Cao, D., Liu, C., et al. (2021). Rapamycin protects skin fibroblasts from UVA-induced photoaging by inhibition of p53 and phosphorylated HSP27. Front. Cell Dev. Biol. 9, 633331. doi:10.3389/fcell.2021.633331
Bastonini, E., Kovacs, D., and Picardo, M. (2016). Skin pigmentation and pigmentary disorders: Focus on epidermal/dermal cross-talk. Ann. Dermatol 28, 279–289. doi:10.5021/ad.2016.28.3.279
Berardesca, E., Gabba, P., Farinelli, N., Borroni, G., and Rabbiosi, G. (1990). In vivo tretinoin-induced changes in skin mechanical properties. Br. J. Dermatol 122, 525–529. doi:10.1111/j.1365-2133.1990.tb14729.x
Bernstein, E. F., Chen, Y. Q., Kopp, J. B., Fisher, L., Brown, D. B., Hahn, P. J., et al. (1996). Long-term sun exposure alters the collagen of the papillary dermis. Comparison of sun-protected and photoaged skin by northern analysis, immunohistochemical staining, and confocal laser scanning microscopy. J. Am. Acad. Dermatol 34, 209–218. doi:10.1016/s0190-9622(96)80114-9
Bernstein, E. F., Lee, J., Brown, D. B., Yu, R., and Van Scott, E. (2001). Glycolic acid treatment increases type I collagen mRNA and hyaluronic acid content of human skin. Dermatol Surg. 27, 429–433. doi:10.1046/j.1524-4725.2001.00234.x
Blair, M. J., Jones, J. D., Woessner, A. E., and Quinn, K. P. (2020). Skin structure-function relationships and the wound healing response to intrinsic aging. Adv. Wound Care (New Rochelle) 9, 127–143. doi:10.1089/wound.2019.1021
Bonta, M., Daina, L., and Mutiu, G. (2013). The process of ageing reflected by histological changes in the skin. Rom. J. Morphol. Embryol. 54, 797–804.
Cayo, A., Segovia, R., Venturini, W., Moore-Carrasco, R., Valenzuela, C., and Brown, N. (2021). mTOR activity and autophagy in senescent cells, a complex partnership. Int. J. Mol. Sci. 22, 8149. doi:10.3390/ijms22158149
Charles-De-Sa, L., Gontijo-De-Amorim, N. F., Maeda Takiya, C., Borojevic, R., Benati, D., Bernardi, P., et al. (2015). Antiaging treatment of the facial skin by fat graft and adipose-derived stem cells. Plast. Reconstr. Surg. 135, 999–1009. doi:10.1097/PRS.0000000000001123
Chen, S. X., Cheng, J., Watchmaker, J., Dover, J. S., and Chung, H. J. (2022). Review of lasers and energy-based devices for skin rejuvenation and scar treatment with histologic correlations. Dermatol Surg. 48, 441–448. doi:10.1097/DSS.0000000000003397
Chun, E. Y., Lee, J. B., and Lee, K. H. (2004). Focal trichloroacetic acid peel method for benign pigmented lesions in dark-skinned patients. Dermatol Surg. 30, 512–516. doi:10.1111/j.1524-4725.2004.30166.x
Chung, C. L., Lawrence, I., Hoffman, M., Elgindi, D., Nadhan, K., Potnis, M., et al. (2019). Topical rapamycin reduces markers of senescence and aging in human skin: An exploratory, prospective, randomized trial. Geroscience 41, 861–869. doi:10.1007/s11357-019-00113-y
Chung, J. H., Kang, S., Varani, J., Lin, J., Fisher, G. J., and Voorhees, J. J. (2000). Decreased extracellular-signal-regulated kinase and increased stress-activated MAP kinase activities in aged human skin in vivo. J. Invest. Dermatol 115, 177–182. doi:10.1046/j.1523-1747.2000.00009.x
Connor, O. K., Cho, S. B., and Chung, H. J. (2021). Wound healing profile after 1064- and 532-nm picosecond lasers with microlens array of in vivo human skin. Lasers Surg. Med. 53, 1059–1064. doi:10.1002/lsm.23390
Coppe, J. P., Desprez, P. Y., Krtolica, A., and Campisi, J. (2010). The senescence-associated secretory phenotype: The dark side of tumor suppression. Annu. Rev. Pathol. 5, 99–118. doi:10.1146/annurev-pathol-121808-102144
Darlenski, R., Surber, C., and Fluhr, J. W. (2010). Topical retinoids in the management of photodamaged skin: From theory to evidence-based practical approach. Br. J. Dermatol 163, 1157–1165. doi:10.1111/j.1365-2133.2010.09936.x
Demaria, M., Ohtani, N., Youssef, S. A., Rodier, F., Toussaint, W., Mitchell, J. R., et al. (2014). An essential role for senescent cells in optimal wound healing through secretion of PDGF-AA. Dev. Cell 31, 722–733. doi:10.1016/j.devcel.2014.11.012
El-Domyati, M., Attia, S., Saleh, F., Brown, D., Birk, D. E., Gasparro, F., et al. (2002). Intrinsic aging vs. photoaging: A comparative histopathological, immunohistochemical, and ultrastructural study of skin. Exp. Dermatol 11, 398–405. doi:10.1034/j.1600-0625.2002.110502.x
Ezure, T., Sugahara, M., and Amano, S. (2019). Senescent dermal fibroblasts negatively influence fibroblast extracellular matrix-related gene expression partly via secretion of complement factor D. Biofactors 45, 556–562. doi:10.1002/biof.1512
Fallacara, A., Baldini, E., Manfredini, S., and Vertuani, S. (2018). Hyaluronic acid in the third millennium. Polym. (Basel) 10, 701. doi:10.3390/polym10070701
Fane, M., and Weeraratna, A. T. (2020). How the ageing microenvironment influences tumour progression. Nat. Rev. Cancer 20, 89–106. doi:10.1038/s41568-019-0222-9
Fang, J., Ouyang, M., Qu, Y., Wang, M., Huang, X., Lan, J., et al. (2022). Advanced glycation end products promote melanogenesis by activating NLRP3 inflammasome in human dermal fibroblasts. J. Invest. Dermatol 142, 2591–2602.e8. doi:10.1016/j.jid.2022.03.025
Fisher, G. J., Shao, Y., He, T., Qin, Z., Perry, D., Voorhees, J. J., et al. (2016). Reduction of fibroblast size/mechanical force down-regulates TGF-beta type II receptor: Implications for human skin aging. Aging Cell 15, 67–76. doi:10.1111/acel.12410
Fitsiou, E., Pulido, T., Campisi, J., Alimirah, F., and Demaria, M. (2021). Cellular senescence and the senescence-associated secretory phenotype as drivers of skin photoaging. J. Invest. Dermatol 141, 1119–1126. doi:10.1016/j.jid.2020.09.031
Gao, W., Wang, X., Si, Y., Pang, J., Liu, H., Li, S., et al. (2021). Exosome derived from ADSCs attenuates ultraviolet B-mediated photoaging in human dermal fibroblasts. Photochem Photobiol. 97, 795–804. doi:10.1111/php.13370
Gazerani, P., Pedersen, N. S., Drewes, A. M., and Arendt-Nielsen, L. (2009). Botulinum toxin type A reduces histamine-induced itch and vasomotor responses in human skin. Br. J. Dermatol 161, 737–745. doi:10.1111/j.1365-2133.2009.09305.x
Gerasymchuk, M., Robinson, G. I., Kovalchuk, O., and Kovalchuk, I. (2022). Modeling of the senescence-associated phenotype in human skin fibroblasts. Int. J. Mol. Sci. 23, 7124. doi:10.3390/ijms23137124
Gniadecka, M., Nielsen, O. F., Wessel, S., Heidenheim, M., Christensen, D. H., and Wulf, H. C. (1998). Water and protein structure in photoaged and chronically aged skin. J. Invest. Dermatol 111, 1129–1133. doi:10.1046/j.1523-1747.1998.00430.x
Goyarts, E., Muizzuddin, N., Maes, D., and Giacomoni, P. U. (2007). Morphological changes associated with aging: Age spots and the microinflammatory model of skin aging. Ann. N. Y. Acad. Sci. 1119, 32–39. doi:10.1196/annals.1404.006
Gu, Y., Han, J., Jiang, C., and Zhang, Y. (2020). Biomarkers, oxidative stress and autophagy in skin aging. Ageing Res. Rev. 59, 101036. doi:10.1016/j.arr.2020.101036
Han, H. S., Hong, J. K., Park, S. J., Park, B. C., and Park, K. Y. (2023). A randomized, prospective, split-face pilot study to evaluate the safety and efficacy of 532-nm and 1,064-nm picosecond-domain neodymium:yttrium-aluminum-garnet lasers using a diffractive optical element for non-ablative skin rejuvenation: Clinical and histological evaluation. Ann. Dermatol 35, 23–31. doi:10.5021/ad.22.070
Hayflick, L. (1965). The limited in vitro lifetime of human diploid cell strains. Exp. Cell Res. 37, 614–636. doi:10.1016/0014-4827(65)90211-9
He, T., Quan, T., Shao, Y., Voorhees, J. J., and Fisher, G. J. (2014). Oxidative exposure impairs TGF-beta pathway via reduction of type II receptor and SMAD3 in human skin fibroblasts. Age (Dordr) 36, 9623. doi:10.1007/s11357-014-9623-6
Heinz, A. (2021). Elastic fibers during aging and disease. Ageing Res. Rev. 66, 101255. doi:10.1016/j.arr.2021.101255
Ho, C. Y., and Dreesen, O. (2021). Faces of cellular senescence in skin aging. Mech. Ageing Dev. 198, 111525. doi:10.1016/j.mad.2021.111525
Hodak, E., Gottlieb, A. B., Anzilotti, M., and Krueger, J. G. (1996). The insulin-like growth factor 1 receptor is expressed by epithelial cells with proliferative potential in human epidermis and skin appendages: Correlation of increased expression with epidermal hyperplasia. J. Invest. Dermatol 106, 564–570. doi:10.1111/1523-1747.ep12344044
Hong, J. Y., Han, H. S., Youn, J. H., Kim, H. W., Ryu, H. S., and Park, K. Y. (2022). Irradiation with 590-nm yellow light-emitting diode light attenuates oxidative stress and modulates UVB-induced change of dermal fibroblasts. Exp. Dermatol 31, 931–935. doi:10.1111/exd.14542
Hou, A., Cohen, B., Haimovic, A., and Elbuluk, N. (2017). Microneedling: A comprehensive review. Dermatol Surg. 43, 321–339. doi:10.1097/DSS.0000000000000924
Hussein, M. R., Ab-Deif, E. E., Abdel-Motaleb, A. A., Zedan, H., and Abdel-Meguid, A. M. (2008). Chemical peeling and microdermabrasion of the skin: Comparative immunohistological and ultrastructural studies. J. Dermatol Sci. 52, 205–209. doi:10.1016/j.jdermsci.2008.07.004
Iriyama, S., Matsunaga, Y., Takahashi, K., Matsuzaki, K., Kumagai, N., and Amano, S. (2011a). Activation of heparanase by ultraviolet B irradiation leads to functional loss of basement membrane at the dermal-epidermal junction in human skin. Arch. Dermatol Res. 303, 253–261. doi:10.1007/s00403-010-1117-5
Iriyama, S., Ogura, Y., Nishikawa, S., Hosoi, J., and Amano, S. (2022). Regeneration of collagen fibrils at the papillary dermis by reconstructing basement membrane at the dermal-epidermal junction. Sci. Rep. 12, 795. doi:10.1038/s41598-022-04856-1
Iriyama, S., Ono, T., Aoki, H., and Amano, S. (2011b). Hyperpigmentation in human solar lentigo is promoted by heparanase-induced loss of heparan sulfate chains at the dermal-epidermal junction. J. Dermatol Sci. 64, 223–228. doi:10.1016/j.jdermsci.2011.09.007
Jiang, D., Liang, J., and Noble, P. W. (2007). Hyaluronan in tissue injury and repair. Annu. Rev. Cell Dev. Biol. 23, 435–461. doi:10.1146/annurev.cellbio.23.090506.123337
Kim, H., Jang, J., Song, M. J., Kim, G., Park, C. H., Lee, D. H., et al. (2022a). Attenuation of intrinsic ageing of the skin via elimination of senescent dermal fibroblasts with senolytic drugs. J. Eur. Acad. Dermatol Venereol. 36, 1125–1135. doi:10.1111/jdv.18051
Kim, H., Jang, J., Song, M. J., Park, C. H., Lee, D. H., Lee, S. H., et al. (2022b). Inhibition of matrix metalloproteinase expression by selective clearing of senescent dermal fibroblasts attenuates ultraviolet-induced photoaging. Biomed. Pharmacother. 150, 113034. doi:10.1016/j.biopha.2022.113034
Kim, H. S., Park, W. S., Baek, J. I., Lee, B. S., Yoo, D. S., and Park, S. J. (2015). Continuous irradiation with a 633-nm light-emitting diode exerts an anti-aging effect on human skin cells. Int. J. Mol. Med. 35, 383–390. doi:10.3892/ijmm.2014.2030
Kim, J. C., Park, T. J., and Kang, H. Y. (2022c). Skin-aging pigmentation: Who is the real enemy? Cells 11, 2541. doi:10.3390/cells11162541
Kim, J. H., Kwon, T. R., Lee, S. E., Jang, Y. N., Han, H. S., Mun, S. K., et al. (2020a). Comparative evaluation of the effectiveness of novel hyaluronic acid-polynucleotide complex dermal filler. Sci. Rep. 10, 5127. doi:10.1038/s41598-020-61952-w
Kim, J., Nakasaki, M., Todorova, D., Lake, B., Yuan, C. Y., Jamora, C., et al. (2014). p53 Induces skin aging by depleting Blimp1+ sebaceous gland cells. Cell Death Dis. 5, e1141. doi:10.1038/cddis.2014.87
Kim, M., Han, J. H., Kim, J. H., Park, T. J., and Kang, H. Y. (2016). Secreted frizzled-related protein 2 (sFRP2) functions as a melanogenic stimulator; the role of sFRP2 in UV-induced hyperpigmentary disorders. J. Invest. Dermatol 136, 236–244. doi:10.1038/JID.2015.365
Kim, M., Kim, S. M., Kwon, S., Park, T. J., and Kang, H. Y. (2019). Senescent fibroblasts in melasma pathophysiology. Exp. Dermatol 28, 719–722. doi:10.1111/exd.13814
Kim, Y., Kang, B., Kim, J. C., Park, T. J., and Kang, H. Y. (2020b). Senescent fibroblast-derived GDF15 induces skin pigmentation. J. Invest. Dermatol 140, 2478–2486. doi:10.1016/j.jid.2020.04.016
Kitzmiller, W. J., Visscher, M. O., Maclennan, S., Butterfield, J. L., Fuhrman, E., Doughty, D., et al. (2003). Comparison of a series of superficial chemical peels with a single midlevel chemical peel for the correction of facial actinic damage. Aesthet. Surg. J. 23, 339–344. doi:10.1016/S1090-820X(03)00201-2
Kuilman, T., Michaloglou, C., Vredeveld, L. C., Douma, S., Van Doorn, R., Desmet, C. J., et al. (2008). Oncogene-induced senescence relayed by an interleukin-dependent inflammatory network. Cell 133, 1019–1031. doi:10.1016/j.cell.2008.03.039
Kwon, S. H., Na, J. I., Huh, C. H., and Park, K. C. (2021). A clinical and biochemical evaluation of a temperature-controlled continuous non-invasive radiofrequency device for the treatment of melasma. Ann. Dermatol 33, 522–530. doi:10.5021/ad.2021.33.6.522
Landau, M., and Fagien, S. (2015). Science of hyaluronic acid beyond filling: Fibroblasts and their response to the extracellular matrix. Plast. Reconstr. Surg. 136, 188S–195S. doi:10.1097/PRS.0000000000001823
Lee, D. H., Oh, J. H., and Chung, J. H. (2016). Glycosaminoglycan and proteoglycan in skin aging. J. Dermatol Sci. 83, 174–181. doi:10.1016/j.jdermsci.2016.05.016
Lee, Y. I., Kim, E., Lee, D. W., Kim, J., Kim, J., Lee, W. J., et al. (2021). Synergistic effect of 300 μm needle-depth fractional microneedling radiofrequency on the treatment of senescence-induced aging hyperpigmentation of the skin. Int. J. Mol. Sci. 22, 7480. doi:10.3390/ijms22147480
Lewis, D. A., Travers, J. B., and Spandau, D. F. (2009). A new paradigm for the role of aging in the development of skin cancer. J. Invest. Dermatol 129, 787–791. doi:10.1038/jid.2008.293
Li, Y., Xia, W., Liu, Y., Remmer, H. A., Voorhees, J., and Fisher, G. J. (2013). Solar ultraviolet irradiation induces decorin degradation in human skin likely via neutrophil elastase. PLoS One 8, e72563. doi:10.1371/journal.pone.0072563
Loesch, M. M., Collier, A. E., Southern, D. H., Ward, R. E., Tholpady, S. S., Lewis, D. A., et al. (2016). Insulin-like growth factor-1 receptor regulates repair of ultraviolet B-induced DNA damage in human keratinocytes in vivo. Mol. Oncol. 10, 1245–1254. doi:10.1016/j.molonc.2016.06.002
Majidian, M., Kolli, H., and Moy, R. L. (2021). Management of skin thinning and aging: Review of therapies for neocollagenesis; hormones and energy devices. Int. J. Dermatol 60, 1481–1487. doi:10.1111/ijd.15541
Mamalis, A., and Jagdeo, J. (2018). High-fluence light-emitting diode-generated red light modulates the transforming growth factor-beta pathway in human skin fibroblasts. Dermatol Surg. 44, 1317–1322. doi:10.1097/DSS.0000000000001549
Mast, B. A., Diegelmann, R. F., Krummel, T. M., and Cohen, I. K. (1993). Hyaluronic acid modulates proliferation, collagen and protein synthesis of cultured fetal fibroblasts. Matrix 13, 441–446. doi:10.1016/s0934-8832(11)80110-1
Milosheska, D., and Roskar, R. (2022). Use of retinoids in topical antiaging treatments: A focused review of clinical evidence for conventional and nanoformulations. Adv. Ther. 39, 5351–5375. doi:10.1007/s12325-022-02319-7
Mine, S., Fortunel, N. O., Pageon, H., and Asselineau, D. (2008). Aging alters functionally human dermal papillary fibroblasts but not reticular fibroblasts: A new view of skin morphogenesis and aging. PLoS One 3, e4066. doi:10.1371/journal.pone.0004066
Muraguchi, T., Nanba, D., Nishimura, E. K., and Tashiro, T. (2019). IGF-1R deficiency in human keratinocytes disrupts epidermal homeostasis and stem cell maintenance. J. Dermatol Sci. 94, 298–305. doi:10.1016/j.jdermsci.2019.05.001
Nanni, C. A., and Alster, T. S. (1998). Complications of carbon dioxide laser resurfacing. An evaluation of 500 patients. Dermatol Surg. 24, 315–320. doi:10.1111/j.1524-4725.1998.tb04161.x
Naylor, E. C., Watson, R. E., and Sherratt, M. J. (2011). Molecular aspects of skin ageing. Maturitas 69, 249–256. doi:10.1016/j.maturitas.2011.04.011
Oh, H., Lee, S., Na, J., and Kim, J. H. (2021). Comparative evaluation of safety and efficacy of a novel hyaluronic acid-polynucleotide/poly-L-lactic acid composite dermal filler. Aesthetic Plast. Surg. 45, 1792–1801. doi:10.1007/s00266-021-02295-3
Oh, J. H., Kim, Y. K., Jung, J. Y., Shin, J. E., and Chung, J. H. (2011a). Changes in glycosaminoglycans and related proteoglycans in intrinsically aged human skin in vivo. Exp. Dermatol 20, 454–456. doi:10.1111/j.1600-0625.2011.01258.x
Oh, J. H., Kim, Y. K., Jung, J. Y., Shin, J. E., Kim, K. H., Cho, K. H., et al. (2011b). Intrinsic aging- and photoaging-dependent level changes of glycosaminoglycans and their correlation with water content in human skin. J. Dermatol Sci. 62, 192–201. doi:10.1016/j.jdermsci.2011.02.007
Oh, M., Lee, J., Kim, Y. J., Rhee, W. J., and Park, J. H. (2018). Exosomes derived from human induced pluripotent stem cells ameliorate the aging of skin fibroblasts. Int. J. Mol. Sci. 19, 1715. doi:10.3390/ijms19061715
Olsen, E. A., Katz, H. I., Levine, N., Shupack, J., Billys, M. M., Prawer, S., et al. (1992). Tretinoin emollient cream: A new therapy for photodamaged skin. J. Am. Acad. Dermatol 26, 215–224. doi:10.1016/0190-9622(92)70030-j
Orringer, J. S., Sachs, D. L., Shao, Y., Hammerberg, C., Cui, Y., Voorhees, J. J., et al. (2012). Direct quantitative comparison of molecular responses in photodamaged human skin to fractionated and fully ablative carbon dioxide laser resurfacing. Dermatol Surg. 38, 1668–1677. doi:10.1111/j.1524-4725.2012.02518.x
Papaccio, F., Caputo, S., and Bellei, B. (2022). Focus on the contribution of oxidative stress in skin aging. Antioxidants (Basel) 11, 1121. doi:10.3390/antiox11061121
Papadopoli, D., Boulay, K., Kazak, L., Pollak, M., Mallette, F., Topisirovic, I., et al. (2019). mTOR as a central regulator of lifespan and aging. F1000Res 8, F1000Res. doi:10.12688/f1000research.17196.1
Park, J. H., Yoon, J. E., Kim, Y. H., Kim, Y., Park, T. J., and Kang, H. Y. (2022). The potential skin-lightening candidate, senolytic drug ABT263, for photoageing pigmentation. Br. J. Dermatol 186, 740–742. doi:10.1111/bjd.20893
Park, K. Y., Seok, J., Rho, N. K., Kim, B. J., and Kim, M. N. (2016). Long-chain polynucleotide filler for skin rejuvenation: Efficacy and complications in five patients. Dermatol Ther. 29, 37–40. doi:10.1111/dth.12299
Park, S. (2022). Biochemical, structural and physical changes in aging human skin, and their relationship. Biogerontology 23, 275–288. doi:10.1007/s10522-022-09959-w
Qin, Z., Voorhees, J. J., Fisher, G. J., and Quan, T. (2014). Age-associated reduction of cellular spreading/mechanical force up-regulates matrix metalloproteinase-1 expression and collagen fibril fragmentation via c-Jun/AP-1 in human dermal fibroblasts. Aging Cell 13, 1028–1037. doi:10.1111/acel.12265
Quan, T., He, T., Kang, S., Voorhees, J. J., and Fisher, G. J. (2004). Solar ultraviolet irradiation reduces collagen in photoaged human skin by blocking transforming growth factor-beta type II receptor/Smad signaling. Am. J. Pathol. 165, 741–751. doi:10.1016/s0002-9440(10)63337-8
Quan, T., Qin, Z., Robichaud, P., Voorhees, J. J., and Fisher, G. J. (2011). CCN1 contributes to skin connective tissue aging by inducing age-associated secretory phenotype in human skin dermal fibroblasts. J. Cell Commun. Signal 5, 201–207. doi:10.1007/s12079-011-0144-0
Quan, T., Shao, Y., He, T., Voorhees, J. J., and Fisher, G. J. (2010). Reduced expression of connective tissue growth factor (CTGF/CCN2) mediates collagen loss in chronologically aged human skin. J. Invest. Dermatol 130, 415–424. doi:10.1038/jid.2009.224
Quan, T., Xiang, Y., Liu, Y., Qin, Z., Yang, Y., Bou-Gharios, G., et al. (2021). Dermal fibroblast CCN1 expression in mice recapitulates human skin dermal aging. J. Invest. Dermatol 141, 1007–1016. doi:10.1016/j.jid.2020.07.019
Rangarajan, S., Trivedi, A., Ubeid, A. A., and Hantash, B. M. (2013). Minimally invasive bipolar fractional radiofrequency treatment upregulates anti-senescence pathways. Lasers Surg. Med. 45, 201–206. doi:10.1002/lsm.22135
Ressler, S., Bartkova, J., Niederegger, H., Bartek, J., Scharffetter-Kochanek, K., Jansen-Durr, P., et al. (2006). p16INK4A is a robust in vivo biomarker of cellular aging in human skin. Aging Cell 5, 379–389. doi:10.1111/j.1474-9726.2006.00231.x
Rigotti, G., Charles-De-Sa, L., Gontijo-De-Amorim, N. F., Takiya, C. M., Amable, P. R., Borojevic, R., et al. (2016). Expanded stem cells, stromal-vascular fraction, and platelet-rich plasma enriched fat: Comparing results of different facial rejuvenation approaches in a clinical trial. Aesthet. Surg. J. 36, 261–270. doi:10.1093/asj/sjv231
Roig-Rosello, E., and Rousselle, P. (2020). The human epidermal basement membrane: A shaped and cell instructive platform that aging slowly alters. Biomolecules 10, 1607. doi:10.3390/biom10121607
Sadighha, A., Saatee, S., and Muhaghegh-Zahed, G. (2008). Efficacy and adverse effects of Q-switched ruby laser on solar lentigines: A prospective study of 91 patients with fitzpatrick skin type II, III, and IV. Dermatol Surg. 34, 1465–1468. doi:10.1111/j.1524-4725.2008.34310.x
Samuel, M., Brooke, R. C., Hollis, S., and Griffiths, C. E. (2005). Interventions for photodamaged skin. Cochrane Database Syst. Rev. 2005, CD001782. doi:10.1002/14651858.CD001782.pub2
Sharma, M. R., Mitrani, R., and Werth, V. P. (2020). Effect of TNFα blockade on UVB-induced inflammatory cell migration and collagen loss in mice. J. Photochem Photobiol. B 213, 112072. doi:10.1016/j.jphotobiol.2020.112072
Shin, S. H., Roh, Y. J., Jin, S. C., Hong, E. P., Park, J. K., Li, K., et al. (2022). Rheological properties and preclinical data of novel hyaluronic acid filler containing epidermal growth factor. Exp. Dermatol 31, 1685–1692. doi:10.1111/exd.14638
Shvedova, M., Samdavid Thanapaul, R. J. R., Thompson, E. L., Niedernhofer, L. J., and Roh, D. S. (2022). Cellular senescence in aging, tissue repair, and regeneration. Plast. Reconstr. Surg. 150, 4S–11S. doi:10.1097/PRS.0000000000009667
Solé-Boldo, L., Raddatz, G., Schütz, S., Mallm, J. P., Rippe, K., Lonsdorf, A. S., et al. (2020). Single-cell transcriptomes of the human skin reveal age-related loss of fibroblast priming. Commun. Biol. 3 (1), 188. doi:10.1038/s42003-020-0922-4
Stachelscheid, H., Ibrahim, H., Koch, L., Schmitz, A., Tscharntke, M., Wunderlich, F. T., et al. (2008). Epidermal insulin/IGF-1 signalling control interfollicular morphogenesis and proliferative potential through Rac activation. EMBO J. 27, 2091–2101. doi:10.1038/emboj.2008.141
Steenvoorden, D. P., and Van Henegouwen, G. M. (1997). The use of endogenous antioxidants to improve photoprotection. J. Photochem Photobiol. B 41, 1–10. doi:10.1016/s1011-1344(97)00081-x
Suh, D. H., Choi, J. H., Lee, S. J., Jeong, K. H., Song, K. Y., and Shin, M. K. (2015). Comparative histometric analysis of the effects of high-intensity focused ultrasound and radiofrequency on skin. J. Cosmet. Laser Ther. 17, 230–236. doi:10.3109/14764172.2015.1022189
Tanghetti, E. A. (2016). The histology of skin treated with a picosecond alexandrite laser and a fractional lens array. Lasers Surg. Med. 48, 646–652. doi:10.1002/lsm.22540
Terlecki-Zaniewicz, L., Pils, V., Bobbili, M. R., Lämmermann, I., Perrotta, I., Grillenberger, T., et al. (2019). Extracellular Vesicles in Human Skin: Cross-Talk from Senescent Fibroblasts to Keratinocytes by miRNAs. J. Invest. Dermatol. 139 (12), 2425–2436.e5. doi:10.1016/j.jid.2019.05.015
Thompson, E. L., Pitcher, L. E., Niedernhofer, L. J., and Robbins, P. D. (2022). Targeting cellular senescence with senotherapeutics: Development of new approaches for skin care. Plast. Reconstr. Surg. 150, 12S–19S. doi:10.1097/PRS.0000000000009668
Tsutsui, K., Machida, H., Nakagawa, A., Ahn, K., Morita, R., Sekiguchi, K., et al. (2021). Mapping the molecular and structural specialization of the skin basement membrane for inter-tissue interactions. Nat. Commun. 12, 2577. doi:10.1038/s41467-021-22881-y
Turley, E. A., Noble, P. W., and Bourguignon, L. Y. (2002). Signaling properties of hyaluronan receptors. J. Biol. Chem. 277, 4589–4592. doi:10.1074/jbc.R100038200
Turlier, V., Delalleau, A., Casas, C., Rouquier, A., Bianchi, P., Alvarez, S., et al. (2013). Association between collagen production and mechanical stretching in dermal extracellular matrix: In vivo effect of cross-linked hyaluronic acid filler. A randomised, placebo-controlled study. J. Dermatol Sci. 69, 187–194. doi:10.1016/j.jdermsci.2012.12.006
Vachiramon, V., Panmanee, W., Techapichetvanich, T., and Chanprapaph, K. (2016). Comparison of Q-switched Nd: YAG laser and fractional carbon dioxide laser for the treatment of solar lentigines in asians. Lasers Surg. Med. 48, 354–359. doi:10.1002/lsm.22472
Victorelli, S., Lagnado, A., Halim, J., Moore, W., Talbot, D., Barrett, K., et al. (2019). Senescent human melanocytes drive skin ageing via paracrine telomere dysfunction. EMBO J. 38, e101982. doi:10.15252/embj.2019101982
Walker, M. (2022). Human skin through the ages. Int. J. Pharm. 622, 121850. doi:10.1016/j.ijpharm.2022.121850
Wang, A. S., and Dreesen, O. (2018). Biomarkers of cellular senescence and skin aging. Front. Genet. 9, 247. doi:10.3389/fgene.2018.00247
Wang, F., Garza, L. A., Kang, S., Varani, J., Orringer, J. S., Fisher, G. J., et al. (2007). In vivo stimulation of de novo collagen production caused by cross-linked hyaluronic acid dermal filler injections in photodamaged human skin. Arch. Dermatol 143, 155–163. doi:10.1001/archderm.143.2.155
Wang, S. T., Neo, B. H., and Betts, R. J. (2021). Glycosaminoglycans: Sweet as sugar targets for topical skin anti-aging. Clin. Cosmet. Investig. Dermatol 14, 1227–1246. doi:10.2147/CCID.S328671
Weinstein, G. D., Nigra, T. P., Pochi, P. E., Savin, R. C., Allan, A., Benik, K., et al. (1991). Topical tretinoin for treatment of photodamaged skin. A multicenter study. Arch. Dermatol 127, 659–665. doi:10.1001/archderm.127.5.659
Wlaschek, M., Maity, P., Makrantonaki, E., and Scharffetter-Kochanek, K. (2021). Connective tissue and fibroblast senescence in skin aging. J. Invest. Dermatol 141, 985–992. doi:10.1016/j.jid.2020.11.010
Woodley, D. T. (2017). Distinct fibroblasts in the papillary and reticular dermis: Implications for wound healing. Dermatol Clin. 35, 95–100. doi:10.1016/j.det.2016.07.004
Woodley, D. T., Zelickson, A. S., Briggaman, R. A., Hamilton, T. A., Weiss, J. S., Ellis, C. N., et al. (1990). Treatment of photoaged skin with topical tretinoin increases epidermal-dermal anchoring fibrils. A preliminary report. JAMA 263, 3057–3059. doi:10.1001/jama.263.22.3057
Xue, M., Zhao, R., March, L., and Jackson, C. (2022). Dermal fibroblast heterogeneity and its contribution to the skin repair and regeneration. Adv. Wound Care (New Rochelle) 11, 87–107. doi:10.1089/wound.2020.1287
Yim, S., Lee, Y. H., Choi, Y. J., and Kim, W. S. (2020). Split-face comparison of the picosecond 1064-nm Nd:YAG laser using a microlens array and the quasi-long-pulsed 1064-nm Nd:YAG laser for treatment of photoaging facial wrinkles and pores in Asians. Lasers Med. Sci. 35, 949–956. doi:10.1007/s10103-019-02906-1
Yoon, J. E., Kim, Y., Kwon, S., Kim, M., Kim, Y. H., Kim, J. H., et al. (2018). Senescent fibroblasts drive ageing pigmentation: A potential therapeutic target for senile lentigo. Theranostics 8, 4620–4632. doi:10.7150/thno.26975
Youssef, A., Aboalola, D., and Han, V. K. (2017). The roles of insulin-like growth factors in mesenchymal stem cell niche. Stem Cells Int. 2017, 9453108. doi:10.1155/2017/9453108
Zhu, J., Ji, X., Xu, Y., Liu, J., Miao, Y. Y., Zhang, J. A., et al. (2017). The efficacy of intradermal injection of type A botulinum toxin for facial rejuvenation. Dermatol Ther. 30, e12433. doi:10.1111/dth.12433
Zhu, Y., Tchkonia, T., Pirtskhalava, T., Gower, A. C., Ding, H., Giorgadze, N., et al. (2015). The achilles' heel of senescent cells: from transcriptome to senolytic drugs. Aging Cell 14, 644–658. doi:10.1111/acel.12344
Keywords: cellular senescence, dermal fibroblast, rejuvenation, skin aging, senotherapeutics
Citation: Shin SH, Lee YH, Rho N-K and Park KY (2023) Skin aging from mechanisms to interventions: focusing on dermal aging. Front. Physiol. 14:1195272. doi: 10.3389/fphys.2023.1195272
Received: 28 March 2023; Accepted: 02 May 2023;
Published: 10 May 2023.
Edited by:
Dong Hun Lee, Seoul National University Hospital, Republic of KoreaReviewed by:
Si-Hyung Lee, Seoul National University, Republic of KoreaCopyright © 2023 Shin, Lee, Rho and Park. This is an open-access article distributed under the terms of the Creative Commons Attribution License (CC BY). The use, distribution or reproduction in other forums is permitted, provided the original author(s) and the copyright owner(s) are credited and that the original publication in this journal is cited, in accordance with accepted academic practice. No use, distribution or reproduction is permitted which does not comply with these terms.
*Correspondence: Kui Young Park, a3lreUBjYXVocy5vci5rcg==
Disclaimer: All claims expressed in this article are solely those of the authors and do not necessarily represent those of their affiliated organizations, or those of the publisher, the editors and the reviewers. Any product that may be evaluated in this article or claim that may be made by its manufacturer is not guaranteed or endorsed by the publisher.
Research integrity at Frontiers
Learn more about the work of our research integrity team to safeguard the quality of each article we publish.