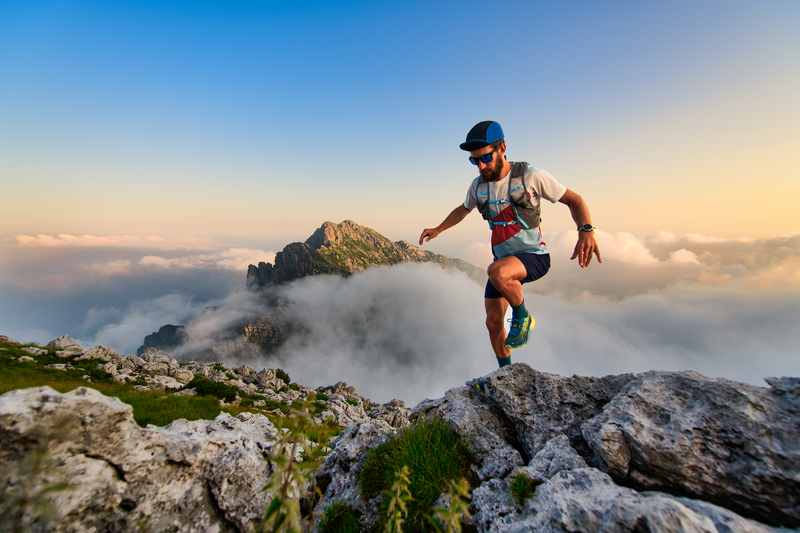
95% of researchers rate our articles as excellent or good
Learn more about the work of our research integrity team to safeguard the quality of each article we publish.
Find out more
ORIGINAL RESEARCH article
Front. Physiol. , 21 April 2023
Sec. Skeletal Physiology
Volume 14 - 2023 | https://doi.org/10.3389/fphys.2023.1160261
This article is part of the Research Topic Biomechanical Performance and Relevant Mechanism of Physical Medicine and Rehabilitation for Neuromusculoskeletal Disorders View all 32 articles
Objective: Anterior cruciate ligament reconstruction (ACLR) cannot decrease the risk of knee osteoarthritis after anterior cruciate ligament rupture, and tibial contact force is associated with the development of knee osteoarthritis. The purpose of this study was to compare the difference in bilateral tibial contact force for patients with unilateral ACLR during walking and jogging based on an EMG-assisted method in order to evaluate the risk of knee osteoarthritis following unilateral ACLR.
Methods: Seven unilateral ACLR patients participated in experiments. The 14-camera motion capture system, 3-Dimension force plate, and wireless EMG test system were used to collect the participants’ kinematics, kinetics, and EMG data during walking and jogging. A personalized neuromusculoskeletal model was established by combining scaling and calibration optimization. The inverse kinematics and inverse dynamics algorithms were used to calculate the joint angle and joint net moment. The EMG-assisted model was used to calculate the muscle force. On this basis, the contact force of the knee joint was analyzed, and the tibial contact force was obtained. The paired sample t-test was used to analyze the difference between the participants’ healthy and surgical sides of the participants.
Results: During jogging, the peak tibial compression force on the healthy side was higher than on the surgical side (p = 0.039). At the peak moment of tibial compression force, the muscle force of the rectus femoris (p = 0.035) and vastus medialis (p = 0.036) on the healthy side was significantly higher than that on the surgical side; the knee flexion (p = 0.042) and ankle dorsiflexion (p = 0.046) angle on the healthy side was higher than that on the surgical side. There was no significant difference in the first (p = 0.122) and second (p = 0.445) peak tibial compression forces during walking between the healthy and surgical sides.
Conclusion: Patients with unilateral ACLR showed smaller tibial compression force on the surgical side than on the healthy side during jogging. The main reason for this may be the insufficient exertion of the rectus femoris and vastus medialis.
Anterior cruciate ligament (ACL) injury is one of the most common sports-related injuries (Agel et al., 2005; Yu and Garrett, 2007), and anterior cruciate ligament reconstruction (ACLR) cannot decrease the risk of knee osteoarthritis. A study suggested that patients with a single ACL rupture have a 10%–13% risk of developing knee osteoarthritis 10 years after injury (Oiestad et al., 2009). This risk increases from 21% to 48% in patients with a torn meniscus associated with ACL rupture (Oiestad et al., 2009). ACLR surgery is a common treatment for ACL rupture, but previous studies have shown that ACLR surgery cannot reduce the incidence of knee osteoarthritis (Lohmander et al., 2007; Barenius et al., 2014; Cinque et al., 2018; Grassi et al., 2022). A study showed that the incidence of knee osteoarthritis on the ACLR surgical side increased by three times compared with the contralateral side (Barenius et al., 2014). Studies have also shown that, on average, 50% of patients with ACL or meniscus tears will develop knee osteoarthritis after 10–20 years (Lohmander et al., 2007). Despite the high incidence of knee osteoarthritis after ACLR, the exact cause remains unclear.
There will be a series of changes in biomechanical characteristics for ACLR patients in daily activities, which may increase the risk of knee osteoarthritis. Studies conducted on patients who have undergone ACLR have reported a decrease in peak knee flexion angles (Roewer et al., 2011; Hall et al., 2012; Webster et al., 2012; Saxby et al., 2016), knee flexion moments (Bush-Joseph et al., 2001; Lewek et al., 2002; Webster et al., 2005; Di Stasi et al., 2013; Zabala et al., 2013), and external knee adduction moments (Webster and Feller, 2012; Zabala et al., 2013; Kaur et al., 2016) during prolonged walking or jogging after the surgery. Additionally, ACLR patients’ muscle activation strategy also changed at the same time, which is known in rehabilitation field as arthrogenic muscle inhibition. After ACLR, quadriceps activation failure caused by neural inhibition occurs (Sonnery-Cottet et al., 2019). Mechanisms for this phenomenon include changes in muscle resting motor thresholds, in the discharge of articular sensory receptors (Rice and McNair, 2010) and in the cortical activity (Baumeister et al., 2008; Baumeister et al., 2011). Arthrogenic muscle inhibition also shows in the daily movement. Several studies’ results suggested that there is an increase in hamstring activation (Knoll et al., 2004; Mantashloo et al., 2020) and a decrease in quadriceps femoris activation (Leporace et al., 2016) among ACLR patients during walking. The altered biomechanics in movement will significantly affect the mechanical environment of the knee joint, which may increase the risk of knee osteoarthritis. Bone loads are crucial to the growth and remodeling process of joint tissues (Beaupre et al., 2000; Carter et al., 2004). There is a dynamic balance between the reabsorption of bone matrix by osteoclasts and the formation of new bone by osteoblasts. However, this dynamic balance can be disrupted by abnormal loads, which may cause degenerative changes in joint tissue (Radin and Paul, 1971). Therefore, assessing tibial contact forces can improve understanding of knee osteoarthritis mechanisms.
The contribution of muscle forces cannot be ignored when assessing the tibial contact force, but evaluating muscle forces non-invasively during movement has always been a challenge because the human musculoskeletal system has redundancy (Crowninshield and Brand, 1981; Lloyd and Besier, 2003). Two studies estimated tibial contact forces for ACLR patients, and both used the EMG-driven method to evaluate muscle forces during a gait task (Saxby et al., 2016; Wellsandt et al., 2016). Wellsandt et al. (Wellsandt et al., 2016) suggested that patients who get knee osteoarthritis 5 years after ACLR surgery have a smaller tibial compression force during walking. Saxby et al. (Saxby et al., 2016) obtained the consistent result that the patients in the ACLR group show a smaller tibial compression force than the healthy group during walking, jogging, and cutting. Although the EMG-driven method uses EMG signal as input to the musculoskeletal simulation to solve the muscle redundancy problem, it still has some limitations like the difficult measurement of EMG signal of deep muscles and the large error with experimental results caused by signal noise (Sartori et al., 2014; Hoang et al., 2019). The EMG-assisted method combines the optimization and EMG-driven methods, which can significantly improve the experimental joint moment tracking accuracy than current EMG-driven models (Sartori et al., 2014; Pizzolato et al., 2015; Hoang et al., 2019).
The purpose of this study was to compare the difference in bilateral tibial contact force for patients with unilateral ACLR during walking and jogging based on an EMG-assisted method to evaluate the risk of knee osteoarthritis. We hypothesized that: 1) ACLR patients’ tibial compression force on the surgical side would be smaller than on the healthy side during walking and jogging; 2) there would be difference in muscle forces around the knee joint between the surgical and healthy sides during walking and jogging; 3) there would be difference in lower limb kinematics between the surgical and healthy sides during walking and jogging.
Seven ACLR participants (three males and four females, height: 1.72 ± 0.13 m, weight: 67.4 ± 14.3 kg, 1.5–3.5 years post-ACLR, the surgical side of the five participants was the dominant side) participated in this study. None of the participants had other lower limb injuries and did not engage in high-intensity exercise within 24 h before the test. The study protocol and ethics were approved by the Institutional Review Board of Beijing Sport University (2019018H).
Before the gait test, the participant was in tight-fitting clothes and self-provided running shoes. Then participants warmed up and practiced to become familiar with the test process and ensure that they could step on the force plate naturally and accurately during the formal test. After that, a total of 23 reflective markers were placed on the participant’s vertex of the head, the upper edge of the sternum, the midpoint of the fourth and fifth lumbar vertebrae, and bilaterally on acromions, anterior superior iliac spines, anterior thighs, medial femoral condyles, lateral femoral condyles, tibial trochanters, medial tibial condyles, lateral fibular condyles, heels, and toe tips. Surface electrodes were placed bilaterally on the participant’s long head of biceps femoris, semitendinosus, rectus femoris, vastus medialis, vastus lateralis, medial head of gastrocnemius, and lateral head of the gastrocnemius. The skin was shaved and exfoliated, then cleaned with alcohol before electrode placement.
In the gait test, participants performed repeated trials twice for each movement, including walking and jogging at their self-selected speed on both sides. During the test, participants started from 10 m away from the force plate and walked or jogged through the data collection area at their self-selected speed. During the whole process, participants kept smooth movements without pace adjustment. The action cycle of walking was defined from the tested foot strike and untested foot off to the tested foot strike again. The action cycle of jogging was defined from the tested foot strike to the tested foot strike again.
Muscle maximum voluntary contractions (MVCs) tests were performed after the gait test. During MVC tests of the medial head of gastrocnemius and lateral head of gastrocnemius, the participants were held in a standing position with their shoulders immobilized and used maximal effort to raise their heels. During MVC tests of the rectus femoris, vastus medialis, and vastus lateralis, participants were in a seated position with knee flexion angles of about 90 degrees. After fixing the participants’ shank and thigh, they used maximal effort to extend the knee. During MVC tests of the long head of biceps femoris and semitendinosus, participants were in a seated position with knee flexion angles of about 90 degrees. After fixing the participants’ shank and thigh, they used maximal effort to bend the knee. MVCs tests were repeated three times (5 s each) on both sides for each participant, with 1-min rest between repetitions.
The reflective marker trajectories in the gait test were captured using a 14-camera motion capture system (Qualisys, Gothenburg, Sweden) at 200 Hz. The ground reaction force (GRF) in the gait test was obtained using three 3-D force plates (Kistler Instruments, Winterthur, Switzerland) at 1000 Hz. Electromyographic (EMG) data were recorded using a wireless test system (Delsys Trigno Mobile, USA) at 2000 Hz. The acquisition of marker trajectories, GRF, and EMG data were synchronized by signals from Qualisys external triggers.
Marker trajectories, GRF, and EMG data were processed by an open-source Matlab (The Mathworks, MA) toolbox (MOtoNMS v2.2). GRF data were filtered using a second-order Butterworth low-pass filter at 50 Hz. The marker trajectories data were filtered using a second-order Butterworth low-pass filter at 13 Hz. The raw EMG data were removed mean, bandpass-filtered (second-order Butterworth, 50–450 Hz), full-wave rectified, and low-pass filtered (second-order Butterworth, 6 Hz) to produce linear envelopes. EMG envelopes were standardized using the maximum value during gait and MVCs tests.
To obtain a participant’s personalized model, a generic musculoskeletal model (gait 2,392) was scaled in OpenSim version 4.0 (Stanford, CA, United States). The scaling setting detail was in the Supplementary Material (Supplementary Table S1). The 3-D marker trajectories and GRFs collected in the gait test were used to calculate joint angles and moments through the Inverse Kinematics and Inverse Dynamics (ID) tools in OpenSim. Muscle analysis tools in OpenSim were used to calculate muscle-tendon unit (MTU) lengths and moment arms.
Muscle forces were estimated using an EMG-assisted method in the OpenSim plug-in CEINMS (Pizzolato et al., 2015). Based on the purpose of this study, we only analyzed the muscle forces around knee joints. There were two stages for muscle force estimation. In the first stage, data from one of the two repeated trials were used to calibrate the neuromuscular parameters of the personalized model. Seven experimental muscle excitations were mapped to 12 MTUs based on previous studies (Lloyd and Besier, 2003; Sartori et al., 2014; Hoang et al., 2019) (Supplementary Table S2). Then, the calibration process was performed in CEINMS to adjust the neuromuscular parameters to minimize joint moment prediction errors for the knee flexion-extension. The neuromuscular parameters included tendon slack length, optimal fiber length, maximum isometric force, non-linear shape factor, and EMG-to-activation recursive filter coefficients (Lloyd and Besier, 2003). The default value of neuromuscular parameters was from the scaled model. In the second stage, the calibrated neuromuscular model was used to predict muscle forces through the EMG-assisted model in CEINMS in the remaining trials. The objective function was:
where EtrackMOM is the sum of the squared differences between EMG-assisted predicted and experimental joint moments, EtrackEMG is the sum of the squared differences between EMG-assisted predicted and experimental muscle excitations for seven MTUs recorded in the gait test, EsumEMG is the sum of squared muscle excitations for all 12 MTUs. After predicting muscle forces, tibial contact forces (knee joint applied to tibia segment) were calculated using the Joint Reaction Analysis tools in OpenSim. The reference coordinate system was the tibia coordinate system. Results of muscle forces, joint moments, and tibial contact forces were normalized to body mass.
The muscle co-contraction index (CCI) was used to reflect muscle activation strategy (Hoang et al., 2019). We analyzed the CCI of knee flexors-extensors (CCIflex-ext) in this study. The calculating function was:
where M (ext) is the mean value of knee extensors activation (rectus femoris, vastus medialis, and vastus lateralis, vastus intermedius); M (flex) is the mean value of major knee flexors activation (long head of biceps femoris, semitendinosus, semimembranosus, medial head of gastrocnemius, lateral head of the gastrocnemius). When CCIflex-ext = 0, means full co-contraction; when CCIflex-ext = 1, means no co-contraction and only flexor activation; when CCIflex-ext = −1, means no co-contraction and only extensor activation.
Tibial compression forces calculated by the musculoskeletal were qualitatively compared with available instrumented knee implant data in the OrthoLoad dataset (Bergmann and Graichen, 2008; Bergmann et al., 2014) (Jog: a 70-year-old male, weighing 81.5 kg and standing 1.74 m, who jogged at a constant speed of 1.67 m/s on a treadmill; Walk: a 74-year-old female weighing 69.1 kg and standing 1.66 m, who walked at a speed of 1.11 m/s on a treadmill with bare feet) (Supplementary Figure S1). The coefficient of determination (R2) and root-mean-square error (RMSE) was calculated for the EMG-assisted predicted and ID knee flexion-extension net moment, which was used to evaluate EMG-assisted prediction results. Shapiro-Wilk test was used to determine the normality of lower limb biomechanical indexes on both sides. The paired sample t-test was used to analyze the difference in lower limb biomechanics between the participants’ healthy and surgical sides. A type I error rate no greater than 0.05 was chosen as an indication of statistical significance. All statistical analyses were performed using SPSS 22.0 software (SPSS, Chicago, IL, United States).
The knee flexion-extension net moment predicted by the EMG-assisted method showed high R2 and low RMSE with ID results during jogging (R2 = 0.95 ± 0.05, RMSE = 0.11 ± 0.05 Nm/kg) and walking (R2 = 0.94 ± 0.04, RMSE = 0.06 ± 0.03 Nm/kg) (Figure 1).
FIGURE 1. Knee flexion-extension net moments during jogging and walking (Black solid line: mean value of ID results on the both sides for seven patients, blue dashed line: mean value of EMG-assisted results on the both sides for seven patients, grey area: standard deviation of ID results on the both sides for seven patients, blue area: standard deviation of EMG-assisted results on the both sides for seven patients).
Participants’ action cycle on the healthy side was significantly longer than the surgical side during jogging (healthy = 0.741 ± 0.031 s, surgical = 0.721 ± 0.033 s, p = 0.009) and walking (healthy = 0.925 ± 0.023 s, surgical = 0.909 ± 0.026 s, p = 0.014) (Table 1). Participants’ stance phase in jogging (healthy = 0.256 ± 0.013 s, surgical = 0.234 ± 0.017 s, p = 0.005) and swing phase in walking (healthy = 0.256 ± 0.013 s, surgical = 0.234 ± 0.017 s, p = 0.005) on the healthy side was significantly longer than the surgical side (Table 1). There was no difference in other characteristics.
Participants’ peak tibial compression force on the healthy side (68.76 ± 11.95 N/kg) was significantly higher than the surgical side (61.86 ± 5.46 N/kg) during jogging (p = 0.039) (Figure 2). There was no significant difference in the first (healthy = 43.00 ± 12.74 N/kg, surgical = 35.05 ± 12.61 N/kg, p = 0.122) and second (healthy = 31.12 ± 8.96 N/kg, surgical = 31.72 ± 14.32 N/kg, p = 0.445) peak tibial compression forces during walking between the healthy and surgical side (Figure 2).
FIGURE 2. Knee compression forces on both sides during jogging and walking (Black solid line: mean value of results on the healthy sides for seven patients, blue dashed line: mean value of results on the surgical sides for seven patients, grey area: standard deviation of results on the healthy sides for seven patients, blue area: standard deviation of results on the surgical sides for seven patients).
Participants’ rectus femoris (healthy = 32.08 ± 10.79 N/kg, surgical = 22.58 ± 9.16 N/kg, p = 0.035) and vastus medialis (healthy = 6.08 ± 2.13 N/kg, surgical = 4.75 ± 2.18 N/kg, p = 0.036) forces on the healthy side were significantly larger than the surgical side at the peak moment of tibial compression forces during jogging (Table 2; Figure 3). There was no difference in other bilateral muscle forces at the peak moment of tibial compression forces during jogging and walking (Tables 2–4 and Figures 3, 4).
TABLE 2. Muscle forces at the peak moment of tibial compression forces on the both sides during jogging.
FIGURE 3. Muscle forces on both sides during jogging (Black solid line: mean value of results on the healthy sides for seven patients, blue dashed line: mean value of results on the surgical sides for seven patients).
TABLE 3. Muscle forces at the first peak moment of tibial compression forces on the both sides during walking.
TABLE 4. Muscle forces at the second peak moment of tibial compression forces on the both sides during walking.
FIGURE 4. Muscle forces on both sides during walking (Black solid line: mean value of results on the healthy sides for seven patients, blue dashed line: mean value of results on the surgical sides for seven patients).
There was no difference in bilateral CCIflex-ext at the peak moment of tibial compression forces during jogging and walking (Table 5).
TABLE 5. CCIflex-ext at peak moments of tibial compression forces on the both sides during jogging and walking.
Participants’ knee flexion angles (healthy = 49.50 ± 3.77°, surgical = 44.51 ± 7.03°, p = 0.042) and ankle dorsiflexion angles (healthy = 23.84 ± 6.50°, surgical = 19.88 ± 5.04°, p = 0.046) on the healthy side were significantly higher than that on the surgical side at the peak moment of tibial compression forces during jogging (Table 6). Participants’ ankle dorsiflexion angles (healthy = 6.94 ± 3.85°, surgical = 4.91 ± 4.09°, p = 0.038) on the healthy side were significantly higher than that on the surgical side at the first peak moment of tibial compression forces during walking (Table 6). There was no difference in other bilateral joint angles at the peak moment of tibial compression forces during jogging and walking (Table 6).
TABLE 6. Joint angles at peak moments of tibial compression forces on the both sides during jogging and walking.
The purpose of this study was to compare the difference in bilateral tibial contact force for unilateral ACLR patients in order to assess the risk of knee osteoarthritis. The EMG-assisted method was used to predict muscle forces based on the neuromusculoskeletal model. We evaluated model simulation results in two ways. Firstly, the joint net moment calculated by EMG-assisted methods was compared to the ID joint net moment. Studies that use the EMG-driven method usually evaluated results in this way (Lloyd and Besier, 2003; Shao et al., 2009; Nikooyan et al., 2012; Sartori et al., 2012; Sartori et al., 2014). Our results showed a high correlation and low error between these two results. Secondly, the tibial compression force calculated by the model was compared with the measured values of knee implants obtained by the patients after total knee replacement when they performed similar movement tasks. Our results showed a similar trend and magnitude to Bergmann et al.’s results (Bergmann et al., 2014). Our results in jogging were larger than the Bergmann et al.’s results. This may be due to differences in the participants’ age, jogging speed, and history of joint surgery.
The results of tibial compression forces support our first hypothesis that the tibial compression force on the surgical side for ACLR patients will be smaller than on the healthy side. This study showed that the peak tibial compression force on the healthy side was higher than on the surgical side during jogging. This result is consistent with the literature. One study followed a group of ACLR patients for up to 5 years after surgery and reported that those who developed osteoarthritis 5 years later had lower tibial compression forces in their gait 6 months to 2 years after surgery than those who did not (Wellsandt et al., 2016). Another study suggested that knee joints with ACLR surgery showed lower tibial contact forces during jogging, walking, and side stepping than the healthy group (Saxby et al., 2016).
Lower tibial compression force may lead to an increased risk of knee osteoarthritis. On the one hand, the thickness of healthy cartilage increases under a higher repetitive load and joint unloading is associated with regional cartilage thinning after ACLR (Koo and Andriacchi, 2007). A smaller tibial compression force may cause regional cartilage thinning and joint degeneration. The patient’s physical activity level will gradually increase to return to daily life after surgery. If the joint load-bearing capacity has not recovered to its pre-operative level, the risk of knee osteoarthritis may be increased. On the other hand, Saxby et al. suggested that the total area of contact between the tibiofemoral articulating surfaces may reduce because of a smaller knee flexion angle and knee flexion excursion, so articular contact forces were focused to smaller regions although the magnitudes of tibial contact forces were small (Saxby et al., 2016). Our result also supported that the surgical side had a smaller knee flexion angle at the moment of peak tibial compression force than the healthy side. This may be another explanation for the increased risk of knee osteoarthritis following ACLR.
The results of biomechanical characteristics support our second and third hypothesis that there will be differences in muscle forces around the knee joint and lower limb kinematics between the surgical and healthy sides during walking and jogging. The reason for the lower tibial compression force after ACLR may be the lower quadriceps muscle forces. We analyzed the lower extremity biomechanical characteristics at the moment of peak tibial compression force to understand why the peak tibial compression force on the surgical side is smaller than that on the healthy side. In this paper, it was suggested that compared with the healthy side, the ACLR side had a smaller knee flexion and ankle dorsiflexion angle at the peak moment of tibial compression force, which was consistent with the results of multiple previous studies (Lewek et al., 2002; Tsai et al., 2012; Kaur et al., 2016; Saxby et al., 2016). The reduced knee flexion angle is a typical change of gait after ACLR. However, this change does not appear to be the cause of the decreased tibial compression force since a smaller knee flexion and ankle dorsiflexion angle should theoretically increase the tibial compression force due to the direction of the force of gravity. Therefore, we infer that changes in knee and ankle joint motion patterns are not the primary reason for the decreased tibial compression force. Instead, muscle forces around the knee are directly related to the joint load (Saxby et al., 2016), and we found that the quadriceps femoris force on the surgical side was lower than that on the healthy side, which may be responsible for the reduced tibial compression force. Interestingly, we did not find any differences in CCIflex-ext between the two sides at the peak moment of tibial compression force. The activation patterns of muscles can affect joint contact forces, and that the high degree of co-contraction of muscles may increase joint compression forces. This inference is based on the positive correlation between muscle activation levels and muscle strength. However, although this study reported differences in bilateral tibial compression forces and muscle forces, it did not show any differences in CCIflex-ext. One possible explanation is that the muscle model used in this study is based on the Hill model, which mainly includes the relationship between muscle force and muscle length, velocity, and activation level (Sartori et al., 2014; Pizzolato et al., 2015). CCIflex-ext only reflects the characteristics of muscle activation, but this is not the only factor that affects the muscle force.
The neuromusculoskeletal model for the EMG-assisted method in this paper only included knee flexion-extension degrees of freedom. Although the range of motion of the knee joint in jogging and walking is tiny, the influence of muscles around other joints on the development of knee osteoarthritis can be further analyzed in the future study. In addition, the sample size of this study was not large, and the participants’ postoperative time ranged from 1.5 to 3.5 years, which does not fully explain the time frame for the increased risk of knee osteoarthritis, but additional participant data is being collected to test the approach across a larger cohort.
Unilateral ACLR patients had smaller tibial compression forces on the surgical side than on the healthy side while jogging, which may increase the risk of knee osteoarthritis. The main reason may be that the quadriceps muscles do not exert enough force. We recommend that ACLR patients pay attention to strengthening their quadriceps muscle through strength training during the postoperative rehabilitation process, and try to increase the use of the quadriceps muscle on the surgical side to an appropriate level in daily activities.
The raw data supporting the conclusion of this article will be made available by the authors, without undue reservation.
The studies involving human participants were reviewed and approved by the Sports Science Experiment Ethics Committee of Beijing Sport University. The patients/participants provided their written informed consent to participate in this study.
TL: Conceptualization, Methodology, Software, Formal Analysis, Writing Original Draft JF: Revising the manuscript critically for important intellectual content, Supervision HuL: Revising the manuscript critically for important intellectual content, Supervision HaL: Conceptualization, Funding Acquisition, Resources, Supervision.
This study was funded by Key Program of National Natural Science Foundation of China (12132009). The support provided by the China Scholarship Council during a study of TL at the University of Auckland is acknowledged.
The authors declare that the research was conducted in the absence of any commercial or financial relationships that could be construed as a potential conflict of interest.
All claims expressed in this article are solely those of the authors and do not necessarily represent those of their affiliated organizations, or those of the publisher, the editors and the reviewers. Any product that may be evaluated in this article, or claim that may be made by its manufacturer, is not guaranteed or endorsed by the publisher.
The Supplementary Material for this article can be found online at: https://www.frontiersin.org/articles/10.3389/fphys.2023.1160261/full#supplementary-material
Agel, J., Arendt, E. A., and Bershadsky, B. (2005). Anterior cruciate ligament injury in national collegiate athletic association basketball and soccer: A 13-year review. Am. J. Sports Med. 33, 524–530. doi:10.1177/0363546504269937
Barenius, B., Ponzer, S., Shalabi, A., Bujak, R., Norlen, L., and Eriksson, K. (2014). Increased risk of osteoarthritis after anterior cruciate ligament reconstruction A 14-year follow-up study of a randomized controlled trial. Am. J. Sports Med. 42, 1049–1057. doi:10.1177/0363546514526139
Baumeister, J., Reinecke, K., Schubert, M., and Weiss, M. (2011). Altered electrocortical brain activity after ACL reconstruction during force control. J. Orthop. Res. 29, 1383–1389. doi:10.1002/jor.21380
Baumeister, J., Reinecke, K., and Weiss, M. (2008). Changed cortical activity after anterior cruciate ligament reconstruction in a joint position paradigm: An EEG study. Scand. J. Med. Sci. Sports 18, 473–484. doi:10.1111/j.1600-0838.2007.00702.x
Beaupre, G. S., Stevens, S. S., and Carter, D. R. (2000). Mechanobiology in the development, maintenance, and degeneration of articular cartilage. J. Rehabil. Res. Dev. 37, 145–151.
Bergmann, G., Bender, A., Graichen, F., Dymke, J., Rohlmann, A., Trepczynski, A., et al. (2014). Standardized loads acting in knee implants. PLoS One 9, e86035. doi:10.1371/journal.pone.0086035
Bergmann, G., and Graichen, F. (2008). OrthoLoad. Charite–Universitaetsmedizin berlin. Available at: http://www.OrthoLoad.com.
Bush-Joseph, C. A., Hurwitz, D. E., Patel, R. R., Bahrani, Y., Garretson, R., Bach, B. R., et al. (2001). Dynamic function after anterior cruciate ligament reconstruction with autologous patellar tendon. Am. J. Sports Med. 29, 36–41. doi:10.1177/03635465010290011101
Carter, D. R., Beaupre, G. S., Wong, M., Smith, R. L., Andriacchi, T. P., and Schurman, D. J. (2004). The mechanobiology of articular cartilage development and degeneration. Clin. Orthop. Relat. Res. 427, S69–S77. doi:10.1097/01.blo.0000144970.05107.7e
Cinque, M. E., Dornan, G. J., Chahla, J., Moatshe, G., and Laprade, R. F. (2018). High rates of osteoarthritis develop after anterior cruciate ligament surgery: An analysis of 4108 patients. Am. J. Sports Med. 46, 2011–2019. doi:10.1177/0363546517730072
Crowninshield, R. D., and Brand, R. A. (1981). A physiologically based criterion of muscle force prediction in locomotion. J. Biomech. 14, 793–801. doi:10.1016/0021-9290(81)90035-x
di Stasi, S. L., Logerstedt, D., Gardinier, E. S., and Snyder-Mackler, L. (2013). Gait patterns differ between ACL-reconstructed athletes who pass return-to-sport criteria and those who fail. Am. J. Sports Med. 41, 1310–1318. doi:10.1177/0363546513482718
Grassi, A., Pizza, N., Al-Zu’Bi, B. B. H., Fabbro, G. D., Lucidi, G. A., and Zaffagnini, S. (2022). Clinical outcomes and osteoarthritis at very long-term follow-up after ACL reconstruction: A systematic review and meta-analysis. Orthop. J. Sports Med. 10, 23259671211062238. doi:10.1177/23259671211062238
Hall, M., Stevermer, C. A., and Gillette, J. C. (2012). Gait analysis post anterior cruciate ligament reconstruction: Knee osteoarthritis perspective. Gait Posture 36, 56–60. doi:10.1016/j.gaitpost.2012.01.003
Hoang, H. X., Diamond, L. E., Lloyd, D. G., and Pizzolato, C. (2019). A calibrated EMG-informed neuromusculoskeletal model can appropriately account for muscle co-contraction in the estimation of hip joint contact forces in people with hip osteoarthritis. J. Biomech. 83, 134–142. doi:10.1016/j.jbiomech.2018.11.042
Kaur, M., Ribeiro, D. C., Theis, J. C., Webster, K. E., and Sole, G. (2016). Movement patterns of the knee during gait following ACL reconstruction: A systematic review and meta-analysis. Sports Med. 46, 1869–1895. doi:10.1007/s40279-016-0510-4
Knoll, Z., Kiss, R. M., and Kocsis, L. (2004). Gait adaptation in ACL deficient patients before and after anterior cruciate ligament reconstruction surgery. J. Electromyogr. Kinesiol 14, 287–294. doi:10.1016/j.jelekin.2003.12.005
Koo, S., and Andriacchi, T. P. (2007). A comparison of the influence of global functional loads vs. local contact anatomy on articular cartilage thickness at the knee. J. Biomech. 40, 2961–2966. doi:10.1016/j.jbiomech.2007.02.005
Leporace, G., Metsavaht, L., Pereira, G. R., Oliveira, L. P., Crespo, B., and Batista, L. A. (2016). Knee synergism during gait remain altered one year after acl reconstruction. Acta Ortop. Bras. 24, 137–141. doi:10.1590/1413-785220162403153479
Lewek, M., Rudolph, K., Axe, M., and Snyder-Mackler, L. (2002). The effect of insufficient quadriceps strength on gait after anterior cruciate ligament reconstruction. Clin. Biomech. (Bristol, Avon) 17, 56–63. doi:10.1016/s0268-0033(01)00097-3
Lloyd, D. G., and Besier, T. F. (2003). An EMG-driven musculoskeletal model to estimate muscle forces and knee joint moments in vivo. J. Biomech. 36, 765–776. doi:10.1016/s0021-9290(03)00010-1
Lohmander, L. S., Englund, P. M., Dahl, L. L., and Roos, E. M. (2007). The long-term consequence of anterior cruciate ligament and meniscus injuries: Osteoarthritis. Am. J. Sports Med. 35, 1756–1769. doi:10.1177/0363546507307396
Mantashloo, Z., Letafatkar, A., and Moradi, M. (2020). Vertical ground reaction force and knee muscle activation asymmetries in patients with ACL reconstruction compared to healthy individuals. Knee Surg. Sports Traumatol. Arthrosc. 28, 2009–2014. doi:10.1007/s00167-019-05743-5
Nikooyan, A. A., Veeger, H. E., Westerhoff, P., Bolsterlee, B., Graichen, F., Bergmann, G., et al. (2012). An EMG-driven musculoskeletal model of the shoulder. Hum. Mov. Sci. 31, 429–447. doi:10.1016/j.humov.2011.08.006
Oiestad, B. E., Engebretsen, L., Storheim, K., and Risberg, M. A. (2009). Knee osteoarthritis after anterior cruciate ligament injury: A systematic review. Am. J. Sports Med. 37, 1434–1443. doi:10.1177/0363546509338827
Pizzolato, C., Lloyd, D. G., Sartori, M., Ceseracciu, E., Besier, T. F., Fregly, B. J., et al. (2015). Ceinms: A toolbox to investigate the influence of different neural control solutions on the prediction of muscle excitation and joint moments during dynamic motor tasks. J. Biomech. 48, 3929–3936. doi:10.1016/j.jbiomech.2015.09.021
Radin, E. L., and Paul, I. L. (1971). Response of joints to impact loading. I. in vitro wear. Arthritis Rheum. 14, 356–362. doi:10.1002/art.1780140306
Rice, D. A., and Mcnair, P. J. (2010). Quadriceps arthrogenic muscle inhibition: Neural mechanisms and treatment perspectives. Semin. Arthritis Rheum. 40, 250–266. doi:10.1016/j.semarthrit.2009.10.001
Roewer, B. D., di Stasi, S. L., and Snyder-Mackler, L. (2011). Quadriceps strength and weight acceptance strategies continue to improve two years after anterior cruciate ligament reconstruction. J. Biomech. 44, 1948–1953. doi:10.1016/j.jbiomech.2011.04.037
Sartori, M., Farina, D., and Lloyd, D. G. (2014). Hybrid neuromusculoskeletal modeling to best track joint moments using a balance between muscle excitations derived from electromyograms and optimization. J. Biomech. 47, 3613–3621. doi:10.1016/j.jbiomech.2014.10.009
Sartori, M., Reggiani, M., Farina, D., and Lloyd, D. G. (2012). EMG-driven forward-dynamic estimation of muscle force and joint moment about multiple degrees of freedom in the human lower extremity. PLoS One 7, e52618. doi:10.1371/journal.pone.0052618
Saxby, D. J., Bryant, A. L., Modenese, L., Gerus, P., Killen, B. A., Konrath, J., et al. (2016). Tibiofemoral contact forces in the anterior cruciate ligament-reconstructed knee. Med. Sci. Sports Exerc 48, 2195–2206. doi:10.1249/MSS.0000000000001021
Shao, Q., Bassett, D. N., Manal, K., and Buchanan, T. S. (2009). An EMG-driven model to estimate muscle forces and joint moments in stroke patients. Comput. Biol. Med. 39, 1083–1088. doi:10.1016/j.compbiomed.2009.09.002
Sonnery-Cottet, B., Saithna, A., Quelard, B., Daggett, M., Borade, A., Ouanezar, H., et al. (2019). Arthrogenic muscle inhibition after ACL reconstruction: A scoping review of the efficacy of interventions. Br. J. Sports Med. 53, 289–298. doi:10.1136/bjsports-2017-098401
Tsai, L. C., Mclean, S., Colletti, P. M., and Powers, C. M. (2012). Greater muscle co-contraction results in increased tibiofemoral compressive forces in females who have undergone anterior cruciate ligament reconstruction. J. Orthop. Res. 30, 2007–2014. doi:10.1002/jor.22176
Webster, K. E., and Feller, J. A. (2012). Tibial rotation in anterior cruciate ligament reconstructed knees during single limb hop and drop landings. Clin. Biomech. (Bristol, Avon) 27, 475–479. doi:10.1016/j.clinbiomech.2011.12.008
Webster, K. E., Feller, J. A., and Wittwer, J. E. (2012). Longitudinal changes in knee joint biomechanics during level walking following anterior cruciate ligament reconstruction surgery. Gait Posture 36, 167–171. doi:10.1016/j.gaitpost.2012.02.004
Webster, K. E., Wittwer, J. E., O'Brien, J., and Feller, J. A. (2005). Gait patterns after anterior cruciate ligament reconstruction are related to graft type. Am. J. Sports Med. 33, 247–254. doi:10.1177/0363546504266483
Wellsandt, E., Gardinier, E. S., Manal, K., Axe, M. J., Buchanan, T. S., and Snyder-Mackler, L. (2016). Decreased knee joint loading associated with early knee osteoarthritis after anterior cruciate ligament injury. Am. J. Sports Med. 44, 143–151. doi:10.1177/0363546515608475
Yu, B., and Garrett, W. E. (2007). Mechanisms of non-contact ACL injuries. Br. J. Sports Med. 41 (1), 47–51. doi:10.1136/bjsm.2007.037192
Keywords: anterior cruciate ligament reconstruction, knee osteoarthritis, tibial contact force, EMG-assisted model, gait
Citation: Long T, Fernandez J, Liu H and Li H (2023) Evaluating the risk of knee osteoarthritis following unilateral ACL reconstruction based on an EMG-assisted method. Front. Physiol. 14:1160261. doi: 10.3389/fphys.2023.1160261
Received: 07 February 2023; Accepted: 10 April 2023;
Published: 21 April 2023.
Edited by:
Qipeng Song, Shandong Sport University, ChinaReviewed by:
Nathan D. Schilaty, University of South Florida, United StatesCopyright © 2023 Long, Fernandez, Liu and Li. This is an open-access article distributed under the terms of the Creative Commons Attribution License (CC BY). The use, distribution or reproduction in other forums is permitted, provided the original author(s) and the copyright owner(s) are credited and that the original publication in this journal is cited, in accordance with accepted academic practice. No use, distribution or reproduction is permitted which does not comply with these terms.
*Correspondence: Ting Long, bG9uZ3RpbmcwODE3QDE2My5jb20=; Hanjun Li, bGloYW5qdW5AYnN1LmVkdS5jbg==
Disclaimer: All claims expressed in this article are solely those of the authors and do not necessarily represent those of their affiliated organizations, or those of the publisher, the editors and the reviewers. Any product that may be evaluated in this article or claim that may be made by its manufacturer is not guaranteed or endorsed by the publisher.
Research integrity at Frontiers
Learn more about the work of our research integrity team to safeguard the quality of each article we publish.