- 1Department of Microbiology and Immunology, Drexel University College of Medicine, Philadelphia, PA, United States
- 2Department of Psychiatry, New York University School of Medicine, New York City, NY, United States
The dopamine transporter (DAT) plays an integral role in dopamine neurotransmission through the clearance of dopamine from the extracellular space. Dysregulation of DAT is central to the pathophysiology of numerous neuropsychiatric disorders and as such is an attractive therapeutic target. DAT belongs to the solute carrier family 6 (SLC6) class of Na+/Cl− dependent transporters that move various cargo into neurons against their concentration gradient. This review focuses on DAT (SCL6A3 protein) while extending the narrative to the closely related transporters for serotonin and norepinephrine where needed for comparison or functional relevance. Cloning and site-directed mutagenesis experiments provided early structural knowledge of DAT but our contemporary understanding was achieved through a combination of crystallization of the related bacterial transporter LeuT, homology modeling, and subsequently the crystallization of drosophila DAT. These seminal findings enabled a better understanding of the conformational states involved in the transport of substrate, subsequently aiding state-specific drug design. Post-translational modifications to DAT such as phosphorylation, palmitoylation, ubiquitination also influence the plasma membrane localization and kinetics. Substrates and drugs can interact with multiple sites within DAT including the primary S1 and S2 sites involved in dopamine binding and novel allosteric sites. Major research has centered around the question what determines the substrate and inhibitor selectivity of DAT in comparison to serotonin and norepinephrine transporters. DAT has been implicated in many neurological disorders and may play a role in the pathology of HIV and Parkinson’s disease via direct physical interaction with HIV-1 Tat and α-synuclein proteins respectively.
1 Introduction
The solute carrier 6 (SLC6) family consists of a class of membrane proteins that utilize the Na+/Cl− gradient to transport various substrates across the membrane (Pramod et al., 2013). The substrates include monoamines such as dopamine (DA), serotonin, norepinephrine and other neurotransmitters like GABA, amino acids or amino acid-like molecules such as glycine, creatine, and taurine. SLC6A3 is the dopamine transporter (DAT) involved in the release and reuptake of dopamine from the synapse and extrasynaptic space into neurons and is integral to dopaminergic neurotransmission (Amara and Pacholczyk, 1991). DAT is localized predominantly to DA neurons that form the mesolimbic, mesocortical, and mesostriatal pathways further supporting the role of DAT in dopaminergic neurotransmission (Ciliax et al., 1999). DAT is abundantly visualized using immunohistochemistry in cell bodies, axons and dendrites of DA neurons in the ventral tegmental area (VTA), substantia nigra (SNc) (Ciliax et al., 1995; Nirenberg et al., 1997a), and presynaptic terminals of the nucleus accumbens (NAc) (Xu and Chen, 2020). In the mesocortical pathway, DAT has been localized to motor, prefrontal, anterior cingulate, and visual cortex suggesting a major role in motivational and cognitive behaviors (Ciliax et al., 1999). Dysregulation of DAT is linked to several disorders including depression, bipolar disorder (BD), and attention-deficit/hyperactivity disorder (ADHD) (Reith et al., 2022). DAT is a major target for drugs of abuse including cocaine, amphetamine (AMPH), and methamphetamine (METH) as well as various medications such as methylphenidate and bupropion. In this review, we will summarize the structure, function, conformational dynamics, posttranslational modifications (PTMs), and interactions of DAT with ligands and other proteins such as Tat and α-synuclein.
2 Structure of DAT
All SLC6 transporters possess 12 transmembrane (TM) helices and share a high degree of sequence homology and three-dimensional structural architecture. The structural and conformational information of most members of SLC family including DAT was obtained from the bacterial leucine transporter (LeuT) which has high sequence similarity to the eukaryotic SLC6 transporters (Penmatsa and Gouaux, 2014; Grouleff et al., 2015). The crystal structure of LeuT was first resolved in 2005 and all early efforts at developing structure-function studies of DAT were inferred from homology models using LeuT as a template (Indarte et al., 2008). Recently the crystal structures of both transport-inactive and transport-active drosophila melanogaster DAT (dDAT) have been co-crystallized with a variety of ligands and these structures have provided more accurate insights into the structural models of human DAT (hDAT) (Penmatsa et al., 2013; Wang et al., 2015). Crystal structures of dDAT confirmed the architecture of hDAT is comprised of 12 TM helices interspersed with intracellular and extracellular loops (Figure 1). Similar to the structure of LeuT, TM one to five and 6–10 exhibit pseudo-two-fold symmetry along the plane of the membrane as represented by two triangles in Figure 1. The non-helical mid-regions of TM1 and TM6 along with TM3 and TM8 provide the binding site for the substrate and the ions while TM11 and TM12 occupy the peripheral position. Although the structure shows high-level similarity with LeuT, there are some specific structural and functional differences that make DAT specific for the transport of dopamine across the membrane. For example, a kink in the mid-region of TM12 (due to Pro572) in dDAT is different from LeuT. Additionally, cholesterol molecules occupy the grooves between TM5:TM7 and TM2:TM7 which have been demonstrated to influence the conformation of DAT during the transport cycle of the substrate (Hong and Amara, 2010; Zeppelin et al., 2018). While dDAT and hDAT have similar structural topology, caution is required to interpret structure-activity relationships of inhibitors that bind to hDAT because dDAT has a norepinephrine transporter-like inhibitor pharmacology (Pörzgen et al., 2001).
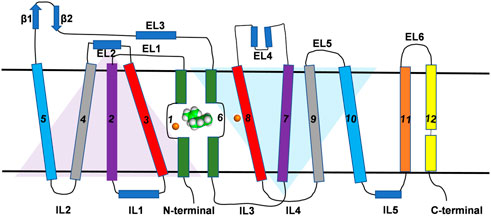
FIGURE 1. Topological representation of DAT. The TM helices, intracellular loops and extracellular loops are labeled. The orange spheres represent the Na+ ions. Green and white spherical model represents the substrate molecule. Two inverted triangles represent the two domains with an inverted axis of symmetry that contributes to substrate binding pocket.
3 Conformational dynamics of DAT
The crystal structure of bacterial LeuT in various conformational states provided an excellent insight into the events of the transport cycle of DAT (Penmatsa and Gouaux, 2014). During the transport cycle, DAT attains three putative conformational states: outward-open, substrate-occluded, and inward-open states (Figure 2). In addition, intermediate states including the outward-occluded and inward-occluded states that can be inferred from the crystal structures of LeuT and SERT (MaryCheng and Bahar, 2015; Coleman et al., 2019; Gotfryd et al., 2020). The outward-open structure is the apo form of the protein and opens towards the extracellular site. After the substrates and ions enter through the opening and bind to their respective sites, extracellular loop 4 (EL4) locks the opening of the gate and the transporter shifts to the substrate occluded state. Subsequently, the transporter undergoes conformational changes resulting in the opening of the lumen to the intracellular side and giving it the aptly named inward-open state. The crystal structures of dDAT are only available in the outward-open conformational state (Penmatsa et al., 2013; Wang et al., 2015); however, the crystal structures of LeuT have provided additional insight into the structural changes associated with other transition states. Site-mutagenesis experiments and molecular dynamics (MD) simulations have revealed further details on the role of specific residues and the movement of specific TM domains within DAT (Loland et al., 2002; Loland et al., 2004; Kniazeff et al., 2008; MaryCheng and Bahar, 2015; Xu and Chen, 2022). In particular, the intracellular network between the N-terminus TM1, intracellular loop 1 (IL1), IL3 and TM8 consisting of ionic and cation-pi interactions is conserved among many members of the SLC6 family (Kniazeff et al., 2008). This network of interactions is believed to contribute to the opening and closing of the intracellular gate (Kniazeff et al., 2008). In hDAT, salt bridge between Arg60 and Asp436, and cation-pi interaction between Arg60 and Tyr335 have been identified to stabilize the outward-open conformation (Kniazeff et al., 2008; Kniazeff et al., 2008). Tyr335, located in IL3, largely alters the distribution between different conformational states in the transport cycle and modulates the effect of Zn2+ binding to hDAT (Loland et al., 2002) which is predicted to bind and stabilize the outward-open state (Loland et al., 1999; Stockner et al., 2013).
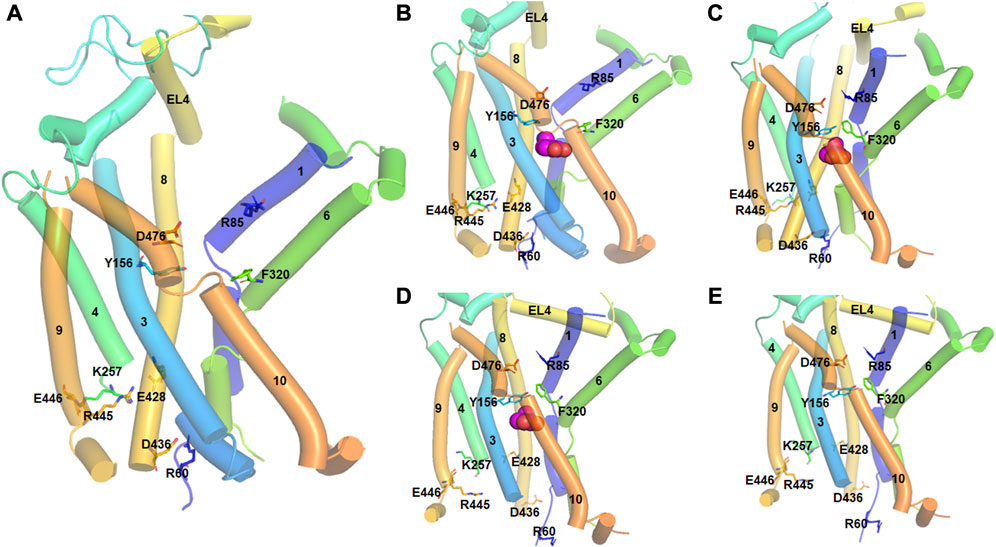
FIGURE 2. Different conformational states of hDAT during the transport cycle. The TM helices are numbered and represented by tubes. Important residues involved in conformational changes are shown in stick model and labeled. Substrate molecule (DA) is represented in spherical model. (A). In the outward-open state (apo form), the salt bridge between D476 (TM10) and R85 (TM1) which acts as the first extracellular gate is interrupted. Similarly, the interaction between Y156 and F320 which function as the second extracellular gate is also broken. At the intracellular side, there are strong ionic interactions between E448-K257, R445-E428 and D436-R60 residues. Particularly, D436-R60 acts as the intracellular gate keeper. (B). In the outward-open substrate bound state, a DA molecule binds at the orthostatic binding site. All the ionic interactions are the same as in the apo form. (C). In the substrate-occluded state, the extracellular gates, D476-R85 and Y156 and F320 are closed. There is also movement of the EL4 loop to close the extracellular vestibule. (D). In the inward-open state, the ionic interactions E448-K257, R445-E428 are interrupted, and the intracellular gate formed by D436-R60 is opened. (E). In the inward-open state (apo from), DA is released into the intracellular sites and the cycle is reset.
A study by Cheng et al. using all atom MD simulations demonstrated that TM5 and TM10 reorientations facilitate the transition from outward-open to inward-open states (MaryCheng and Bahar, 2015). This study also showed that the formation of a salt bridge between Arg445 and Glu428 is critical for the stability of the open state of the intracellular vestibule. Site-directed mutagenesis and structure-based analysis have revealed that this salt bridge enforces the inward-closed protein state, as R445 acts as a trigger for the inward-open state when the salt bridge is broken (Reith et al., 2018).
Recently, the importance of the N-terminal region in the conformational dynamics of hDAT was highlighted by MD simulations (Xu and Chen, 2022). This study demonstrates that when the N-terminal region interacts with the various intracellular loops and C-terminus, hDAT tends to attain an outward-open conformation. Conversely, when the N-terminal region mainly occupies the cytosolic region, an inward-open conformation of hDAT is observed.
A representative set of DAT substrates/inhibitors with their putative binding site and DAT’s preferred conformational state is indicated in Table 1. The conformational preferences were determined experimentally using either the cysteine accessibility experiments or by comparing binding affinity to specific mutants that shift the conformational state. For example, Tyr335Ala mutation shifts the equilibrium towards an inward-open state (Loland et al., 2002; Loland et al., 2004) while Trp84Leu and Asp313Asn mutation bias DAT conformation towards the outward-open state (Chen et al., 2001). Solvent accessibility measurements indicate Cys129 is highly solvent accessible in the outward-open state of DAT but not in the inward-open or occluded state (Loland et al., 2008). Similarly, the Ile159Cys mutant (in EL2 of DAT) provides better accessibility to bind to either R or S modafinil (Loland et al., 2012). Most DAT inhibitors have the propensity to bind either in the outward-open or the substrate-occluded states of DAT (Table 1). A few inhibitors such as JHW007 (benztropine analogue), SRI-31142, GBR12909, and ibogaine tend to stabilize the inward-open state of DAT and represent a type of atypical inhibitors (Reith et al., 2015; Newman et al., 2019). Computational docking studies have provided valuable insights into their binding mode and suggest that they may bind to either the S1 or the S2 site without disrupting the hydrogen bond gating between Asp79 and Tyr156 (Schmitt and Reith, 2011) which acts as an extracellular gate and preventing the transition of DAT to the outward-open state. In contrast, typical inhibitors like cocaine disrupt this extracellular gate thereby favoring an outward-open state of DAT.
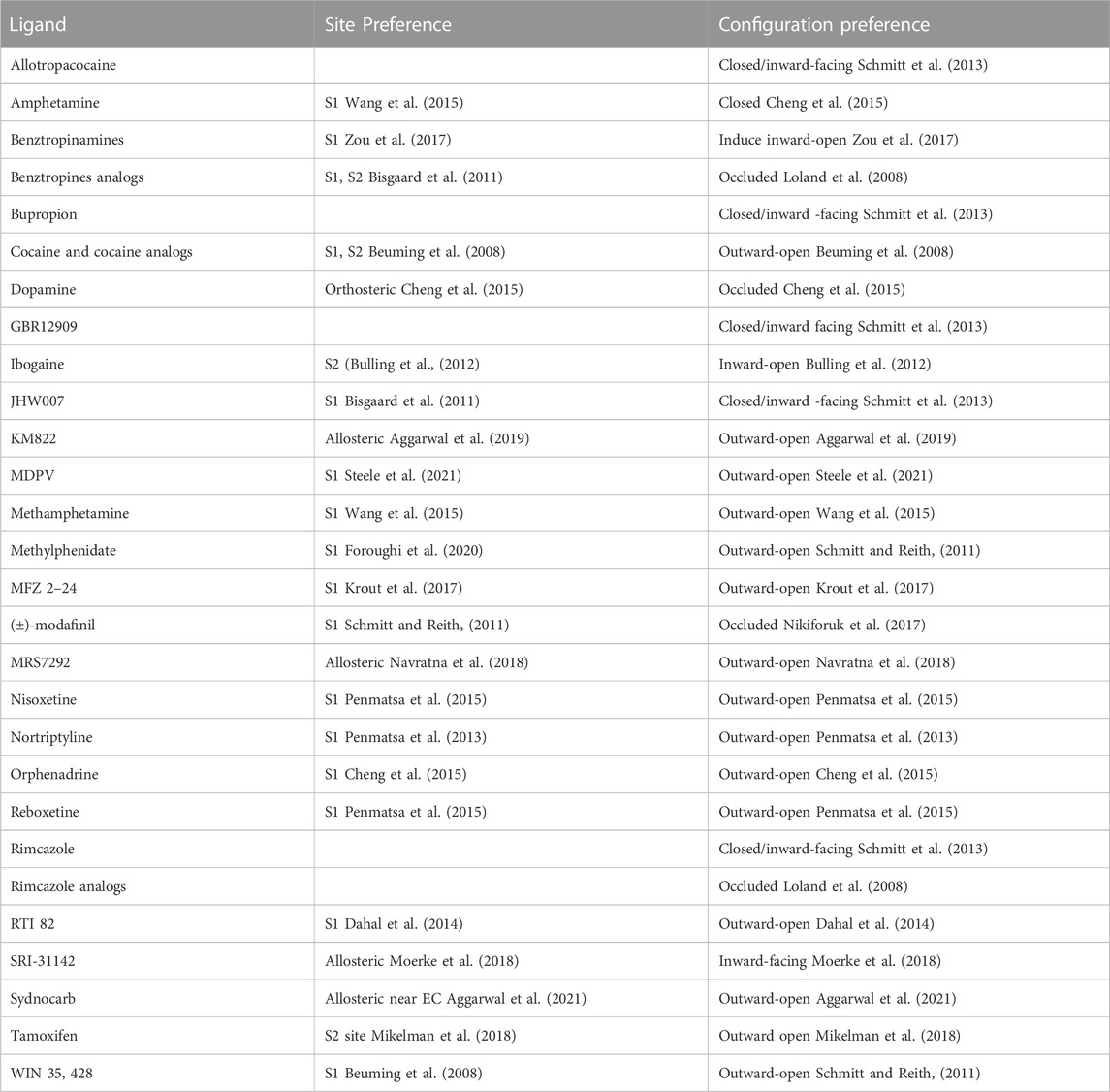
TABLE 1. List of some DAT substrates/inhibitors with their preferential binding site and DAT’s conformational state.
The binding of both substrate and Na+ was also shown to trigger conformational changes in DAT (Cheng et al., 2018; Nielsen et al., 2019). Shan et al. proposed an allosteric mechanism for the substrate transport in DAT (Shan et al., 2011). The binding of the second substrate molecule at the S2 site triggers the conformational changes at the S1 site which leads to the permeation of the water molecules at the S1 site and facilitates the inward-open conformation. The hinge regions in TM1 and TM6 enable these large conformational transitions. Cholesterol also plays an important role in stabilizing the outward-open conformation (Jones et al., 2012). In addition to these two sites, other allosteric sites that are conformation-specific have been identified using MD simulations and site directed mutagenesis/transporter truncation methods (Aggarwal et al., 2019). Small molecule allosteric modulators such as KM822 bind to these sites and allosterically modulate the binding of cocaine while restoring the substrate function (Mortensen and Kortagere, 2015; Aggarwal et al., 2019).
In a recent study, we mapped familial mutations associated with several neurological and neuropsychiatric disorders in DAT (Reith et al., 2022). Some of these mutations are located at domains of DAT that are involved in promoting conformational changes when DAT undergoes transitions during the substrate transport cycle. For example, Thr356 in hDAT facilitates a change in hDAT to the outward-open conformation upon substrate binding and promotes the substrate efflux, however, the mutation Thr356Met associated with autism spectrum disorder blocks conformational changes in hDAT leading to effects on transport cycle (Hamilton et al., 2013). Similarly, Thr62Asp-mutated hDAT is locked in an inward-open conformation. The inward-open to outward-open conformational dynamics were affected by a Tyr470His mutation in hDAT (Midde et al., 2013). Leu368Gln and Pro395Leu mutations in hDAT are found to be associated with infantile parkinsonism-dystonia presumably due to the alteration in the conformational dynamics of its transport cycle (Kurian et al., 2009). Ala559Val mutation in hDAT is linked to ADHD and this mutant DAT showed elevated levels of DA efflux at the depolarization potential (Mazei-Robison et al., 2008). Ile312Phe and Asp421Asn mutations in hDAT are associated with adult parkinsonism and ADHD (Hansen et al., 2014). Asp421Asn mutation reduced sodium binding and is hypothesized to favor the inward-open conformation of hDAT (Hansen et al., 2014).
4 Post-translational modification of DAT
Synthesis of DAT protein occurs in the soma of SNc and VTA DA neurons. It is then processed and trafficked through the axonal processes projecting into the dorsal striatum and NAc terminals of DA neurons (Nirenberg et al., 1997b; Nirenberg et al., 1997a). Trafficking of DAT is tightly regulated by several posttranslational modifications (PTMs) including phosphorylation, palmitoylation, ubiquitination, and glycosylation which ultimately determine the localization of DAT to the cytoplasm or plasma membrane (Li et al., 2004; Sorkina et al., 2006; Gorentla et al., 2009; Vina-Vilaseca et al., 2011; Moritz et al., 2015) and regulate function by altering membrane kinetics (Patel et al., 1994; Li et al., 2004). Other regulatory mechanisms of DAT include nitrosylation and oxidation (Berman et al., 1996; Fleckenstein et al., 1997; Volz and Schenk, 2004). The sites for PTM are located on the N-terminal domain (NTD) or intracellular loop (IL) 1 and the C-terminal cytoplasmic domain (CTD) and the extracellular segments between the third and fourth transmembrane domains (EL2) (Figure 3).
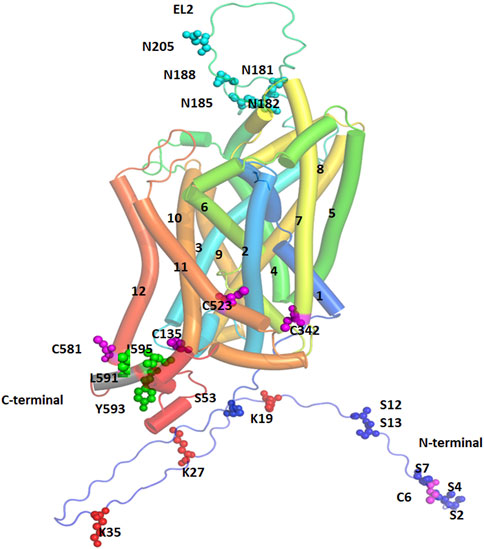
FIGURE 3. Post-Translational Modification (PTM) sites for hDAT are depicted. TM helices are labeled, and color coded. The sites of PTM are shown in spherical models and are color coded. Magenta spheres represent sites for palmitoylation, red spheres represent ubiquitination sites, cyan spheres represent sites for glycosylation, and green spheres represent the site for PKC-mediated endocytosis.
4.1 Phosphorylation
Several sites of phosphorylation have been predicted for DAT that are distributed on its NTD and some of these have been validated experimentally including serine residues 2, 4, 7, 12 and 13 (Foster et al., 2002; Granas et al., 2003; Cervinski et al., 2005; Karam and Javitch, 2018), and Thr53 (Foster et al., 2012). Several serine and threonine residues in the NTD may be phosphorylated by protein kinase C (PKC) and protein kinase A (PKA), protein kinase G, and Ca2+/Calmodulin protein kinase II, ERK1, JNK, and P38 kinases (Giambalvo, 1992; Batchelor and Schenk, 1998; Giambalvo, 2003; Lin et al., 2003; Gorentla et al., 2009). Deletion of the first 22 residues of the NTD or mutations of the 5 NTD serine residues to alanine lead to loss of phosphorylation by PKC in cultured cells suggesting that transporter phosphorylation is almost entirely limited to the NTD (Granas et al., 2003). Proline-directed phosphorylation at Thr53 by extracellular kinase1/2 (ERK1/2) increases the membrane localization and activity of DAT (Vaughan and Foster, 2013; Foster and Vaughan, 2017). The point mutation of this residue decreases the Vmax of DAT, potentially important for reverse transport when induced by amphetamine (AMPH) (Gorentla et al., 2009; Foster et al., 2012).
Phosphorylation at Thr53 (pDAT) is implicated in many functions including sleep, stress, and cocaine effects. Sleep/wake state irrespective of light/dark phase is reported to influence DA uptake via pDAT (Alonso et al., 2021). Phosphorylation of DAT at Thr53 increases plasma membrane localization which enhances the rate of DA uptake (Schmitt and Reith, 2010; Foster et al., 2012; Calipari et al., 2017; Challasivakanaka et al., 2017). Further, phosphorylation of DAT at Thr53 also contributes to enhanced DAT sensitivity to cocaine and its analogs (Foster et al., 2012; Vaughan and Foster, 2013; Challasivakanaka et al., 2017). In these studies, pDAT levels correlated with cocaine and opioid induced increases in DAT kinetics (Alonso et al., 2021; Samson et al., 2022). However, PKC-mediated phosphorylation was found to reduce the Vmax of DAT and enhanced clathrin-mediated DAT internalization. Further, treatment with PKC inhibitors blocked the internalization of DAT (Daniels and Amara, 1999; Melikian and Buckley, 1999; Sorkina et al., 2005; Foster et al., 2008; Sorkina et al., 2013) (Foster et al., 2002). Membrane DAT abundance was found to be reduced by phorbol ester through PKC-mediated phosphorylation (Copeland et al., 1996; Huff et al., 1997; Vaughan et al., 1997; Zhu et al., 1997; Daniels and Amara, 1999; Melikian and Buckley, 1999; Karam and Javitch, 2018), or via diacylglycerol analogs (Vaughan et al., 1997), or by GPCR agonists mediated Gq activation (Granas et al., 2003). The transporter is dephosphorylated by protein phosphatase (PPI or PP2A), and metabolic labeling experiments using P32 labeling confirmed that when protein phosphatases are inhibited by okadaic acid, an increase in P32 labeling occurs, suggesting a steady state reduction in phosphorylated DAT (Yang et al., 2019) (Foster and Vaughan, 2017). Although the role of NTD serine phosphorylation remains unclear, it is assumed to be involved in protein-protein interactions, regulating constitutive DAT turnover, and altering substrate transport during stimulation exposures (Khoshbouei et al., 2004; Eriksen et al., 2010; Bu et al., 2021). PKC interaction at the CTD may be involved in DAT internalization by clathrin mediated endocytosis and the required residues at the CTD are Leu591, Tyr593 and Ile595 (Boudanova et al., 2008), whereas the segment of residues from Phe587 to Leu591 is implicated in DAT downregulation (Holton et al., 2005). Further, it was found that the removal of consensus sites for PKC-mediated phosphorylation failed to create any alteration in the transportation and the internalization activity of the transporter (Holton et al., 2005; Boudanova et al., 2008). Increasing evidence suggests the dysfunctional regulation of DAT is associated with disease by creating hypo or hyper DA’ergic conditions. DA’ergic disorders are mostly associated with genetic polymorphism which alters the phosphorylation of DAT (Hahn and Blakely, 2007). ADHD and BD are two examples wherein mutation of Ala559Val (extracellular end of TM12) (Mazei-Robison et al., 2008) and Arg615Cys (CTD CamKII binding site) have been identified as causal for the alterations in DA efflux due to transporter hyperphosphorylation (Sakrikar et al., 2012). Val328Ala is another polymorphism in the transporter found in the patients of Tourette’s syndrome where enhanced trafficking-independent downregulation occurs due to hyperdopaminergia by PKC domain phosphorylation (Mazei-Robison et al., 2005). For hypodopaminergic conditions associated with DAT phosphorylation several genetic disorders like Angelman Syndrome have been linked and a decrease in CAMKII activity leading to a decrease in DA efflux has been noted (Mabb et al., 2011; Dichter et al., 2012; Steinkellner et al., 2012).
4.2 Palmitoylation
DAT is palmitoylated (16-carbon fatty acid chain) via thioester bonding mainly at Cys580 in the membrane CTD helical region (Rastedt et al., 2017). Catalysis of palmitoylation is driven by palmitoyl acetyl transferase which is also known as DHHC derived from the short peptide composed of Asp-His-His-Cys in humans (Bolland et al., 2019). DAT is depalmitoylated by acyl protein thioesterase, a superfamily of serine hydrolases and palmitoyl protein thioesterase is associated with lysosomal degradation of DAT (Fukata and Fukata, 2010). Although not belonging to these families, another member of α/β hydrolase domain containing serine hydrolase was found to depalmitoylate neuronal Post Synaptic Density 95 protein suggesting the presence of other undiscovered proteins regulating DAT palmitoylation (Yokoi et al., 2016). Among the five possible conserved Cys residues in rodents (r) and human (h), each (rDAT: 6, 135, 341, 522, 580; hDAT: 6, 135, 342, 523 and 581) are exposed for palmitoylation, and mutational studies indicate 60% reduction in incorporation at the Cys580 (rDAT) site (Rastedt et al., 2017). Furthermore, Cys581 palmitoylation of hDAT leads to formation of stable and energetically favorable hDAT dimers with functional effects on DA uptake (Zeppelin et al., 2021). Various studies found that DAT palmitoylation increases the DA uptake Vmax and reduces the likelihood of degradation whereas depalmitoylation enhances DA efflux, decreases DA uptake Vmax, and increases the occurrence of lysosomal-associated degradation similar to the effects of phosphorylation (Khoshbouei et al., 2004; Moritz et al., 2015; Wang C. et al., 2016). Activation of PKC enhances Ser7 phosphorylation which consequently reduces DAT palmitoylation, and either treatment with PKC inhibitors or Ser7Ala mutation elevates DAT palmitoylation (Vaughan et al., 1997; Foster and Vaughan, 2011; Davda et al., 2013; Moritz et al., 2015). Impairment of DAT palmitoylation may lead to several psychiatric and neurological diseases as indicated by single nucleotide polymorphisms identified in ADHD and BD (Mazei-Robison et al., 2005). Reduction of palmitoylation was linked to alteration in phosphorylation leading to increase in DA efflux and hyperdopaminergia (Khoshbouei et al., 2004; Wang Q. et al., 2016). Thus, DAT phosphorylation and palmitoylation are reciprocally regulated and alteration in palmitoylation posits DA imbalance (Moritz et al., 2015).
4.3 Ubiquitination
Ubiquitination of DAT occurs in the NTD on lysine residues in a PKC-dependent manner (Miranda et al., 2005). Mutation of three lysine residues (Lys19, 27 and 35) in the NTD of DAT lead to loss of PKC-dependent ubiquitination and endocytosis of DAT (Miranda et al., 2007). In temporary ubiquitination, DAT is recycled back to the membrane via the constitutive recycling pathway whereas permanent ubiquitination leads to DAT degradation via the late endocytic lysosomal degradation pathway (Daniels and Amara, 1999; Miranda et al., 2005; Staub and Rotin, 2006; Miranda et al., 2007). Ubiquitin ligases which degrade DAT via the lysosomal degradation pathway include neural precursor cells developmental downregulated protein four to two or NEDD4-2 (Sorkina et al., 2006) and Parkin (Jiang et al., 2004).
Small ubiquitin like modifier1 (SUMO1) and Ubc9 SUMO were reported to increase DAT levels in the plasma membrane by inducing DAT SUMOylation and reducing the possibility of DAT ubiquitination and degradation (Cartier et al., 2019). Hence, SUMOylation plays a crucial and novel role in regulating DAT proteostasis, DA uptake, and DA signaling in neurons. SUMOylation is a potential therapeutic target to increase DAT activity and DA clearance in physiological and pathological states without the use of stimulants. Numerous neurological disorders including depression, ADHD, PD, and BD are associated with functional alteration of DAT levels caused by these PTMs (Vaughan and Foster, 2013). Mutation of Parkin related to DAT ubiquitination causes PD due to loss of regulation of DAT recycling and failure to ubiquitylate misfolded transporter (Jiang et al., 2004).
4.4 Glycosylation
DAT glycosylation has been deemed necessary for the localization of DAT to the plasma membrane and to increase DA transport rate and Vmax (Patel et al., 1994; Li et al., 2004). The asparagine residue (Asn181,188, 205) on the EL2 loop between TM 2 and 3 is the target for glycosylation of DAT and mutation or removal of those sites results in reduction or blocking of DAT glycosylation (Vaughan and Kuhar, 1996; Torres et al., 2003; Li et al., 2004). Partially or non-glycosylated DATs are preferentially endocytosed compared to fully glycosylated DATs (Torres et al., 2003; Li et al., 2004) and N-glycosylation of DAT may facilitate its oligomerization in relation to its intracellular trafficking (Torres et al., 2003). Furthermore, glycosylation increases DAT’s susceptibility to bind to various drugs such as cocaine (Li et al., 2004) and reduced glycosylation of DAT in the human striatum and midbrain leads to increased PD susceptibility (Afonso-Oramas et al., 2009). These studies validate DAT as a therapeutic target and provide avenues for pharmacological manipulation of DAT to reduce disease severity.
4.5 Nitrosylation and oxidation
Nitric oxide-mediated DAT nitrosylation was found to contribute to DAT regulation. Nitrosylation occurs on the Cys residues 135 and 342 (Pogun et al., 1994; Zahniser and Doolen, 2001). L-Arg, a substrate of nitric oxide synthase (NOS) was found to enhance DAT activity by either increasing the Vmax, or decreasing Km of DA uptake (Chaparro-Huerta et al., 1997; Volz and Schenk, 2004). L-Arg was further found to be effective by protecting against sulfhydryl agents like N-ethylmaleimide (Volz and Schenk, 2004). These results suggest that L-Arg is crucial in regulating DAT activity in rat striatum through nitric oxide (Volz and Schenk, 2004). Nitric oxide was further reported to increase (Lin et al., 1995; Chaparro-Huerta et al., 1997) or decrease (Lonart and Johnson, 1994; Pogun et al., 1994) DAT activity in rat striatum or inhibit DA uptake without accelerating reversed transport of substrates (Cao and Reith, 2002). Cocaine and DA were found to block nitrosylation by inhibiting the interaction of nitric oxide with DAT at Cys135 and Cys342 due to alteration in DAT structure, however Cys90 in EL2 could be a target for nitrosylation due to its increased accessibility in the outward open conformation (Meiergerd and Schenk, 1994a; Ferrer and Javitch, 1998; Reith et al., 2001).
In addition to nitrosylation, reactive oxygen species (ROS) were also found to decrease DAT activity (Berman et al., 1996; Fleckenstein et al., 1997). ROS-mediated oxidative stress during pathological conditions including ischemia and neurodegenerative disease decreases DAT activity. It is likely that DAT may be regulated by ROS in the form of DA quinonens (Berman et al., 1996). Lipid peroxidation in striatal slices was found to suppress DAT activity (Page et al., 1998) and peroxynitrile was found to reduce DAT activity by modulation of Cys342 in IL3 (Park et al., 2002). H2O2 produced either by physiological stimulation (Sundaresan et al., 1995; Bae et al., 1997) or pathological insult like ischemia (Hyslop et al., 1995) was further found to be potential detrimental factors for DAT activity (Huang et al., 2003).
5 DAT oligomerization
Evidence for DAT oligomerization was deduced using a radiation inactivation technique that identified the tetrameric form of DAT in canine striatal membranes (Milner et al., 1994). Oligomerization is one of the key characteristics of SLC6 transporters, playing a critical role in their physiological and functional activity (Jayaraman et al., 2021). Several pieces of evidence suggest oligomerization is essential for the transport of DAT from the endoplasmic reticulum (ER) to the plasma membrane during synthesis (Sorkina et al., 2003; Ingram et al., 2021). The presence of oligomeric structures in the endosome implies oligomerization has roles in DAT endocytosis and recycling (Sorkina et al., 2003). Additionally, DAT oligomers are found in the plasma membrane as dimers, tetramers, and other higher order molecular structures (Hastrup et al., 2001; Zhen and Reith, 2018) while cysteine crosslinking experiments have detected dimer, trimer, and tetramer plasma membrane forms (Hastrup et al., 2001; Hastrup et al., 2003; Sorkina et al., 2018). The oligomerization of the various SLC6 transporters can be modulated by lipid composition (Anderluh et al., 2017) but in contrast to other SLC transporters, perturbation of cholesterol and phosphatidylinositol 4,5-bisphosphate (PIP2) do not have an intrinsic impact on hDAT oligomerization as revealed by single-molecule brightness analysis (Das et al., 2019). Single molecule microscopy also unveiled that in the ER membrane (which is devoid of PIP2) and the plasma membrane (where PIP2 is present), mixtures of monomers up to pentamers occurred for hSERT (Anderluh et al., 2017) whereas monomers and dimers were observed for hDAT regardless of PIP2 presence (Anderluh et al., 2017). However, this does not discount the role of PIP2 in SLC6 oligomer dynamics as at ER, hSERT oligomer subunits are continuously exchanged, whereas neither hDAT nor hSERT exchange in the plasma membrane even over the course of minutes (Hastrup et al., 2001; Anderluh et al., 2017). It has been concluded that PIP2 is instrumental in determining the oligomeric state of hSERT and likely also hDAT on the plasma membrane (Hastrup et al., 2001; Zhen et al., 2015). Finally, PIP2 can modulate hSERT and hDAT function (uptake, efflux, current) through precise binding interactions (Buchmayer et al., 2013; Hamilton et al., 2014; Belovich et al., 2021); however, it is not known whether these PIP2 binding sites (core protein of hSERT and hDAT, and N-terminal of hDAT) play a direct role in the quaternary arrangements.
One of the critical questions to answer is the effect of hDAT oligomerization on function. A study by Zhen et al. using copper phenanthroline (CuP) cross-linked hDAT indicates dimerization decreases the uptake activity by 25% compared to the individual protomers (Zhen and Reith, 2018). This is consistent with previous reports on other monoamine transporters SERT and NET suggesting that only one protomer in the dimer is active at a time and the activity of the one monomer could antagonize the activity of the other (Kocabas et al., 2003). Besides the DA uptake activity, oligomerization of DAT also alters the binding affinity of some inhibitors. For example, the binding affinity of the cocaine analog 2β-carbomethoxy-3β-[4-fluorophenyl]-tropane (CFT) was reduced by almost five fold on hDAT homo-dimer cross-linked with CuP in comparison to the monomeric state (Zhen and Reith, 2018). Similarly, amphetamine-stimulated DA efflux was also decreased in the dimeric form compared to the monomeric form in the same study. However, the results are more complicated with DAT hetero-oligomers when the two protomers have differential inhibitor binding affinities. For instance, a study with co-expressed DAT constructs with varying binding affinities for CFT revealed that the binding affinity in hetero-oligomers can increase or decrease in comparison to singly expressed DAT constructs where such oligomers could not be detected (Zhen et al., 2015). This indicates that DAT protomers within the oligomers do not function independently, rather a significant cooperative effect occurs among the protomers. An earlier observation implicated oligomerization of SLC6 transporters in linking drug substrate uptake with dopamine efflux (Seidel et al., 2005) and one might consider substrate transport by this class of transporters as being asymmetric depending of oligomerization (Jayaraman et al., 2021).
It is important to consider the structural features of the oligomerization interface on DAT function; however, no resolved oligomeric state crystal structures of DAT or other monoamine SLC6 family members exist to date. The dimeric crystal structure of LeuT shows TM9 and TM12 form the oligomeric interface with EL2 and EL6 loops coordinating extracellularly (Krishnamurthy and Gouaux, 2012); though, due to the presence of a kink in DAT TM12 (Pro572), the DAT oligomerization interface may differ (Penmatsa et al., 2013). Cystine crosslinking, site-directed mutagenesis, and MD simulations have provided some insights but a complete picture of the structural basis of oligomerization in DAT is still not available. Since TM11 and TM12 are surface exposed, it is expected that these helices may reside at the oligomeric interface. Indeed, biochemical studies have suggested that TM11 and TM12 contribute to the oligomeric interfaces in hSERT (Just et al., 2004). Cysteine crosslinking suggests that Cys243 in TM4 and Cys306 at the extracellular end of TM6 contribute to the oligomeric interface of hDAT dimers and higher order oligomers (Hastrup et al., 2001; Hastrup et al., 2003). Site-directed mutagenesis indicates that leucine zipper-like motifs present in TM2 may play a critical role in DAT in oligomerization (Torres et al., 2003). Similarly, truncation of the C-terminal of the hDAT suggests that the intracellular C-terminus is essential for the trafficking of DAT to the plasma membrane but does not play a significant role in oligomerization (Torres et al., 2003).
Palmitoylation on TM12 has a favorable impact on oligomerization but the functional consequences on DAT are unclear (Zeppelin et al., 2021). Many attempts to understand the conformational dynamics of DAT oligomers and to predict distinct oligomeric interfaces have been made using MD simulations (Gur et al., 2017; Jayaraman et al., 2018; Zeppelin et al., 2021). Coarse-grained MD simulations of hDAT show that TM9, TM11, and/or TM12 provide the dimeric interface for oligomerization (Zeppelin et al., 2021). Unrestrained and unbiased coarse-grained MD simulation show that hDAT can form stable dimers through 6 different interfaces that include both symmetric and asymmetric dimers (Jayaraman et al., 2018). Additionally, this simulation indicates that TM4, TM9, TM5, TM11, and extracellular and intracellular terminal ends of TM3, TM8, TM12 contribute to the dimer interface. Unfortunately, this study was unable to identify the most relevant dimer conformation that could explain DAT function (Jayaraman et al., 2018). Gur et al. carried out MD simulations of dimers of hDAT in both inward-open and outward-open conformations with dimers containing TM2, TM6, and TM11 at the oligomeric interface with Cys306 at TM6 facing each other (Gur et al., 2017). The authors predicted that dimerization facilitates the transition between inward-open to outward-open conformation enabling higher substrate transport efficiency in DAT compared to the monomeric forms; however, experimental evidence suggests dimerization decreases transport efficiency by nearly 25% for hDAT (Zhen and Reith, 2018). The effect of the small molecule inhibitor AIM-100 on inducing trimerization of DAT was studied by combined site-directed mutagenesis and MD simulation (Sorkina et al., 2021). The results show that DAT trimerization is favored by inward-open conformation, involves the hydrophobic residues in the TM4 and TM9, and inhibitors like AIM-100 facilitate the transition from outward-open to inward-open conformation (Sorkina et al., 2021).
Oligomerization of DAT on the cell surface can also be modulated by substrates and various inhibitors. Methamphetamine and AIM-100 are reported to induce higher order DAT oligomers in turn decreasing the concentration of DAT on the plasma membrane (Baucum et al., 2004; Cheng et al., 2019). AIM-100 can promote dynamin-independent DAT endocytosis presumably due to DAT oligomerization (Sorkina et al., 2021). The induction of oligomerization by methamphetamine is also observed in an in vivo study in rats (Baucum et al., 2004). However, not all stimulants can promote oligomerization of DAT as cross-linking with copper sulfate phenanthroline shows that both dopamine and amphetamine decrease DAT oligomerization (Chen and Reith, 2008; Li et al., 2010). In agreement with this result, amphetamine was also found to dissociate DAT oligomers induced by cocaine treatment (Siciliano et al., 2018). The above observations suggest that inhibition of DAT can regulate its oligomerization state and further influence its surface expression and function.
6 DAT ligand interaction sites S1, S2, and allosteric sites
DAT binds to several classes of ligands besides dopamine including stimulants such as cocaine and amphetamine, antidepressants like imipramine, and the allosteric modulator KM822 (Table 1) (Tatsumi et al., 1997; Saunders et al., 2000; Schmitt et al., 2013; Reith et al., 2015; Nikiforuk et al., 2017; Aggarwal et al., 2019). These ligands bind at distinct sites and hence differentially affect the activity of DAT. Early evidence for the binding of these various ligands to DAT came from site-directed mutagenesis and kinetic experiments (McElvain and Schenk, 1992; Reith and Selmeci, 1992; Meiergerd and Schenk, 1994b; Wayment et al., 1998; Lin et al., 1999; Wayment et al., 1999). These studies confirmed the effects of inhibitors and substrates on DAT function but not on their binding pose at the transporter leading to inconclusive reports on the binding of cocaine and its mechanism of action (Wayment et al., 1998; Loland et al., 2004; Chen et al., 2005; Sen et al., 2005). The crystal structures of LeuT (Yamashita et al., 2005) and dDAT (Penmatsa et al., 2013; Wang et al., 2015) with validation from site-directed mutagenesis have provided reliable results (Singh et al., 2007; Zhou et al., 2007). Accordingly, the orthosteric binding pocket, also called the S1 site, is formed by the non-helical mid-regions of TM1 and TM6 along with the mid-regions of TM3 and TM8 which binds dopamine (Figure 4). Residue Asp79 in TM1 forms a salt bridge with the amine group of dopamine, while Tyr156 in TM3 forms π-π interactions with the catechol ring. Further, Val152, Phe76, and Phe326 form the hydrophobic-aromatic cluster interactions with the catechol ring. The binding site for cocaine and its tropane analogs has been debated with some reports suggesting binding occurs at the orthosteric site while other reports demonstrating that the cocaine binds at a distinct site with shared residues from the S1 site (Figure 4) (Beuming et al., 2008; Huang et al., 2009; Wang et al., 2015). Based on the co-crystallization of various inhibitors with LeuT, a second site referred to as the S2 site was identified approximately 11Å above the substrate binding site in a vestibule open toward the extracellular side (Shi et al., 2008; Nyola et al., 2010; Khelashvili et al., 2013). This site has been shown to bind several non-competitive and allosteric inhibitors of DAT (Aggarwal et al., 2021).
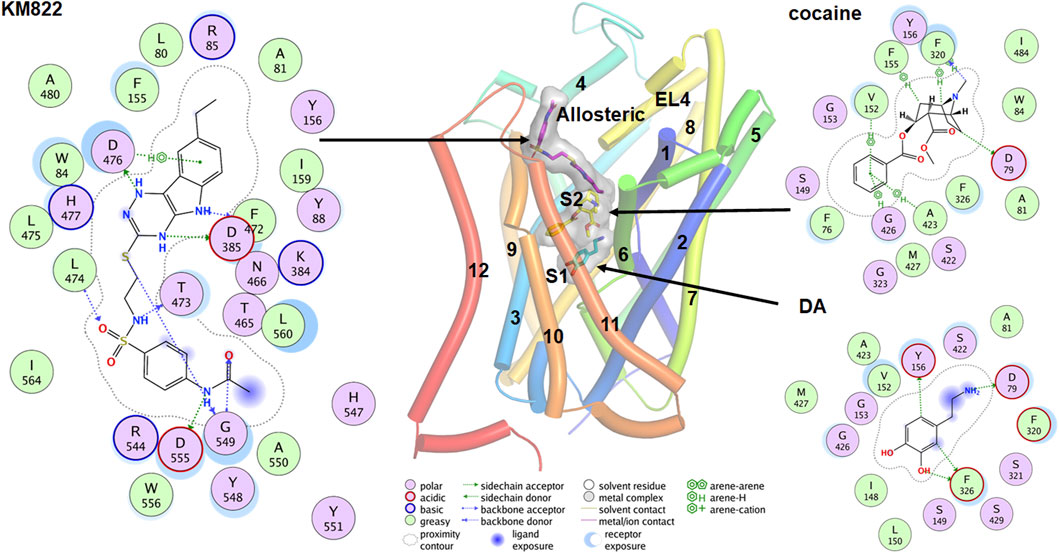
FIGURE 4. Binding poses for the DA, cocaine and KM822 molecules at the orthosteric (S1), S2, and allosteric site respectively. The ligand molecules are shown by the stick model with a transparent surface. The 2D ligand interactions map is also shown for the ligands.
Co-crystallization of DAT with the relevant ligand provides more direct evidence for the possible binding sites. Co-crystallization of the antidepressant nortriptyline with inactive drosophila DAT (dDAT) indicated that nortriptyline binds at the S1 binding site (Penmatsa et al., 2013). Similarly, co-crystallization of various ligand including dopamine, D-amphetamine, methamphetamine, cocaine, and cocaine analogs with active dDAT indicates all ligands bind at the same orthosteric binding site (Wang et al., 2015). Conformational changes in dDAT accommodate the different-size ligands at the same site (Wang et al., 2015). An MD simulation predicted that cocaine binds near the extracellular end of the substrate-entry tunnel bounded by TMs 1, 3, 6, 8, and 10 (site S2). In this conformation, Tyr88, Ile390, Phe391, and Phe472 interact with the charged head group and Leu80, Phe155, Tyr156, Ile159, and Phe330 provide a hydrophobic cavity for the benzoyl ester group of cocaine (Huang et al., 2009). A steered molecular dynamics (SMD) simulation predicted that substrate at site S2 causes a substantial allosteric effect at site S1 (Shan et al., 2011), shifting the center of mass of the substrate at the S1 site by as much as 1Å. Cheng et al. conducted combined docking and MD simulations for the binding of dopamine, amphetamine, orphenadrine, and cocaine in the hDAT model. Although their docking result showed that all ligands tend to bind near the vicinity of the S1, MD simulations showed that larger-size ligands are also stabilized at the S2 site (Cheng et al., 2015). A more recent docking and MD simulation study demonstrates that in addition to the S1, cocaine can also bind in another new allosteric site formed by TMs 3, 9, 10, and 12 (Xu and Chen, 2020). The authors claim this allosteric site has a higher affinity for cocaine than site S1, and that the binding of cocaine in this site induces profound conformational changes inhibiting the binding of dopamine at site S1 (Xu and Chen, 2020).
Although there is a substantial discrepancy in the possible binding sites for different inhibitors, it is likely that substrates bind to the S2 site and slowly translocate to the S1 site which is accompanied by some conformational changes. Related studies with bivalent substrate ligands by Schmitt et al. (2010) showed increasing DAT affinity. In this study a series of bivalent phenethylamines with the two phenylalkylamine moieties separated by an aliphatic spacer of increasing length were investigated, with one compound with an 8-carbon linker displaying an 82-fold gain in affinity. Computational modeling confirmed that the two dopamine-like pharmacophoric “heads” of this bivalent compound simultaneously occupied S1 and S2. Anderson et al. (2016) also provided evidence that both S1 and S2 can bind substrate with high affinity. The authors synthesized combinations of bivalent substrates (two substrate molecules connected by a flexible linker) which can bind to the sites S1 and S2 simultaneously. These experiments show that bivalent substrates bind with significantly higher affinity compared to their corresponding monovalent substrates and suggest that both binding sites are relevant for binding the substrate molecules.
Ligands which bind at sites other than S1 and S2 have great pharmacological potential. For example, ligands which selectively bind to the allosteric sites and inhibit the binding of cocaine without modulating DAT uptake behavior can be utilized for the treatment of cocaine-related substance use disorder. Based on this hypothesis, we designed KM822, an allosteric modulator of DAT using the hybrid structure-based screening method and demonstrated that KM822 can modulate the effects of cocaine on DAT without affecting dopamine uptake rates (Aggarwal et al., 2019). KM822 binds to a new allosteric site lined by residues Trp84, Tyr88, Phe155, Tyr156, Phe472, and His477 and hydrophilic residues Arg85, Lys384, Asp385, Thr473, and Asp476. This allosteric site is different from the new allosteric site for cocaine discovered by molecular docking and MD simulation (Xu and Chen, 2020). These findings regarding binding to the monomeric state of DAT raise the question as to whether the binding site is conserved in the oligomeric forms of DAT. It is clear that ligands like AIM-100 and methamphetamine are capable of inducing the oligomerization of DAT (Baucum et al., 2004; Sorkina et al., 2021). To understand whether they bind to the same site in oligomers as they do in the monomer, Cheng et al. (2019) did an extensive study on the trimerization of DAT induced by AIM-100 combining MD simulations, site-directed mutagenesis, and live-cell imaging. Their results show that the cavity at the trimeric interface forming the binding site for AIM-100 in oligomers was significantly different from the S2 binding site in the protomer (Cheng et al., 2019). These findings need validation using cryoEM or other methods to further understand the molecular architecture of DAT oligomers.
7 Selectivity of DAT compared to SERT and NET
DAT, SERT, and NET show an elevated degree of structural and sequence similarity with ∼40% sequence identity in humans making design of transporter-targeted drugs challenging. It can be assumed that the selectivity of these transporters is dictated by the type of residues that are present on the binding sites; however, the conformational changes of the transporters during the transport cycle (Figure 2) can largely alter the structure of the binding sites complicating the rationale for the observed selectivity for known inhibitors. As discussed, these transporters consist of well-defined ligand binding sites S1 and S2 along with other allosteric binding sites which poses significant challenges to design selective and potent drugs to target specific transporters.
Due to the limited knowledge in the structural factors that dictate selectivity, past discoveries of selective inhibitors relied on hit-and-trial or structure-activity relationship (SAR) methods. Tatsumi et al. (1997) determined the selectivity of the 37 different antidepressants for human SERT, NET, and DAT using radioligand binding assays. It is interesting that all tricyclic and amino tricyclic antidepressants are SERT selective whereas all secondary amine tricyclic antidepressants are selective for NET over SERT. Rational design of selective inhibitors has become more prominent over the last decade due to the advent of computational tools and the availability of structural information. Santra et al. synthesized several potent pyran-based inhibitors that are selective for DAT-NET or SERT or equally inhibitory towards all three (Gopishetty et al., 2011; Santra et al., 2012). Computational docking studies indicate that the stereochemistry of the-OH group at the pyran ring plays a critical role in the binding of these inhibitors (Santra et al., 2021). The-OH group facilitates a H-bond interaction with the Asp residue (Asp79 in DAT, Asp473 in NET, and Asp98 in SERT). The-OH and-OCH3 groups in the amino-benzyl substituent group are important for the selectivity for DAT and NET over SERT.
It can be assumed that the non-conserved residues at the S1 and S2 sites along with the EL4 which acts as the gatekeeper for the inhibitor entry to the transporter dictate the selectivity of the ligands. Andersen et al. conducted a systematic mutational analysis of the non-conserved residues at the binding sites S1 and S2, and EL4, to investigate their effect on the selectivity of substrates and inhibitors to these transporters (Andersen et al., 2015; Andersen et al., 2016). In the case of hNET but not hDAT and hSERT, the non-conserved residues in the S1 site primarily control the selectivity of the ligands indicating that selectivity of these transporters is more complex than initially thought. Koldsø et al. (2013) conducted extensive modeling and docking studies and predicted that the primary binding sites of these transporters have some specific hydrophilic residues which dictate the selectivity of these transporters. The authors further show that the extra OH group in norepinephrine does not contribute significantly to the binding. Recently, Ortore et al. (2020) performed an extensive computational docking and radioligand binding study on three human monoamine transporters and docking of 19 commonly known inhibitors revealed non-conserved residues as key elements for transporter selectivity. For example, Trp84, Arg85, Asp476, Pro387, and Phe472 dictate the hDAT selectivity and Phe431 and Ile172 are critical for hSERT selectivity. More recently, it was shown that EL2 and EL4 limit the access of the inhibitors to their binding site in SERT and act as the selectivity filter (Esendir et al., 2021). Sweeney et al. designed various chimeric structures to demonstrate the importance of C-and N-terminal regions in the substrate and common inhibitor affinities (Sweeney et al., 2017). Their result reveals that C-and N-terminal regions are also important for substrate and common inhibitor affinities for hDAT.
An interesting question is how these transporters are selective toward their corresponding neurotransmitter substrates compared to other transporters? Larsen et al. indicated that human and rat SERTs are also excellent DA transporters, but the mechanism is quite different from their serotonin transport, perhaps involving a differential role for oligomers in the two processes (Larsen et al., 2011). It is interesting to note that DA transport has an even higher maximum velocity than that of serotonin transport, although the Km for DA uptake is higher than that for serotonin transport. Furthermore, the transport requires significantly higher concentration of Na+/Cl− transport and was inhibited non-competitively in the presence of serotonin. A number of brain regions implicated in motivated behavior such as the prefrontal cortex, nucleus accumbens shell, and basolateral amygdala have low presence of DAT, and SERT and NET may become more prominent in volume transmission clearance of DA (Tatsumi et al., 1997). Besides the selectivity displayed towards various pharmacological drugs, the selectivity of DAT, SERT, and NET towards various abusive drugs like cocaine, amphetamine, and methamphetamine is also interesting. Previously, DAT was considered as the only target for psychostimulants; however, studies using DAT knockout mice have disproved this notion (Di Chiara and Imperato, 1988; Sora et al., 1998; Sora et al., 2001). This adds a further challenge to designing pharmacotherapeutic agents to treat psychostimulant addiction.
One very interesting development based on the differential selectivity of substrates for DAT, SERT, and NET is that of releasers with a mixed monoamine releasing profile (Chen and Reith, 2008). Just as certain DAT blockers have inhibitory effects at SERT and/or NET, a DA releaser can also exert a releasing effect on 5-HT and/or NE and increased 5-HT release in vivo blunts phenotypic DA-mediated behaviors (Baumann et al., 2011). When additional substitutions to a substrate drug makes it less transportable, a partial releaser can be the result (Rothman et al., 2012) and this can be observed in addition to a mixed (hybrid) profile where transmitter release at one monoamine transporter occurs but is combined with inhibition of transmitter uptake at another transporter (Blough et al., 2014). All of these complexities bestow properties to a drug making it part of the growing category of atypical monoamine transporter ligands (Chen and Reith, 2008).
8 DAT interactions with other proteins
DAT interacts with several other proteins to perform its function (Eriksen et al., 2010). For example, an in vivo study in rats revealed that the interaction between DA D2 receptor and DAT increases DAT localization to the plasma membrane and increases DA uptake activity and neurotransmission (Dickinson et al., 1999; Lee et al., 2007). Protein co-immunoprecipitation suggests that the IC3 loop of D2 receptor interacts directly with the NTD of DAT (Lee et al., 2007). It is also reported that pramipexole, a DA D3 receptor preferring agonist, can promote interactions of DAT with D2/D3 receptors and α-synuclein (Castro-Hernández et al., 2015). Interestingly, this physical interaction of DAT with D2/D3 receptors diminishes DAT reuptake activity contradicting previous results with D2 receptor (Lee et al., 2007). The direct interaction of DAT and other monoamine transporters with Syntaxin 1A is well studied (Quick, 2002; Sung et al., 2003). Syntaxin 1A interacts with the NTD of DAT and suppresses DA uptake as well as DAT phosphorylation (Lee et al., 2004; Carvelli et al., 2008; Cervinski et al., 2010). Further, direct interaction of DAT with Syntaxin 1A enhances amphetamine-induced DA efflux. Amphetamine facilitates the direct interaction of DAT with Syntaxin 1A which is dependent on the Ca2+/calmodulin-dependent protein kinase II (CaMKII) (Binda et al., 2008). Supporting the above findings, Syntaxin 1A knock out animals failed to show amphetamine-induced locomotor activity indicating amphetamine-induced DA efflux requires Syntaxin 1A (Lanzo et al., 2018). Rickhag et al. (2013) revealed that blocking the interaction of the CTD of DAT with other proteins like CaMKII diminishes amphetamine-induced DA efflux. Receptor for Activated C Kinase 1 (RACK1) is another adapter protein that directly interacts with DAT and regulates DAT activity which was validated by deletion of the DAT NTD (Franekova et al., 2008). PICK1 is a PDZ domain-containing protein that interacts with the LKV motif of the CTD of hDAT, although some studies have questioned the importance of the LKV motif (Torres et al., 2001; Bjerggaard et al., 2004). DAT uptake activity was increased by ∼2-fold when DAT was co-expressed with PICK1 in HEK-293 cells indicating positive modulation of DAT by the PICK1 protein (Torres et al., 2001). The interaction of DAT with PICK1 helps in ER export and surface expression of DAT (Bjerggaard et al., 2004). We will highlight DAT’s interactions with HIV associated protein Tat and α-synuclein, two highly disordered proteins.
8.1 Interactions of DAT with Tat
Transactivator of transcription (Tat) protein is a human immunodeficiency virus type 1 (HIV-1) secreted viral protein involved in viral replication (Feng and Holland, 1988). In patients treated with anti-retroviral therapies, low levels of Tat have been detected in the cerebrospinal fluid which has been correlated with HIV-associated neurocognitive disorders (HAND) (King et al., 2006; Bagashev and Sawaya, 2013; Zhu et al., 2018) which affects more than 50% of patients (Heaton et al., 2010). Several studies have suggested that Tat causes neurological disorders by interacting with monoamine transporters (DAT, serotonin, norepinephrine) as well as with N-methyl-D-Aspartate (NMDA) receptors in the central nervous system impairing their functional activity (Aksenova et al., 2006; Kumar et al., 2011; Purohit et al., 2011; Adeniran et al., 2021; Ayoub et al., 2023). Several experiments have confirmed that Tat protein interacts with DAT and decreases dopamine uptake activity leading to CNS neurotoxicity (Zhu et al., 2009). HAND is more severe in HIV-1 patients with cocaine use disorder due to the synergistic effect of Tat and cocaine in DAT function inhibition. Tat is a small non-structural polypeptide consisting of six domains and a NMR structure shows that other than a short helical peptide, the rest of the protein is highly disordered (Péloponèse et al., 2000; Campbell and Loret, 2009). The first region (residues 1–21) is a proline-rich domain, the second region (residues 22–37) is a cystine-rich domain, the third region (residues 38–48) is the hydrophobic-core domain, the fourth region (residues 49–59) is rich in basic residues (basic-domain), and the fifth region (60–72) is the glutamate-rich domain (Kuppuswamy et al., 1989). Region 1–72 is involved in viral transcription and is encoded by exon 1. The sixth region is the C-terminal region, is variable in length (73–86 or 73–101) depending on the HIV isolate and is encoded by exon 2. In an effort to identify interactions of Tat with DAT, protein-protein docking, and MD simulations were performed on Tat-DAT complex. The results from this study suggest that Tat allosterically modulates DAT and interacts with the outward-open conformation (Zhu et al., 2011; Midde et al., 2013; Yuan et al., 2015; Sun et al., 2019). DAT residues Tyr88, Lys92, His547, and Tyr470 are critical for binding Tat with Tyr470 forming a strong cation-π interaction with the Tat M1 residue. Similarly, His547 has hydrogen bonded interactions with Arg49 and Pro18 of Tat as confirmed by site-directed mutagenesis and DA uptake assays (Yuan et al., 2015). A contemporary site-directed mutation and computational modeling analysis indicates that Asp206 of hDAT is also a key player in Tat binding where Asp206Leu mutation inhibits Tat binding without altering the basal DA uptake (Quizon et al., 2021). Although this binding site of Tat is different from the orthosteric site at which DA binds, the allosteric site at which Tat is predicted to bind sterically obstructs the substrate entry pathway and hence may inhibit DAT function. Furthermore, since DAT cannot bind to Tat in other conformational states, it is probable that Tat binding would freeze the outward-open state and prevent DAT’s transitions to other states affecting its kinetics.
Developing an appropriate allosteric ligand that can bind to hDAT and inhibits Tat or cocaine binding to hDAT without altering DAT uptake activity is an active area of research. Ligands that can bind with the residues Tyr88 and/or Tyr470 and/or His547 without altering DA uptake activity of the hDAT would be ideal for inhibiting Tat with hDAT. A study by Sun et al. investigated the modulatory effects of two allosteric ligands SRI-20041 and SRI-30827 on cocaine- and Tat-induced inhibition of hDAT (Sun et al., 2017). Results indicate that the ligands were able to attenuate the inhibitory effect of cocaine and Tat on hDAT. In addition to the direct inhibition of DAT with Tat, there is also evidence that Tat may induce internalization of DAT and decrease its plasma membrane expression (Midde et al., 2012). Moreover, Tat inhibition of DAT is dependent on PKC-mediated phosphorylation (see Section 3).
8.2 Interactions of DAT with α-synuclein
α-synuclein is predominantly expressed by neurons in dynamic equilibrium between cytosolic and membrane-bound forms and plays a pivotal role in synaptic vesicle trafficking and neurotransmitter release. The multimeric membrane-bound form acts as the molecular chaperone assisting in the assembly of synaptic fusion proteins called the SNARE complex (Burré et al., 2010). A wide range of evidence suggested that α-synuclein is a curvature sensing and curvature generating protein and prefers to bind to membranes with high positive curvature (Nepal et al., 2018). α-synuclein contains 140 residues and consists of three domains: the N-terminal lipid-binding α-helix residues 1–60, amyloid-binding central domain (NAC) residues 61–95, and C-terminal acidic tail residues 96–140 (Wang C. et al., 2016). The NMR structure of human micelle-bound α-synuclein has been resolved; in an aqueous solution, α-synuclein adopts a random coil structure but it attains a secondary structure in membrane proximity (Ulmer et al., 2005). Co-immunoprecipitation studies suggest that α-synuclein directly interacts with DAT, modulating DAT’s uptake activity (Wersinger et al., 2003a). Single neuron confocal microscopy indicates that α-synuclein is distributed throughout the neuron but localizes to the plasma membrane in the presence of DAT (Butler et al., 2015). The non-amyloid part of α-synuclein interacts with the CTD of DAT (last 22 amino acid residues) to form a DAT/α-synuclein complex (Lee et al., 2001; Eriksen et al., 2010). There is conflicting evidence on whether α-synuclein positively or negatively modulates DA uptake activity of DAT. In vitro studies in ltk-mouse fibroblasts indicate that α-synuclein:DAT interactions promote an aberrant increase in DA uptake activity via clustering of DATs on the membrane inducing apoptosis (Lee et al., 2001). Supporting evidence from human neuronal cell lines reveal a 50% reduction in DAT activity upon 80% knockdown of α-synuclein (Fountaine and Wade-Martins, 2007). It was also shown that the knockdown of α-synuclein caused a decrease in surface expression of hDAT (Fountaine et al., 2008); conversely, Wersinger et al. (Wersinger et al., 2003a; Wersinger and Sidhu, 2003) demonstrated that α-synuclein decreases DAT uptake activity (35%–40%) in vitro studies with co-transfected cells. The authors pointed out that α-synuclein:DAT complexation does not alter DA affinity for DAT but decreases the velocity of DA uptake. Swant et al. (2011) also found that over-expression of α-synuclein decreases the uptake activity of DAT and depolarizes the cell from −36.5 mV to −20.3 mV. Decreased DA uptake by α-synuclein was also reported by Butler et al. (Butler et al., 2012; Butler et al., 2015). α-synuclein is linked to the pathogenesis of PD (Spillantini et al., 1998) and in this context it is of interest that deficient DA uptake has also been linked with early-onset PD in multiple studies by Kurian and others (Reith et al., 2022).
Although mutation of α-synuclein is not a requirement for neurotoxicity (Moussa et al., 2004), Ala30Pro and Ala53Thr mutants are commonly detected in early-onset PD (Petrucelli et al., 2008). Expression of either of these mutants with hDAT in HEK-293 cells induces substantial ATP loss when exposed to 6-hydroxydopamine; however, this loss is absent without hDAT indicating hDAT: α-synuclein interactions are required for cellular toxicity (Lehmensiek et al., 2006). A set of separate studies show that cytotoxicity of Ala30Pro and Ala53Thr mutants is debated (Wersinger et al., 2003b; Moussa et al., 2004) and may be cell-type specific (Wersinger et al., 2004). Other functions of α-synuclein include modulation of DAT plasma membrane trafficking (Oaks et al., 2013) and clathrin-mediated endocytosis (Kisos et al., 2014).
Modulation of DAT activity by α-synuclein observed in vitro is not recapitulated in vivo. α-synuclein knockdown transgenic mice did not show any alteration of DAT levels in the striatum. Furthermore, α-synuclein null mice displayed remarkable resistance to neurotoxin MPTP-induced degeneration of DA neurons (Dauer et al., 2002). Simultaneous alpha- and gamma-synuclein knocked down transgenic mice showed a high level of extracellular DA due to higher DA release (Senior et al., 2008). Triple-synuclein-null mice revealed a substantial decrease in synaptic DA neurotransmission in dorsal striatum without alterations in the number of dopaminergic neurons (Anwar et al., 2011). SYN120—a human α-synuclein transgenic mice showed DAT/α-synuclein complexes that were significantly redistributed in the striatum and substantia nigra with higher expression of striatal DAT than wild type (Bellucci et al., 2011). However Ala53Thr mutant α-synuclein expressing transgenic mice did not show any change in DAT levels in the striatum compared to wild type (Oaks et al., 2013).
9 Conclusion
The crystallization of the related bacterial protein LeuT and dDAT has greatly increased our knowledge of hDAT’s structure and subsequently our understanding of the binding sites and conformational changes of DAT during substrate transport. The availability of these structure has accelerated the development of new DAT ligands with therapeutic potential, especially atypical drugs such as the allosteric modulator class. This structural knowledge has revealed post-translational modification sites of DAT; however, there is a lack of consensus regarding the functional consequences of DAT phosphorylation. The dynamics of DAT oligomerization and the role of cholesterol during these conformational transitions are poorly understood and stymied by the use of systems that may not fully reflect the native environment. Despite our current knowledge there is still a gap in our understanding of the conformational states of hDAT during the translocation cycle, exacerbated by the lack of an hDAT crystal or cryo-EM structure. Nevertheless, the available structural and functional knowledge of DAT has already spurred the development of drugs that regulate DAT in ways not understood before, allowing optimism regarding our capability of generating novel treatments. In particular, our existing knowledge of DAT’s interaction with other proteins including HIV-1 Tat and α-synuclein could potentially drive development of other novel disease-specific therapeutics.
Author contributions
SK conceived the idea, NB, SD, MER, and SK wrote the manuscript. All authors contributed to editing and preparing the manuscript.
Acknowledgments
We thank all our collaborators who have provided valuable input into developing this review. We thank Frank Bearoff for proof reading and editing the manuscript.
Conflict of interest
The authors declare that the research was conducted in the absence of any commercial or financial relationships that could be construed as a potential conflict of interest.
Publisher’s note
All claims expressed in this article are solely those of the authors and do not necessarily represent those of their affiliated organizations, or those of the publisher, the editors and the reviewers. Any product that may be evaluated in this article, or claim that may be made by its manufacturer, is not guaranteed or endorsed by the publisher.
References
Adeniran, C., Yuan, Y., Davis, S. E., Lin, C., Xu, J., Zhu, J., et al. (2021). Binding mode of human norepinephrine transporter interacting with HIV-1 tat. ACS Chem. Neurosci. 12, 1519–1527. doi:10.1021/acschemneuro.0c00792
Afonso-Oramas, D., Cruz-Muros, I., Alvarez de la Rosa, D., Abreu, P., Giraldez, T., Castro-Hernandez, J., et al. (2009). Dopamine transporter glycosylation correlates with the vulnerability of midbrain dopaminergic cells in Parkinson's disease. Neurobiol. Dis. 36, 494–508. doi:10.1016/j.nbd.2009.09.002
Aggarwal, S., Cheng, M. H., Salvino, J. M., Bahar, I., and Mortensen, O. V. (2021). Functional characterization of the dopaminergic psychostimulant sydnocarb as an allosteric modulator of the human dopamine transporter. Biomedicines 9, 634. doi:10.3390/biomedicines9060634
Aggarwal, S., Liu, X., Rice, C., Menell, P., Clark, P. J., Paparoidamis, N., et al. (2019). Identification of a novel allosteric modulator of the human dopamine transporter. ACS Chem. Neurosci. 10, 3718–3730. doi:10.1021/acschemneuro.9b00262
Aksenova, M. V., Silvers, J. M., Aksenov, M. Y., Nath, A., Ray, P. D., Mactutus, C. F., et al. (2006). HIV-1 Tat neurotoxicity in primary cultures of rat midbrain fetal neurons: Changes in dopamine transporter binding and immunoreactivity. Neurosci. Lett. 395, 235–239. doi:10.1016/j.neulet.2005.10.095
Alonso, I. P., Pino, J. A., Kortagere, S., Torres, G. E., and Espana, R. A. (2021). Dopamine transporter function fluctuates across sleep/wake state: Potential impact for addiction. Neuropsychopharmacology 46, 699–708. doi:10.1038/s41386-020-00879-2
Amara, S. G., and Pacholczyk, T. (1991). Sodium-dependent neurotransmitter reuptake systems. Curr. Opin. Neurobiol. 1, 84–90. doi:10.1016/0959-4388(91)90014-x
Anderluh, A., Hofmaier, T., Klotzsch, E., Kudlacek, O., Stockner, T., Sitte, H. H., et al. (2017). Direct PIP(2) binding mediates stable oligomer formation of the serotonin transporter. Nat. Commun. 8, 14089. doi:10.1038/ncomms14089
Andersen, J., Ladefoged, L. K., Kristensen, T. N. B., Munro, L., Grouleff, J., Stuhr-Hansen, N., et al. (2016). Interrogating the molecular basis for substrate recognition in serotonin and dopamine transporters with high-affinity substrate-based bivalent ligands. ACS Chem. Neurosci. 7, 1406–1417. doi:10.1021/acschemneuro.6b00164
Andersen, J., Ringsted, K. B., Bang-Andersen, B., Strømgaard, K., and Kristensen, A. S. (2015). Binding site residues control inhibitor selectivity in the human norepinephrine transporter but not in the human dopamine transporter. Sci. Rep. 5, 15650. doi:10.1038/srep15650
Anwar, S., Peters, O., Millership, S., Ninkina, N., Doig, N., Connor-Robson, N., et al. (2011). Functional alterations to the nigrostriatal system in mice lacking all three members of the synuclein family. J. Neurosci. 31, 7264–7274. doi:10.1523/JNEUROSCI.6194-10.2011
Ayoub, S., Kenton, J. A., Milienne-Petiot, M., Deben, D. S., Achim, C., Geyer, M. A., et al. (2023). iTat transgenic mice exhibit hyper-locomotion in the behavioral pattern monitor after chronic exposure to methamphetamine but are unaffected by Tat expression. Pharmacol. Biochem. Behav. 222, 173499. doi:10.1016/j.pbb.2022.173499
Bae, Y. S., Kang, S. W., Seo, M. S., Baines, I. C., Tekle, E., Chock, P. B., et al. (1997). Epidermal growth factor (EGF)-induced generation of hydrogen peroxide. J. Biol. Chem. 272, 217–221. doi:10.1074/jbc.272.1.217
Bagashev, A., and Sawaya, B. E. (2013). Roles and functions of HIV-1 tat protein in the CNS: An overview. Virology J. 10, 358. doi:10.1186/1743-422X-10-358
Batchelor, M., and Schenk, J. O. (1998). Protein kinase A activity may kinetically upregulate the striatal transporter for dopamine. J. Neurosci. 18, 10304–10309. doi:10.1523/JNEUROSCI.18-24-10304.1998
Baucum, A. J., Rau, K. S., Riddle, E. L., Hanson, G. R., and Fleckenstein, A. E. (2004). Methamphetamine increases dopamine transporter higher molecular weight complex formation via a dopamine- and hyperthermia-associated mechanism. J. Neurosci. 24, 3436–3443. doi:10.1523/JNEUROSCI.0387-04.2004
Baumann, M. H., Clark, R. D., Woolverton, W. L., Wee, S., Blough, B. E., and Rothman, R. B. (2011). In vivo effects of amphetamine analogs reveal evidence for serotonergic inhibition of mesolimbic dopamine transmission in the rat. J. Pharmacol. Exp. Ther. 337, 218–225. doi:10.1124/jpet.110.176271
Bellucci, A., Navarria, L., Falarti, E., Zaltieri, M., Bono, F., Collo, G., et al. (2011). Redistribution of DAT/α-Synuclein complexes visualized by “in situ” proximity ligation assay in transgenic mice modelling early Parkinson's disease. PLOS ONE 6, e27959. doi:10.1371/journal.pone.0027959e27959
Belovich, A. N., Aguilar, J. I., Mabry, S. J., Cheng, M. H., Zanella, D., Hamilton, P. J., et al. (2021). A network of phosphatidylinositol (4,5)-bisphosphate (PIP2) binding sites on the dopamine transporter regulates amphetamine behavior in Drosophila Melanogaster. Mol. Psychiatry 26, 4417–4430. doi:10.1038/s41380-019-0620-0
Berman, S. B., Zigmond, M. J., and Hastings, T. G. (1996). Modification of dopamine transporter function: Effect of reactive oxygen species and dopamine. J. Neurochem. 67, 593–600. doi:10.1046/j.1471-4159.1996.67020593.x
Beuming, T., Kniazeff, J., Bergmann, M. L., Shi, L., Gracia, L., Raniszewska, K., et al. (2008). The binding sites for cocaine and dopamine in the dopamine transporter overlap. Nat. Neurosci. 11, 780–789. doi:10.1038/nn.2146
Binda, F., Dipace, C., Bowton, E., Robertson, S. D., Lute, B. J., Fog, J. U., et al. (2008). Syntaxin 1A interaction with the dopamine transporter promotes amphetamine-induced dopamine efflux. Mol. Pharmacol. 74, 1101–1108. doi:10.1124/mol.108.048447
Bisgaard, H., Larsen, M. A. B., Mazier, S., Beuming, T., Newman, A. H., Weinstein, H., et al. (2011). The binding sites for benztropines and dopamine in the dopamine transporter overlap. Neuropharmacology 60, 182–190. doi:10.1016/j.neuropharm.2010.08.021
Bjerggaard, C., Fog, J. U., Hastrup, H., Madsen, K., Loland, C. J., Javitch, J. A., et al. (2004). Surface targeting of the dopamine transporter involves discrete epitopes in the distal C terminus but does not require canonical PDZ domain interactions. J. Neurosci. 24, 7024–7036. doi:10.1523/JNEUROSCI.1863-04.2004
Blough, B. E., Landavazo, A., Partilla, J. S., Baumann, M. H., Decker, A. M., Page, K. M., et al. (2014). Hybrid dopamine uptake blocker–serotonin releaser ligands: A new twist on transporter-focused therapeutics. ACS Med. Chem. Lett. 5, 623–627. doi:10.1021/ml500113s
Bolland, D. E., Moritz, A. E., Stanislowski, D. J., Vaughan, R. A., and Foster, J. D. (2019). Palmitoylation by multiple DHHC enzymes enhances dopamine transporter function and stability. ACS Chem. Neurosci. 10, 2707–2717. doi:10.1021/acschemneuro.8b00558
Boudanova, E., Navaroli, D. M., Stevens, Z., and Melikian, H. E. (2008). Dopamine transporter endocytic determinants: Carboxy terminal residues critical for basal and PKC-stimulated internalization. Mol. Cell Neurosci. 39, 211–217. doi:10.1016/j.mcn.2008.06.011
Bu, M., Farrer, M. J., and Khoshbouei, H. (2021). Dynamic control of the dopamine transporter in neurotransmission and homeostasis. NPJ Park. Dis. 7, 22. doi:10.1038/s41531-021-00161-2
Buchmayer, F., Schicker, K., Steinkellner, T., Geier, P., Stübiger, G., Hamilton, P. J., et al. (2013). Amphetamine actions at the serotonin transporter rely on the availability of phosphatidylinositol-4,5-bisphosphate. Proc. Natl. Acad. Sci. 110, 11642–11647. doi:10.1073/pnas.1220552110
Bulling, S., Schicker, K., Zhang, Y.-W., Steinkellner, T., Stockner, T., Gruber, C. W., et al. (2012). The mechanistic basis for noncompetitive ibogaine inhibition of serotonin and dopamine transporters*. J. Biol. Chem. 287, 18524–18534. doi:10.1074/jbc.M112.343681
Burré, J., Sharma, M., Tsetsenis, T., Buchman, V., Etherton, M. R., and Südhof, T. C. (2010). Alpha-synuclein promotes SNARE-complex assembly in vivo and in vitro. Science 329, 1663–1667. doi:10.1126/science.1195227
Butler, B., Saha, K., and Khoshbouei, H. (2012). α-synuclein regulation of dopamine transporter. Transl. Neurosci. 3, 249–257. doi:10.2478/s13380-012-0036-7
Butler, B., Saha, K., Rana, T., Becker, J. P., Sambo, D., Davari, P., et al. (2015). Dopamine transporter activity is modulated by α-synuclein *. J. Biol. Chem. 290, 29542–29554. doi:10.1074/jbc.M115.691592
Calipari, E. S., Juarez, B., Morel, C., Walker, D. M., Cahill, M. E., Ribeiro, E., et al. (2017). Dopaminergic dynamics underlying sex-specific cocaine reward. Nat. Commun. 8, 13877. doi:10.1038/ncomms13877
Campbell, G. R., and Loret, E. P. (2009). What does the structure-function relationship of the HIV-1 Tat protein teach us about developing an AIDS vaccine? Retrovirology 6, 50. doi:10.1186/1742-4690-6-50
Cao, B. J., and Reith, M. E. (2002). Nitric oxide inhibits uptake of dopamine and N-methyl-4-phenylpyridinium (MPP+) but not release of MPP+ in rat C6 glioma cells expressing human dopamine transporter. Br. J. Pharmacol. 137, 1155–1162. doi:10.1038/sj.bjp.0704974
Cartier, E., Garcia-Olivares, J., Janezic, E., Viana, J., Moore, M., Lin, M. L., et al. (2019). The SUMO-conjugase Ubc9 prevents the degradation of the dopamine transporter, enhancing its cell surface level and dopamine uptake. Front. Cell Neurosci. 13, 35. doi:10.3389/fncel.2019.00035
Carvelli, L., Blakely, R. D., and DeFelice, L. J. (2008). Dopamine transporter/syntaxin 1A interactions regulate transporter channel activity and dopaminergic synaptic transmission. Proc. Natl. Acad. Sci. 105, 14192–14197. doi:10.1073/pnas.0802214105
Castro-Hernández, J., Afonso-Oramas, D., Cruz-Muros, I., Salas-Hernández, J., Barroso-Chinea, P., Moratalla, R., et al. (2015). Prolonged treatment with pramipexole promotes physical interaction of striatal dopamine D3 autoreceptors with dopamine transporters to reduce dopamine uptake. Neurobiol. Dis. 74, 325–335. doi:10.1016/j.nbd.2014.12.007
Cervinski, M. A., Foster, J. D., and Vaughan, R. A. (2005). Psychoactive substrates stimulate dopamine transporter phosphorylation and down-regulation by cocaine-sensitive and protein kinase C-dependent mechanisms. J. Biol. Chem. 280, 40442–40449. doi:10.1074/jbc.M501969200
Cervinski, M. A., Foster, J. D., and Vaughan, R. A. (2010). Syntaxin 1A regulates dopamine transporter activity, phosphorylation and surface expression. Neuroscience 170, 408–416. doi:10.1016/j.neuroscience.2010.07.025
Challasivakanaka, S., Zhen, J., Smith, M. E., Reith, M. E. A., Foster, J. D., and Vaughan, R. A. (2017). Dopamine transporter phosphorylation site threonine 53 is stimulated by amphetamines and regulates dopamine transport, efflux, and cocaine analog binding. J. Biol. Chem. 292, 19066–19075. doi:10.1074/jbc.M117.787002
Chaparro-Huerta, V., Beas-Zarate, C., Guerrero, M. U., and Feria-Velasco, A. (1997). Nitric oxide involvement in regulating the dopamine transport in the striatal region of rat brain. Neurochem. Int. 31, 607–616. doi:10.1016/s0197-0186(96)00141-6
Chen, N., and Reith, M. E. (2008). Substrates dissociate dopamine transporter oligomers. J. Neurochem. 105, 910–920. doi:10.1111/j.1471-4159.2007.05195.x
Chen, N., Vaughan, R. A., and Reith, M. E. A. (2001). The role of conserved tryptophan and acidic residues in the human dopamine transporter as characterized by site-directed mutagenesis. J. Neurochem. 77, 1116–1127. doi:10.1046/j.1471-4159.2001.00312.x
Chen, R., Han, D. D., and Gu, H. H. (2005). A triple mutation in the second transmembrane domain of mouse dopamine transporter markedly decreases sensitivity to cocaine and methylphenidate. J. Neurochem. 94, 352–359. doi:10.1111/j.1471-4159.2005.03199.x
Cheng, M. H., Block, E., Hu, F., Cobanoglu, M. C., Sorkin, A., and Bahar, I. (2015). Insights into the modulation of dopamine transporter function by amphetamine, orphenadrine, and cocaine binding. Front. Neurology 6, 134. doi:10.3389/fneur.2015.00134
Cheng, M. H., Kaya, C., and Bahar, I. (2018). Quantitative assessment of the energetics of dopamine translocation by human dopamine transporter. J. Phys. Chem. B 122, 5336–5346. doi:10.1021/acs.jpcb.7b10340
Cheng, M. H., Ponzoni, L., Sorkina, T., Lee, J. Y., Zhang, S., Sorkin, A., et al. (2019). Trimerization of dopamine transporter triggered by AIM-100 binding: Molecular mechanism and effect of mutations. Neuropharmacology 161, 107676. doi:10.1016/j.neuropharm.2019.107676
Ciliax, B., Heilman, C., Demchyshyn, L., Pristupa, Z., Ince, E., Hersch, S., et al. (1995). The dopamine transporter: Immunochemical characterization and localization in brain. J. Neurosci. 15, 1714–1723. doi:10.1523/JNEUROSCI.15-03-01714.1995
Ciliax, B. J., Drash, G. W., Staley, J. K., Haber, S., Mobley, C. J., Miller, G. W., et al. (1999). Immunocytochemical localization of the dopamine transporter in human brain. J. Comp. Neurology 409, 38–56. doi:10.1002/(sici)1096-9861(19990621)409:1<38:aid-cne4>3.0.co;2-1
Coleman, J. A., Yang, D., Zhao, Z., Wen, P.-C., Yoshioka, C., Tajkhorshid, E., et al. (2019). Serotonin transporter–ibogaine complexes illuminate mechanisms of inhibition and transport. Nature 569, 141–145. doi:10.1038/s41586-019-1135-1
Copeland, B. J., Vogelsberg, V., Neff, N. H., and Hadjiconstantinou, M. (1996). Protein kinase C activators decrease dopamine uptake into striatal synaptosomes. J. Pharmacol. Exp. Ther. 277, 1527–1532.
Dahal, R. A., Pramod, A. B., Sharma, B., Krout, D., Foster, J. D., Cha, J. H., et al. (2014). Computational and biochemical docking of the irreversible cocaine analog RTI 82 directly demonstrates ligand positioning in the dopamine transporter central substrate-binding site *. J. Biol. Chem. 289, 29712–29727. doi:10.1074/jbc.M114.571521
Daniels, G. M., and Amara, S. G. (1999). Regulated trafficking of the human dopamine transporter. Clathrin-mediated internalization and lysosomal degradation in response to phorbol esters. J. Biol. Chem. 274, 35794–35801. doi:10.1074/jbc.274.50.35794
Das, A. K., Kudlacek, O., Baumgart, F., Jaentsch, K., Stockner, T., Sitte, H. H., et al. (2019). Dopamine transporter forms stable dimers in the live cell plasma membrane in a phosphatidylinositol 4,5-bisphosphate-independent manner. J. Biol. Chem. 294, 5632–5642. doi:10.1074/jbc.RA118.006178
Dauer, W., Kholodilov, N., Vila, M., Trillat, A. C., Goodchild, R., Larsen, K. E., et al. (2002). Resistance of alpha -synuclein null mice to the parkinsonian neurotoxin MPTP. Proc. Natl. Acad. Sci. U. S. A. 99, 14524–14529. doi:10.1073/pnas.172514599
Davda, D., El Azzouny, M. A., Tom, C. T., Hernandez, J. L., Majmudar, J. D., Kennedy, R. T., et al. (2013). Profiling targets of the irreversible palmitoylation inhibitor 2-bromopalmitate. ACS Chem. Biol. 8, 1912–1917. doi:10.1021/cb400380s
Di Chiara, G., and Imperato, A. (1988). Drugs abused by humans preferentially increase synaptic dopamine concentrations in the mesolimbic system of freely moving rats. Proc. Natl. Acad. Sci. 85, 5274–5278. doi:10.1073/pnas.85.14.5274
Dichter, G. S., Damiano, C. A., and Allen, J. A. (2012). Reward circuitry dysfunction in psychiatric and neurodevelopmental disorders and genetic syndromes: Animal models and clinical findings. J. Neurodev. Disord. 4, 19. doi:10.1186/1866-1955-4-19
Dickinson, S. D., Sabeti, J., Larson, G. A., Giardina, K., Rubinstein, M., Kelly, M. A., et al. (1999). Dopamine D2 receptor-deficient mice exhibit decreased dopamine transporter function but No changes in dopamine release in dorsal striatum. J. Neurochem. 72, 148–156. doi:10.1046/j.1471-4159.1999.0720148.x
Eriksen, J., Jorgensen, T. N., and Gether, U. (2010). Regulation of dopamine transporter function by protein-protein interactions: New discoveries and methodological challenges. J. Neurochem. 113, 27–41. doi:10.1111/j.1471-4159.2010.06599.x
Esendir, E., Burtscher, V., Coleman, J. A., Zhu, R., Gouaux, E., Freissmuth, M., et al. (2021). Extracellular loops of the serotonin transporter act as a selectivity filter for drug binding. J. Biol. Chem. 297, 100863. doi:10.1016/j.jbc.2021.100863
Feng, S., and Holland, E. C. (1988). HIV-1 tat trans-activation requires the loop sequence within tar. Nature 334, 165–167. doi:10.1038/334165a0
Ferrer, J. V., and Javitch, J. A. (1998). Cocaine alters the accessibility of endogenous cysteines in putative extracellular and intracellular loops of the human dopamine transporter. Proc. Natl. Acad. Sci. U. S. A. 95, 9238–9243. doi:10.1073/pnas.95.16.9238
Fleckenstein, A. E., Metzger, R. R., Beyeler, M. L., Gibb, J. W., and Hanson, G. R. (1997). Oxygen radicals diminish dopamine transporter function in rat striatum. Eur. J. Pharmacol. 334, 111–114. doi:10.1016/s0014-2999(97)01175-8
Foroughi, K., Khaksari, M., and Shayannia, A. (2020). Molecular docking studies of methamphetamine and amphetamine- related derivatives as an inhibitor against dopamine receptor. Curr. Comput. Aided Drug Des. 16, 122–133. doi:10.2174/1573409915666181204144411
Foster, J. D., Adkins, S. D., Lever, J. R., and Vaughan, R. A. (2008). Phorbol ester induced trafficking-independent regulation and enhanced phosphorylation of the dopamine transporter associated with membrane rafts and cholesterol. J. Neurochem. 105, 1683–1699. doi:10.1111/j.1471-4159.2008.05262.x
Foster, J. D., Pananusorn, B., and Vaughan, R. A. (2002). Dopamine transporters are phosphorylated on N-terminal serines in rat striatum. J. Biol. Chem. 277, 25178–25186. doi:10.1074/jbc.M200294200
Foster, J. D., and Vaughan, R. A. (2011). Palmitoylation controls dopamine transporter kinetics, degradation, and protein kinase C-dependent regulation. J. Biol. Chem. 286, 5175–5186. doi:10.1074/jbc.M110.187872
Foster, J. D., and Vaughan, R. A. (2017). Phosphorylation mechanisms in dopamine transporter regulation. J. Chem. Neuroanat. 83-84 83-84, 10–18. doi:10.1016/j.jchemneu.2016.10.004
Foster, J. D., Yang, J. W., Moritz, A. E., Challasivakanaka, S., Smith, M. A., Holy, M., et al. (2012). Dopamine transporter phosphorylation site threonine 53 regulates substrate reuptake and amphetamine-stimulated efflux. J. Biol. Chem. 287, 29702–29712. doi:10.1074/jbc.M112.367706
Fountaine, T. M., Venda, L. L., Warrick, N., Christian, H. C., Brundin, P., Channon, K. M., et al. (2008). The effect of α-synuclein knockdown on MPP+ toxicity in models of human neurons. Eur. J. Neurosci. 28, 2459–2473. doi:10.1111/j.1460-9568.2008.06527.x
Fountaine, T. M., and Wade-Martins, R. (2007). RNA interference-mediated knockdown of α-synuclein protects human dopaminergic neuroblastoma cells from MPP+ toxicity and reduces dopamine transport. J. Neurosci. Res. 85, 351–363. doi:10.1002/jnr.21125
Franekova, V., Baliova, M., and Jursky, F. (2008). Truncation of human dopamine transporter by protease calpain. Neurochem. Int. 52, 1436–1441. doi:10.1016/j.neuint.2008.04.001
Fukata, Y., and Fukata, M. (2010). Protein palmitoylation in neuronal development and synaptic plasticity. Nat. Rev. Neurosci. 11, 161–175. doi:10.1038/nrn2788
Giambalvo, C. T. (2003). Differential effects of amphetamine transport vs. dopamine reverse transport on particulate PKC activity in striatal synaptoneurosomes. Synapse 49, 125–133. doi:10.1002/syn.10223
Giambalvo, C. T. (1992). Protein kinase C and dopamine transport–1. Effects of amphetamine in vivo. Neuropharmacology 31, 1201–1210. doi:10.1016/0028-3908(92)90048-t
Gopishetty, B., Hazeldine, S., Santra, S., Johnson, M., Modi, G., Ali, S., et al. (2011). Further Structure−Activity relationship studies on 4-((((3S,6S)-6-Benzhydryltetrahydro-2H-pyran-3-yl)amino)methyl)phenol: Identification of compounds with triple uptake inhibitory activity as potential antidepressant agents. J. Med. Chem. 54, 2924–2932. doi:10.1021/jm200020a
Gorentla, B. K., Moritz, A. E., Foster, J. D., and Vaughan, R. A. (2009). Proline-directed phosphorylation of the dopamine transporter N-terminal domain. Biochemistry 48, 1067–1076. doi:10.1021/bi801696n
Gotfryd, K., Boesen, T., Mortensen, J. S., Khelashvili, G., Quick, M., Terry, D. S., et al. (2020). X-ray structure of LeuT in an inward-facing occluded conformation reveals mechanism of substrate release. Nat. Commun. 11, 1005. doi:10.1038/s41467-020-14735-w
Granas, C., Ferrer, J., Loland, C. J., Javitch, J. A., and Gether, U. (2003). N-terminal truncation of the dopamine transporter abolishes phorbol ester- and substance P receptor-stimulated phosphorylation without impairing transporter internalization. J. Biol. Chem. 278, 4990–5000. doi:10.1074/jbc.M205058200
Grouleff, J., Ladefoged, L. K., Koldsø, H., and Schiøtt, B. (2015). Monoamine transporters: Insights from molecular dynamics simulations. Front. Pharmacol. 6, 235. doi:10.3389/fphar.2015.00235
Gur, M., Cheng, M. H., Zomot, E., and Bahar, I. (2017). Effect of dimerization on the dynamics of neurotransmitter:sodium symporters. J. Phys. Chem. B 121, 3657–3666. doi:10.1021/acs.jpcb.6b09876
Hahn, M. K., and Blakely, R. D. (2007). The functional impact of SLC6 transporter genetic variation. Annu. Rev. Pharmacol. Toxicol. 47, 401–441. doi:10.1146/annurev.pharmtox.47.120505.105242
Hamilton, P. J., Belovich, A. N., Khelashvili, G., Saunders, C., Erreger, K., Javitch, J. A., et al. (2014). PIP2 regulates psychostimulant behaviors through its interaction with a membrane protein. Nat. Chem. Biol. 10, 582–589. doi:10.1038/nchembio.1545
Hamilton, P. J., Campbell, N. G., Sharma, S., Erreger, K., Herborg Hansen, F., Saunders, C., et al. (2013). De novo mutation in the dopamine transporter gene associates dopamine dysfunction with autism spectrum disorder. Mol. Psychiatry 18, 1315–1323. doi:10.1038/mp.2013.102
Hansen, F. H., Skjørringe, T., Yasmeen, S., Arends, N. V., Sahai, M. A., Erreger, K., et al. (2014). Missense dopamine transporter mutations associate with adult parkinsonism and ADHD. J. Clin. Investigation 124, 3107–3120. doi:10.1172/JCI73778
Hastrup, H., Karlin, A., and Javitch, J. A. (2001). Symmetrical dimer of the human dopamine transporter revealed by cross-linking Cys-306 at the extracellular end of the sixth transmembrane segment. Proc. Natl. Acad. Sci. 98, 10055–10060. doi:10.1073/pnas.181344298
Hastrup, H., Sen, N., and Javitch, J. A. (2003). The human dopamine transporter forms a tetramer in the plasma membrane: CROSS-LINKING of a cysteine in the fourth transmembrane segment IS sensitive to cocaine analogs*. J. Biol. Chem. 278, 45045–45048. doi:10.1074/jbc.C300349200
Heaton, R. K., Clifford, D. B., Franklin, D. R., Woods, S. P., Ake, C., Vaida, F., et al. (2010). HIV-Associated neurocognitive disorders persist in the era of potent antiretroviral therapy: CHARTER study. Chart. Study 75, 2087–2096. doi:10.1212/WNL.0b013e318200d727
Holton, K. L., Loder, M. K., and Melikian, H. E. (2005). Nonclassical, distinct endocytic signals dictate constitutive and PKC- regulated neurotransmitter transporter internalization. Nat. Neurosci. 8, 881–888. doi:10.1038/nn1478
Hong, W. C., and Amara, S. G. (2010). Membrane cholesterol modulates the outward facing conformation of the dopamine transporter and alters cocaine binding *. J. Biol. Chem. 285, 32616–32626. doi:10.1074/jbc.M110.150565
Huang, C. L., Huang, N. K., Shyue, S. K., and Chern, Y. (2003). Hydrogen peroxide induces loss of dopamine transporter activity: A calcium-dependent oxidative mechanism. J. Neurochem. 86, 1247–1259. doi:10.1046/j.1471-4159.2003.01936.x
Huff, R. A., Vaughan, R. A., Kuhar, M. J., and Uhl, G. R. (1997). Phorbol esters increase dopamine transporter phosphorylation and decrease transport Vmax. J. Neurochem. 68, 225–232. doi:10.1046/j.1471-4159.1997.68010225.x
Hyslop, P. A., Zhang, Z., Pearson, D. V., and Phebus, L. A. (1995). Measurement of striatal H2O2 by microdialysis following global forebrain ischemia and reperfusion in the rat: Correlation with the cytotoxic potential of H2O2 in vitro. Brain Res. 671, 181–186. doi:10.1016/0006-8993(94)01291-o
Indarte, M., Madura, J. D., and Surratt, C. K. (2008). Dopamine transporter comparative molecular modeling and binding site prediction using the LeuTAa leucine transporter as a template. Proteins Struct. Funct. Bioinforma. 70, 1033–1046. doi:10.1002/prot.21598
Ingram, S. M., Rana, T., Manson, A. M., Yayah, F. M., Jackson, E. G. B., Anderson, C., et al. (2021). Optogenetically-induced multimerization of the dopamine transporter increases uptake and trafficking to the plasma membrane. J. Biol. Chem. 296, 100787. doi:10.1016/j.jbc.2021.100787
Jayaraman, K., Das, A. K., Luethi, D., Szöllősi, D., Schütz, G. J., Reith, M. E. A., et al. (2021). SLC6 transporter oligomerization. J. Neurochem. 157, 919–929. doi:10.1111/jnc.15145
Jayaraman, K., Morley, A. N., Szöllősi, D., Wassenaar, T. A., Sitte, H. H., and Stockner, T. (2018). Dopamine transporter oligomerization involves the scaffold domain, but spares the bundle domain. PLOS Comput. Biol. 14, e1006229. doi:10.1371/journal.pcbi.1006229e1006229
Jiang, H., Jiang, Q., and Feng, J. (2004). Parkin increases dopamine uptake by enhancing the cell surface expression of dopamine transporter. J. Biol. Chem. 279, 54380–54386. doi:10.1074/jbc.M409282200
Jones, K. T., Zhen, J., and Reith, M. E. A. (2012). Importance of cholesterol in dopamine transporter function. J. Neurochem. 123, 700–715. doi:10.1111/jnc.12007
Just, H., Sitte, H. H., Schmid, J. A., Freissmuth, M., and Kudlacek, O. (2004). Identification of an additional interaction domain in transmembrane domains 11 and 12 that supports oligomer formation in the human serotonin transporter *. J. Biol. Chem. 279, 6650–6657. doi:10.1074/jbc.M306092200
Karam, C. S., and Javitch, J. A. (2018). Phosphorylation of the amino terminus of the dopamine transporter: Regulatory mechanisms and implications for amphetamine action. Adv. Pharmacol. 82, 205–234. doi:10.1016/bs.apha.2017.09.002
Khelashvili, G., LeVine, M. V., Shi, L., Quick, M., Javitch, J. A., and Weinstein, H. (2013). The membrane protein LeuT in micellar systems: Aggregation dynamics and detergent binding to the S2 site. J. Am. Chem. Soc. 135, 14266–14275. doi:10.1021/ja405984v
Khoshbouei, H., Sen, N., Guptaroy, B., Johnson, L., Lund, D., Gnegy, M. E., et al. (2004). N-terminal phosphorylation of the dopamine transporter is required for amphetamine-induced efflux. PLoS Biol. 2, E78. doi:10.1371/journal.pbio.0020078E78
King, J. E., Eugenin, E. A., Buckner, C. M., and Berman, J. W. (2006). HIV tat and neurotoxicity. Microbes Infect. 8, 1347–1357. doi:10.1016/j.micinf.2005.11.014
Kisos, H., Ben-Gedalya, T., and Sharon, R. (2014). The clathrin-dependent localization of dopamine transporter to surface membranes is affected by α-synuclein. J. Mol. Neurosci. 52, 167–176. doi:10.1007/s12031-013-0118-1
Kniazeff, J., Shi, L., Loland, C. J., Javitch, J. A., Weinstein, H., and Gether, U. (2008). An intracellular interaction network regulates conformational transitions in the dopamine transporter. J. Biol. Chem. 283, 17691–17701. doi:10.1074/jbc.M800475200
Kocabas, A. M., Rudnick, G., and Kilic, F. (2003). Functional consequences of homo- but not hetero-oligomerization between transporters for the biogenic amine neurotransmitters. J. Neurochem. 85, 1513–1520. doi:10.1046/j.1471-4159.2003.01793.x
Koldsø, H., Christiansen, A. B., Sinning, S., and Schiøtt, B. (2013). Comparative modeling of the human monoamine transporters: Similarities in substrate binding. ACS Chem. Neurosci. 4, 295–309. doi:10.1021/cn300148r
Krishnamurthy, H., and Gouaux, E. (2012). X-ray structures of LeuT in substrate-free outward-open and apo inward-open states. Nature 481, 469–474. doi:10.1038/nature10737
Krout, D., Pramod, A. B., Dahal, R. A., Tomlinson, M. J., Sharma, B., Foster, J. D., et al. (2017). Inhibitor mechanisms in the S1 binding site of the dopamine transporter defined by multi-site molecular tethering of photoactive cocaine analogs. Biochem. Pharmacol. 142, 204–215. doi:10.1016/j.bcp.2017.07.015
Kumar, A. M., Ownby, R. L., Waldrop-Valverde, D., Fernandez, B., and Kumar, M. (2011). Human immunodeficiency virus infection in the CNS and decreased dopamine availability: Relationship with neuropsychological performance. J. NeuroVirology 17, 26–40. doi:10.1007/s13365-010-0003-4
Kuppuswamy, M., Subramanian, T., Srinivasan, A., and Chinnadurai, G. (1989). Multiple functional domains of Tat, the trans-activator of HIV-1, defined by mutational analysis. Nucleic Acids Res. 17, 3551–3561. doi:10.1093/nar/17.9.3551
Kurian, M. A., Zhen, J., Cheng, S.-Y., Li, Y., Mordekar, S. R., Jardine, P., et al. (2009). Homozygous loss-of-function mutations in the gene encoding the dopamine transporter are associated with infantile parkinsonism-dystonia. J. Clin. Investigation 119, 1595–1603. doi:10.1172/JCI39060
Lanzo, A., Safratowich, B. D., Kudumala, S. R., Gallotta, I., Zampi, G., Di Schiavi, E., et al. (2018). Silencing of syntaxin 1A in the dopaminergic neurons decreases the activity of the dopamine transporter and prevents amphetamine-induced behaviors in C. elegans. Front. Physiology 9, 576. doi:10.3389/fphys.2018.00576
Larsen, M. B., Sonders, M. S., Mortensen, O. V., Larson, G. A., Zahniser, N. R., and Amara, S. G. (2011). Dopamine transport by the serotonin transporter: A mechanistically distinct mode of substrate translocation. J. Neurosci. 31, 6605–6615. doi:10.1523/JNEUROSCI.0576-11.2011
Lee, F. J., Pei, L., Moszczynska, A., Vukusic, B., Fletcher, P. J., and Liu, F. (2007). Dopamine transporter cell surface localization facilitated by a direct interaction with the dopamine D2 receptor. Embo J. 26, 2127–2136. doi:10.1038/sj.emboj.7601656
Lee, F. J. S., Liu, F., Pristupa, Z. B., and Niznik, H. B. (2001). Direct binding and functional coupling of α-synuclein to the dopamine transporters accelerate dopamine-induced apoptosis. FASEB J. 15, 916–926. doi:10.1096/fj.00-0334com
Lee, K.-H., Kim, M.-Y., Kim, D.-H., and Lee, Y.-S. (2004). Syntaxin 1A and receptor for activated C kinase interact with the N-terminal region of human dopamine transporter. Neurochem. Res. 29, 1405–1409. doi:10.1023/b:nere.0000026404.08779.43
Lehmensiek, V., Tan, E. M., Liebau, S., Lenk, T., Zettlmeisl, H., Schwarz, J., et al. (2006). Dopamine transporter-mediated cytotoxicity of 6-hydroxydopamine in vitro depends on expression of mutant alpha-synucleins related to Parkinson's disease. Neurochem. Int. 48, 329–340. doi:10.1016/j.neuint.2005.11.008
Li, L. B., Chen, N., Ramamoorthy, S., Chi, L., Cui, X. N., Wang, L. C., et al. (2004). The role of N-glycosylation in function and surface trafficking of the human dopamine transporter. J. Biol. Chem. 279, 21012–21020. doi:10.1074/jbc.M311972200
Li, Y., Cheng, S.-Y., Chen, N., and Reith, M. E. A. (2010). Interrelation of dopamine transporter oligomerization and surface presence as studied with mutant transporter proteins and amphetamine. J. Neurochem. 114, 873–885. doi:10.1111/j.1471-4159.2010.06818.x
Lin, A. M., Kao, L. S., and Chai, C. Y. (1995). Involvement of nitric oxide in dopaminergic transmission in rat striatum: An in vivo electrochemical study. J. Neurochem. 65, 2043–2049. doi:10.1046/j.1471-4159.1995.65052043.x
Lin, Z., Wang, W., Kopajtic, T., Revay, R. S., and Uhl, G. R. (1999). Dopamine transporter: Transmembrane phenylalanine mutations can selectively influence dopamine uptake and cocaine analog recognition. Mol. Pharmacol. 56, 434–447. doi:10.1124/mol.56.2.434
Lin, Z., Zhang, P. W., Zhu, X., Melgari, J. M., Huff, R., Spieldoch, R. L., et al. (2003). Phosphatidylinositol 3-kinase, protein kinase C, and MEK1/2 kinase regulation of dopamine transporters (DAT) require N-terminal DAT phosphoacceptor sites. J. Biol. Chem. 278, 20162–20170. doi:10.1074/jbc.M209584200
Loland, C. J., Desai, R. I., Zou, M.-F., Cao, J., Grundt, P., Gerstbrein, K., et al. (2008). Relationship between conformational changes in the dopamine transporter and cocaine-like subjective effects of uptake inhibitors. Mol. Pharmacol. 73, 813–823. doi:10.1124/mol.107.039800
Loland, C. J., Grånäs, C., Javitch, J. A., and Gether, U. (2004). Identification of intracellular residues in the dopamine transporter critical for regulation of transporter conformation and cocaine binding *. J. Biol. Chem. 279, 3228–3238. doi:10.1074/jbc.M304755200
Loland, C. J., Mereu, M., Okunola, O. M., Cao, J., Prisinzano, T. E., Mazier, S., et al. (2012). R-Modafinil (armodafinil): A unique dopamine uptake inhibitor and potential medication for psychostimulant abuse. Biol. Psychiatry 72, 405–413. doi:10.1016/j.biopsych.2012.03.022
Loland, C. J., Norregaard, L., and Gether, U. (1999). Defining proximity relationships in the tertiary structure of the dopamine transporter: Identification of a conserved glutamic acid as a third coordinate in the endogenous Zn2+-binding site. J. Biol. Chem. 274, 36928–36934. doi:10.1074/jbc.274.52.36928
Loland, C. J., Norregaard, L., Litman, T., and Gether, U. (2002). Generation of an activating Zn2+ switch in the dopamine transporter: Mutation of an intracellular tyrosine constitutively alters the conformational equilibrium of the transport cycle. Proc. Natl. Acad. Sci. 99, 1683–1688. doi:10.1073/pnas.032386299
Lonart, G., and Johnson, K. M. (1994). Inhibitory effects of nitric oxide on the uptake of [3H]dopamine and [3H]glutamate by striatal synaptosomes. J. Neurochem. 63, 2108–2117. doi:10.1046/j.1471-4159.1994.63062108.x
Mabb, A. M., Judson, M. C., Zylka, M. J., and Philpot, B. D. (2011). Angelman syndrome: Insights into genomic imprinting and neurodevelopmental phenotypes. Trends Neurosci. 34, 293–303. doi:10.1016/j.tins.2011.04.001
MaryCheng, H., and Bahar, I. (2015). Molecular mechanism of dopamine transport by human dopamine transporter. Structure 23, 2171–2181. doi:10.1016/j.str.2015.09.001
Mazei-Robison, M. S., Bowton, E., Holy, M., Schmudermaier, M., Freissmuth, M., Sitte, H. H., et al. (2008). Anomalous dopamine release associated with a human dopamine transporter coding variant. J. Neurosci. 28, 7040–7046. doi:10.1523/JNEUROSCI.0473-08.2008
Mazei-Robison, M. S., Couch, R. S., Shelton, R. C., Stein, M. A., and Blakely, R. D. (2005). Sequence variation in the human dopamine transporter gene in children with attention deficit hyperactivity disorder. Neuropharmacology 49, 724–736. doi:10.1016/j.neuropharm.2005.08.003
McElvain, J. S., and Schenk, J. O. (1992). A multisubstrate mechanism of striatal dopamine uptake and its inhibition by cocaine. Biochem. Pharmacol. 43, 2189–2199. doi:10.1016/0006-2952(92)90178-l
Meiergerd, S. M., and Schenk, J. O. (1994a). Kinetic evaluation of the commonality between the site(s) of action of cocaine and some other structurally similar and dissimilar inhibitors of the striatal transporter for dopamine. J. Neurochem. 63, 1683–1692. doi:10.1046/j.1471-4159.1994.63051683.x
Meiergerd, S. M., and Schenk, J. O. (1994b). Striatal transporter for dopamine: Catechol structure-activity studies and susceptibility to chemical modification. J. Neurochem. 62, 998–1008. doi:10.1046/j.1471-4159.1994.62030998.x
Melikian, H. E., and Buckley, K. M. (1999). Membrane trafficking regulates the activity of the human dopamine transporter. J. Neurosci. 19, 7699–7710. doi:10.1523/jneurosci.19-18-07699.1999
Midde, N. M., Gomez, A. M., and Zhu, J. (2012). HIV-1 Tat protein decreases dopamine transporter cell surface expression and vesicular monoamine transporter-2 function in rat striatal synaptosomes. J. Neuroimmune Pharmacol. 7, 629–639. doi:10.1007/s11481-012-9369-9
Midde, N. M., Huang, X., Gomez, A. M., Booze, R. M., Zhan, C.-G., and Zhu, J. (2013). Mutation of tyrosine 470 of human dopamine transporter is critical for HIV-1 tat-induced inhibition of dopamine transport and transporter conformational transitions. J. Neuroimmune Pharmacol. 8, 975–987. doi:10.1007/s11481-013-9464-6
Mikelman, S. R., Guptaroy, B., Schmitt, K. C., Jones, K. T., Zhen, J., Reith, M. E. A., et al. (2018). Tamoxifen directly interacts with the dopamine transporter. J. Pharmacol. Exp. Ther. 367, 119–128. doi:10.1124/jpet.118.248179
Milner, H. E., Béliveau, R., and Jarvis, S. M. (1994). The in situ size of the dopamine transporter is a tetramer as estimated by radiation inactivation. Biochimica Biophysica Acta (BBA) - Biomembr. 1190, 185–187. doi:10.1016/0005-2736(94)90051-5
Miranda, M., Dionne, K. R., Sorkina, T., and Sorkin, A. (2007). Three ubiquitin conjugation sites in the amino terminus of the dopamine transporter mediate protein kinase C-dependent endocytosis of the transporter. Mol. Biol. Cell 18, 313–323. doi:10.1091/mbc.e06-08-0704
Miranda, M., Wu, C. C., Sorkina, T., Korstjens, D. R., and Sorkin, A. (2005). Enhanced ubiquitylation and accelerated degradation of the dopamine transporter mediated by protein kinase C. J. Biol. Chem. 280, 35617–35624. doi:10.1074/jbc.M506618200
Moerke, M. J., Ananthan, S., Banks, M. L., Eltit, J. M., Freitas, K. C., Johnson, A. R., et al. (2018). Interactions between cocaine and the putative allosteric dopamine transporter ligand SRI-31142. J. Pharmacol. Exp. Ther. 367, 222–233. doi:10.1124/jpet.118.250902
Moritz, A. E., Rastedt, D. E., Stanislowski, D. J., Shetty, M., Smith, M. A., Vaughan, R. A., et al. (2015). Reciprocal phosphorylation and palmitoylation control dopamine transporter kinetics. J. Biol. Chem. 290, 29095–29105. doi:10.1074/jbc.M115.667055
Mortensen, O. V., and Kortagere, S. (2015). Designing modulators of monoamine transporters using virtual screening techniques. Front. Pharmacol. 6, 223. doi:10.3389/fphar.2015.00223
Moussa, C. E. H., Wersinger, C., Tomita, Y., and Sidhu, A. (2004). Differential cytotoxicity of human wild type and mutant α-synuclein in human neuroblastoma SH-SY5Y cells in the presence of dopamine. Biochemistry 43, 5539–5550. doi:10.1021/bi036114f
Navratna, V., Tosh, D. K., Jacobson, K. A., and Gouaux, E. (2018). Thermostabilization and purification of the human dopamine transporter (hDAT) in an inhibitor and allosteric ligand bound conformation. PLOS ONE 13, e0200085. doi:10.1371/journal.pone.0200085e0200085
Nepal, B., Leveritt, J., and Lazaridis, T. (2018). Membrane curvature sensing by amphipathic helices: Insights from implicit membrane modeling. Biophysical J. 114, 2128–2141. doi:10.1016/j.bpj.2018.03.030
Newman, A. H., Cao, J., Keighron, J. D., Jordan, C. J., Bi, G.-H., Liang, Y., et al. (2019). Translating the atypical dopamine uptake inhibitor hypothesis toward therapeutics for treatment of psychostimulant use disorders. Neuropsychopharmacology 44, 1435–1444. doi:10.1038/s41386-019-0366-z
Nielsen, A. K., Möller, I. R., Wang, Y., Rasmussen, S. G. F., Lindorff-Larsen, K., Rand, K. D., et al. (2019). Substrate-induced conformational dynamics of the dopamine transporter. Nat. Commun. 10, 2714. doi:10.1038/s41467-019-10449-w
Nikiforuk, A., Kalaba, P., Ilic, M., Korz, V., Dragačević, V., Wackerlig, J., et al. (2017). A novel dopamine transporter inhibitor CE-123 improves cognitive flexibility and maintains impulsivity in healthy male rats. Front. Behav. Neurosci. 11, 222. doi:10.3389/fnbeh.2017.00222
Nirenberg, M. J., Chan, J., Liu, Y., Edwards, R. H., and Pickel, V. M. (1997a). Vesicular monoamine transporter-2: Immunogold localization in striatal axons and terminals. Synapse 26, 194–198. doi:10.1002/(SICI)1098-2396(199706)26:2<194:AID-SYN10>3.0.CO;2-Y
Nirenberg, M. J., Chan, J., Vaughan, R. A., Uhl, G. R., Kuhar, M. J., and Pickel, V. M. (1997b). Immunogold localization of the dopamine transporter: An ultrastructural study of the rat ventral tegmental area. J. Neurosci. 17, 5255–5262. doi:10.1523/JNEUROSCI.17-14-05255.1997
Nyola, A., Karpowich, N. K., Zhen, J., Marden, J., Reith, M. E., and Wang, D.-N. (2010). Substrate and drug binding sites in LeuT. Curr. Opin. Struct. Biol. 20, 415–422. doi:10.1016/j.sbi.2010.05.007
Oaks, A. W., Frankfurt, M., Finkelstein, D. I., and Sidhu, A. (2013). Age-dependent effects of A53T alpha-synuclein on behavior and dopaminergic function. PLOS ONE 8, e60378. doi:10.1371/journal.pone.0060378
Ortore, G., Orlandini, E., Betti, L., Giannaccini, G., Mazzoni, M. R., Camodeca, C., et al. (2020). Focus on human monoamine transporter selectivity. New human DAT and NET models, experimental validation, and SERT affinity exploration. ACS Chem. Neurosci. 11, 3214–3232. doi:10.1021/acschemneuro.0c00304
Page, G., Barrier, L., Morel, P., Schulzberg, M., Piriou, A., and Huguet, F. (1998). Possible relationship between changes in [3H]DA uptake and autoxidation in rat striatal slices. Exp. Neurol. 152, 88–94. doi:10.1006/exnr.1998.6816
Park, S. U., Ferrer, J. V., Javitch, J. A., and Kuhn, D. M. (2002). Peroxynitrite inactivates the human dopamine transporter by modification of cysteine 342: Potential mechanism of neurotoxicity in dopamine neurons. J. Neurosci. 22, 4399–4405. doi:10.1523/JNEUROSCI.22-11-04399.2002
Patel, A. P., Cerruti, C., Vaughan, R. A., and Kuhar, M. J. (1994). Developmentally regulated glycosylation of dopamine transporter. Brain Res. Dev. Brain Res. 83, 53–58. doi:10.1016/0165-3806(94)90178-3
Péloponèse, J. M., Grégoire, C., Opi, S., Esquieu, D., Sturgis, J., Lebrun, E., et al. (2000). 1H-13C nuclear magnetic resonance assignment and structural characterization of HIV-1 Tat protein. C R. Acad. Sci. III 323, 883–894. doi:10.1016/s0764-4469(00)01228-2
Penmatsa, A., and Gouaux, E. (2014). How LeuT shapes our understanding of the mechanisms of sodium-coupled neurotransmitter transporters. J. Physiol. 592, 863–869. doi:10.1113/jphysiol.2013.259051
Penmatsa, A., Wang, K. H., and Gouaux, E. (2013). X-ray structure of dopamine transporter elucidates antidepressant mechanism. Nature 503, 85–90. doi:10.1038/nature12533
Penmatsa, A., Wang, K. H., and Gouaux, E. (2015). X-ray structures of Drosophila dopamine transporter in complex with nisoxetine and reboxetine. Nat. Struct. Mol. Biol. 22, 506–508. doi:10.1038/nsmb.3029
Petrucelli, L., and Dickson, D. W. (2008). “P50-AG25711, P50-AG16574, P01-AG17216, and P01-AG03949,”Chapter 3-neuropathology of Parkinson's Disease11Supported by NIH grants P50-ns40256, in Parkinson's disease. Editors R. Nass, and S. Przedborski (San Diego: Academic Press), 35–48.
Pogun, S., Baumann, M. H., and Kuhar, M. J. (1994). Nitric oxide inhibits [3H]dopamine uptake. Brain Res. 641, 83–91. doi:10.1016/0006-8993(94)91818-x
Pörzgen, P., Park, S. K., Hirsh, J., Sonders, M. S., and Amara, S. G. (2001). The antidepressant-sensitive dopamine transporter in Drosophila melanogaster: A primordial carrier for catecholamines. Mol. Pharmacol. 59, 83–95. doi:10.1124/mol.59.1.83
Pramod, A. B., Foster, J., Carvelli, L., and Henry, L. K. (2013). SLC6 transporters: Structure, function, regulation, disease association and therapeutics. Mol. Asp. Med. 34, 197–219. doi:10.1016/j.mam.2012.07.002
Purohit, V., Rapaka, R., and Shurtleff, D. (2011). Drugs of abuse, dopamine, and HIV-associated neurocognitive disorders/HIV-associated dementia. Mol. Neurobiol. 44, 102–110. doi:10.1007/s12035-011-8195-z
Quick, M. W. (2002). Role of syntaxin 1A on serotonin transporter expression in developing thalamocortical neurons. Int. J. Dev. Neurosci. 20, 219–224. doi:10.1016/s0736-5748(02)00021-7
Quizon, P. M., Yuan, Y., Zhu, Y., Zhou, Y., Strauss, M. J., Sun, W. L., et al. (2021). Mutations of human DopamineTransporter at Tyrosine88, aspartic Acid206, and Histidine547 influence basal and HIV-1 tat-inhibited dopamine transport. J. Neuroimmune Pharmacol. 16, 854–869. doi:10.1007/s11481-021-09984-5
Rastedt, D. E., Vaughan, R. A., and Foster, J. D. (2017). Palmitoylation mechanisms in dopamine transporter regulation. J. Chem. Neuroanat. 83, 3–9. doi:10.1016/j.jchemneu.2017.01.002
Reith, M. E. A., Blough, B. E., Hong, W. C., Jones, K. T., Schmitt, K. C., Baumann, M. H., et al. (2015). Behavioral, biological, and chemical perspectives on atypical agents targeting the dopamine transporter. Drug Alcohol Dependence 147, 1–19. doi:10.1016/j.drugalcdep.2014.12.005
Reith, M. E. A., Jones, K. T., Zhen, J., and Topiol, S. (2018). Latch and trigger role for R445 in DAT transport explains molecular basis of DTDS. Bioorg. Med. Chem. Lett. 28, 470–475. doi:10.1016/j.bmcl.2017.12.016
Reith, M. E. A., Kortagere, S., Wiers, C. E., Sun, H., Kurian, M. A., Galli, A., et al. (2022). The dopamine transporter gene SLC6A3: Multidisease risks. Mol. Psychiatry 27, 1031–1046. doi:10.1038/s41380-021-01341-5
Reith, M. E., Berfield, J. L., Wang, L. C., Ferrer, J. V., and Javitch, J. A. (2001). The uptake inhibitors cocaine and benztropine differentially alter the conformation of the human dopamine transporter. J. Biol. Chem. 276, 29012–29018. doi:10.1074/jbc.M011785200
Reith, M. E., and Selmeci, G. (1992). Radiolabeling of dopamine uptake sites in mouse striatum: Comparison of binding sites for cocaine, mazindol, and GBR 12935. Naunyn Schmiedeb. Arch. Pharmacol. 345, 309–318. doi:10.1007/BF00168692
Rickhag, M., Owens, W. A., Winkler, M.-T., Strandfelt, K. N., Rathje, M., Sørensen, G., et al. (2013). Membrane-permeable C-terminal dopamine transporter peptides attenuate amphetamine-evoked dopamine release*, ♦. J. Biol. Chem. 288, 27534–27544. doi:10.1074/jbc.M112.441295
Rothman, R. B., Partilla, J. S., Baumann, M. H., Lightfoot-Siordia, C., and Blough, B. E. (2012). Studies of the biogenic amine transporters. 14. Identification of low-efficacy “partial” substrates for the biogenic amine transporters. J. Pharmacol. Exp. Ther. 341, 251–262. doi:10.1124/jpet.111.188946
Sakrikar, D., Mazei-Robison, M. S., Mergy, M. A., Richtand, N. W., Han, Q., Hamilton, P. J., et al. (2012). Attention deficit/hyperactivity disorder-derived coding variation in the dopamine transporter disrupts microdomain targeting and trafficking regulation. J. Neurosci. 32, 5385–5397. doi:10.1523/JNEUROSCI.6033-11.2012
Samson, K. R., Xu, W., Kortagere, S., and Espana, R. A. (2022). Intermittent access to oxycodone decreases dopamine uptake in the nucleus accumbens core during abstinence. Addict. Biol. 27, e13241. doi:10.1111/adb.13241e13241
Santra, S., Gogoi, S., Gopishetty, B., Antonio, T., Zhen, J., Reith, M. E. A., et al. (2012). Structural exploration of (3S,6S)-6-Benzhydryl-N-benzyltetrahydro-2H-pyran-3-amine analogues: Identification of potent triple monoamine reuptake inhibitors as potential antidepressants. ChemMedChem 7, 2093–2100. doi:10.1002/cmdc.201200352
Santra, S., Kortagere, S., Vedachalam, S., Gogoi, S., Antonio, T., Reith, M. E. A., et al. (2021). Novel potent dopamine–norepinephrine and triple reuptake uptake inhibitors based on asymmetric pyran template and their molecular interactions with monoamine transporters. ACS Chem. Neurosci. 12, 1406–1418. doi:10.1021/acschemneuro.1c00078
Saunders, C., Ferrer, J. V., Shi, L., Chen, J., Merrill, G., Lamb, M. E., et al. (2000). Amphetamine-induced loss of human dopamine transporter activity: An internalization-dependent and cocaine-sensitive mechanism. Proc. Natl. Acad. Sci. 97, 6850–6855. doi:10.1073/pnas.110035297
Schmitt, K. C., Mamidyala, S., Biswas, S., Dutta, A. K., and Reith, M. E. A. (2010). Bivalent phenethylamines as novel dopamine transporter inhibitors: Evidence for multiple substrate-binding sites in a single transporter. J. Neurochem. 112, 1605–1618. doi:10.1111/j.1471-4159.2010.06583.x
Schmitt, K. C., and Reith, M. E. A. (2011). The atypical stimulant and nootropic modafinil interacts with the dopamine transporter in a different manner than classical cocaine-like inhibitors. PLOS ONE 6, e25790. doi:10.1371/journal.pone.0025790e25790
Schmitt, K. C., and Reith, M. E. (2010). Regulation of the dopamine transporter: Aspects relevant to psychostimulant drugs of abuse. Ann. N. Y. Acad. Sci. 1187, 316–340. doi:10.1111/j.1749-6632.2009.05148.x
Schmitt, K. C., Rothman, R. B., and Reith, M. E. A. (2013). Nonclassical pharmacology of the dopamine transporter: Atypical inhibitors, allosteric modulators, and partial substrates. J. Pharmacol. Exp. Ther. 346, 2–10. doi:10.1124/jpet.111.191056
Seidel, S., Singer, E. A., Just, H., Farhan, H., Scholze, P., Kudlacek, O., et al. (2005). Amphetamines take two to tango: An oligomer-based counter-transport model of neurotransmitter transport explores the amphetamine action. Mol. Pharmacol. 67, 140–151. doi:10.1124/mol.67.1
Sen, N., Shi, L., Beuming, T., Weinstein, H., and Javitch, J. A. (2005). A pincer-like configuration of TM2 in the human dopamine transporter is responsible for indirect effects on cocaine binding. Neuropharmacology 49, 780–790. doi:10.1016/j.neuropharm.2005.08.014
Senior, S. L., Ninkina, N., Deacon, R., Bannerman, D., Buchman, V. L., Cragg, S. J., et al. (2008). Increased striatal dopamine release and hyperdopaminergic-like behaviour in mice lacking both alpha-synuclein and gamma-synuclein. Eur. J. Neurosci. 27, 947–957. doi:10.1111/j.1460-9568.2008.06055.x
Shan, J., Javitch, J. A., Shi, L., and Weinstein, H. (2011). The substrate-driven transition to an inward-facing conformation in the functional mechanism of the dopamine transporter. PLOS ONE 6, e16350. doi:10.1371/journal.pone.0016350e16350
Shi, L., Quick, M., Zhao, Y., Weinstein, H., and Javitch, J. A. (2008). The mechanism of a neurotransmitter:sodium symporter—inward release of Na+ and substrate is triggered by substrate in a second binding site. Mol. Cell 30, 667–677. doi:10.1016/j.molcel.2008.05.008
Siciliano, C. A., Saha, K., Calipari, E. S., Fordahl, S. C., Chen, R., Khoshbouei, H., et al. (2018). Amphetamine reverses escalated cocaine intake via restoration of dopamine transporter conformation. J. Neurosci. 38, 484–497. doi:10.1523/JNEUROSCI.2604-17.2017
Singh, S. K., Yamashita, A., and Gouaux, E. (2007). Antidepressant binding site in a bacterial homologue of neurotransmitter transporters. Nature 448, 952–956. doi:10.1038/nature06038
Sora, I., Hall, F. S., Andrews, A. M., Itokawa, M., Li, X. F., Wei, H. B., et al. (2001). Molecular mechanisms of cocaine reward: Combined dopamine and serotonin transporter knockouts eliminate cocaine place preference. Proc. Natl. Acad. Sci. U. S. A. 98, 5300–5305. doi:10.1073/pnas.091039298
Sora, I., Wichems, C., Takahashi, N., Li, X.-F., Zeng, Z., Revay, R., et al. (1998). Cocaine reward models: Conditioned place preference can be established in dopamine- and in serotonin-transporter knockout mice. Proc. Natl. Acad. Sci. 95, 7699–7704. doi:10.1073/pnas.95.13.7699
Sorkina, T., Caltagarone, J., and Sorkin, A. (2013). Flotillins regulate membrane mobility of the dopamine transporter but are not required for its protein kinase C dependent endocytosis. Traffic 14, 709–724. doi:10.1111/tra.12059
Sorkina, T., Cheng, M. H., Bagalkot, T. R., Wallace, C., Watkins, S. C., Bahar, I., et al. (2021). Direct coupling of oligomerization and oligomerization-driven endocytosis of the dopamine transporter to its conformational mechanics and activity. J. Biol. Chem. 296, 100430. doi:10.1016/j.jbc.2021.100430
Sorkina, T., Doolen, S., Galperin, E., Zahniser, N. R., and Sorkin, A. (2003). Oligomerization of dopamine transporters visualized in living cells by fluorescence resonance energy transfer microscopy. J. Biol. Chem. 278, 28274–28283. doi:10.1074/jbc.M210652200
Sorkina, T., Hoover, B. R., Zahniser, N. R., and Sorkin, A. (2005). Constitutive and protein kinase C-induced internalization of the dopamine transporter is mediated by a clathrin-dependent mechanism. Traffic 6, 157–170. doi:10.1111/j.1600-0854.2005.00259.x
Sorkina, T., Ma, S., Larsen, M. B., Watkins, S. C., and Sorkin, A. (2018). Small molecule induced oligomerization, clustering and clathrin-independent endocytosis of the dopamine transporter. eLife 7, e32293. doi:10.7554/eLife.32293
Sorkina, T., Miranda, M., Dionne, K. R., Hoover, B. R., Zahniser, N. R., and Sorkin, A. (2006). RNA interference screen reveals an essential role of Nedd4-2 in dopamine transporter ubiquitination and endocytosis. J. Neurosci. 26, 8195–8205. doi:10.1523/JNEUROSCI.1301-06.2006
Spillantini, M. G., Crowther, R. A., Jakes, R., Hasegawa, M., and Goedert, M. (1998). alpha-Synuclein in filamentous inclusions of Lewy bodies from Parkinson's disease and dementia with lewy bodies. Proc. Natl. Acad. Sci. 95, 6469–6473. doi:10.1073/pnas.95.11.6469
Staub, O., and Rotin, D. (2006). Role of ubiquitylation in cellular membrane transport. Physiol. Rev. 86, 669–707. doi:10.1152/physrev.00020.2005
Steele, T. W. E., Spires, Z., Jones, C. B., Glennon, R. A., Dukat, M., and Eltit, J. M. (2021). Non-conserved residues dictate dopamine transporter selectivity for the potent synthetic cathinone and psychostimulant MDPV. Neuropharmacology 200, 108820. doi:10.1016/j.neuropharm.2021.108820
Steinkellner, T., Yang, J. W., Montgomery, T. R., Chen, W. Q., Winkler, M. T., Sucic, S., et al. (2012). Ca(2+)/calmodulin-dependent protein kinase IIα (αCaMKII) controls the activity of the dopamine transporter: Implications for angelman syndrome. J. Biol. Chem. 287, 29627–29635. doi:10.1074/jbc.M112.367219
Stockner, T., Montgomery, T. R., Kudlacek, O., Weissensteiner, R., Ecker, G. F., Freissmuth, M., et al. (2013). Mutational analysis of the high-affinity zinc binding site validates a refined human dopamine transporter homology model. PLOS Comput. Biol. 9, e1002909. doi:10.1371/journal.pcbi.1002909e1002909
Sun, W.-L., Quizon, P. M., Yuan, Y., Strauss, M. J., McCain, R., Zhan, C.-G., et al. (2019). Mutational effects of human dopamine transporter at tyrosine88, lysine92, and histidine547 on basal and HIV-1 Tat-inhibited dopamine transport. Sci. Rep. 9, 3843. doi:10.1038/s41598-019-39872-1
Sun, W. L., Quizon, P. M., Yuan, Y., Zhang, W., Ananthan, S., Zhan, C. G., et al. (2017). Allosteric modulatory effects of SRI-20041 and SRI-30827 on cocaine and HIV-1 Tat protein binding to human dopamine transporter. Sci. Rep. 7, 3694. doi:10.1038/s41598-017-03771-0
Sundaresan, M., Yu, Z. X., Ferrans, V. J., Irani, K., and Finkel, T. (1995). Requirement for generation of H2O2 for platelet-derived growth factor signal transduction. Science 270, 296–299. doi:10.1126/science.270.5234.296
Sung, U., Apparsundaram, S., Galli, A., Kahlig, K. M., Savchenko, V., Schroeter, S., et al. (2003). A regulated interaction of syntaxin 1A with the antidepressant-sensitive norepinephrine transporter establishes catecholamine clearance capacity. J. Neurosci. 23, 1697–1709. doi:10.1523/JNEUROSCI.23-05-01697.2003
Swant, J., Goodwin, J. S., North, A., Ali, A. A., Gamble-George, J., Chirwa, S., et al. (2011). α-Synuclein stimulates a dopamine transporter-dependent chloride current and modulates the activity of the transporter *. J. Biol. Chem. 286, 43933–43943. doi:10.1074/jbc.M111.241232
Sweeney, C. G., Tremblay, B. P., Stockner, T., Sitte, H. H., and Melikian, H. E. (2017). Dopamine transporter amino and carboxyl termini synergistically contribute to substrate and inhibitor affinities. J. Biol. Chem. 292, 1302–1309. doi:10.1074/jbc.M116.762872
Tatsumi, M., Groshan, K., Blakely, R. D., and Richelson, E. (1997). Pharmacological profile of antidepressants and related compounds at human monoamine transporters. Eur. J. Pharmacol. 340, 249–258. doi:10.1016/s0014-2999(97)01393-9
Torres, G. E., Carneiro, A., Seamans, K., Fiorentini, C., Sweeney, A., Yao, W. D., et al. (2003). Oligomerization and trafficking of the human dopamine transporter. Mutational analysis identifies critical domains important for the functional expression of the transporter. J. Biol. Chem. 278, 2731–2739. doi:10.1074/jbc.M201926200
Torres, G. E., Yao, W.-D., Mohn, A. R., Quan, H., Kim, K.-M., Levey, A. I., et al. (2001). Functional interaction between monoamine plasma membrane transporters and the synaptic PDZ domain–containing protein PICK1. Neuron 30, 121–134. doi:10.1016/s0896-6273(01)00267-7
Ulmer, T. S., Bax, A., Cole, N. B., and Nussbaum, R. L. (2005). Structure and dynamics of micelle-bound human α-synuclein*. J. Biol. Chem. 280, 9595–9603. doi:10.1074/jbc.M411805200
Vaughan, R. A., and Foster, J. D. (2013). Mechanisms of dopamine transporter regulation in normal and disease states. Trends Pharmacol. Sci. 34, 489–496. doi:10.1016/j.tips.2013.07.005
Vaughan, R. A., Huff, R. A., Uhl, G. R., and Kuhar, M. J. (1997). Protein kinase C-mediated phosphorylation and functional regulation of dopamine transporters in striatal synaptosomes. J. Biol. Chem. 272, 15541–15546. doi:10.1074/jbc.272.24.15541
Vaughan, R. A., and Kuhar, M. J. (1996). Dopamine transporter ligand binding domains. Structural and functional properties revealed by limited proteolysis. J. Biol. Chem. 271, 21672–21680. doi:10.1074/jbc.271.35.21672
Vina-Vilaseca, A., Bender-Sigel, J., Sorkina, T., Closs, E. I., and Sorkin, A. (2011). Protein kinase C-dependent ubiquitination and clathrin-mediated endocytosis of the cationic amino acid transporter CAT-1. J. Biol. Chem. 286, 8697–8706. doi:10.1074/jbc.M110.186858
Volz, T. J., and Schenk, J. O. (2004). L-arginine increases dopamine transporter activity in rat striatum via a nitric oxide synthase-dependent mechanism. Synapse 54, 173–182. doi:10.1002/syn.20075
Wang, C., Zhao, C., Li, D., Tian, Z., Lai, Y., Diao, J., et al. (2016). Versatile structures of α-synuclein. Front. Mol. Neurosci. 9, 48. doi:10.3389/fnmol.2016.00048
Wang, Q., Bubula, N., Brown, J., Wang, Y., Kondev, V., and Vezina, P. (2016). PKC phosphorylates residues in the N-terminal of the DA transporter to regulate amphetamine-induced DA efflux. Neurosci. Lett. 622, 78–82. doi:10.1016/j.neulet.2016.04.051
Wang, K. H., Penmatsa, A., and Gouaux, E. (2015). Neurotransmitter and psychostimulant recognition by the dopamine transporter. Nature 521, 322–327. doi:10.1038/nature14431
Wayment, H. K., Deutsch, H., Schweri, M. M., and Schenk, J. O. (1999). Effects of methylphenidate analogues on phenethylamine substrates for the striatal dopamine transporter: Potential as amphetamine antagonists? J. Neurochem. 72, 1266–1274. doi:10.1046/j.1471-4159.1999.0721266.x
Wayment, H., Meiergerd, S. M., and Schenk, J. O. (1998). Relationships between the catechol substrate binding site and amphetamine, cocaine, and mazindol binding sites in a kinetic model of the striatal transporter of dopamine in vitro. J. Neurochem. 70, 1941–1949. doi:10.1046/j.1471-4159.1998.70051941.x
Wersinger, C., Prou, D., Vernier, P., Niznik, H. B., and Sidhu, A. (2003a). Mutations in the lipid-binding domain of α-synuclein confer overlapping, yet distinct, functional properties in the regulation of dopamine transporter activity. Mol. Cell. Neurosci. 24, 91–105. doi:10.1016/s1044-7431(03)00124-6
Wersinger, C., Prou, D., Vernier, P., and Sidhu, A. (2003b). Modulation of dopamine transporter function by alpha-synuclein is altered by impairment of cell adhesion and by induction of oxidative stress. Faseb J. 17, 2151–2153. doi:10.1096/fj.03-0152fje
Wersinger, C., and Sidhu, A. (2003). Attenuation of dopamine transporter activity by alpha-synuclein. Neurosci. Lett. 340, 189–192. doi:10.1016/s0304-3940(03)00097-1
Wersinger, C., Vernier, P., and Sidhu, A. (2004). Trypsin disrupts the trafficking of the human dopamine transporter by alpha-synuclein and its A30P mutant. Biochemistry 43, 1242–1253. doi:10.1021/bi035308s
Huang, X., Gu, H. H., and Zhan, C.-G., Mechanism for cocaine blocking the transport of dopamine: Insights from molecular modeling and dynamics simulations. The journal of physical chemistry B 113 (2009) 15057–15066.
Xu, L., and Chen, L. Y. (2022). Effects of the N-terminal dynamics on the conformational states of human dopamine transporter. Biophys. Chem. 283, 106765. doi:10.1016/j.bpc.2022.106765
Xu, L., and Chen, L. Y. (2020). Identification of a new allosteric binding site for cocaine in dopamine transporter. J. Chem. Inf. Model. 60, 3958–3968. doi:10.1021/acs.jcim.0c00346
Yamashita, A., Singh, S. K., Kawate, T., Jin, Y., and Gouaux, E. (2005). Crystal structure of a bacterial homologue of Na+/Cl–dependent neurotransmitter transporters. Nature 437, 215–223. doi:10.1038/nature03978
Yang, J. W., Larson, G., Konrad, L., Shetty, M., Holy, M., Jantsch, K., et al. (2019). Dephosphorylation of human dopamine transporter at threonine 48 by protein phosphatase PP1/2A up-regulates transport velocity. J. Biol. Chem. 294, 3419–3431. doi:10.1074/jbc.RA118.005251
Yokoi, N., Fukata, Y., Sekiya, A., Murakami, T., Kobayashi, K., and Fukata, M. (2016). Identification of PSD-95 depalmitoylating enzymes. J. Neurosci. 36, 6431–6444. doi:10.1523/JNEUROSCI.0419-16.2016
Yuan, Y., Huang, X., Midde, N. M., Quizon, P. M., Sun, W.-L., Zhu, J., et al. (2015). Molecular mechanism of HIV-1 tat interacting with human dopamine transporter. ACS Chem. Neurosci. 6, 658–665. doi:10.1021/acschemneuro.5b00001
Zahniser, N. R., and Doolen, S. (2001). Chronic and acute regulation of Na+/Cl- -dependent neurotransmitter transporters: Drugs, substrates, presynaptic receptors, and signaling systems. Pharmacol. Ther. 92, 21–55. doi:10.1016/s0163-7258(01)00158-9
Zeppelin, T., Ladefoged, L. K., Sinning, S., Periole, X., and Schiøtt, B. (2018). A direct interaction of cholesterol with the dopamine transporter prevents its out-to-inward transition. PLOS Comput. Biol. 14, e1005907. doi:10.1371/journal.pcbi.1005907
Zeppelin, T., Pedersen, K. B., Berglund, N. A., Periole, X., and Schiott, B. (2021). Effect of palmitoylation on the dimer formation of the human dopamine transporter. Sci. Rep. 11, 4164. doi:10.1038/s41598-021-83374-y
Zhen, J., Antonio, T., Cheng, S.-Y., Ali, S., Jones, K. T., and Reith, M. E. A. (2015). Dopamine transporter oligomerization: Impact of combining protomers with differential cocaine analog binding affinities. J. Neurochem. 133, 167–173. doi:10.1111/jnc.13025
Zhen, J., and Reith, M. E. A. (2018). Functional properties of dopamine transporter oligomers after copper linking. J. Neurochem. 144, 162–171. doi:10.1111/jnc.14259
Zhou, Z., Zhen, J., Karpowich, N. K., Goetz, R. M., Law, C. J., Reith, M. E., et al. (2007). LeuT-desipramine structure reveals how antidepressants block neurotransmitter reuptake. Science 317, 1390–1393. doi:10.1126/science.1147614
Zhu, J., Ananthan, S., Mactutus, C. F., and Booze, R. M. (2011). Recombinant human immunodeficiency virus-1 transactivator of transcription1–86 allosterically modulates dopamine transporter activity. Synapse 65, 1251–1254. doi:10.1002/syn.20949
Zhu, J., Ananthan, S., and Zhan, C.-G. (2018). The role of human dopamine transporter in NeuroAIDS. Pharmacol. Ther. 183, 78–89. doi:10.1016/j.pharmthera.2017.10.007
Zhu, J., Mactutus, C. F., Wallace, D. R., and Booze, R. M. (2009). HIV-1 tat protein-induced rapid and reversible decrease in [3H]dopamine uptake: Dissociation of [3H]dopamine uptake and [3H]2β-Carbomethoxy-3-β-(4-fluorophenyl)tropane (WIN 35,428) binding in rat striatal synaptosomes. J. Pharmacol. Exp. Ther. 329, 1071–1083. doi:10.1124/jpet.108.150144
Zhu, S. J., Kavanaugh, M. P., Sonders, M. S., Amara, S. G., and Zahniser, N. R. (1997). Activation of protein kinase C inhibits uptake, currents and binding associated with the human dopamine transporter expressed in Xenopus oocytes. J. Pharmacol. Exp. Ther. 282, 1358–1365.
Zou, M.-F., Cao, J., Abramyan, A. M., Kopajtic, T., Zanettini, C., Guthrie, D. A., et al. (2017). Structure–activity relationship studies on a series of 3α-[Bis(4-fluorophenyl)methoxy]tropanes and 3α-[Bis(4-fluorophenyl)methylamino]tropanes as novel atypical dopamine transporter (DAT) inhibitors for the treatment of cocaine use disorders. J. Med. Chem. 60, 10172–10187. doi:10.1021/acs.jmedchem.7b01454
Keywords: α-synuclein, allosteric modulators, dopamine transporter, inward-open, oligomerization, outward-open, post translational modifications, tat
Citation: Nepal B, Das S, Reith ME and Kortagere S (2023) Overview of the structure and function of the dopamine transporter and its protein interactions. Front. Physiol. 14:1150355. doi: 10.3389/fphys.2023.1150355
Received: 24 January 2023; Accepted: 21 February 2023;
Published: 03 March 2023.
Edited by:
Tiziano Verri, University of Salento, ItalyReviewed by:
Sonja Sucic, Medical University of Vienna, AustriaPeter Hamilton, Virginia Commonwealth University, United States
Lucia Carvelli, Florida Atlantic University, United States
Copyright © 2023 Nepal, Das, Reith and Kortagere. This is an open-access article distributed under the terms of the Creative Commons Attribution License (CC BY). The use, distribution or reproduction in other forums is permitted, provided the original author(s) and the copyright owner(s) are credited and that the original publication in this journal is cited, in accordance with accepted academic practice. No use, distribution or reproduction is permitted which does not comply with these terms.
*Correspondence: Sandhya Kortagere, c2s2NzNAZHJleGVsLmVkdQ==