- 1Multicenter Postgraduate Program in Physiological Sciences, State University of Londrina, Londrina, Brazil
- 2Postgraduate Program in Physiological Sciences, State University of Londrina, Londrina, Brazil
- 3Department of Physiological Sciences, State University of Londrina, Londrina, Brazil
- 4Department of Physiology and Biophysics, University of Sao Paulo, Sao Paulo, Brazil
- 5Department of Chemistry, State University of Londrina, Londrina, Brazil
Introduction: Lactation overnutrition is a programming agent of energy metabolism, and litter size reduction leads to the early development of obesity, which persists until adulthood. Liver metabolism is disrupted by obesity, and increased levels of circulating glucocorticoids are pointed as a possible mediator for the obesity development, since bilateral adrenalectomy (ADX) can reduce obesity in different models of obesity.
Methods: This study aimed to evaluate the effects of glucocorticoids on metabolic changes and liver lipogenesis and insulin pathway induced by lactation overnutrition. For this, on the postnatal day 3 (PND), 3 pups (small litter—SL) or 10 pups (normal litter—NL) were kept with each dam. On PND 60, male Wistar rats underwent bilateral adrenalectomy (ADX) or fictitious surgery (sham), and half of ADX animals received corticosterone (CORT- 25 mg/L) diluted in the drinking fluid. On PND 74, the animals were euthanized by decapitation for trunk blood collection, and liver dissection and storage.
Results and Discussion: SL rats presented increased corticosterone, free fatty acids, total and LDL-cholesterol plasma levels, without changes in triglycerides (TG) and HDL-cholesterol. The SL group also showed increased content of liver TG, and expression of fatty acid synthase (FASN), but decreased expression of PI3Kp110 in the liver, compared to NL rats. In the SL group, the ADX decreased plasma levels of corticosterone, FFA, TG and HDL cholesterol, liver TG, and liver expression of FASN, and IRS2, compared to sham animals. In SL animals, CORT treatment increased plasma levels of TG and HDL cholesterol, liver TG, and expression of FASN, IRS1, and IRS2, compared with the ADX group. In summary, the ADX attenuated plasma and liver changes observed after lactation overnutrition, and CORT treatment could reverse most ADX-induced effects. Thus, increased circulating glucocorticoids are likely to play a pivotal role in liver and plasma impairments induced by lactation overnutrition in male rats.
Introduction
The nutritional or hormonal setting during fetal and/or postnatal periods plays an important modulatory role. It is known that the development of several organs is not completed at birth and it often continues during the lactation period (Ellsworth et al., 2018). So, this window may be considered a vulnerable time for offspring, once the systems are plastic and sensitive to changes in the environment, which will result in effects on adult life, as well-established by the “Developmental origins of health and disease” (DOHaD) concept (Barker, 1998).
Food intake during the neonatal period is a relevant factor for the development of obesity in adulthood (Fiorotto et al., 1991). In this context, the litter size reduction method in rodents is an important tool to observe the long-term effects of lactation overnutrition as demonstrated for the first time by Kennedy, 1957 (Plagemann, 2006). This method consists of allocating the pups in smaller litters (SL), with 3 pups/dam compared with the control with 10 pups/dam. Then, the animals from SL develop earlier obesity which persists until adulthood (Plagemann, 2006; Spencer, 2013).
One of the comorbidities of obesity is triglycerides (TG) accumulation in the liver, which occurs from an imbalance between lipid input, as fatty acid uptake and de novo lipogenesis (DNL), and lipid output as very low-density lipoprotein (VLDL) (Donnelly et al., 2005; Bradburry, 2006; Doege and Stahl, 2006). Under physiological conditions, the concentration of hepatic TG is low, since the liver is not a fat storage organ. Therefore, in a condition of excess of carbohydrates, the liver produces fatty acids by DNL and exports triglyceride as VLDL (Donnelly et al., 2005; Doege and Stahl, 2006; Bradburry, 2006; Nestel et al., 1962; Cohen et al., 2011; Kawano and Cohen, 2013).
Obesity is associated TG accumulation in the liver, once the lipid storage capacity of an expanded adipose tissue is limited, and the excess of lipids, and TG are stored in hepatocytes. The sources of TG in the liver are increased lipolysis (approximately 60%) and DNL in the liver (25%) (Donnelly et al., 2005). In this context, the DNL consists in synthesizing non-esterified fatty acids from glucose. For this, glucose is converted to acetyl-CoA through glycolysis, and acetyl-CoA is then converted to malonyl-CoA by acetyl-CoA carboxylase (ACC). Fatty acid synthase enzyme (FASN) catalyzes the formation of palmitic acid from malonyl-CoA and acetyl-CoA. Palmitic acid is then elongated and desaturated to generate monounsaturated fatty acids, which are the major fatty acid constituents of TG. Acyl-CoAs are esterified to form diacylglycerol, which is esterified to another acyl-CoA molecule to form triglyceride by the acyl-CoA diacylglycerol acyltransferase (DGAT), which seems to be especially important for VLDL production (Wurie et al., 2012; Heeren and Scheja, 2021). Another important molecule in DNL is the Apolipoprotein B100 (Apo B100), which is synthesized by hepatocytes and has a unique feature of the ApoB molecule the ability to interact with lipid species (phospholipids, cholesterol, TG, and cholesteryl esters) used to arrangement of VLDL (Rutledge et al., 2010).
Lipid accumulation in the liver has a relationship with fetal and neonatal programming impairment in liver lipid metabolism (Oben et al., 2010). Animals with childhood obesity present metabolic liver dysfunctions such as TG accumulation (Oben et al., 2010), increased circulating free fatty acids, hyperinsulinemia, impairment of the insulin signaling pathway in the liver and skeletal muscle, in addition to a higher concentration of circulating glucocorticoids (Hou et al., 2011), once that hypothalamic-pituitary-adrenal axis (HPA) of these animals matured more quickly (Boullu-ciocca et al., 2005; Spencer and Tilbrook, 2009). According to the literature, adrenal glucocorticoids have an important role in energy homeostasis. Glucocorticoids are known to increase appetite and body weight in humans and rodents (Tataranni et al., 1996; Zakrzewska et al., 1999), and their excess can increase central adiposity, as seen in Cushing’s syndrome (Rebuffé-Scrive et al., 1988). Different models of obesity have increased plasma concentrations of glucocorticoids, and conversely, bilateral removal of the adrenal glands, adrenalectomy (ADX), can attenuate or prevent the development of obesity (Bruce et al., 1982; Yukimura et al., 1978; Dubuc and Wilden, 1986). Within lipid metabolism, glucocorticoids act in the synthesis and oxidation of fatty acids, the esterification of fatty acids to triglyceride and phospholipid, and the outward hepatic transport of lipoprotein lipids (Klausner and Heimberg, 1957).
Thus, it is known that: 1) glucocorticoids are pointed as possible mediators for obesity development and its comorbidities in different experimental models; 2) animals with obesity due to litter reduction show metabolic liver dysfunctions and increased circulating glucocorticoids; 3) the litter size reduction method is a model that leads to obesity and its comorbidities; 4) ADX reverses several anabolic effects in models of obesity. Therefore, this study hypothesized that glucocorticoids contribute to dyslipidemia and metabolic liver dysfunctions in adult male rats induced by neonatal obesity. For the investigation of this hypothesis, the current work aimed to evaluate the effects of ADX on biometric parameters, plasma lipid profile, lipid metabolism and insulin signaling pathway in the liver in adult male animals reared in small litters.
Materials and methods
Animals
Male Wistar rats (n = 77) were obtained from the mating of 37 females with males from the Animal Facility of State University of Londrina (UEL). Of these 37 females, the pregnancy was confirmed by the presence of sperm on the vaginal smear of 33 females (89%). The adjustment of the litter size occurred on the postnatal day (PND) 3, with PND 0 considered the day of birth. The lactation overnutrition was induced remaining with dam 3 pups, 2 males and 1 female to compose the small litter (SL), and the normal litter (NL) remained with dam 10 pups, 5 males and 5 females (Stopa et al., 2021). The surplus pups were anesthetized with an association of ketamine (100 mg/kg, 10%, Agener União, Apucarana, Brazil) and xylazine hydrochloride (20 mg/kg, 2%, Anasedan®, Vetbrands, Jacareí, Brazil) intraperitoneally, and euthanized by decapitation. After weaning, the dams were also anesthetized with the above-mentioned association of ketamine and xylazine hydrochloride intraperitoneally, and euthanized by decapitation, the female pups were used in other experiments and the male pups were used in the current study and were housed in groups of 3–4 rats of the same experimental group in each cage. The animals were kept in controlled conditions of light (12 h light/dark cycle: 6 a.m. to 6p.m./6 p.m. to 6 a.m.) and temperature (22 ± 2°C), with fluid and feed ad libitum, except for the hours of feed restriction before the euthanasia. All experiments were performed at the Department of Physiological Sciences/UEL. The experimental procedures were approved by the Ethics Committee on Animal Use for experimentation (CEUA number: 3457.2109.11, Of. Circ. CEUA 60/2019).
Adrenalectomy or fictitious surgery (sham)
Bilateral ADX and sham surgeries were performed under anesthesia with the association of ketamine (100 mg/kg, Agener União) and xylazine hydrochloride (20 mg/kg, Anasedan®, Vetbrands, Jacareí, Brazil, 2%) intraperitoneal, and a single dorsal midline incision on the skin and a bilateral small cut through the muscle layer was made. After the surgery and during the experimental period, ADX animals were given 0.9% saline with 0.5% ethanol, without corticosterone (ADX) or with corticosterone (B: corticosterone, Sigma Co., CA) (ADX + B) at the concentration of 25 mg/L (Uchoa et al., 2009a; Uchoa et al., 2009b; Uchoa et al., 2010; Uchoa et al., 2012). There was about 15% of mortality of animals submitted to ADX surgery during or after the first days of the procedure. Sham-operated animals underwent similar surgical procedures without removal of adrenal glands and were given tap water with 0.5% ethanol to drink.
Experiment protocol
On PND 60, animals of both litters were submitted to bilateral adrenalectomy (ADX) or sham surgery (fictitious surgery). After the surgery, the animals were kept with access to the solution of water containing 0.5% ethanol, or 0.9% NaCl containing 0.5% ethanol, or 0.9% NaCl containing corticosterone (25 mg/L) diluted in ethanol 0.5% for 14 days, according to the experimental group (NL-sham; SL-sham; NL- ADX; SL-ADX; NL- ADX + B and SL-ADX + B). All animals were weighed, and the amount of food ingested was daily evaluated for a period of 14 days. On PND 74, the rats were weighed, and the nasoanal length was measured, for Lee index determination (Bernardis and Patterson, 1968), and the animals were kept under food restriction from 8 a.m. to 2 p.m. and non-anesthetized animals were euthanized by decapitation at 2 p.m. (Figure 1). After euthanasia, blood was collected to assess plasma concentrations of corticosterone, free fatty acids (FFA), TG, total, HDL and LDL cholesterol. The liver was removed, and the left lobe was stored for TG content and western blotting analyses.
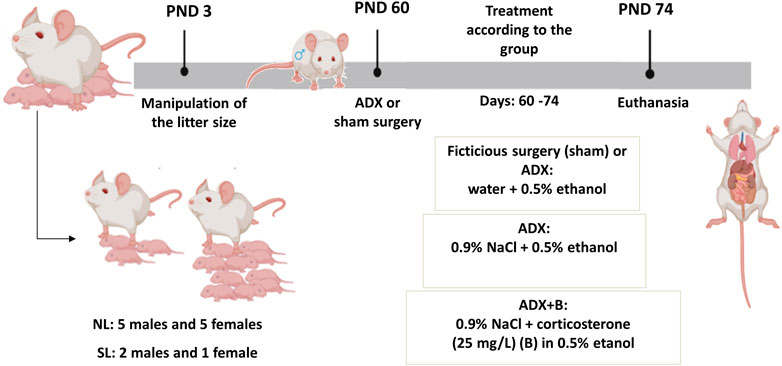
FIGURE 1. Experimental design of protocol. ADX: adrenalectomy; NL: normal litter; SL: small litter; PND: postnatal day.
Euthanasia
For the purpose to avoid hormonal and biochemical changes induced by anesthesia (Guarino et al., 2013; Windeløv et al., 2016), the euthanasia was performed by decapitation in non-anesthetized animals after 6 h feed restriction, as approved by the Ethics Committee on Animal Use for experimentation (CEUA number: 3457.2109.11, Of. Circ. CEUA 60/2019). The blood was immediately collected in heparinized tubes and centrifuged at 14,000 ×g for 20 min at 4°C to plasma obtention, which was stored at −20°C for biochemical analysis.
Measurement of plasma concentration of corticosterone, FFA, TG, total, HDL, and LDL cholesterol
The determination of plasma concentrations of total cholesterol, HDL cholesterol (commercial kits, VIDA biotechnology, MG), LDL cholesterol (commercial kits, Wiener lab., Argentina), TG (commercial kits, Laborclin, PR) and FFA (Falholt et al., 1973) were performed by the spectrophotometric method. The Fluorimetric method of Guillemin et al. (1958) was used to determine corticosterone (de Souza et al., 2019; Stopa et al., 2019; Stopa et al., 2021).
Analysis of liver TG content
To measure the liver TG content, lipids were extracted from liver samples with chloroform-methanol by the Folch method (Folch et al., 1957) as previously described (de Fatima Silva et al., 2022). The TG were determined by enzymatic assay (commercial kit, Labtest, Lagoa Santa, MG, Brazil) and expressed by mg of TG by 100 mg of the liver.
Protein expression by western blotting in the liver
Liver samples were homogenized in extraction buffer (135 mM NaCl, 2.7 mM KCl, 1 mM MgCl2, 10 mM EDTA, 5 mM Na4P2O7, 10 mM NaF, 1% Triton X-100, 10% Glycerol, 20 mM Tris, 2 mM PMSF, 2.5 mM Na3VO4, cOmplete™ tablets, and pH 7.4) to obtain the total protein fraction. The homogenate was centrifuged (12.000 rpm, 40 min, 0°C), and the total proteins of the supernatant were quantified by the Bradford method (Bradford, 1976).
In all analyzes, equal amounts of total proteins (35 µg) plus Laemmli buffer (BioRad, #1610747) were added to 8% polyacrylamide gel, submitted to electrophoresis, and transferred to nitrocellulose membrane. The membranes were blocked with 4% BSA diluted in phosphate-buffered saline with 0.1% Tween 20 (PBST) and incubated overnight (4°C) with the primary antibody diluted in 4% BSA in PBST, as detailed in Supplementary File S2. On the next day, membranes were rinsed (4 × 5 min) with PBST, followed by 75 min incubation with secondary antibody conjugated to peroxidase (rabbit IgG, Jackson ImmunoResearch, #111-035-003; mouse IgG, Jackson ImmunoResearch, #115-035-003; or goat IgG, Jackson ImmunoResearch, #305-035-003; all secondaries 1:5000 diluted in 0.1% PBST; room temperature). A new 4 × 5 min 0.1% PBST rinse protocol was performed and the membranes were incubated for 2 min with peroxidase substrate (Clarity Western ECL, BioRad, #1705061) for chemiluminescence detection (G:BOX, Syngene™). The density of the blots was analyzed in ImageJ software (National Institutes of Health, United States of America) and expressed in arbitrary units after normalization by the constitutive protein vinculin. Different targets were obtained from different membranes, except for the phosphorylated proteins, where after the incubation with the anti-phospho antibodies, the respective membrane was stripped, blocked, and incubated with the total antibody for each protein. For each membrane the control blot vinculin was performed, to assure the sample loading and provide accurate normalization (Supplementary File S1).
Statistical analysis
The normal distribution and homogeneity of the data were tested by Shapiro-Wilk test and Levene’s test, respectively, and the results were analyzed by two-way ANOVA, followed by the Tukey post hoc test. Data are expressed as mean ± standard error of the mean (SEM). Differences were considered significant at p < 0.05.
Results
Adrenalectomy attenuates changes in ponderal, and biochemical parameters induced by lactation overnutrition in male rats:
The body weight along the experimental protocol showed no interaction among days (PND 60–74), litter size (NL and SL) and groups (sham, ADX, and ADX + B) [F (28;924) = 0.888, p = 0.532], but with effects of days [F (14;53) = 63.395, p < 0.0001], litter size [F (1;66) = 89.42, p < 0.0001] and groups [F (2;66) = 3.641, p = 0.032]. This body weight response was integrated into the area under the curve (AUC) of body weight, which showed no interaction between the groups and litter size on the AUC of body weight [F (2;66) = 0.055, p = 0.946], with effects of litter size [F (1;66) = 89.6, p < 0.0001] and groups [F (2;66) = 3.83, p = 0.027]. SL animals demonstrated an increase in body weight compared with NL groups. ADX animals reduced body weight compared with the sham group and the treatment with corticosterone increased body weight, compared with the ADX group in both litters (Figures 2A,B).
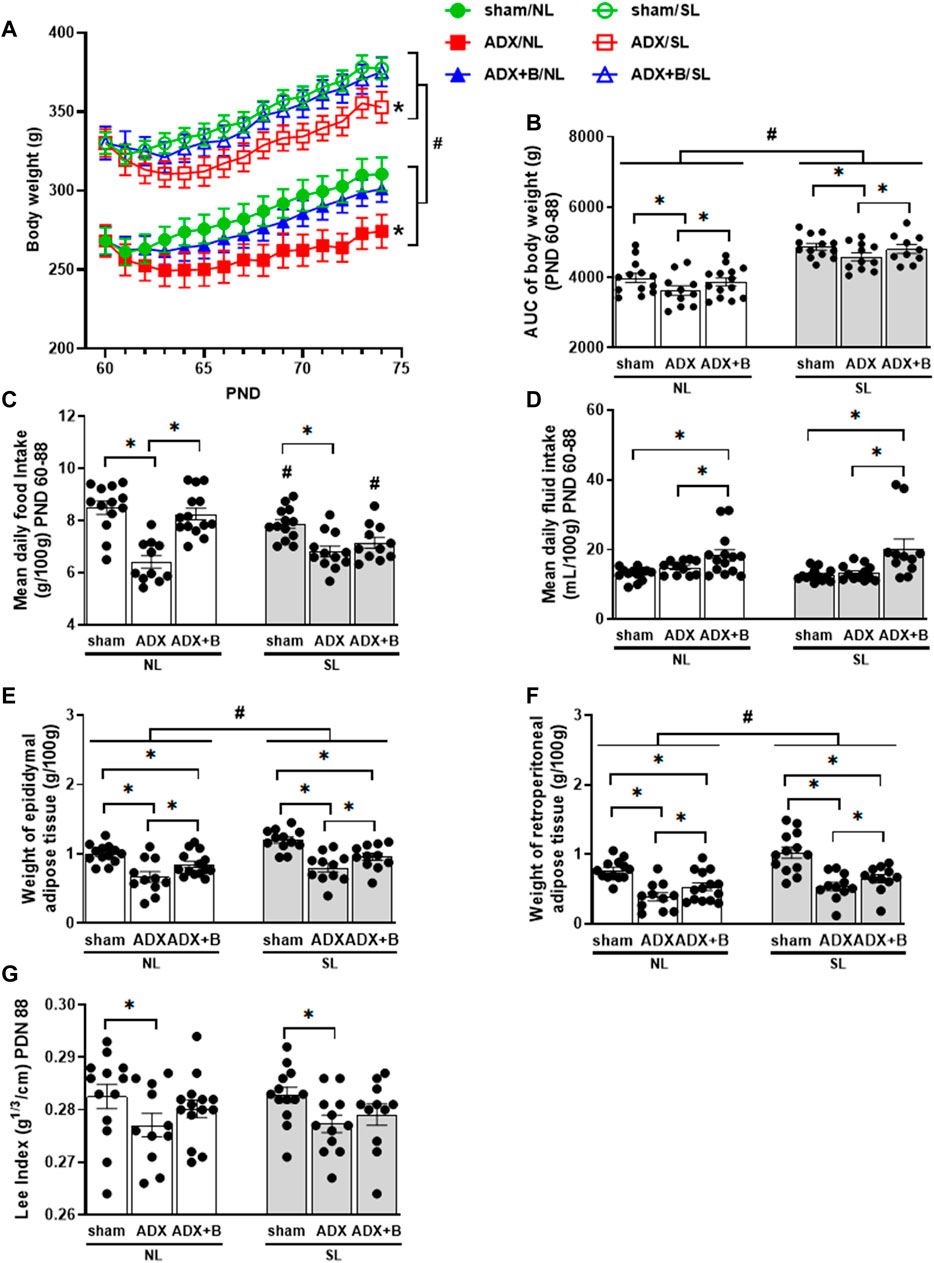
FIGURE 2. Body weight from PND 60 to 74 (A), AUC of body weight from PND 60 to 74 (B), mean daily food intake (C) and mean daily fluid intake (mL/100 g) from PND 60 to 74 (D), weights of epididymal (E) and retroperitoneal (F) adipose tissues on PND 74, and Lee Index (g ⅓/cm) on PND 74 (G) of male rats from normal (NL) and small (SL) litters: sham; ADX; ADX + B. Data were analyzed by two-way Anova, followed by the Tukey post hoc test and expressed as mean ± SEM. *p < 0.05; #p < 0.05 SL versus NL; B = corticosterone (n = 13).
An interaction was observed between groups and litter size on food intake [F (2;68) = 5.88, p = 0.004]. Food intake of SL animals of sham and ADX + B groups was lower than shown in NL groups. ADX groups in both litters showed a reduction in food intake, compared with sham animals, and treatment with corticosterone reversed ADX effects in NL animals (Figure 2C). No interaction was observed between groups and litter size on fluid intake [F (2;68) = 1.31, p = 0.28], without effects of litter size [F (1;68) = 2.05, p = 0.16] and with effects of groups [F (2;68) = 15.33, p < 0.001]. ADX + B groups in both litters showed increased fluid intake compared with ADX and sham animals (Figure 2D).
No interaction was observed between the groups and litter size on the weights of epididymal [F (2;66) = 0.394, p = 0.676] and retroperitoneal [F (2;67) = 0.668, p = 0.516] adipose tissues, with effects of litter size for epididymal [F (1;66) = 11.46, p < 0.001] and retroperitoneal depots [F (1;67) = 12.2, p < 0.001], as well as with effects of groups for epididymal [F (2;66) = 23.6, p < 0.0001] and retroperitoneal depots [F (2;67) = 27.3, p < 0.0001]. SL animals showed increased weights of both fat depots compared with NL groups. ADX animals reduced the weights of epididymal and retroperitoneal adipose tissues compared with the sham group and the treatment with corticosterone increased the weight of these fat depots, compared with the ADX group in both litters (Figures 2E,F). No interaction was observed between the groups and litter size on Lee index [F (2;68) = 0.09, p = 0.914], with no effects of litter size [F (1;68) = 0.015, p = 0.903] and with effects of groups [F (2;68) = 4.11, p = 0.021]. ADX groups in both litters demonstrated a decrease in the Lee index compared with their respective sham animals (Figure 2G).
There was an interaction between groups and litter size on FFA [F (2;57) = 5.59, p = 0.006] and TG [F (2;59) = 3.29, p = 0.044] plasma levels. In sham animals, SL induced an increase in FFA plasma levels, and ADX reduced this response, without the effects of corticosterone treatment. ADX reduced plasma levels of TG and corticosterone treatment reversed this response in both litters, but TG levels were lower in ADX + B animals of SL than ADX-SL and ADX + B-NL groups. (Figures 3A,B).
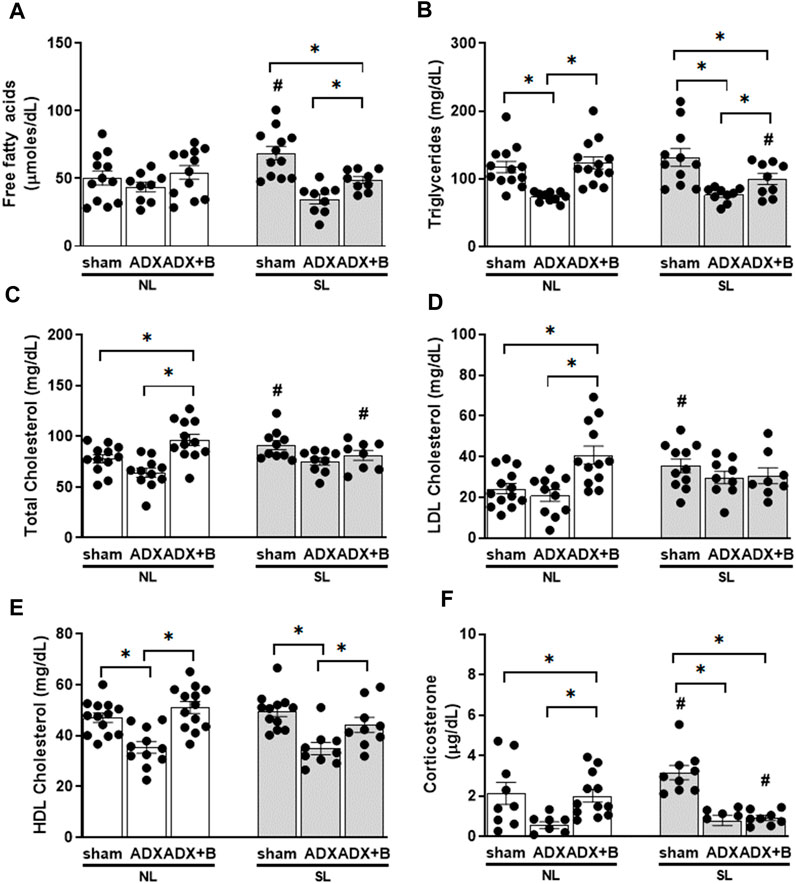
FIGURE 3. Plasma concentrations of free fatty acids (A), triglycerides (B), total cholesterol (C), HDL cholesterol (D), LDL cholesterol (E) and corticosterone (F) of male rats from normal (NL) and small (SL) litters on PND 74: sham; ADX; ADX + B, from PND 60 to 74. Data were analyzed by two-way Anova, followed by the Tukey post hoc test and expressed as mean ± SEM. *p < 0.05; #p < 0.05 SL versus NL; B = corticosterone (n = 13).
An interaction was observed between groups and litter size (NL and SL) on plasma levels of total [F (2;56) = 5.54, p = 0.006] and LDL cholesterol [F (2;58) = 5.52, p = 0.006], without interaction in HDL cholesterol [F (1;61) = 2.22, p = 0.117]. The reduction the litter size promoted higher plasma levels of total and LDL cholesterol in the sham group compared to NL-sham animals. In the NL group, the treatment with corticosterone in ADX group induced higher concentrations of total and LDL cholesterol than in sham and ADX group (Figures 3C,D). Plasma levels of HDL cholesterol showed effects of the group [F (2;61) = 19.72, p < 0.001], without effects of the litter size [F (1;61) = 0.7, p = 0.407]. ADX reduced (p < 0.001) HDL plasma levels, compared to the sham group, and corticosterone treatment reversed (p < 0.001) this effect in both litters (Figure 3E).
Interaction was observed between group and litter size on the plasma concentrations of corticosterone [F (2;47) = 5.16, p = 0.009)]. Reduction of the litter size induced higher (p < 0.05) plasma concentrations of corticosterone in sham animals compared to their respective NL animals. Adrenalectomy reduced (p < 0.05) plasma concentration of corticosterone in both litters and treatment with corticosterone enhanced the plasma concentration of corticosterone only in NL animals, without effects in SL animals (Figure 3F).
Effects of adrenalectomy and treatment with glucocorticoid on liver insulin signaling pathway of adult male rats with lactation overnutrition:
No interaction was observed between the groups and litter size on pIR/IR [F (2;50) = 1.08, p = 0.35], with no effects of litter size [F (1;50) = 2.56, p = 0.12] and groups [F (2; 50) = 0.18, p = 0.84] (Figure 4A). There was also no interaction between the groups and litter size on IRS1 [F (2;50) = 1.00, p = 0.37], with no effects of litter size [F (1;50) = 0.32, p = 0.57] and with effects of groups [F (2;50) = 4.67, p = 0.014] (Figure 4B). Treatment with corticosterone in ADX animals promoted higher expression of IRS1 in the liver of both litters. Interaction was not observed between the groups and litter size (NL and SL) on IRS2 [F (2;50) = 0.13, p = 0.88], with no effects of litter size [F (1;50) = 2.55, p = 0.12] and with effects of groups [F (2;50) = 12.59, p < 0.001]. ADX animals of both litters showed a decrease in expression of IRS2, compared to sham, and treatment with corticosterone reversed this response (Figure 4C).
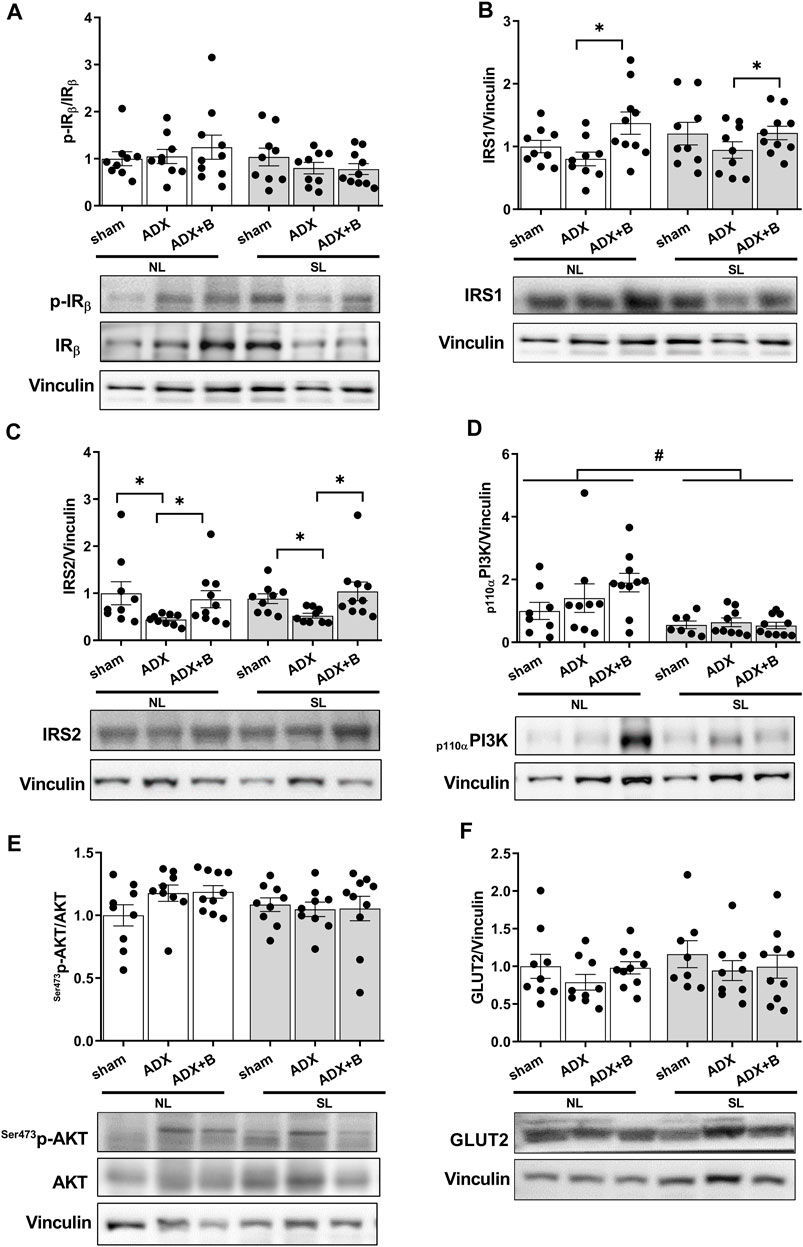
FIGURE 4. Liver expression of insulin receptor (IR) (A), insulin receptor substrate (IRS) 1(B), insulin receptor substrate (IRS) 2 (C), PI3Kp110 (D), Ser473pAKT/AKT (E) and GLUT2 (F) of male rats from normal (NL) and small (SL) litters on PND 74: sham; ADX; ADX + B. Data are expressed as mean ± SEM. *p < 0.05; #p < 0.05 SL versus NL; B = corticosterone (n = 13).
Interaction was not observed between the groups and litter size on Ser473pAKT/AKT [F (2; 50) = 1.41, p = 0.25], with no effects of litter size [F (1;50) = 1.71, p = 0.20] and groups [F (2; 50) = 1.21, p = 0.31] (Figure 4D). There was no interaction between the groups and litter size on p110αPI3K [F (2;47) = 1.72, p = 0.19], with effects of litter size [F (1;47) = 15.76, p < 0.001] and with no effects of groups [F (2;47) = 0.88, p = 0.42] (Figure 4E). The reduction of the litter size promoted a decrease in the expression of p110αPI3K compared with NL animals. No interaction was also between the groups and litter size on GLUT 2 [F (2;49) = 0.19, p = 0.83], without effects of litter size [F (1;49) = 0.99, p = 0.33] and groups [F (2;49) = 1.19, p = 0.31] (Figure 4F).
Effects of adrenalectomy and treatment with glucocorticoid on lipid metabolism in the liver of adult male rats with lactation overnutrition:
No interaction was observed between the groups and litter size on liver TG [F (2;52) = 0.27, p = 0.76], with effects of litter size [F (1;52) = 48.73, p < 0.001] and groups [F (2;52) = 12.73, p < 0.001]. SL groups presented higher (p < 0.05) liver TG content than NL animals. ADX promoted reduction (p < 0.05) in liver TG content and the treatment with corticosterone reversed this response in both litters (Figure 5A).
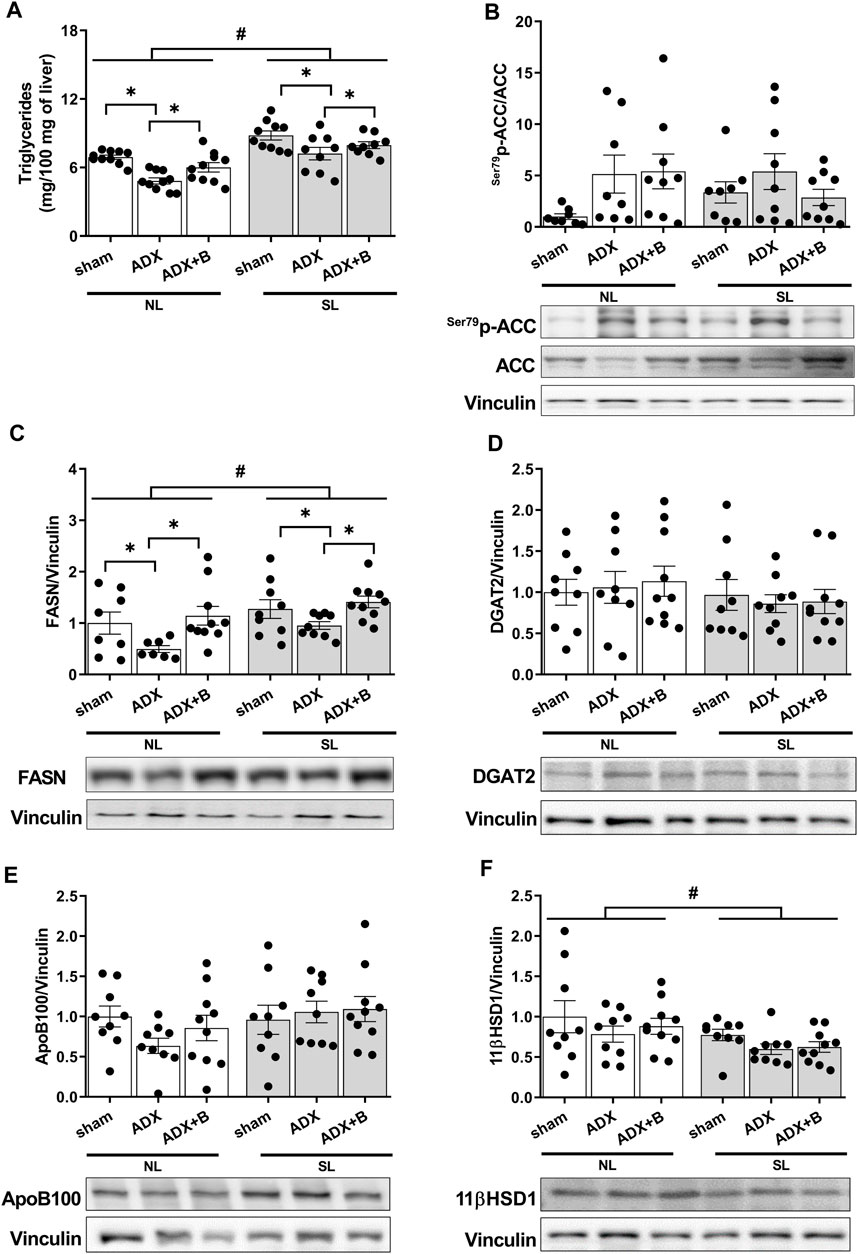
FIGURE 5. Liver triglycerides (mg/100 mg of liver) (A), liver expression of fatty acid synthase (FASN) (B), acetyl-CoA carboxylase (p-ACC/ACC) (C), diacylglycerol acyltransferase (DGAT) 2 (D), Apo B100 (E) and 11 β- Hydroxysteroid dehydrogenase 1 (11 βHSD1) (F) of male rats from normal (NL) and small (SL) litters on PND 74: sham; ADX; ADX + B. Data are expressed as mean ± SEM. *p < 0.05; #p < 0.05 SL versus NL; B = corticosterone (n = 13).
There was no interaction between the groups and litter size on p-ACC/ACC [F (2;45) = 1.60, p = 0.21], with no effects of litter size [F (1;45) = 0.0003, p = 0.99] and groups [F (2;45) = 2.49, p = 0.21] (Figure 5B). No interaction was observed between the groups and litter size (NL and SL) on FASN [F (2;47) = 0.24, p = 0.79], with effects of litter size [F (1;47) = 7.01, p = 0.011] and groups [F (2;47) = 6.82, p = 0.003] (Figure 5C). The reduction of the litter size promoted an increase of FASN expression, compared with NL animals, while the adrenalectomy reduced the FASN expression, and the treatment with corticosterone reversed this parameter in both litters.
Additionally, there was no interaction between the groups and litter size (NL and SL) on DGAT2 [F (2;50) = 0.06, p = 0.94], with no effects of litter size [F (1;50) = 1.92, p = 0.17] and groups [F (2;50) = 0.06, p = 0.94] (Figure 5D). Also, no interaction was observed between the groups and litter size (NL and SL) on APOB100 [F (2;50) = 1.22, p = 0.30], with no effects of litter size [F (1;50) = 2.96, p = 0.092] and groups [F (2;50) = 0.53, p = 0.59] (Figure 5E).
Finally, no interaction was observed between the groups and litter size (NL and SL) on 11β hydroxysteroid dehydrogenase 1 (11βHSD1) [F (2; 50) = 0.05, p = 0.95], with effects of litter size [F (1; 50) = 6.27, p = 0.02] and without effects of groups [F (2;50) = 1.64, p = 0.20] (Fig. 6 A). All SL animals showed decreased 11βHSD1 expression compared with NL groups (Figure 5F).
Discussion
The present study aimed to investigate the glucocorticoids’ contribution to metabolic and lipidic liver functions in adult male Wistar rats with lactation overnutrition. For this purpose, the effects of ADX and the treatment with corticosterone were analyzed as tools to reach these goals. Indeed, this study is the pioneer to show that ADX attenuated plasma and liver changes observed after lactation overnutrition, and corticosterone treatment reversed most of the ADX-induced effects, indicating that circulating glucocorticoids contribute to liver and plasma impairments induced by childhood obesity in adult male rats.
There are different models to achieve obesity in rodents, and one of them is to observe the consequences of early neonatal overfeeding using the litter size reduction method (Plagemann et al., 1999a; Plagemann et al., 1999b). In this model, there is a decrease in competition for milk during the breastfeeding period and thus, several authors also affirm that the composition of breast milk is modified, presenting a higher concentration of lipids, mainly an increase in TG (Cunha et al., 2009; Moreira et al., 2009; Šefčíková et al., 2011).
The litter size reduction method programs overweight in adulthood according to several data (Plagemann et al., 1992; Boullu-Ciocca et al., 2005; Rodrigues et al., 2009; Conceição et al., 2011; Ferretti et al., 2011; Rodrigues et al., 2011). The increased body weight, adiposity and the impairment of lipidic profile in SL animals corroborates with the literature, where animals reared in small litters present obesity-related changes that persist until adulthood, as higher body weight, accompanied by higher FFA (Bei et al., 2015) and total cholesterol, as well as fat depots (Hahn, 1984; Enes-Marques et al., 2020), without changes in plasma TG (Rodrigues et al., 2007; Conceição et al., 2013).
Interestingly, besides dyslipidemia, adult animals with childhood obesity also presented lipid accumulation in the liver, observed by increased liver TG content, accompanied by increased DNL, seen by enhanced expression of FASN in the liver, following previous studies (Duff and Snell, 1982; Ribas-Aulinas et al., 2021; Yang et a., 2018). Indeed, increased content of liver TG, as well as plasma levels of TG and FFA, might be ascribed to increased expression of FASN in the liver in SL animals, which could account for increased synthesis of fatty acids and consequently DNL.
In addition to higher body weight and impairment in lipidic profile, the SL animals also had a higher concentration of circulating glucocorticoids, as previously shown (Hou et al., 2011; de Souza et al., 2022). Animals with obesity in different experimental models have increased levels of circulating glucocorticoids (Freedman et al., 1986; Mantha et al., 1999; Tokuyama and Himms-Hagen, 1989), and removal of them by adrenalectomy attenuates or prevents the development of obesity (Romsos, 1991; Tokuyama and Himms-Hagen, 1989; Freedman et al., 1986), pointing glucocorticoids as a possible mediator of obesity in these experimental models. Accordingly, the role of glucocorticoids as an important modulator of energy balance in obesity induced by early overnutrition was also demonstrated in the current work, where ADX was able to reduce body weight gain and food intake, to induce an improvement in lipid profile, with reduction of FFA, TG, total and LDL cholesterol, and to reduce TG content as well as expression of FASN in the liver of SL animals, and treatment with corticosterone could restore most of these changes induced by ADX.
ADX-induced reduction of body weight and plasma lipid profile in childhood obesity is following the reversal effect of ADX on metabolic parameters in other obesity models (Alarrayed et al., 1992; Mantha et al., 1999; Kibenge and Chan, 2001; Yilmaz et al., 2002). Additionally, the decrease of triglyceride content and FASN expression in the liver by ADX may be due to the well-established stimulatory effects of glucocorticoids on both the accumulation of lipids and TG in the liver (Suzuki and Mizugashira, 1979) and the expression of key enzymes of DNL in the liver, as FASN (Wang et al., 2004; Lee et al., 2011). It is tempting to suggest that enhanced levels of circulating glucocorticoids in SL animals might have permissive effects on FASN expression, and consequently DNL, and TG accumulation in the liver, since ADX was able to attenuate these parameters in the present work, and glucocorticoids are known to induce FASN and DNL (Wang et al., 2004; Lee et al., 2011), and TG content in the liver (Suzuki and Mizugashira, 1979). Conversely, in vitro studies showed that both lipid accumulation and TG content in HepG2 cells was significantly increased by glucocorticoid treatment by an increase of DNL (Yang et al., 2018). Thus, the lack of effects of litter size reduction and adrenal removal on the other molecules involved in lipid metabolism in the liver is likely to indicate that FASN is a key molecule underlying glucocorticoids-induced upregulation of DNL and TG accumulation in the liver on adult male rats with childhood obesity.
Despite plasma insulin was not measured, it is known that animals reared in small litters present increased concentrations of circulating insulin (Hou et al., 2011; Conceição et al., 2013; Liu et al., 2013), and insulin acts in lipid metabolism, stimulating DNL mainly by upregulating the transcription factor sterol regulatory element binding protein (SREBP)-1c for expression of ACC and FASN (Horton et al., 2002; Csaki and Reue, 2010), important for fatty acid synthesis and lipid biosynthesis. In addition to this, insulin regulates glucose metabolism in the hepatocytes, stimulating glycolysis and synthesis of glycogen and inhibiting gluconeogenesis (Titchenell et al., 2017). For these outcomes, insulin binds to its receptor, insulin receptor (IR) in the membrane, a tyrosine kinase receptor which autophosphorylates itself in the internal surface of the cell upon insulin binding, and thereby activates its intrinsic tyrosine kinase activity, resulting in phosphorylation of substrates of insulin receptor (IRS) proteins on tyrosine residues, which can activate phosphatidylinositol- 3- kinase (PI3K). PI3K is a dimer composed of a catalytic (p110) and a regulatory (p85) subunit, and the target proteins of PI3K are Akt and PKC isoforms (Backer et al., 1992; Czech and Corvera, 1999; Lietzke et al., 2000). In this context, the reduction of the expression of PI3K in the liver of adult animals with neonatal overnutrition observed in the present work is according to impairment of hepatic PI3K pathway in neonatal overfed animals in adulthood shown in previous studies (Conceição et al., 2013; Huang et al., 2020), despite the other molecules of this pathway was unchanged in the current data, suggesting a partial disruption of the insulin signaling pathway in the liver of adult male rats with neonatal overnutrition.
GLUT2 in the liver is responsible for the influx of glucose in the postprandial period, and the efflux of glucose in the post-absorptive and fasting periods (Chadt and Al-Hasani, 2020). In addition to the insulin signaling pathway, the lack of changes in the expression of GLUT2 in the liver by either litter size reduction or adrenalectomy also points that increased DNL observed in SL animals is not related to insulin-induced glucose utilization in the liver, since PI3K-AKT pathway in this tissue was impaired in animals with childhood obesity. On the other hand, a decrease of IRS2 expression in the liver following ADX might underly reduction of FASN expression and TG content in this tissue, since IRS2 in the liver has been pointed as an important mediator of lipid synthesis and DNL in the liver (Eckstein et al., 2017).
The major function of the 11β-HSD1 is to convert inactive cortisone to active cortisol (corticosterone). This is in the endoplasmic reticulum and widely expressed in liver and adipose tissue, being responsible for amplifying local glucocorticoids action (Jamieson et al., 1995; Bujalska et al., 1997; Masuzaki et al., 2001). The majority of obesity animal models show that hepatic 11β- HSD1 expression and/or activity are unchanged (Morton et al., 2004) or reduced (Drake et al., 2005). Despite Yang et al. (2018) have reported that postnatal overfeeding-induced litter size reduction leads to increased mRNA expression of 11β-HSD1 in the liver of adult male rats, the present data indicate the opposite, hepatic protein expression of 11β-HSD1 being downregulated by neonatal overnutrition. Accordingly, it is likely that hepatic lipid accumulation during adulthood in animals with lactation overnutrition may be ascribed to increased circulating glucocorticoids, rather than glucocorticoids overexposure through 11β-HSD1 upregulation in the liver. This reduction in liver 11β-HSD1 may account for partial impairment of the insulin signaling pathway in the liver in the present study, once overexpression of 11β -HSD1 in the liver in mice results in insulin resistance (Masuzaki et al., 2001; Paterson et al., 2004).
In spite of recovery of body weight in the SL/ADX + B group without recovery of food intake was unexpected, it seems to be a specific profile of SL animals to some challenges, since Marangon et al. (2020) have reported that SL animals also showed changes on body weight gain without changes on food intake. Within this, context, the lack of corticosteronemia elevation in the SL/ADX + B group, in spite of the highest values of fluid intake, is likely because SL animals might have been adapted to increased corticosterone plasma levels, and that the concentration of corticosterone treatment of 25 mg/L, which was enough to restore corticosterone plasma levels to NL animals, could not be sufficient to restore corticosterone levels in SL animals. Accordingly, the treatment with corticosterone was, at least in part, effective in reversing or attenuating the effects of ADX even in SL animals, since some parameters have been partially or fully restored in ADX + B animals. However, it cannot be ruled out the possibility that other hormones of adrenal glands, as aldosterone, could also participate on these responses. In fact, replacement with aldosterone + cortiscosterone to ADX rats was more effective in enhancing food intake and body weight gain than only corticosterone replacement (Devenport et al., 1983; 1985). Furthermore, aldosterone replacement to ADX animals restored daily caloric intake, especially lipid intake, and body fat deposition (Devenport and Devenport, 1982; Tempel and Leibowitz, 1989). Altogether, it is important to point out that, besides corticosterone, aldosterone is another adrenal hormone important to be considered in the control of energy balance. Finally, it is important to highlight that a limitation of the current study was that female animals were not included in the analysis; thus, the present conclusion is only to male rats, since male and female animals may have different responses.
Conclusion
In summary, metabolic and liver dysfunctions in adult male rats induced by neonatal overnutrition were attenuated by ADX, and the treatment with corticosterone restored most of them. Altogether, these data show that, likely in other obesity models, in childhood obesity, glucocorticoids also have permissive effects to induce hyperlipidemia and TG accumulation in the liver, probably by specific upregulation of one of the key enzymes of DNL: FASN. In conclusion, the role of glucocorticoids in the metabolic impairments in obesity should be considered, and possibly be a target for interventions in obesity and metabolic syndrome.
Data availability statement
The raw data supporting the conclusion of this article will be made available by the authors, without undue reservation.
Ethics statement
The animal study was reviewed and approved by the Ethics Committee on Animal Use for experimentation.
Author contributions
CS: Conceptualization (equal), Data curation (equal), Formal analysis, (equal) Investigation (equal), Methodology (equal), Project administration (equal), Supervision (equal), Validation (equal), Visualization (equal), Writing - original draft (equal), Writing—review and editing (equal). LS: Investigation (equal). AM: Investigation (equal). AM: Investigation (equal). GL: Investigation (equal). FF: Investigation (equal), Methodology (lead), Writing—review and editing (lead). AK Investigation (equal), Methodology (lead), Writing—review and editing (lead). DZ: Funding acquisition (equal), Investigation (lead), Methodology (lead). CZ: Funding acquisition (equal), Investigation (equal), Methodology (equal). FL: Funding acquisition (equal), Investigation (lead), Methodology (lead). EU: Conceptualization (equal), Data curation (equal), Formal analysis (equal), Funding acquisition (equal), Methodology (equal), Project administration (equal), Resources (equal), Supervision (equal), Validation (equal), Visualization (equal), Writing—original draft (equal), Writing—review and editing (equal).
Funding
This work received financial support from the Conselho Nacional de Desenvolvimento Científico e Tecnológico (CNPq), Fundação Araucária de Apoio ao Desenvolvimento Científico e Tecnológico do Estado do Paraná (FA), and Coordenação de Aperfeiçoamento de Pessoal de Nível Superior (CAPES—Finance Code 001), Brazil.
Acknowledgments
The authors are grateful to Superintendência de Ciência, Tecnologia e Ensino Superior (SETI), Fundação Araucária (FA) and Universidade Estadual de Londrina (PROPPG) for providing financial support for the publication fee.
Conflict of interest
The authors declare that the research was conducted in the absence of any commercial or financial relationships that could be construed as a potential conflict of interest.
Publisher’s note
All claims expressed in this article are solely those of the authors and do not necessarily represent those of their affiliated organizations, or those of the publisher, the editors and the reviewers. Any product that may be evaluated in this article, or claim that may be made by its manufacturer, is not guaranteed or endorsed by the publisher.
Supplementary material
The Supplementary Material for this article can be found online at: https://www.frontiersin.org/articles/10.3389/fphys.2023.1161582/full#supplementary-material
References
Alarrayed, F., Hartman, A. D., and Porter, J. R. (1992). Is there a role for the adrenals in the development of hypercholesterolemia in Zucker fatty rats? Am. J. physiology 263 (2), E287–E295. doi:10.1152/ajpendo.1992.263.2.E287
Backer, J. M., Myers, M. G., Shoelson, S. E., Chin, D. J., Sun, X. J., Miralpeix, M., et al. (1992). Phosphatidylinositol 3'-kinase is activated by association with IRS-1 during insulin stimulation. EMBO J. 11 (9), 3469–3479. doi:10.1002/j.1460-2075.1992.tb05426.x
Barker, D. J. P. (1998). In utero programming of chronic disease. Clin. Sci. 95 (2), 115–128. doi:10.1042/cs0950115
Bei, F., Jia, J., Jia, Y. Q., Sun, J. H., Liang, F., Yu, Z. Y., et al. (2015). Long-term effect of early postnatal overnutrition on insulin resistance and serum fatty acid profiles in male rats. Lipids health Dis. 14, 96. doi:10.1186/s12944-015-0094-2
Bernardis, L. L., and Patterson, B. D. (1968). Correlation between "Lee Index" and carcass fat content in weanling and adult female rats with hypothalamic lesions. J. Endocrinol. 40, 527–528. doi:10.1677/joe.0.0400527
Boullu-Ciocca, S., Dutour, A., Guillaume, V., Achard, V., Oliver, C., and Grino, M. (2005). Postnatal diet-induced obesity in rats upregulates systemic and adipose tissue glucocorticoid metabolism during development and in adulthood: Its relationship with the metabolic syndrome. Diabetes 54 (1), 197–203. doi:10.2337/diabetes.54.1.197
Bradbury, M. W. (2006). Lipid metabolism and liver inflammation. I. Hepatic fatty acid uptake: Possible role in steatosis. Am. J. physiology. Gastrointest. liver physiology 290 (2), G194–G198. doi:10.1152/ajpgi.00413.2005
Bradford, M. M. (1976). A rapid and sensitive method for the quantitation of microgram quantities of protein utilizing the principle of protein-dye binding. Anal. Biochem. 72, 248–254. doi:10.1006/abio.1976.9999
Bruce, B. K., King, B. M., Phelps, G. R., and Veitia, M. C. (1982). Effects of adrenalectomy and corticosterone administration on hypothalamic obesity in rats. Am. J. Physiology-Endocrinology Metabolism 243 (2), E152–E157. doi:10.1152/ajpendo.1982.243.2.E152
Bujalska, I. J., Kumar, S., and Stewart, P. M. (1997). Does central obesity reflect "Cushing's disease of the omentum"?.Lancet (London, Engl., 349(9060), 1210–1213. doi:10.1016/S0140-6736(96)11222-8
Chadt, A., and Al-Hasani, H. (2020). Glucose transporters in adipose tissue, liver, and skeletal muscle in metabolic health and disease. Pflugers Arch. 472, 1273–1298. doi:10.1007/s00424-020-02417-x
Cohen, J. C., Horton, J. D., and Hobbs, H. H. Human fatty liver disease: Old questions and new insights. Science. 2011;332:1519–1523. doi:10.1126/science.1204265
Conceição, E. P., Franco, J. G., Oliveira, E., Resende, A. C., Amaral, T. A., Peixoto-Silva, N., et al. (2013). Oxidative stress programming in a rat model of postnatal early overnutrition--role of insulin resistance. J. Nutr. Biochem. 24 (1), 81–87. doi:10.1016/j.jnutbio.2012.02.010
Conceição, E. P., Trevenzoli, I. H., Oliveira, E., Franco, J. G., Carlos, A. S., Nascimento-Saba, C. C., et al. (2011). Higher white adipocyte area and lower leptin production in adult rats overfed during lactation. Hormone metabolic Res. 43 (7), 513–516. doi:10.1055/s-0031-1275702
Csaki, L. S., and Reue, K. (2010). Lipins: Multifunctional lipid metabolism proteins. Annu. Rev. Nutr. 30, 257–272. doi:10.1146/annurev.nutr.012809.104729
Cunha, A. C., Pereira, R. O., Pereira, M. J., Soares, V. deM., Martins, M. R., Teixeira, M. T., et al. (2009). Long-term effects of overfeeding during lactation on insulin secretion--the role of GLUT-2. J. Nutr. Biochem. 20 (6), 435–442. doi:10.1016/j.jnutbio.2008.05.002
Czech, M. P., and Corvera, S. (1999). Signaling mechanisms that regulate glucose transport. J. Biol. Chem. 274 (4), 1865–1868. doi:10.1074/jbc.274.4.1865
de Fatima Silva, F., Komino, A. C. M., Andreotti, S., Boltes Reis, G., Caminhotto, R. O., Landgraf, R. G., et al. (2022). Dexamethasone-induced adipose tissue redistribution and metabolic changes: Is gene expression the main factor? An animal model of chronic hypercortisolism. Biomedicines 10 (9), 2328. doi:10.3390/biomedicines10092328
de Souza, C. F., Stopa, L. R. S., Martins, A. B., Wunderlich, A. L. M., Lopes, G. M., Zaia, D. A. M., et al. (2022). Lactation overnutrition-induced obesity impairs effects of exogenous corticosterone on energy homeostasis and hypothalamic-pituitary-adrenal axis in male rats. Life Sci. 304, 120721. doi:10.1016/j.lfs.2022.120721
de Souza, C. F., Stopa, L. R. S., Santos, G. F., Takasumi, L. C. N., Martins, A. B., Garnica-Siqueira, M. C., et al. (2019). Estradiol protects against ovariectomy-induced susceptibility to the anabolic effects of glucocorticoids in rats. Life Sci. 218, 185–196. doi:10.1016/j.lfs.2018.12.037
Devenport, L. D., and Devenport, J. A. (1982). The effects of adrenal hormones on brain and body size. Physiol. Psychol. 10, 399–404. doi:10.3758/bf03332971
Devenport, L. D., Goodwin, K. G., and Hopkins, P. M. (1985). Continuous infusion of aldosterone: Correlates of body weight gain. Pharmacol. Biochem. Behav. 22, 707–709. doi:10.1016/0091-3057(85)90517-9
Devenport, L. D., Torres, A., and Murray, C. G. (1983). Effects of aldosterone and deoxycorticosterone on food intake and body weight. Behav. Neurosci. 97, 667–669. doi:10.1037//0735-7044.97.4.667
Doege, H., and Stahl, A. (2006). Protein-mediated fatty acid uptake: Novel insights from in vivo models. Physiol. (Bethesda, Md 21, 259–268. doi:10.1152/physiol.00014.2006
Donnelly, K. L., Smith, C. I., Schwarzenberg, S. J., Jessurun, J., Boldt, M. D., and Parks, E. J. (2005). Sources of fatty acids stored in liver and secreted via lipoproteins in patients with nonalcoholic fatty liver disease. J. Clin. investigation 115 (5), 1343–1351. doi:10.1172/JCI23621
Drake, A. J., Livingstone, D. E., Andrew, R., Seckl, J. R., Morton, N. M., and Walker, B. R. (2005). Reduced adipose glucocorticoid reactivation and increased hepatic glucocorticoid clearance as an early adaptation to high-fat feeding in Wistar rats. Endocrinology 146 (2), 913–919. doi:10.1210/en.2004-1063
Dubuc, P. U., and Wilden, N. J. (1986). Adrenalectomy reduces but does not reverse obesity in ob/ob mice. Int. J. Obes. 10 (2), 91–98. Available from: http://www.ncbi.nlm.nih.gov/pubmed/3522453.
Duff, D. A., and Snell, K. (1982). Effect of altered neonatal nutrition on the development of enzymes of lipid and carbohydrate metabolism in the rat. J. Nutr. 112 (6), 1057–1066. doi:10.1093/jn/112.6.1057
Eckstein, S. S., Weigert, C., and Lehmann, R. (2017). Divergent roles of IRS (insulin receptor substrate) 1 and 2 in liver and skeletal muscle. Curr. Med. Chem. 24 (17), 1827–1852. doi:10.2174/0929867324666170426142826
Ellsworth, L., Harman, E., Padmanabhan, V., and Gregg, B. (2018). Lactational programming of glucose homeostasis: A window of opportunity. Reprod. Camb. Engl. 156 (2), R23–R42. doi:10.1530/REP-17-0780
Enes-Marques, S., Rojas, V. C. T., Batista, T. H., Vitor-Vieira, F., Novais, C. O., Vilela, F. C., et al. (2020). Neonatal overnutrition programming impairs cholecystokinin effects in adultmale rats. J. Nutr. Biochem. 86, 108494. doi:10.1016/j.jnutbio.2020.108494
Falholt, K., Lund, B., and Falholt, W. (1973). An easy colorimetric micromethod for routine determination of free fatty acids in plasma. Clin. chimica acta; Int. J. Clin. Chem. 46 (2), 105–111. doi:10.1016/0009-8981(73)90016-8
Ferretti, S., Fornari, A., Pedrazzi, P., Pellegrini, M., and Zoli, M. (2011). Developmental overfeeding alters hypothalamic neuropeptide mRNA levels and response to a high-fat diet in adult mice. Peptides 32 (7), 1371–1383. doi:10.1016/j.peptides.2011.06.001
Fiorotto, M. L., Burrin, D. G., Perez, M., and Reeds, P. J. (1991). Intake and use of milk nutrients by rat pups suckled in small, medium, or large litters. Am. J. Physiology-Regulatory, Integr. Comp. Physiology. 260 (6), R1104–R1113. doi:10.1152/ajpregu.1991.260.6.R1104
Folch, J., Lees, M., and Sloane Stanley, G. H. (1957). A simple method for the isolation and purification of total lipides from animal tissues. J. Biol. Chem. 226. 497–509. doi:10.1016/s0021-9258(18)64849-5
Freedman, M. R., Horwitz, B. A., and Stern, J. S. (1986). Effect of adrenalectomy and glucocorticoid replacement on development of obesity. Am. J. Physiology-Regulatory, Integr. Comp. Physiology 250 (4), R595–R607. doi:10.1152/ajpregu.1986.250.4.R595
Guarino, M. P., Santos, A. I., Mota-Carmo, M., and Costa, P. F. (2013). Effects of anaesthesia on insulin sensitivity and metabolic parameters in Wistar rats. vivo (Athens, Greece) 27 (1), 127–132.
Guillemin, R., Clayton, G. W., Smith, J. D., and Lipscomb, H. S. (1958). Measurement of free corticosteroids in rat plasma: Physiological validation of a method. Endocrinology 63 (3), 349–358. doi:10.1210/endo-63-3-349
Hahn, P. (1984). Effect of litter size on plasma cholesterol and insulin and some liver and adipose tissue enzymes in adult rodents. J. Nutr. 114 (7), 1231–1234. doi:10.1093/jn/114.7.1231
Heeren, J., and Scheja, L. (2021). Metabolic-associated fatty liver disease and lipoprotein metabolism. Mol. Metab. 50, 101238. doi:10.1016/j.molmet.2021.101238
Horton, J. D., Goldstein, J. L., and Brown, M. S. (2002). SREBPs: Activators of the complete program of cholesterol and fatty acid synthesis in the liver. J. Clin. Invest. 109:1125–1131. doi:10.1172/JCI15593
Hou, M., Liu, Y., Zhu, L., Sun, B., Guo, M., Burén, J., et al. (2011). Neonatal overfeeding induced by small litter rearing causes altered glucocorticoid metabolism in rats. PLoS ONE 6 (11), e25726–e25729. doi:10.1371/journal.pone.0025726
Huang, F., Zhu, P., Wang, J., Chen, J., and Lin, W. (2020). Postnatal overfeeding induces hepatic microRNA-221 expression and impairs the PI3K/AKT pathway in adult male rats. Pediatr. Res. V. 89, 143–149. doi:10.1038/s41390-020-0877-7
Jamieson, P. M., Chapman, K. E., Edwards, C. R., and Seckl, J. R. (1995). 11 beta-hydroxysteroid dehydrogenase is an exclusive 11 beta-reductase in primary cultures of rat hepatocytes: Effect of physicochemical and hormonal manipulations. Endocrinology 136 (11), 4754–4761. doi:10.1210/endo.136.11.7588203
Kawano, Y., and Cohen, D. E. (2013). Mechanisms of hepatic triglyceride accumulation in non-alcoholic fatty liver disease. J. gastroenterology 48 (4), 434–441. doi:10.1007/s00535-013-0758-5
Kennedy, G. C. (1957). The development with age of hypothalamic restraint upon the appetite of the rat. J. Endocrinol. 16 (1), 9–17. doi:10.1677/joe.0.0160009
Kibenge, M. T., and Chan, C. B. (2001). Interactions between effects of adrenalectomy and diet on insulin secretion in fa/fa Zucker rats. Can. J. physiology Pharmacol. 79 (1), 1–7. doi:10.1139/y00-106
Klausner, H., and Heimberg, M. (1967). Effect of adrenalcortical hormones on release of triglycerides and glucose by liver. Am. J. physiology 212 (6), 1236–1246. doi:10.1152/ajplegacy.1967.212.6.1236
Lee, M. J., Gong, D. W., Burkey, B. F., and Fried, S. K. (2011). Pathways regulated by glucocorticoids in omental and subcutaneous human adipose tissues: A microarray study. Am. J. physiology. Endocrinol. metabolism 300 (3), E571–E580. doi:10.1152/ajpendo.00231.2010
Lietzke, S. E., Bose, S., Cronin, T., Klarlund, J., Chawla, A., Czech, M. P., et al. (2000). Structural basis of 3-phosphoinositide recognition by pleckstrin homology domains. Mol. Cell 6 (2), 385–394. doi:10.1016/s1097-2765(00)00038-1
Liu, H. W., Srinivasan, M., Mahmood, S., Smiraglia, D. J., and Patel, M. S. (2013). Adult-onset obesity induced by early life overnutrition could be reversed by moderate caloric restriction. Am. J. physiology Endocrinol. metabolism 305 (7), E785–E794. doi:10.1152/ajpendo.00280.2013
Mantha, L., Palacios, E., and Deshaies, Y. (1999). Modulation of triglyceride metabolism by glucocorticoids in diet-induced obesity. Am. J. physiology 277 (2), R455–R464. doi:10.1152/ajpregu.1999.277.2.R455
Marangon, P. B., Mecawi, A. S., Antunes-Rodrigues, J., and Elias, L. L. K. (2020). Perinatal over- and underfeeding affect hypothalamic leptin and ghrelin neuroendocrine response in adult rats. Physiol. Behav. 215, 112793. doi:10.1016/j.physbeh.2019.112793
Masuzaki, H., Paterson, J., Shinyama, H., Morton, N. M., Mullins, J. J., Seckl, J. R., et al. (2001). A transgenic model of visceral obesity and the metabolic syndrome. Sci. (New York, N.Y.) 294 (5549), 2166–2170. doi:10.1126/science.1066285
Moreira, A. S., Teixeira Teixeira, M., da Silveira Osso, F., Pereira, R. O., de Oliveira Silva-Junior, G., Garcia de Souza, E. P., et al. (2009). Left ventricular hypertrophy induced by overnutrition early in life. Nutr. metabolism, Cardiovasc. Dis. NMCD 19 (11), 805–810. doi:10.1016/j.numecd.2009.01.008
Morton, N. M., Ramage, L., and Seckl, J. R. (2004). Down-regulation of adipose 11beta-hydroxysteroid dehydrogenase type 1 by high-fat feeding in mice: A potential adaptive mechanism counteracting metabolic disease. Endocrinology 145 (6), 2707–2712. doi:10.1210/en.2003-1674
Nestel, P. J., Havel, R. J., and Bezman, A. (1962). Sites of initial removal of chylomicron triglyceride fatty acids from the blood. J. Clin. investigation 41 (10), 1915–1921. doi:10.1172/JCI104648
Oben, J. A., Mouralidarane, A., Samuelsson, A. M., Matthews, P. J., Morgan, M. L., McKee, C., et al. (2010). Maternal obesity during pregnancy and lactation programs the development of offspring non-alcoholic fatty liver disease in mice. J. hepatology 52 (6), 913–920. doi:10.1016/j.jhep.2009.12.042
Paterson, J. M., Morton, N. M., Fievet, C., Kenyon, C. J., Holmes, M. C., Staels, B., et al. (2004). Metabolic syndrome without obesity: Hepatic overexpression of 11beta-hydroxysteroid dehydrogenase type 1 in transgenic mice. Proc. Natl. Acad. Sci. U. S. A. 101 (18), 7088–7093. doi:10.1073/pnas.0305524101
Plagemann, A., Harder, T., Rake, A., Melchior, K., Rohde, W., and Dörner, G. (1999a). Increased number of galanin-neurons in the paraventricular hypothalamic nucleus of neonatally overfed weanling rats. Brain Res. 818 (1), 160–163. doi:10.1016/s0006-8993(98)01264-5
Plagemann, A., Harder, T., Rake, A., Voits, M., Fink, H., Rohde, W., et al. (1999b). Perinatal elevation of hypothalamic insulin, acquired malformation of hypothalamic galaninergic neurons, and syndrome x-like alterations in adulthood of neonatally overfed rats. Brain Res. 836 (1-2), 146–155. doi:10.1016/s0006-8993(99)01662-5
Plagemann, A., Heidrich, I., Götz, F., Rohde, W., and Dörner, G. (1992). Obesity and enhanced diabetes and cardiovascular risk in adult rats due to early postnatal overfeeding. Exp. Clin. Endocrinol. 99 (3), 154–158. doi:10.1055/s-0029-1211159
Plagemann, A. (2006). Perinatal nutrition and hormone-dependent programming of food intake. Hormone Res. 65 (Suppl. 3), 83–89. doi:10.1159/000091511
Rebuffé-Scrive, M., Krotkiewski, M., Elfverson, J., and Björntorp, P. (1988). Muscle and adipose tissue morphology and metabolism in Cushing's syndrome. J. Clin. Endocrinol. metabolism 67 (6), 1122–1128. doi:10.1210/jcem-67-6-1122
Ribas-Aulinas, F., Parra-Vargas, M., Ramon-Krauel, M., Diaz, R., Lerin, C., Cambras, T., et al. (2021). Time-restricted feeding during puberty ameliorates adiposity and prevents hepatic steatosis in a mouse model of childhood obesity. Nutrients 13 (10), 3579. doi:10.3390/nu13103579
Rodrigues, A. L., de Moura, E. G., Passos, M. C., Dutra, S. C., and Lisboa, P. C. (2009). Postnatal early overnutrition changes the leptin signalling pathway in the hypothalamic-pituitary-thyroid axis of young and adult rats. J. physiology 587 (11), 2647–2661. doi:10.1113/jphysiol.2009.169045
Rodrigues, A. L., de Moura, E. G., Passos, M. C., Trevenzoli, I. H., da Conceição, E. P., Bonono, I. T., et al. (2011). Postnatal early overfeeding induces hypothalamic higher SOCS3 expression and lower STAT3 activity in adult rats. J. Nutr. Biochem. 22 (2), 109–117. doi:10.1016/j.jnutbio.2009.11.013
Rodrigues, A. L., De Souza, E. P., Da Silva, S. V., Rodrigues, D. S., Nascimento, A. B., Barja-Fidalgo, C., et al. (2007). Low expression of insulin signaling molecules impairs glucose uptake in adipocytes after early overnutrition. J. Endocrinol. 195 (3), 485–494. doi:10.1677/JOE-07-0046
Romsos, D. R. (1991). “Energy balance: Role of adrenal gland secretions,” in Proceedings of the 14th international congress of nutrition. Editors YoungKW, L. Y. Cha, L. K. Yull, J. J. Soon, and K. S. He (Seoul, South Korea: International Congress of Nutrition), 129–132.
Rutledge, A. C., Su, Q., and Adeli, K. (2010). Apolipoprotein B100 biogenesis: A complex array of intracellular mechanisms regulating folding, stability, and lipoprotein assembly. Biochem. Cell Biol. = Biochimie Biol. Cell. 88 (2), 251–267. doi:10.1139/o09-168
Šefčíková, Z., Bujňáková, D., Raček, Ľ., Kmet, V., and Mozeš, Š. (2011). Developmental changes in gut microbiota and enzyme activity predict obesity risk in rats arising from reduced nests. Physiological Res. 60 (2), 337–346. doi:10.33549/physiolres.931939
Spencer, S. J. (2013). Perinatal programming of neuroendocrine mechanisms connecting feeding behavior and stress. Front. Neurosci. 7, 109. doi:10.3389/fnins.2013.00109
Spencer, S. J., and Tilbrook, A. (2009). Neonatal overfeeding alters adult anxiety and stress responsiveness. Psychoneuroendocrinology 34 (8), 1133–1143. doi:10.1016/j.psyneuen.2009.02.013
Stopa, L. R. S., de Souza, C. F., Martins, A. B., Lopes, G. M., Costa, N. O., Gerardin, D. C. C., et al. (2021). Neonatal overfeeding reduces estradiol plasma levels and disrupts noradrenergic-kisspeptin-GnRH pathway and fertility in adult female rats. Mol. Cell. Endocrinol. 524 (2020), 111147. doi:10.1016/j.mce.2020.111147
Stopa, L. R. S., de Souza, C. F., Santos, G. F., Martins, A. B., Ferreira, R. N., de Andrade, F. G., et al. (2019). Sex differences in glucocorticoids-induced anabolic effects in rats. Physiology Behav. 209, 112587. doi:10.1016/j.physbeh.2019.112587
Suzuki, H., and Mizugashira, M. (1979). Effect of adrenalectomy on liver lipid content of fasted rats. J. Nutr. 109 (8), 1413–1418. doi:10.1093/jn/109.8.1413
Tataranni, P. A., Larson, D. E., Snitker, S., Young, J. B., Flatt, J. P., and Ravussin, E. (1996). Effects of glucocorticoids on energy metabolism and food intake in humans. Am. J. Physiol. Endocrinol. Metab. 271, E317–E325. doi:10.1152/ajpendo.1996.271.2.E317
Tempel, D. L., and Leibowitz, S. F. (1989). PVN steroid implants: Effect on feeding patterns and macronutrient selection. Brain Res. Bull. 23, 553–560. doi:10.1016/0361-9230(89)90200-1
Titchenell, P. M., Lazar, M. A., and Birnbaum, M. J. (2017). Unraveling the regulation of hepatic metabolism by insulin. Trends Endocrinol. metabolism TEM 28 (7), 497–505. doi:10.1016/j.tem.2017.03.003
Tokuyama, K., and Himms-Hagen, J. (1989). Enhanced acute response to corticosterone in genetically obese (ob/ob) mice. Am. J. Physiol. Endocrinol. Metab. 257 (2), E133–E138. doi:10.1152/ajpendo.1989.257.2.E133
Uchoa, E. T., Sabino, H. A., Ruginsk, S. G., Antunes-Rodrigues, J., and Elias, L. L. (2009a). Hypophagia induced by glucocorticoid deficiency is associated with an increased activation of satiety-related responses. J. Appl. Physiol. 106, 596–604. doi:10.1152/japplphysiol.90865.2008
Uchoa, E. T., Silva, L. E. C. M., de Castro, M., Antunes-Rodrigues, J., and Elias, L. L. K. (2010). Corticotrophin-releasing factor mediates hypophagia after adrenalectomy, increasing meal-related satiety responses. Horm. Behav. 58, 714–719. doi:10.1016/j.yhbeh.2010.07.003
Uchoa, E. T., Silva, L. E. C. M., de Castro, M., Antunes-Rodrigues, J., and Elias, L. L. K. (2012). Glucocorticoids are required for meal-induced changes in the expression of hypothalamic neuropeptides. Neuropeptides 46, 119–124. doi:10.1016/j.npep.2012.02.002
Uchoa, E. T., Silva, L. E. C. M., de Castro, M., Antunes-Rodrigues, J., and Elias, L. L. K. (2009b). Hypothalamic oxytocin neurons modulate hypophagic effect induced by adrenalectomy. Horm. Behav. 56, 532–538. doi:10.1016/j.yhbeh.2009.09.007
Wang, Y., Jones Voy, B., Urs, S., Kim, S., Soltani-Bejnood, M., Quigley, N., et al. (2004). The human fatty acid synthase gene and de novo lipogenesis are coordinately regulated in human adipose tissue. J. Nutr. 134 (5), 1032–1038. doi:10.1093/jn/134.5.1032
Windeløv, J. A., Pedersen, J., and Holst, J. J. (2016). Use of anesthesia dramatically alters the oral glucose tolerance and insulin secretion in C57Bl/6 mice. Physiol. Rep. 4 (11), e12824. doi:10.14814/phy2.12824
Wurie, H. R., Buckett, L., and Zammit, V. A. (2012). Diacylglycerol acyltransferase 2 acts upstream of diacylglycerol acyltransferase 1 and utilizes nascent diglycerides and de novo synthesized fatty acids in HepG2 cells. FEBS J. 279 (17), 3033–3047. doi:10.1111/j.1742-4658.2012.08684.x
Yang, F., Dai, Y., Min, C., and Li, X. (2018). Neonatal overfeeding induced glucocorticoid overexposure accelerates hepatic lipogenesis in male rats. Nutr. Metabolism 15, 30. doi:10.1186/s12986-018-0272-0
Yilmaz, A., Suleyman, H., Umudum, Z., and Sahin, Y. N. (2002). The effect of adrenalectomy on leptin levels and some metabolic parameters in rats with diet-induced obesity. Biol. Pharm. Bull. 25 (5), 580–583. doi:10.1248/bpb.25.580
Yukimura, Y., Bray, G., and Wolfse, A. (1978). Some effects of adrenalectomy in the fatty rat. Endocrinology 103 (5). 1924–1928. doi:10.1210/endo-103-5-1924
Keywords: adrenalectomy, corticosterone, litter size reduction, triglycerides, obesity
Citation: de Souza CF, Stopa LRS, Martins AB, Wunderlich ALM, Lopes GM, de Fatima Silva F, Komino ACM, Zaia DAM, Zaia CTBV, Lima FB and Uchoa ET (2023) Glucocorticoids contribute to metabolic and liver impairments induced by lactation overnutrition in male adult rats. Front. Physiol. 14:1161582. doi: 10.3389/fphys.2023.1161582
Received: 08 February 2023; Accepted: 20 April 2023;
Published: 10 May 2023.
Edited by:
Antonio Marcus de Andrade Paes, Federal University of Maranhão, BrazilReviewed by:
Alex Rafacho, Federal University of Santa Catarina, BrazilRodrigo Mello Gomes, Universidade Federal de Goiás, Brazil
Copyright © 2023 de Souza, Stopa, Martins, Wunderlich, Lopes, de Fatima Silva, Komino, Zaia, Zaia, Lima and Uchoa. This is an open-access article distributed under the terms of the Creative Commons Attribution License (CC BY). The use, distribution or reproduction in other forums is permitted, provided the original author(s) and the copyright owner(s) are credited and that the original publication in this journal is cited, in accordance with accepted academic practice. No use, distribution or reproduction is permitted which does not comply with these terms.
*Correspondence: Ernane Torres Uchoa, ZXVjaG9hQHVlbC5icg==