- 1Singapore Institute for Clinical Sciences (SICS), Agency for Science, Technology and Research (A*STAR), Singapore, Singapore
- 2Department of Obstetrics and Gynaecology, Yong Loo Lin School of Medicine, National University of Singapore, Singapore, Singapore
- 3NIHR Southampton Biomedical Research Centre, University of Southampton and University Hospital Southampton NHS Foundation Trust, Southampton, United Kingdom
- 4Department of Biochemistry and Precision Medicine Translational Research Programme, Yong Loo Lin School of Medicine, National University of Singapore, Singapore, Singapore
- 5Singapore Lipidomics Incubator, Life Sciences Institute, National University of Singapore, Singapore, Singapore
- 6MRC Lifecourse Epidemiology Centre, University of Southampton, Southampton, United Kingdom
- 7Institute of Developmental Sciences, Faculty of Medicine, University of Southampton, Southampton, United Kingdom
Older pregnant women have increased risks of complications including gestational diabetes and stillbirth. Carnitine palmitoyl transferase (CPT) expression declines with age in several tissues and is linked with poorer metabolic health. Mitochondrial CPTs catalyze acylcarnitine synthesis, which facilitates fatty acid oxidization as fuel. We hypothesized that the placenta, containing maternally-inherited mitochondria, shows an age-related CPT decline that lowers placental acylcarnitine synthesis, increasing vulnerability to pregnancy complications. We assessed CPT1A, CPT1B, CPT1C and CPT2 mRNA expression by qPCR in 77 placentas and quantified 10 medium and long-chain acylcarnitines by LC-MS/MS in a subset of 50 placentas. Older maternal age associated with lower expression of placental CPT1B, but not CPT1A, CPT1C or CPT2. CPT1B expression positively associated with eight acylcarnitines and CPT1C with three acylcarnitines, CPT1A negatively associated with nine acylcarnitines, while CPT2 did not associate with any acylcarnitine. Older maternal age associated with reductions in five acylcarnitines, only in those with BMI≥ 25 kg/m2, and not after adjusting for CPT1B expression. Our findings suggest that CPT1B is the main transferase for placental long-chain acylcarnitine synthesis, and age-related CPT1B decline may underlie decreased placental metabolic flexibility, potentially contributing to pregnancy complications in older women, particularly if they are overweight.
1 Introduction
More women are entering pregnancy at an older age worldwide, particularly in developed countries. These women are at increased risk of pregnancy complications including gestational diabetes, pre-eclampsia and stillbirth (Flenady et al., 2011; Lean et al., 2017; Saccone et al., 2022). Nonetheless, the mechanistic pathways by which older age contributes to adverse pregnancy outcomes are still unclear (Huang et al., 2008; Plows et al., 2018). It is thus important to examine potential mechanisms by which advanced maternal age might affect pregnancy outcomes to aid development of strategies to reduce risk.
Outside of pregnancy, several studies have reported that expression and activity of carnitine palmitoyltransferases (CPTs) decline with age in multiple tissues and that these changes associate with poorer metabolic health. Aging is associated with decreased CPT1 activity in rodent hearts (McMillin et al., 1993; Odiet et al., 1995), where the predominant CPT1 isoform is CPT1B. In mice, genetically-induced deficiency or age-associated reduction of skeletal muscle CPT1B expression leads to the development of insulin resistance provoked by a high fat diet challenge (Kim et al., 2014; Vieira-Lara et al., 2021). Such relationships are consistent with a study in elderly humans showing that higher skeletal CPT1B mRNA expression associated with insulin sensitivity and better metabolic health (Bétry et al., 2019). A negative association of CPT1 expression with age is also observed in peripheral blood mononuclear cells (Karlic et al., 2003).
CPTs catalyze the synthesis of acylcarnitines from fatty-acyl CoAs, a process essential to facilitate the transport of fatty acids into mitochondria for fatty acid oxidation (also known as beta oxidation), and for the production of acylcarnitines for cellular use, secretion and signaling (Ceccarelli et al., 2011; Houten et al., 2020). CPTs are expressed in most tissues with the ratios of isoforms dependent on the tissue type and species (Ceccarelli et al., 2011; Houten et al., 2020). CPT1 and CPT2 are present on the outer and inner mitochondrial membrane respectively and together enable fatty acids to be transported across the mitochondrial membrane as acylcarnitines for utilization (Ceccarelli et al., 2011; Houten et al., 2020). CPT1 is the main regulator of fatty acid oxidation and occurs as three isoforms—CPT1A, CPT1B and CPT1C; their individual characteristics remain under investigation (Ceccarelli et al., 2011; Virmani et al., 2015; Houten et al., 2020). CPTs are also active in peroxisomes and the endoplasmic reticulum, but their role in these organelles is not well understood (Sierra et al., 2008; Ceccarelli et al., 2011; Houten et al., 2020).
In the placenta, CPTs are important for generating acylcarnitines, for use locally as well as for release into both fetal and maternal circulations to serve as both a fuel source and a precursor of activated fatty acids for lipid remodeling and protein palmitoylation (Novak et al., 1981; Schmidt-Sommerfeld et al., 1985; Arduini et al., 1992; Arduini et al., 1994; Lahjouji et al., 2004; Jones et al., 2010). These exported acylcarnitines act as signaling molecules, anti-oxidants and as an alternative fetal fuel source to glucose (Jones et al., 2010; Yli and Kjellmer, 2016; Kolb et al., 2021). Hence, acylcarnitine supply is vital to the fetus when glucose and oxygen supply is limited and when anaerobic metabolism and oxidative stress is high such as during parturition (Jones et al., 2010; Yli and Kjellmer, 2016; Kolb et al., 2021). Indeed, increased umbilical cord blood acylcarnitines are associated with both extremes of birthweight (Giannacopoulou et al., 1998; Sánchez-Pintos et al., 2016; El-Wahed et al., 2017; Sánchez-Pintos et al., 2017), where there is often either a lack or oversupply of nutrients relative to fetal needs.
Therefore, given that developmentally, mitochondria in conceptuses and, hence, placental mitochondrial CPTs are maternally-inherited, we hypothesized that a maternal age-related decline in placental CPT expression and activity may contribute to the development of pregnancy adversity. As an initial step, our study aimed to determine the relationship between maternal age and the placental expression of four CPT isoforms, and associated alterations in placental acylcarnitine abundance.
2 Materials and methods
2.1 Subject recruitment and placental collection
Placentas were collected at term elective cesarean sections of singleton pregnancies at the National University Hospital, Singapore with written informed consent. Only elective cesarean section cases were included to reduce the possible effects of labor on placental expression of CPTs and acylcarnitine content. Indications for elective cesarean section were previous cesarean section, breech presentation, suspected macrosomia or maternal request/social reasons. Participants were of Asian ethnicity (classified as Chinese and non-Chinese: Malay or Indian), self-reported non-smokers, conceived spontaneously and delivered neonates that were not small-for-gestational age (birthweight >10th centile). All participants underwent a routine 75 g oral glucose tolerance test (OGTT) after an overnight fast during pregnancy. Gestational diabetes mellitus (GDM) was diagnosed according to World Health Organization 2013 criteria of a fasting glucose 5.1—6.9 mmol/L, and/or 1 h glucose ≥10.0 mmol/L, and/or 2 h glucose 8.5—11.0 mmol/L (World Health Organization Guideline, 2014). With the exception of GDM in 39 subjects, all participants were otherwise healthy and had uncomplicated pregnancies (Table 1). Ethics approval was granted by the National Healthcare Group Domain Specific Research Board (References 2000/00524 and 2016/00183).
2.2 Sample processing
Five villous tissue biopsies were obtained from random sampling across each placenta. Following removal of the maternal decidua, biopsies were snap frozen in liquid nitrogen within 10 min of delivery and stored at −80°C until use. Considering variation across each placenta, biopsies for each placenta were subsequently pulverized in liquid nitrogen and mixed together for RNA and lipid extractions.
2.3 RNA extraction, cDNA synthesis and real-time quantitative polymerase chain reaction (RT-qPCR)
Placental mRNA expression of carnitine palmitoyltransferases was determined as described previously (Watkins et al., 2022). Briefly, following phenol-chloroform extraction, placental RNA was purified with the RNeasy Mini Kit (Qiagen) and reverse transcribed to cDNA with Superscript III reverse transcriptase (Thermo Fisher Scientific) according to manufacturer’s instructions. RT-qPCR was performed with TaqMan Fast Advanced Master Mix (Thermo Fisher Scientific) on an Applied Biosystems 7500 Fast Real-Time PCR System (Thermo Fisher Scientific). Samples were run in duplicate 10 µL reactions containing 5 ng cDNA at the following settings: 95°C for 20 s, followed by 45 cycles of 95°C for 3 s and 60°C for 30 s. Inventoried FAM-labeled TaqMan probes were used for three housekeeping genes—CYC1 (cytochrome C1, Hs00357718_m1), SDHA (succinate dehydrogenase complex, subunit A, Hs00188166_m1) and TBP (TATA-box binding protein, Hs00427620_m1); and four CPT family genes—CPT1A (Hs00912671_m1), CPT1B (Hs00993896_g1), CPT1C (Hs00380581_m1) and CPT2 (Hs00988962_m1). The average threshold cycle (CT) value of non-GDM subjects served as the calibrator (assigned value of 1) for relative quantification. Relative expression of each CPT isoform was calculated using formula 2(−ΔCT) and normalized to the geometric mean expression of the three housekeeping genes.
2.4 Lipid extraction and quantification by liquid chromatography tandem mass spectrometry (LC-MS/MS)
Lipid extraction and quantification by LC-MS/MS was performed on a subset of 50 placentas using methods similar to previous work on the placental lipidome (Wong et al., 2021; Watkins et al., 2022). In brief, approximately 250 mg of each placental sample was freeze-dried, weighed and homogenized in 1 ml phosphate buffered saline. Following an addition of 800 µL butanol/methanol (1:1) to 40 µL of placental homogenate and 10 µL of internal standard mix containing 151.1 pmol acylcarnitine 16:0 d3 (Larodan Chemicals, Solna, Sweden), samples were vortexed briefly, sonicated for 30 min in an ice bath and shaken for 30 min at 4°C. After centrifugation at 13,000 rpm for 10 min, the supernatant was collected into a La-Pha-Pack HPLC tube (Langerwehe, Germany) and stored at -80°C until LC-MS/MS analysis. Quality control (QC) samples were similarly prepared from placental homogenates pooled from several subjects. Lipid extracts (5 µL) were then injected into an Agilent 6490 triple quadrupole LC-MS/MS instrument with chromatography performed as described in Supplementary Methods. Metabolite peak areas were integrated using Mass Hunter QQQ Quantitative Analysis Version 10. Lipids were considered quantifiable if their %RSD in QC samples was less than 25% and the peak area at least 10 times that of a blank sample extracted under the same conditions. Placental lipid content was expressed as µmol lipid/mg tissue dry weight. Ten medium and long chain acylcarnitines (12:0, 14:0, 14:1, 14:2, 15:0, 16:0, 16:1, 18:0, 18:1 and 18:2) were measured.
2.5 Statistical analysis
To ensure a normal distribution and to standardize comparisons between genes and lipids that had different degrees of interindividual variability, gene expression and lipid data were log2-transformed and then converted to Z-scores for analysis. Linear regression models were run in R version “Kick Things” with ‘tidyverse’ Version: 1.3.1 (Wickham et al., 2019). To account for multiple testing and minimize false discovery, Benjamini–Hochberg correction was applied with statistical significance set at p < 0.05. For each CPT, linear regression was first performed between gene expression (outcome) and maternal age. This model was then rerun with covariate adjustment for maternal fasting glycemia, ethnicity, maternal BMI, gestational age and infant sex. To determine how CPT expression might influence the production of placental acylcarnitines, linear regression was performed between placental acylcarnitine abundance (outcome) and gene expression (for each CPT). Lastly, to explore if maternal factors such as high maternal glycemia and BMI affected the relationship between placental CPT expression with maternal age and acylcarnitine abundance, these associations were examined in the study population as a whole, as well as following stratification by GDM or BMI status, as decided a priori.
3 Results
3.1 Clinical characteristics of study participants
Study participants (n = 77) for the RT-qPCR analysis were predominantly of Chinese ethnicity, with a mean age of 33 years, a mean BMI in early pregnancy of 25.5 kg/m2 and delivered at an average of 38.6 weeks of gestation (Table 1). Approximately 50% of these women had GDM, with an average antenatal OGTT fasting and 2 h glucose of 4.5 and 7.4 mmol/L respectively. The maternal characteristics of the subset (n = 50) used for the lipidomic analysis were similar to those used for the RT-qPCR analysis. The proportion of male to female infants, birthweight mean and average birthweight centiles were also comparable between both groups.
3.2 Participant characteristics associated with placental expression of CPT isoforms
Older maternal age was associated with lower placental CPT1B expression [coefficient estimate: -0.107 (-0.167, -0.047) Z-score of expression per year, p = 0.001], but not with that of CPT1A [0.043 (-0.021, 0.108), p > 0.05], CPT1C [0.018 (-0.047, 0.083), p > 0.05] or CPT2 [0.003 (-0.062, 0.068), p > 0.05] (Figure 1). Associations remained similar [CPT1B coefficient estimate: -0.111 (-0.173, -0.049), p = 0.006] after covariate adjustment for maternal fasting glycemia, ethnicity, maternal BMI, gestational age and infant sex. No associations were observed between maternal fasting glycemia, ethnicity, maternal BMI, gestational age or fetal sex and the expression of any CPT isoform.
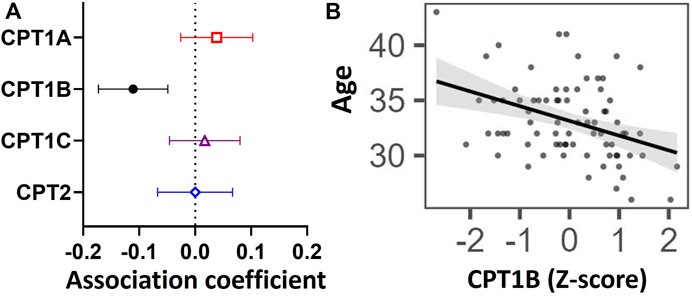
FIGURE 1. Associations between placental mRNA expression of CPT isoforms and maternal age in n = 77 placentas. Forest plot (A) shows coefficient estimates and 95% confidence intervals of associations between each CPT isoform (outcome) and age (years), after adjusting for maternal fasting glycemia, ethnicity, maternal BMI, gestational age and fetal sex. Scatter plot (B) shows the unadjusted relationship between maternal age and Z-score for CPT1B mRNA expression. A black line denotes a significant association. The shaded grey area represents the 95% confidence interval of the regression. Placental mRNA expression data of CPTs were log2-transformed then converted to Z-scores prior to linear regression.
3.3 Association of expression of CPT isoforms with acylcarnitines in the placenta
To determine whether variations in CPT isoform expression related to differences in transferase activity represented by placental acylcarnitine content, we examined the relationship between expression of each CPT isoform with 10 medium and long-chain acylcarnitines in the placenta (Figure 2). Placental CPT1A expression negatively associated with nine acylcarnitines (12:0, 14:0, 14:1, 14:2, 15:0, 16.0, 16:1, 18:0, 18:1), while CPT1B positively associated with eight acylcarnitines (12:0, 14:0, 14:1, 14:2, 16.0, 16:1, 18:0, 18:1) and CPT1C positively associated with three long chain acylcarnitines (16:0, 18:0 and 18:2). The exception was CPT2, which showed no associations with any acylcarnitine.
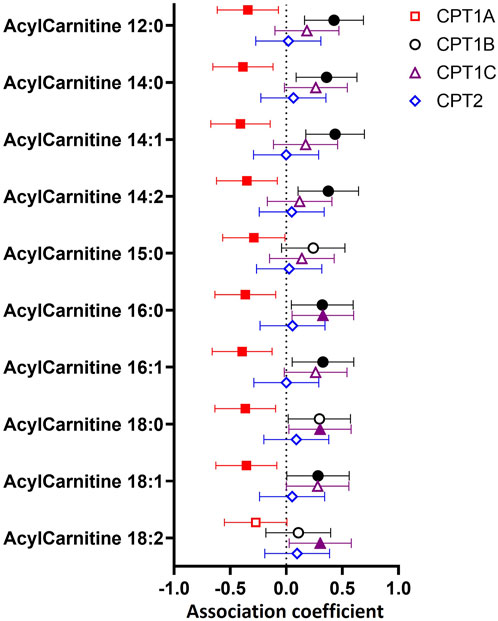
FIGURE 2. Associations between mRNA expression of CPT isoforms and placental acylcarnitines by linear regression. The forest plot shows coefficient estimates and 95% confidence intervals of associations between CPT isoforms and placental acylcarnitines (outcome, n = 50 placentas). Filled symbols show acylcarnitines that are significantly associated after adjustment by Benjamini–Hochberg’s correction. Data for placental acylcarnitine abundance and mRNA expression data of CPT isoforms were log2-transformed then converted to Z-scores prior to linear regression.
3.4 Associations between CPT1B expression and maternal age with placental acylcarnitines following stratification by maternal BMI or GDM status
Both a high maternal BMI (≥25 kg/m2) and GDM are known to increase the supply and availability of fatty acids to the placenta (Duttaroy and Basak, 2021), which could place greater stress on the placenta’s capacity for fatty acid processing. Thus, to determine whether such factors altered the relationships of CPT1B expression and maternal age with placental acylcarnitines, we examined these associations following stratification by BMI (Figure 3) and GDM status (Supplementary Figure S1).
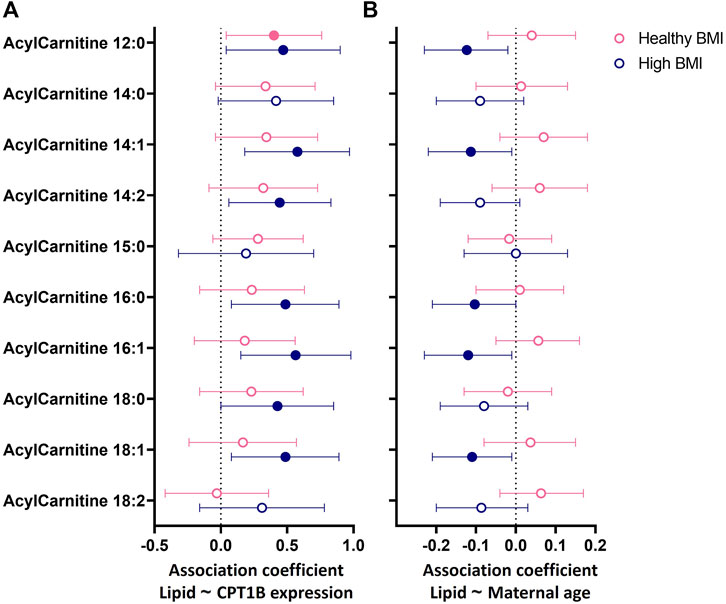
FIGURE 3. Associations between (A) placental CPT1B mRNA expression or (B) maternal age with the abundance of placental acylcarnitines by linear regression stratified by maternal BMI status. The forest plots show coefficient estimates and 95% confidence intervals of associations between placental CPT1B mRNA expression (A) or maternal age (years, (B) with placental acylcarnitines (outcome) in subjects with healthy (<25 kg/m2, n = 25) and high (≥25 kg/m2, n = 25) BMI. Filled symbols show acylcarnitines that are significantly associated after adjustment by Benjamini–Hochberg’s correction. Data for placental acylcarnitine abundance and mRNA expression data of CPT1B were log2-transformed then converted to Z-scores prior to linear regression.
In BMI-stratified analyses (Figure 3), among participants with a healthy BMI (<25 kg/m2; n = 25), CPT1B expression positively associated with only one acylcarnitine (12:0). In contrast, in those with a high BMI (≥25 kg/m2; n = 25), CPT1B expression remained strongly positively associated with seven acylcarnitines (12:0, 14:1, 14:2, 16.0, 16:1, 18:0, 18:1). Only acylcarnitine 12:0 was significantly associated with CPT1B expression with similar coefficient estimates in both BMI groups. Differences by GDM status were less apparent, with the normoglycemic group showing significant positive associations for two acylcarnitines (12:0, 14:1) and the GDM group for three acylcarnitines (14:1, 16:0, 16:1), with an overlap observed for acylcarnitine 14:1 (Supplementary Figure S1A).
Since placental CPT1B expression declined with older maternal age, it was expected that placental acylcarnitines would be negatively associated with age. However, no direct relationships between maternal age and placental acylcarnitines were observed (Supplementary Table S3). Instead, we only observed such a relationship of older maternal age with lower acylcarnitines (12:0, 14:1, 16:0, 16:1, 18:1) in those with a high BMI and not in those with a healthy BMI (Figure 3B). No relationships were seen when stratified by GDM status (Supplementary Figure S1B). The associations seen for the high BMI group remained similar after adjusting for maternal ethnicity, fasting glycemia, gestational age at delivery and infant sex. Following adjustment for placental CPT1B expression, all associations between maternal age and placental acylcarnitines were attenuated and no longer significant.
4 Discussion
4.1 Main findings
Our study demonstrates that older maternal age is associated with lower placental expression of CPT1B, but not that of CPT1A, CPT1C or CPT2. Furthermore, placental CPT1B expression positively associated with eight out of 10 acylcarnitines quantified in the placenta, suggesting it may play a prominent role in placental long-chain acylcarnitine synthesis. Placental acylcarnitines were only reduced with older maternal age in overweight/obese participants. These associations between maternal age and placental acylcarnitines were attenuated after accounting for CPT1B expression.
4.2 Implications of reduced CPT1B expression in the placenta
The inverse relationship between maternal age and placental CPT1B mRNA expression is consistent with past studies in elderly humans and aged rodents demonstrating decreased expression and activity of CPT in tissues such as the heart and skeletal muscles, where the CPT1B is the predominant isoform (McMillin et al., 1993; Odiet et al., 1995; Bétry et al., 2019; Vieira-Lara et al., 2021). Curiously, despite the relatively “young” age of placental tissue (originating from the recent conception), the conceptus and placenta inherits maternal mitochondria—a major site where CPT1B is active. Thus, in certain respects, the placenta may share maternal age-related physiological characteristics.
In a human study, participants with lower skeletal muscle CPT1B mRNA expression were less able to oxidize lipids in a fasted state and were more insulin-resistant (Bétry et al., 2019). In mice, the age-associated decrease in skeletal muscle CPT1B protein exacerbated insulin resistance induced by a high fat diet, indicating that older mice had reduced metabolic flexibility in response to an obesogenic dietary challenge compared with their younger counterparts (Vieira-Lara et al., 2021). Meanwhile, loss of CPT1B activity in the heart increases myocardial lipids in obese mice and causes cardiac lipotoxicity in a heart failure mouse model (He et al., 2012; Zhang et al., 2016). These studies particularly highlight the importance of CPT1B in buffering metabolic stress and its contribution to overall metabolic health. As such, the age-related decline in placental CPT1B may similarly impair the placenta’s ability to appropriately regulate fatty acid oxidation in response to metabolic challenges such as maternal obesity; this could lead to dysregulated placental lipid metabolism and altered lipid-derived signaling, and ultimately placental dysfunction. Nonetheless, while our sample population encompassed a range of maternal BMI and glycemia, our study was restricted to those with relatively uncomplicated pregnancies with a livebirth following an elective cesarean section and thus not representative of the general obstetric population. Therefore, we were unable to test for associations of placental CPT1B expression with adverse pregnancy outcomes such as pre-eclampsia and stillbirth that are linked with placental dysfunction and advanced maternal age. Future studies in large cohorts that are adequately powered could be used to further investigate the link between placental CPT1B expression with these relatively infrequent adverse pregnancy outcomes.
4.3 Significance of the role of maternal BMI in influencing placental fatty oxidation
In addition to advanced maternal age, high BMI is another risk factor for stillbirth and antenatal complications such as GDM (Flenady et al., 2011; Plows et al., 2018). Studies of placentas from women with obesity generally report lower expression of CPTs, reduced acylcarnitines and impaired fatty acid oxidation, although the changes in expression of specific CPT isoforms differed between studies. For instance, Calabuig-Navarro et al. found that obesity increased placental CPT2 mRNA expression, but decreased that of CPT1B and acylcarnitine content (Calabuig-Navarro et al., 2017), while Bucher et al. showed that CPT1A and CPT2 protein expression and acylcarnitines (16:0, 18:2, and 20:4) were reduced only in the placentas of female offspring (female placenta) among women with obesity (Bucher et al., 2021). In contrast, Powell et al. did not observe any changes with placental protein expression of CPTs, though they also demonstrated less fatty acid oxidation in female placentas of women with obesity (Powell et al., 2021). Similarly, we did not identify any associations of maternal BMI or infant sex with placental expression of CPTs. The discrepancies between studies may arise from different BMI cutoffs (i.e. overweight versus obese) and baseline population differences (e.g. Asian and non-Asian). Nonetheless, our finding of a maternal age-associated decline in placental acylcarnitines only among overweight women, provides additional supporting evidence that high BMI may contribute to reduced ability to process excess fatty acids.
Therefore, while maternal age is associated with decreased placental CPT1B expression, this only appears to impact acylcarnitine production when BMI is high, when the placenta is presumably already experiencing an increased fatty acid load. Hence, CPT1B may become the limiting factor in acylcarnitine production in an environment of excess fatty acids. Indeed, placental CPT1B mRNA expression positively associated with more acylcarnitines among overweight participants (BMI ≥25 kg/m2) as compared to just one significant association seen among the non-overweight participants (BMI <25 kg/m2), further highlighting the close relationship between maternal BMI, CPT1B expression and acylcarnitines in the placenta. Moreover, while placental fatty acid oxidation is reportedly reduced with GDM (Visiedo et al., 2013), GDM status had minor implications on the associations of placental CPT1B expression and of maternal age with placental acylcarnitines in our cohort, which suggests that differences in BMI are more important than differences in maternal glycemia.
4.4 Role of other placental CPTs
In addition to being the only CPT associated with age, CPT1B mRNA expression was positively associated with the largest number of placental acylcarnitines, suggesting it may be the main transferase for converting medium and long-chain fatty acids into acylcarnitines in the human placenta. This is similar to a previous finding showing that placental CPT1B mRNA expression positively correlated with total placental acylcarnitine content (Calabuig-Navarro et al., 2017). The positive relationships of placental CPT1C mRNA expression with only the longer chain acylcarnitines (16:0, 18:0 and 18:2) suggests its particular importance in generating the very long chain acylcarnitines. This is corroborated by the localization of CPT1C mainly in the endoplasmic reticulum, hinting at its role in biosynthesis as opposed to catabolism (in mitochondria) and that the loss of CPT1C results in decreased long chain signaling endocannabinoid production (Sierra et al., 2008; Lee and Wolfgang, 2012). Unexpectedly, placental CPT1A expression was negatively associated with acylcarnitines. The underlying reasons remain unclear, but one possibility is that placental increases in CPT1A enhances fatty acid oxidation overall, such that longer chain acylcarnitines become depleted. This is similar to the negative relationship seen in patients with chronic kidney disease, where decreased kidney CPT1A mRNA expression was linked with increased accumulation of short and middle chain acylcarnitines (Miguel et al., 2021). In contrast, CPT2 was not associated with any placental acylcarnitine in our cohort, which suggests it is not the limiting factor in the placenta for synthesis of the medium and long-chain acylcarnitines examined. Nonetheless, as there are no inhibitors currently available to selectively block the activity of each CPT in isolation, we are limited in our ability to determine the specific role of each CPT in vitro studies of the human placenta.
4.5 Possible mechanisms for CPT1B decline with age and potential reversal with carnitine supplementation
The mechanisms by which maternal age affects placental CPT1B expression are unknown. However, insights may be gained from non-placental studies. For example, decreased CPT1 expression with increasing age in tissues such as the heart and skeletal muscle is speculated to result from cumulative mitochondrial oxidative damage over time (Odiet et al., 1995; Vieira-Lara et al., 2021). As such, oxidative damage accumulated in the maternal mitochondria of the aging oocyte that are subsequently inherited by the fetus may be one contributing factor. In vitro studies conducted on placental explants show that acute oxidative stress of up to 4 h does not affect placental CPT1B expression at the mRNA or protein level (Thomas et al., 2019), but the effects of chronic oxidative stress remain to be investigated. Direct signaling from the maternal tissues to the placenta may also influence placental CPT expression, given that advanced maternal age can impair decidualisation and thus alter the biochemical and hormonal environment that the developing placenta is exposed to (Woods et al., 2017; Mendes et al., 2020). Alternatively, a decline in CPT may be due to deficiency of micronutrients needed for optimal fatty acid oxidation. For instance, in conjunction with the age-associated drop in CPT (Bétry et al., 2019; Vieira-Lara et al., 2021), skeletal muscle carnitine content also decreases with age in humans and mice (Costell et al., 1989). Interestingly, carnitine supplementation was able to enhance CPT1 transcription in the liver of aged rats (Karlic et al., 2002). In humans, pregnancy also results in a decline in circulating carnitine (Keller et al., 2009), and dietary carnitine supplementation can increase hepatic CPT1B activity in pregnant pigs (Xi et al., 2008). Furthermore, carnitine supplementation was previously shown to decrease the stillbirth rate in sows (Eder, 2009). Exercise can also increase skeletal muscle and adipose tissue CPT1B mRNA expression in young and middle adults across the BMI spectrum (Lohninger et al., 2005; Otero-Díaz et al., 2018) but whether exercise in pregnancy can increase placental CPT1B remains to be investigated. The notion that maternal physical activity can influence fetal-placental tissues at a molecular level was suggested in a study by Chaves et al. (Chaves et al., 2022), which demonstrated that maternal exercise altered metabolism in isolated umbilical cord mesenchymal stromal cells. It is thus tantalizing to speculate that the CPT-promoting effects of carnitine supplementation and exercise individually or in combination may be able to counter the age-associated placental CPT decline in pregnancy and possibly reduce advanced maternal age-linked stillbirths and other pregnancy adversity, which could be explored in future studies. Therefore, further studies are warranted to improve understanding of CPT regulation at the maternal-fetal interface.
5 Conclusion
In summary, older maternal age is specifically associated with lower placental CPT1B expression and CPT1B appears to be the main CPT that catalyzes acylcarnitine production in the placenta. However, placental acylcarnitines are only lower with older maternal age in overweight/obese women. These findings may underlie decreased placental metabolic flexibility and ability to adapt to adverse intrauterine environments, which may contribute to greater risk of pregnancy complications in older women, particularly if they are overweight/obese.
Data availability statement
The raw data supporting the conclusion of this article will be made available by the authors upon reasonable request.
Ethics statement
The studies involving human participants were reviewed and approved by the National Healthcare Group Domain Specific Research Board. The patients/participants provided their written informed consent to participate in this study.
Author contributions
Conception and/or study design HY, OW, and S-YC. Data acquisition: HY, OW, TM, VC-H, RP, PS, MI, and NS. Data analysis and interpretation: HY, OW, TM, VC-H, and S-YC. Drafting the manuscript HY and OW. Critical revision of the manuscript for intellectual content: HY, OW, TM, VC-H, RP, PS, MI, NS, AC-G, AB, MW, KG, RL, and S-YC. Funding acquisition: AC-G, AB, MW, KG, RL, and S-YC. All authors have approved the submitted version of the manuscript.
Funding
This research is supported by a Clinician Scientist Award awarded to SYC from the Singapore National Medical Research Council (NMRC/CSA-INV/0010/2016, MOH-CSAINV19nov-0002), by the National University of Singapore, National University Health System Singapore and the Singapore Institute for Clinical Sciences A*STAR. The Singapore Lipidomics Incubator receives funding from the Life Sciences Institute, the National University of Singapore Yong Loo Lin School of Medicine, the National Research Foundation (NRFI 2015–05) and A*STAR (IAF-ICP I1901E0040). KMG is supported by the United Kingdom Medical Research Council (MC_UU_12011/4), the National Institute for Health Research (NIHR Senior Investigator (NF-SI-0515–10042) and NIHR Southampton Biomedical Research Centre (NIHR203319)), the European Union (Erasmus + Programme ImpENSA 598488-EPP-1–2018-1-DE-EPPKA2-CBHE-JP), and the US National Institute On Aging of the National Institutes of Health (Award No. U24AG047867). For the purpose of Open Access, the authors have applied a Creative Commons Attribution (CC BY) licence to any Author Accepted Manuscript version arising from this submission. Funders played no role in study design; in the collection, analysis, and interpretation of data; in the writing of the report; and in the decision to submit the paper for publication.
Acknowledgments
The authors would like to thank Samantha Grace Loon Magadia, Celes Maria Catherine Dado and Zhenzhi Chen in coordinating the recruitment of the women involved in this study, the staff of the National University Hospital who kindly assisted with placental collection, and the women who generously donated their placentas for research.
Conflict of interest
S-YC and KG are part of an academic consortium that has received research funding from Société Des Produits Nestlé S.A. and BenevolentAI Bio Ltd for work unrelated to this manuscript. S-YC and KMG are co-inventors on patent filings by Nestlé S.A. which covers the use of inositol in human health applications, but which do not draw on the work in this manuscript.
The remaining authors declare that the research was conducted in the absence of any commercial or financial relationships that could be construed as a potential conflict of interest.
Publisher’s note
All claims expressed in this article are solely those of the authors and do not necessarily represent those of their affiliated organizations, or those of the publisher, the editors and the reviewers. Any product that may be evaluated in this article, or claim that may be made by its manufacturer, is not guaranteed or endorsed by the publisher.
Supplementary material
The Supplementary Material for this article can be found online at: https://www.frontiersin.org/articles/10.3389/fphys.2023.1166827/full#supplementary-material
References
Arduini, A., Denisova, N., Virmani, A., Avrova, N., Federici, G., and Arrigoni-Martelli, E. (1994). Evidence for the involvement of carnitine-dependent long-chain acyltransferases in neuronal triglyceride and phospholipid fatty acid turnover. J. Neurochem. 62 (4), 1530–1538. doi:10.1046/j.1471-4159.1994.62041530.x
Arduini, A., Mancinelli, G., Radatti, G. L., Dottori, S., Molajoni, F., and Ramsay, R. R. (1992). Role of carnitine and carnitine palmitoyltransferase as integral components of the pathway for membrane phospholipid fatty acid turnover in intact human erythrocytes. J. Biol. Chem. 267 (18), 12673–12681. doi:10.1016/s0021-9258(18)42330-7
Bétry, C., Meugnier, E., Pflieger, M., Grenet, G., Hercberg, S., Galan, P., et al. (2019). High expression of CPT1b in skeletal muscle in metabolically healthy older subjects. Diabetes & Metabolism 45 (2), 152–159. doi:10.1016/j.diabet.2018.01.018
Bucher, M., Montaniel, K. R. C., Myatt, L., Weintraub, S., Tavori, H., and Maloyan, A. (2021). Dyslipidemia, insulin resistance, and impairment of placental metabolism in the offspring of obese mothers. J. Dev. Orig. Health Dis. 12 (5), 738–747. doi:10.1017/S2040174420001026
Calabuig-Navarro, V., Haghiac, M., Minium, J., Glazebrook, P., Ranasinghe, G. C., Hoppel, C., et al. (2017). Effect of maternal obesity on placental lipid metabolism. Endocrinology 158 (8), 2543–2555. doi:10.1210/en.2017-00152
Ceccarelli, S. M., Chomienne, O., Gubler, M., and Arduini, A. (2011). Carnitine palmitoyltransferase (CPT) modulators: A medicinal chemistry perspective on 35 years of research. J. Med. Chem. 54 (9), 3109–3152. doi:10.1021/jm100809g
Chaves, A., Weyrauch, L. A., Zheng, D., Biagioni, E. M., Krassovskaia, P. M., Davidson, B. L., et al. (2022). Influence of maternal exercise on glucose and lipid metabolism in offspring stem cells: ENHANCED by mom. J. Clin. Endocrinol. Metab. 107 (8), e3353–e3365. doi:10.1210/clinem/dgac270
Costell, M., O'Connor, J. E., and Grisolía, S. (1989). Age-dependent decrease of carnitine content in muscle of mice and humans. Biochem. Biophysical Res. Commun. 161 (3), 1135–1143. doi:10.1016/0006-291x(89)91360-0
Duttaroy, A. K., and Basak, S. (2021). Maternal fatty acid metabolism in pregnancy and its consequences in the feto-placental development. Front. Physiol. 12, 787848. doi:10.3389/fphys.2021.787848
Eder, K. (2009). Influence of l-carnitine on metabolism and performance of sows. Br. J. Nutr. 102 (5), 645–654. doi:10.1017/S0007114509990778
El-Wahed, M. A., El-Farghali, O., ElAbd, H., El-Desouky, E., and Hassan, S. (2017). Metabolic derangements in IUGR neonates detected at birth using UPLC-MS. Egypt. J. Med. Hum. Genet. 18 (3), 281–287. doi:10.1016/j.ejmhg.2016.12.002
Flenady, V., Koopmans, L., Middleton, P., Froen, J. F., Smith, G. C., Gibbons, K., et al. (2011). Major risk factors for stillbirth in high-income countries: A systematic review and meta-analysis. Lancet 377 (9774), 1331–1340. doi:10.1016/S0140-6736(10)62233-7
Giannacopoulou, C., Evangeliou, A., Matalliotakis, I., Relakis, K., Sbirakis, N., Hatzidaki, E., et al. (1998). Effects of gestation age and of birth weight in the concentration of carnitine in the umbilical plasma. Clin. Exp. Obstetrics Gynaecol. 25 (1), 42–45.
He, L., Kim, T., Long, Q., Liu, J., Wang, P., Zhou, Y., et al. (2012). Carnitine palmitoyltransferase-1b deficiency aggravates pressure overload-induced cardiac hypertrophy caused by lipotoxicity. Circulation 126 (14), 1705–1716. doi:10.1161/CIRCULATIONAHA.111.075978
Houten, S. M., Wanders, R. J. A., and Ranea-Robles, P. (2020). Metabolic interactions between peroxisomes and mitochondria with a special focus on acylcarnitine metabolism. Biochimica Biophysica Acta (BBA) - Mol. Basis Dis. 1866 (5), 165720. doi:10.1016/j.bbadis.2020.165720
Huang, L., Sauve, R., Birkett, N., Fergusson, D., and van Walraven, C. (2008). Maternal age and risk of stillbirth: A systematic review. Can. Med. Assoc. J. 178 (2), 165–172. doi:10.1503/cmaj.070150
Jones, L L., McDonald, D. A., and Borum, P. R. (2010). Acylcarnitines: Role in brain. Prog. Lipid Res. 49 (1), 61–75. doi:10.1016/j.plipres.2009.08.004
Karlic, H., Lohninger, A., Laschan, C., Lapin, A., Böhmer, F., Huemer, M., et al. (2003). Downregulation of carnitine acyltransferases and organic cation transporter OCTN2 in mononuclear cells in healthy elderly and patients with myelodysplastic syndromes. J. Mol. Med. 81 (7), 435–442. doi:10.1007/s00109-003-0447-6
Karlic, H., Lohninger, S., Koeck, T., and Lohninger, A. (2002). Dietary L-carnitine stimulates carnitine acyltransferases in the liver of aged rats. J. Histochem. Cytochem. 50 (2), 205–212. doi:10.1177/002215540205000208
Keller, U., van der Wal, C., Seliger, G., Scheler, C., Röpke, F., and Eder, K. (2009). Carnitine status of pregnant women: Effect of carnitine supplementation and correlation between iron status and plasma carnitine concentration. Eur. J. Clin. Nutr. 63 (9), 1098–1105. doi:10.1038/ejcn.2009.36
Kim, T., Moore, J. F., Sharer, J. D., Yang, K., Wood, P. A., and Yang, Q. (2014). Carnitine palmitoyltransferase 1b deficient mice develop severe insulin resistance after prolonged high fat diet feeding. J. diabetes & metabolism 5, 1000401. doi:10.4172/2155-6156.1000401
Kolb, H., Kempf, K., Röhling, M., Lenzen-Schulte, M., Schloot, N. C., and Martin, S. (2021). Ketone bodies: From enemy to friend and guardian angel. BMC Med. 19 (1), 313–315. doi:10.1186/s12916-021-02185-0
Lahjouji, K., Elimrani, I., Lafond, J., Leduc, L., Qureshi, I. A., and Mitchell, G. A. (2004). L-Carnitine transport in human placental brush-border membranes is mediated by the sodium-dependent organic cation transporter OCTN2. Am. J. Physiology-Cell Physiology 287 (2), C263–C269. doi:10.1152/ajpcell.00333.2003
Lean, S. C., Derricott, H., Jones, R. L., and Heazell, A. E. P. (2017). Advanced maternal age and adverse pregnancy outcomes: A systematic review and meta-analysis. PLoS One 12 (10), e0186287. doi:10.1371/journal.pone.0186287
Lee, J., and Wolfgang, M. J. (2012). Metabolomic profiling reveals a role for CPT1c in neuronal oxidative metabolism. BMC Biochem. 13 (1), 23. doi:10.1186/1471-2091-13-23
Lohninger, A., Sendic, A., Litzlbauer, E., Hofbauer, R., Staniek, H., Blesky, D., et al. (2005). Endurance exercise training and L-carnitine supplementation stimulates gene expression in the blood and muscle cells in young athletes and middle aged subjects. Monatsh. für Chem./Chem. Mon. 136 (8), 1425–1442. doi:10.1007/s00706-005-0335-6
McMillin, J. B., Taffet, G. E., Taegtmeyer, H., Hudson, E. K., and Tate, C. A. (1993). Mitochondrial metabolism and substrate competition in the aging Fischer rat heart. Cardiovasc. Res. 27 (12), 2222–2228. doi:10.1093/cvr/27.12.2222
Mendes, S., Timoteo-Ferreira, F., Soares, A. I., Rodrigues, A. R., Silva, A. M. N., Silveira, S., et al. (2020). Age-related oxidative modifications to uterine albumin impair extravillous trophoblast cells function. Free Radic. Biol. Med. 152, 313–322. doi:10.1016/j.freeradbiomed.2020.03.020
Miguel, V., Tituaña, J., Herrero, J. I., Herrero, L., Serra, D., Cuevas, P., et al. (2021). Renal tubule Cpt1a overexpression protects from kidney fibrosis by restoring mitochondrial homeostasis. J. Clin. Investigation 131 (5), e140695. doi:10.1172/JCI140695
Novak, M., Monkus, E. F., Chung, D., and Buch, M. (1981). Carnitine in the perinatal metabolism of lipids I. Relationship between maternal and fetal plasma levels of carnitine and acylcarnitines. Pediatrics 67 (1), 95–100. doi:10.1542/peds.67.1.95
Odiet, J A., Boerrigter, M. E. T. I., and Wei, J. Y. (1995). Carnitine palmitoyl transferase-I activity in the aging mouse heart. Mech. Ageing Dev. 79 (2), 127–136. doi:10.1016/0047-6374(94)01552-w
Otero-Díaz, B., Rodríguez-Flores, M., Sánchez-Muñoz, V., Monraz-Preciado, F., Ordoñez-Ortega, S., Becerril-Elias, V., et al. (2018). Exercise induces white adipose tissue browning across the weight spectrum in humans. Front. Physiology 9, 1781. doi:10.3389/fphys.2018.01781
Plows, J. F., Stanley, J. L., Baker, P. N., Reynolds, C. M., and Vickers, M. H. (2018). The pathophysiology of gestational diabetes mellitus. Int. J. Mol. Sci. 19 (11), 3342. doi:10.3390/ijms19113342
Powell, T. L., Barner, K., Madi, L., Armstrong, M., Manke, J., Uhlson, C., et al. (2021). Sex-specific responses in placental fatty acid oxidation, esterification and transfer capacity to maternal obesity. Biochim. Biophys. Acta Mol. Cell. Biol. Lipids 1866 (3), 158861. doi:10.1016/j.bbalip.2020.158861
Saccone, G., Gragnano, E., Ilardi, B., Marrone, V., Strina, I., Venturella, R., et al. (2022). Maternal and perinatal complications according to maternal age: A systematic review and meta-analysis. Int. J. Gynecol. Obstetrics 159, 43–55. doi:10.1002/ijgo.14100
Sánchez-Pintos, P., de Castro, M. J., Roca, I., Rite, S., López, M., and Couce, M. L. (2017). Similarities between acylcarnitine profiles in large for gestational age newborns and obesity. Sci. Rep. 7 (1), 16267–16269. doi:10.1038/s41598-017-15809-4
Sánchez-Pintos, P., Perez-Munuzuri, A., Ja, C., Fernández-Lorenzo, J. R., Fraga, J. M., and Couce, M. L. (2016). Evaluation of carnitine deficit in very low birth weight preterm newborns small for their gestational age. J. Maternal-Fetal Neonatal Med. 29 (6), 933–937. doi:10.3109/14767058.2015.1024647
Schmidt-Sommerfeld, E., Penn, D., Sodha, R. J., Prögler, M., Novak, M., and Schneider, H. (1985). Transfer and metabolism of carnitine and carnitine esters in the in vitro perfused human placenta. Pediatr. Res. 19 (7), 700–706. doi:10.1203/00006450-198507000-00013
Sierra, A. Y., Gratacós, E., Carrasco, P., Clotet, J., Ureña, J., Serra, D., et al. (2008). CPT1c is localized in endoplasmic reticulum of neurons and has carnitine palmitoyltransferase activity. J. Biol. Chem. 283 (11), 6878–6885. doi:10.1074/jbc.M707965200
Thomas, M. M., Haghiac, M., Grozav, C., Minium, J., Calabuig-Navarro, V., and O'Tierney-Ginn, P. (2019). Oxidative stress impairs fatty acid oxidation and mitochondrial function in the term placenta. Reprod. Sci. 26 (7), 972–978. doi:10.1177/1933719118802054
Vieira-Lara, M. A., Dommerholt, M. B., Zhang, W., Blankestijn, M., Wolters, J C., Abegaz, F., et al. (2021). Age-related susceptibility to insulin resistance arises from a combination of CPT1B decline and lipid overload. BMC Biol. 19 (1), 154. doi:10.1186/s12915-021-01082-5
Virmani, A., Pinto, L., Bauermann, O., Zerelli, S., Diedenhofen, A., Binienda, Z. K., et al. (2015). The carnitine palmitoyl transferase (CPT) System and possible relevance for neuropsychiatric and neurological conditions. Mol. Neurobiol. 52 (2), 826–836. doi:10.1007/s12035-015-9238-7
Visiedo, F., Bugatto, F., Sanchez, V., Cozar-Castellano, I., Bartha, J. L., and Perdomo, G. (2013). High glucose levels reduce fatty acid oxidation and increase triglyceride accumulation in human placenta. Am. J. Physiol. Endocrinol. Metab. 305 (2), E205–E212. doi:10.1152/ajpendo.00032.2013
Watkins, O. C., Yong, H. E. J., Mah, T. K. L., Cracknell-Hazra, V. K. B., Pillai, R. A., Selvam, P., et al. (2022). Sex-dependent regulation of placental oleic acid and palmitic acid metabolism by maternal glycemia and associations with birthweight. Int. J. Mol. Sci. 23 (15), 8685. doi:10.3390/ijms23158685
Wickham, H., Averick, M., Bryan, J., Chang, W., McGowan, L. D A., François, R., et al. (2019). Welcome to the tidyverse. J. open source Softw. 4 (43), 1686. doi:10.21105/joss.01686
Wong, G., Weir, J. M., Mishra, P., Huynh, K., Nijagal, B., Gupta, V., et al. (2021). The placental lipidome of maternal antenatal depression predicts socio-emotional problems in the offspring. Transl. Psychiatry 11 (1), 107. doi:10.1038/s41398-021-01208-x
Woods, L., Perez-Garcia, V., Kieckbusch, J., Wang, X., DeMayo, F., Colucci, F., et al. (2017). Decidualisation and placentation defects are a major cause of age-related reproductive decline. Nat. Commun. 8 (1), 352. doi:10.1038/s41467-017-00308-x
World Health Organization Guideline (2014). Diagnostic criteria and classification of hyperglycaemia first detected in pregnancy: A World health organization guideline. Diabetes Res. Clin. Pract. 103 (3), 341–363. doi:10.1016/j.diabres.2013.10.012
Xi, L., Brown, K., Woodworth, J., Shim, K., Johnson, B., and Odle, J. (2008). Maternal dietary L-carnitine supplementation influences fetal carnitine status and stimulates carnitine palmitoyltransferase and pyruvate dehydrogenase complex activities in swine. J. Nutr. 138 (12), 2356–2362. doi:10.3945/jn.108.095638
Yli, B. M., and Kjellmer, I. (2016). Pathophysiology of foetal oxygenation and cell damage during labour. Best Pract. Res. Clin. Obstetrics Gynaecol. 30, 9–21. doi:10.1016/j.bpobgyn.2015.05.004
Keywords: placenta, maternal age, lipid metabolism, carnitine palmitoyltransferases, obesity, overweight, CPT1B
Citation: Yong HEJ, Watkins OC, Mah TKL, Cracknell-Hazra VKB, Pillai RA, Selvam P, Islam MO, Sharma N, Cazenave-Gassiot A, Bendt AK, Wenk MR, Godfrey KM, Lewis RM and Chan S-Y (2023) Increasing maternal age associates with lower placental CPT1B mRNA expression and acylcarnitines, particularly in overweight women. Front. Physiol. 14:1166827. doi: 10.3389/fphys.2023.1166827
Received: 21 February 2023; Accepted: 04 May 2023;
Published: 18 May 2023.
Edited by:
Gendie Lash, Guangzhou Medical University, ChinaReviewed by:
Céline Aguer, McGill University, CanadaPerrie O'Tierney-Ginn, Tufts Medical Center, United States
Copyright © 2023 Yong, Watkins, Mah, Cracknell-Hazra, Pillai, Selvam, Islam, Sharma, Cazenave-Gassiot, Bendt, Wenk, Godfrey, Lewis and Chan. This is an open-access article distributed under the terms of the Creative Commons Attribution License (CC BY). The use, distribution or reproduction in other forums is permitted, provided the original author(s) and the copyright owner(s) are credited and that the original publication in this journal is cited, in accordance with accepted academic practice. No use, distribution or reproduction is permitted which does not comply with these terms.
*Correspondence: Shiao-Yng Chan, obgchan@nus.edu.sg
†These authors have contributed equally to this work