- 1Institute of Cardiovascular Sciences, College of Medical and Dental Sciences, University of Birmingham, Birmingham, United Kingdom
- 2Cardiovascular Medicine, Radcliffe Department of Medicine and British Heart Foundation Centre of Research Excellence Oxford, University of Oxford, Oxford, United Kingdom
The sarcomere is the smallest functional unit of muscle contraction. It is delineated by a protein-rich structure known as the Z-disk, alternating with M-bands. The Z-disk anchors the actin-rich thin filaments and plays a crucial role in maintaining the mechanical stability of the cardiac muscle. A multitude of proteins interact with each other at the Z-disk and they regulate the mechanical properties of the thin filaments. Over the past 2 decades, the role of the Z-disk in cardiac muscle contraction has been assessed widely, however, the impact of genetic variants in Z-disk proteins has still not been fully elucidated. This review discusses the various Z-disk proteins (alpha-actinin, filamin C, titin, muscle LIM protein, telethonin, myopalladin, nebulette, and nexilin) and Z-disk-associated proteins (desmin, and obscurin) and their role in cardiac structural stability and intracellular signaling. This review further explores how genetic variants of Z-disk proteins are linked to inherited cardiac conditions termed cardiomyopathies.
1 Introduction
Inherited cardiomyopathies are a group of genetic diseases affecting the heart muscle, which are caused by pathogenic variants in different cardiac proteins. They are a major cause of mortality and morbidity worldwide (Sabater-Molina et al., 2018). Most cardiomyopathies are inherited in an autosomal-dominant pattern (Ehsan et al., 2017) and are classified based on the dominant functional or morphological changes in the myocardium. The different types include hypertrophic cardiomyopathy (HCM), dilated cardiomyopathy (DCM), arrhythmogenic cardiomyopathy (ACM), restrictive cardiomyopathy (RCM) and left ventricular non-compaction (LVNC) (Ehsan et al., 2017).
HCM is one of the most common cardiomyopathies with a prevalence of 1:500, and is often associated with sudden cardiac death (Mendes de Almeida et al., 2017). This disease is characterized by left ventricular hypertrophy and a non-dilated left ventricle with diastolic dysfunction, but with preserved or increased ejection fraction (Marian and Braunwald, 2017). At the cellular level, cardiomyocyte disarray, cellular hypertrophy, and interstitial fibrosis are hallmarks of the disease (Marian and Braunwald, 2017).
DCM is characterized by increased left ventricular chamber size and systolic dysfunction leading to progressive heart failure (Begay et al., 2016). It is known as one of the leading causes of cardiovascular mortality and heart failure, and patients with this disease often require heart transplantation (Zhao et al., 2015). Estimates of prevalence vary between 1:250 and 1:2500 (Rakar et al., 1997; Hershberger et al., 2013).
ACM is pathologically characterized by fibrofatty myocardial replacement and clinically by ventricular electrical instability, which predisposes patients to life-threatening ventricular arrhythmias and sudden cardiac death (Corrado and Zorzi, 2018). It is prevalence is reported to be 1:2000 (Groeneweg et al., 2014).
RCM is a rare, fatal myocardial disease caused by diastolic dysfunction, i.e., increased myocardial stiffness and impaired ventricular filling, with usually preserved ventricular dimensions (Muchtar et al., 2017).
LVNC can occur in isolation or as part of other cardiomyopathies. It is an abnormally prominent trabeculation of the left ventricle, usually diagnosed by imaging (echocardiography or cardiac magnet resonance imaging) (Srivastava et al., 2022).
Inherited cardiomyopathies result in structural and contractile alterations in the cardiac muscle. Structurally, myocardial filaments are composed of sarcomeres, the basic contractile units. At the boundaries of the sarcomeres, a multiprotein complex termed the Z-disk tethers actin-rich thin filaments. The Z-disk plays an integral role in the structure and function of striated muscle; it provides, together with the M-band tethering thick filaments, the structural integrity of the sarcomeres. Moreover, the Z-disk also functions as a signaling hub by converting biomechanical stress into biochemical signals that are important for adaptation to mechanical stress (Frank and Frey, 2011; Filomena et al., 2021). Furthermore, there are numerous proteins that interact with each other at the Z-disk (see Figure 1). The Z-disk proteins also connect the contractile apparatus to the cytoskeleton and the extracellular matrix (Clark et al., 2002; Zhang et al., 2007). Pathogenic variants in these structural or regulatory proteins can in turn lead to structural and functional impairment at the Z-disk, resulting in cardiomyopathies or other pathologies, e.g., skeletal muscle diseases (Avnika et al., 2012; Wadmore et al., 2021).
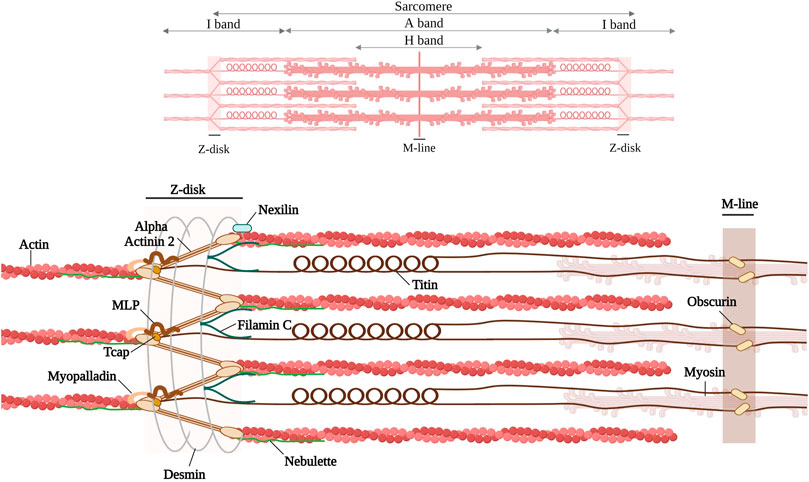
FIGURE 1. Schematic representation of the discussed Z-disk proteins (alpha-actinin 2, Filamin C, Titin, muscle LIM protein, telethonin, myopalladin, nebulette, nexilin) and Z-disk associated proteins (desmin and obscurin). Created with BioRender.com. Adapted from (Pyle and Solaro, 2004; Mukund and Subramaniam, 2020).
This review focuses on various cardiac Z-disk proteins (alpha-actinin, filamin C, titin muscle LIM protein, telethonin, myopalladin, nebulette, nexilin), all known to bind actin and to be associated with cardiomyopathies, and how genetic variants in these proteins are associated with different cardiomyopathies. We have further included two and Z-disk-associated proteins, desmin and obscurin (Figure 1). Due to space constraints we cannot discuss all components of the Z-disk, e.g., for alpha-beta crystallin, myotilin, alpha-actinin associated LIM protein (ALP), Z-band Alternative Spliced PDZ motif (ZASP), or filamin-, α-actinin-, and telethonin-binding protein of the Z-disk (FATZ), we refer to excellent reviews (Faulkner et al., 2001; Knöll et al., 2011a; Wadmore et al., 2021).
The evaluation of genetic variants and their role in cardiomyopathies is complex and multidimensional as outlaid in the American College of Medical Genetics and Genomics guidelines (Richards et al., 2015). In addition, with the advancement of high-throughput sequencing techniques the understanding of genetic variation in normal populations has evolved. This means that genetic variants or entire genes previously implicated in a disease, based on Sanger sequencing of small cohorts, may not be considered to contribute significantly to the disease today, as was demonstrated for HCM (Ingles et al., 2019) and DCM (Jordan et al., 2021).
A recent large scale genetic study has suggested a threshold of minor allelic frequency (MAF) lower than 1 × 10−4 in the cohort database of the Exome Aggregation Consortium for a missense variant to be considered pathogenic. This is based in the fact that the most common HCM-associated MYPBC3 missense variant p. Arg502Trp was only found at MAF of 2.5 × 10−5 in the database, hence no other cardiomyopathy variant is likely to be more common. While this review cannot re-evaluate all reported genetic variants in the genes we discuss according to the American College of Medical Genetics and Genomics guidelines (Richards et al., 2015), we have applied the same MAF cutoff and only discuss variants with MAF <1 × 10−4, using the Genome Aggregation Database (GnomAD, which is the largest cohort database available at the moment). This frequency threshold will help to better distinguish rare pathogenic variants in Z-disk protein from more common variants, which are unlikely to cause monogenic disease (Fahed et al., 2020).
2 Z-disc proteins and their association with cardiomyopathies
2.1 Alpha-actinin
2.1.1 Structure and function of alpha-actinin
Alpha-actinin is a protein with a molecular weight of 94 kDa–104 kDa. It forms antiparallel, actin-crosslinking homodimers (Hein et al., 2009). Alpha-actinin belongs to a highly conserved family of actin-binding proteins, the spectrin superfamily (Dixson et al., 2003; Sjöblom et al., 2008; Chiu et al., 2010). This family consists of short and long actin cross-linkers (such as the alpha-actinins and the alpha- and beta-spectrin) as well as dystrophin and utrophin, which are monomeric actin filament binding proteins and membrane adaptors (Ylänne et al., 2001; Dixson et al., 2003; Sjöblom et al., 2008; Foley and Young, 2014). The name of the spectrin superfamily derives from the presence of specific spectrin repeats (Foley and Young, 2014). All members of this family, including alpha-actinin have an amino-terminal actin-binding domain (ABD), consisting of two consecutive calponin homology domains (CH1 and CH2) (Figure 2A) (Sjöblom et al., 2008). The adjacent alpha-helical neck region connects the ABD with a central rod consisting of spectrin repeats with the number of repeats determining the flexibility and the nature of actin-binding properties (Ylänne et al., 2001; Sjöblom et al., 2008). The rod domain of alpha-actinin monomers comprises of four spectrin repeats, which interact to form antiparallel dimers (Figure 2B) that act on cross-linking actin filaments (Clark et al., 2002). In the carboxy-terminal region, there is a calmodulin homology domain (Critchley, 2000) that consists of two pairs of EF-hand motifs (EF1/2 and EF 3/4) (Sjöblom et al., 2008; Ribeiro Ede et al., 2014; Lindholm et al., 2021). EFs act on distinguishing between the four isoforms of alpha-actinin. The non-muscle isoforms (ACTN1 and ACTN4) bind calcium through their EFs, whereas the EFs of the muscle isoforms (ACTN2 and ACTN3) have lost their ability to bind calcium (Sjöblom et al., 2008; Hein et al., 2009; Chiu et al., 2010).
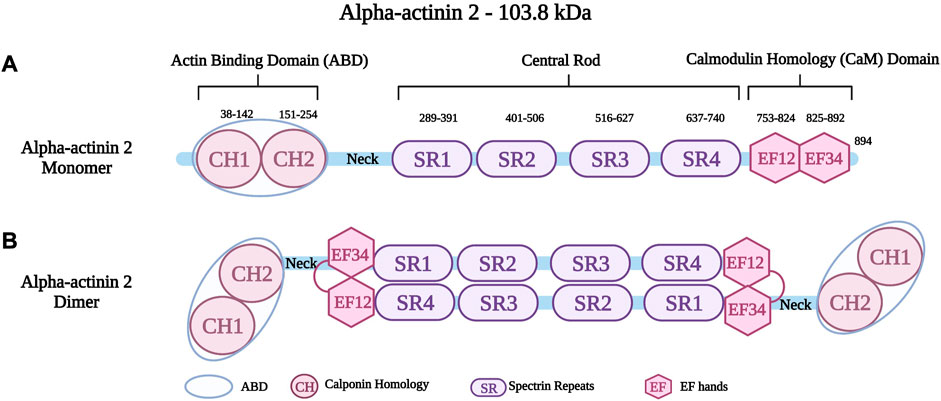
FIGURE 2. (A) Schematic representation of alpha-actinin two monomer with different residues in the Actin Binding Domain (ABD), Spectrin repeats (SR) and EF hands (EF). (B) Schematic representation of alpha-actinin two dimer showing the interaction between the neck region and the EF hands. Created with BioRender.com. Adapted from (Haywood et al., 2016).
Alpha-actinin 2 (encoded by ACTN2 on chromosome 1q43), is a major isoform localized at the Z-disk of the sarcomere (Hein et al., 2009; Prondzynski et al., 2019). Like all alpha-actinins, it forms antiparallel dimers. An intramolecular interaction of the neck region with EF3/4 keeps the dimer in a closed confirmation, which can be opened upon phospholipid binding (Ribeiro Ede et al., 2014). It is known to anchor and cross-link actin and titin filaments from adjacent sarcomeres (Ylänne et al., 2001; Chiu et al., 2010; Lindholm et al., 2021). This crucial protein stabilizes the contractile muscle apparatus by forming a lattice-like structure between titin and actin filaments (Sjöblom et al., 2008; Good et al., 2020). Alpha-actinin two further plays a crucial role in the organization of thin filaments by linking the cytoskeleton to different transmembrane proteins (Sjöblom et al., 2008). In addition to the role of alpha-actinin two in the structural integrity of the sarcomere, it also has important cellular roles in regulating the transactivation activity of various receptors, such as glucocorticoid receptor interacting protein-1 (GRIP1) (Huang et al., 2004). Moreover, it also regulates ion channels such as potassium channels (Kv1.5 and Kv1.4) (Cukovic et al., 2001) and the Ca2+ activated K+ channel (SK2 channel) (Lu et al., 2009). Other roles include connecting the sarcomeric cytoskeleton to various transmembrane proteins (Otey and Carpen, 2004). Global inactivation of alpha-actinin two in mice is not compatible with life, but a cardiac specific mosaic inactivation suggests a crucial role of the protein for cardimyocyte maturation via serum response factor signaling (Guo et al., 2021). Moreover, a role of alpha-actinin two in regulating cardiac mitochondrial function has been proposed recently (Zech et al., 2022), in part by acting as a scaffold for mitochondrial messenger RNAs via binding to RNA-binding proteins (Ladha et al., 2020).
2.1.2 Alpha-actinin two and cardiomyopathies
Several studies have shown an association between pathogenic variants in ACTN2 and various cardiac manifestations, including HCM, DCM, and ACM. A study by Prondzynski et al. identified a rare variant in ACTN2 (p.Thr247Met) in one HCM patient with left ventricular hypertrophy, outflow tract obstruction, and atrial fibrillation (Prondzynski et al., 2019). Human induced pluripotent stem cells (iPSCs) were reprogrammed from the patient, and subsequently, differentiated into iPSC cardiomyocytes (iPSC-CMs), and engineered heart tissues. The results revealed several features of HCM including myofibrillar disarray, cardiomyocyte hypertrophy, impaired relaxation, hypercontractility, increased myofilament calcium sensitivity, and prolonged action potential duration (Prondzynski et al., 2019). A recent study by Zech et al. involved used iPSC-CMs heterozygous and homozygous for this ACTN2 variant (p.Thr247Met) and showed multinucleation, cardiomyocyte hypertrophy, and myofibrillar disarray in the cellular models (Zech et al., 2022).
A study by Chiu et al. involved 23 patients with HCM and the results identified an ACTN2 missense variant (p.Ala119Thr). In addition, the overexpression of this variant resulted in a significant increase in RNA markers for cardiomyocyte hypertrophy (Chiu et al., 2010). Another study examined a large four-generation family with autosomal dominant cardiomyopathic features including mid-apical HCM with marked bilateral dilatation, LVNC, and early onset of atrial fibrillation and atrioventricular block. The study identified a pathogenic variant (p.Met228Thr) in ACTN2 (Girolami et al., 2014). In another study by Theis et al., two ACTN2 genetic variants (p.Gly111Val and p. Thr495Met) were mapped from two patients with HCM, and a pathology report showed marked cardiomyocyte hypertrophy, focal myocyte disarray, endocardial fibrosis, and interstitial fibrosis (Theis et al., 2006). A study by Haywood et al. functionally characterized two ACTN2 variants (p.Gly111Val and p. Ala119Thr), located in the actin-binding domain. These variants impaired alpha-actinin two function by decreasing F-actin binding affinity and altering Z-disk localization. This contributes to the disease phenotype of HCM (Haywood et al., 2016).
Another study by Lindholm et al. derived iPSC-CMs from a patient presenting with tachypnea and reduced ejection fraction. This patient was found to carry a rare ACTN2 truncating variant (p.Gln860Ter). The iPSC-CMs were hypertrophic, exhibited structural disarray of the sarcomere, and impaired contractility. Moreover, this variant resulted in a loss in the carboxy-terminus of alpha-actinin 2, hence leading to disruption of the interaction with two sarcolemma-associated proteins: alpha-actinin 1 (ACTN1) and Gap junction alpha-1 protein (GJA1), which may explain the clinical arrhythmic and relaxation defects (Lindholm et al., 2021).
A study by Fan et al. involved a Chinese family with DCM, and ventricular tachycardia. This family was found to have a likely pathogenic variant in ACTN2 (p.Leu320Arg) which was identified by whole-genome sequencing in four out of 12 affected family members (Fan et al., 2019). In another study, a ACTN2 variant (p.Ala119Thr) was detected in an Australian family that exhibited a cardiac phenotype of DCM as well as idiopathic ventricular fibrillation, LVNC, and sudden death. Using exome sequencing, this variant was identified as responsible for the cardiac phenotype that the family exhibited (Bagnall et al., 2014). In a study by Good et al., a clinical investigation was performed on a family with left-dominant ACM. The ACTN2 missense variant (p.Tyr473Cys) was identified in four family members and was considered causative of familial left dominant ACM (Good et al., 2020).
In conclusion, several studies have localized ACTN2 pathogenic variants in different regions of alpha-actinin 2 (Supplementary Table S1). Studies have best documented the association of ACTN2 pathogenic variants and HCM, albeit as rare causes of the disease (Theis et al., 2006; Chiu et al., 2010; Haywood et al., 2016). In contrast, the role of ACTN2 variants in both DCM and ACM is less well understood.
2.2 Filamin C
2.2.1 Structure and function of filamin C
Filamins are elongated dimers (Stossel et al., 2001) that cross-link and bind to F-actin filaments (Stossel et al., 2001; Popowicz et al., 2006; Leber et al., 2016). The filamin dimer is also called actin-binding protein-280 (ABP-280) (van der Ven et al., 2000) and it consists of two 280 kDa subunits (Himmel et al., 2003). Filamins have an ABD at their amino-terminus, which consists of two calponin homology domains (CH1 and CH2) (Bañuelos et al., 1998; van der Flier and Sonnenberg, 2001; Himmel et al., 2003). The ABD is followed by a rod domain consisting of 24 immunoglobulin-like (Ig) repeats structured into two rod regions (Rod one consists of Ig1 to Ig15 and Rod2 consists of Ig16 to Ig23) interrupted by two hinges (hinge one and 2) (Figure 3) (van der Flier and Sonnenberg, 2001; Pudas et al., 2005; Leber et al., 2016). The last Ig domain (Ig24) is present at the terminal end, and it allows filamins to dimerize. This structural organization allow filamins to adopt a flexible V-shaped structure that is essential for their function (Feng and Walsh, 2004; Zhou et al., 2007).
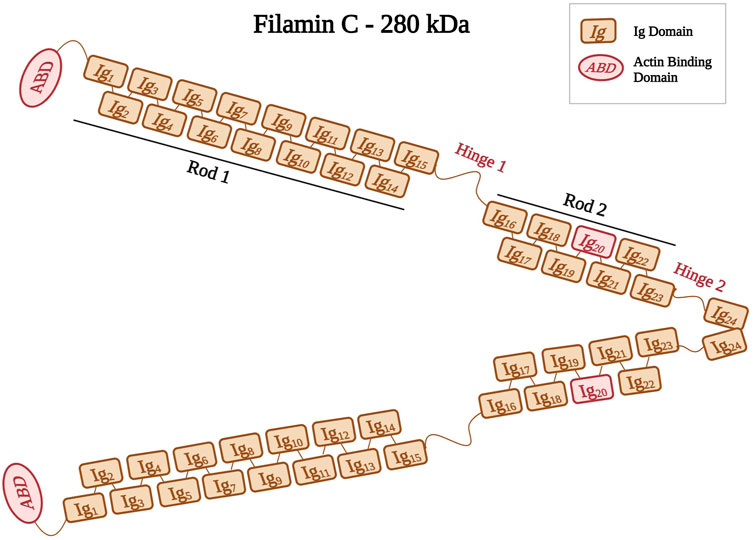
FIGURE 3. Schematic representation of filamin C shown as a dimer with different Ig domains forming both Rods (Rod1, Ig1 to Ig15; Rod2, Ig16 to Ig23). Positions of hinges one and two are also indicated. Ig20 is highlighted in red because of its unique 80 amino acid insertion. Created with BioRender.com.
In addition, filamins have numerous binding partners, including intracellular signaling molecules, integrin receptors (β1D-integrin) (Loo et al., 1998), δ- and γ-sarcoglycans (Thompson et al., 2000), ion channels, and transcription factors (Himmel et al., 2003; Zhou et al., 2007; Nakamura et al., 2011). The interaction of filamins with different binding partners affects cellular activities, such as cytoskeletal organization, cellular motility, and differentiation (Razinia et al., 2012). Filamins also play an important role in maintaining the stability of the sarcomere and transmitting and responding to the mechanical forces acting on the F-actin network (Stossel et al., 2001; Feng and Walsh, 2004; Razinia et al., 2012).
The filamin family includes three members, filamin A, filamin B, and filamin C (Feng and Walsh, 2004). Filamin A and B are widely expressed, whereas filamin C is expressed predominantly in both skeletal and cardiac muscles (Stossel et al., 2001; Zhou et al., 2007). Filamin C is located at the Z-disks, costameres, and intercalated disks (Brodehl et al., 2017). Its gene FLNC is mapped to the chromosomal region 7q32–q35 (Gariboldi et al., 1994; Kley et al., 2012). Filamin C acts on anchoring and cross-linking actin filaments into a meshwork (van der Ven et al., 2000; Himmel et al., 2003; Begay et al., 2016). It plays an important role in the Z-disk assembly (van der Ven et al., 2006), where it anchors various proteins, such as myopodin, and myotilin (Thompson et al., 2000), to the actin cytoskeleton (van der Flier and Sonnenberg, 2001). Filamin C has a unique 80 amino acid insertion in its 20th Ig domain (Ig20) that mediates binding to various ligands and muscle proteins, such as Xin actin-binding repeat-containing proteins (XIRP1 and 2) (Molt et al., 2014; Mao and Nakamura, 2020). This interaction between Filamin C and XIRPs allows the stabilization of actin filaments by regulating cell adhesion dynamics (Seppälä et al., 2015).
The importance of filamin C for cardiac integrity is highlighted in two independent animal studies: cardiac-specific, and inducible FLNC knockout mouse models led to rapid DCM and heart failure. The study by Powers et al. demonstrated rapid onset DCM, with significant cardiomyocyte lengthening without a change in width. The mice were also found to have disrupted Z-disk myofilament organization (Powers et al., 2022). In another study by Zhuo et al., death of an inducible, cardiac specific FLNC knockout mice model occurred within 1 week of ablation of the protein due to heart failure. Z-disk, costameres and intercalated disc structures were affected in the hearts (Zhou et al., 2020).
2.2.2 Filamin C and cardiomyopathies
Pathogenic variants in FLNC can affect sarcomere structure and function, leading to cardiac dysfunction and cardiomyopathies (Fürst et al., 2013). Missense variants in FLNC have been identified as novel causes of HCM. In a study by Valdés-Mas et al., whole-exome sequencing (WES) was performed in 92 HCM cases, and the following FLNC variants were identified (p.Val123Ala, p. Ala1539Thr, and p. Arg2133His). Patients with these FLNC variants show marked abnormalities in sarcomeres and large aggregates of filamin C (Valdés-Mas et al., 2014).
Gomez et al. performed next-generation sequencing (NGS) in 448 patients with HCM, and 20 different FLNC variants were identified (19 missense and one non-sense), suggesting that FLNC pathogenic variants are an important cause of this cardiomyopathy (Gómez et al., 2017).
A study by Ader et al. included sequencing of 1150 patients (among them 700 with HCM and 300 with DCM), and multiple pathogenic FLNC variants were identified in 28 patients (See Supplementary Table S2) (Ader et al., 2019). They suggest that truncation variants in FLNC are prevalent in DCM, while missense or small in frame deletions are seen in other types of cardiomyopathies.
In a study by Chanavat et al., NGS was performed on a cohort of 90 patients, with two variants identified in HCM cases (p.Arg2045GLn and p. Arg2018His) and one variant identified in a DCM case (p.Tyr1840Ter) (Chanavat et al., 2016). A study by Begay et al., a FLNC variant (p.Gly1891Val) was identified by WES in two Italian families with DCM and a clinical presentation of supraventricular tachycardia and atrial fibrillation (Begay et al., 2016).
In a further study, 319 families with DCM from the United States and Europe were examined using WES and NGS. Six different FLNC truncation variants were identified where 11 of the 13 truncation carriers had ventricular arrhythmias or sudden cardiac death (Begay et al., 2018). In a study by Augusto et al., seven DCM patients out of 89 had different FLNC truncations and showed abnormalities in left ventricular wall motion, left ventricular impairment, and non-sustained ventricular tachycardia (see Supplementary Table S2) (Augusto et al., 2020).
In addition, FLNC variants have been associated with the pathogenesis of other cardiomyopathies, e.g., ACM. In a study by Ortiz-Genga et al., NGS was used on 2,877 patients with inherited cardiovascular diseases including (1078 with HCM, 508 with DCM, 219 with ACM, and several other diseases). 23 FLNC truncating variants were identified in 28 patients (20 with DCM, seven with ACM, and one with RCM) (Ortiz-Genga et al., 2016).
Another study by Celeghin et al. included 270 participants, 12 FLNC variants (five missense and seven truncating ones) were mapped in 18 patients with ACM (Celeghin et al., 2022). In another study, WES of 120 gene-elusive ACM index cases identified four truncating FLNC variants (Hall et al., 2020). Moreover, Brun et al. (2020) also tested 156 patients with ACM and detected two FLNC truncations (p.Glu2189Ter and p. Asp2703ThrfsTer69) (Brun et al., 2020).
Some studies also investigated the association between FLNC variants and RCM. A study by Brodehl et al. tested 123 patients and revealed two novel FLNC missense variants (p.Ser1624Leu and p. Ile2160Phe) (Brodehl et al., 2016). Another study also identified two FLNC variants (p.Pro2298Leu and p. Tyr2563Cys) in two families with RCM (Schubert et al., 2018). In a further study by Kiselev et al., a cohort of 28 patients with early-onset RCM was screened and the FLNC variant (p.Ala1186Val) was identified in three patients and (p.Ala1183Leu) in one patient (Kiselev et al., 2018).
A functional study by Tucker et al. involved eight patients with RCM and WES identified a novel FLNC missense variant (p.Val2297Met). iPSC-CMs with the variant displayed decreased contractility compared to the wild type, thus suggesting a defect in the contractile apparatus (Tucker et al., 2017). Another study by Agarwal et al. detected the formation of filamin C aggregates when a heterozygous in-frame deletion of FLNC (p.Val1668_Gly1674del) was introduced into iPSC-CMs. Other observations included disassembly of the sarcomere structure and a reduction in cardiomyocyte contractility (Agarwal et al., 2021). Further, Chen et al. generated iPSC lines from two DCM patients with two FLNC truncating variants (p.Gly1891Valfs61Ter, and p. Glu2189Ter). These iPSC-CMs displayed impaired contraction evidenced by a weaker contraction and slower relaxation. They also exhibited spontaneous arrhythmia and iPSC-CMs with the variants were found to have a lower expression of filamin C protein and mRNA (Chen et al., 2022).
In conclusion, FLNC variants are associated with different cardiomyopathies (see Supplementary Table S2). It emerges that truncating variants in FLNC are associated with DCM and ACM without overt skeletal muscle pathologies. Currently it is enigmatic why other truncating variants in the same gene cause predominantly skeletal muscle disease (Selcen and Carpén, 2008). Moreover, missense variants in FLNC have been associated with HCM and RCM, however assigning causality to individual variants is challenging as missense variants in FLNC also occur frequently in normal cohorts.
2.3 Titin
2.3.1 Structure and function of titin
Titin protein, also known as connectin, is a giant elastic filament that spans half the sarcomere (Bang et al., 2001a). It is the third most abundant muscle protein (after myosin and actin) in the mammalian organisms and has a molecular weight of 3 MDa (Maruyama, 1997; Granzier and Labeit, 2002; Au, 2004). The titin gene (TTN) is located on chromosome 2q31, which contains 363 exons (Bang et al., 2001a) and codes for 26,926 amino acids (Labeit and Kolmerer, 1995). Titin extends over half of the sarcomere, and it connects the thin filaments at the Z-disk with its amino-terminus to the thick filaments at the M-band with its C-terminus (Fürst et al., 1988; Maruyama, 1997; Au, 2004). The I-band region of titin is responsible for the elasticity of muscle tissues (Chanavat et al., 2016). Titin’s A-band region helps to stabilize thick filaments (reviewed in (Chauveau et al., 2014; Gigli et al., 2016)).
Titin exons undergo extensive alternative splicing thus producing two titin isoforms (N2B and N2BA) that are expressed in the myocardium. The two isoforms span the sarcomere Z-disk to M-band but differ primarily in the I-band resulting in different sizes. N2BA isoform consists of both the N2A and N2B segments while the N2B isoform consist of a smaller PEVK segment, fewer Ig domains and it lacks the N2A segment (Granzier and Labeit, 2004; Nagueh et al., 2004; Roberts et al., 2015).
Titin has several roles including the generation of the passive force (Kollár et al., 2010), maintaining sarcomeric alignment and regulating myosin filament assembly (Whiting et al., 1989; Labeit and Kolmerer, 1995). By binding a multitude of signaling proteins, titin also plays a central role in integrating mechanical and hypertrophic signals from and to the sarcomere (Granzier et al., 2009; Kollár et al., 2010).
Global inactivation of titin in animals is not compatible with life, highlighting the crucial function of the protein for muscle integrity. However, spontaneous natural mutations or targeted engineered genetic variants, or deletions of titin regions, in fish or rodents, have shed light on important functions of the protein and are reviewed in (Marcello et al., 2022).
2.3.2 Titin Z-disk portion
This review will focus on the Z-disk portion of titin (first 15 exons, approximately 80 kDa). Titin Z-disk portion is composed of ∼900 residues and it consists of consecutive immunoglobulin (Ig)-like domains and 45-residue repeating modules (called Z-repeats or Zr) (Figure 4) (Gautel et al., 1996; Tonino et al., 2019). The Z-repeats vary in number according to the muscle type (Luther and Squire, 2002). The first two Ig domains (termed Z1 and Z2) in titin’s amino-terminus bind telethonin (also known as T-cap). This interaction is the strongest, non-covalent interaction described between two proteins (Bertz et al., 2009) and it allows anti-parallel alignment of adjacent titin molecules within the Z-disk (Gregorio et al., 1998; Young et al., 1998; Zou et al., 2006). In addition, the fifth Z-repeat (Zr5) of titin has been found to bind the C-terminus of alpha-actinin (Ohtsuka et al., 1997). Several studies discussed the interaction between titin and alpha-actinin to take place between the seventh Z-repeat (Zr7) of titin and the EF hands (EF34) of alpha-actinin (Sorimachi et al., 1997; Young et al., 1998; Atkinson et al., 2000; Joseph et al., 2001; Haywood et al., 2016).
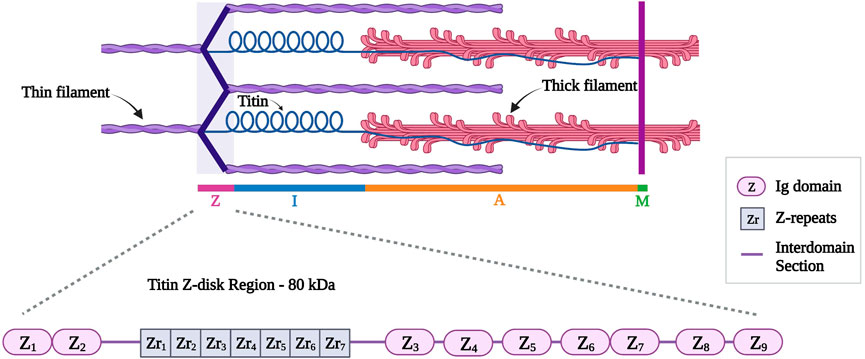
FIGURE 4. Schematic representation of titin (in blue) different regions with a detailed representation of titin Z-disk region with different Ig domains (Z1 to Z9) and Z-repeats (Zr1 to Zr7). Created with BioRender.com.
2.3.3 Titin and cardiomyopathies
Several studies have shown that pathogenic variants in TTN are associated with DCM. The role of truncating variants in TTN (TTNtv) is well established in DCM (Herman et al., 2012), where a large study found that TTNtv associated with DCM are found in the exons with high cardiac expression (Roberts et al., 2015). In addition, the position of the variant within the gene plays an important role. For instance, the association between TTN truncating variants and DCM in the A-band portion has a much higher odds ratio (odds ratio 49.8) than in the Z-disk portion (odd ratio 5.3) (Schafer et al., 2017).
In contrast, truncating variants in TTN are not associated with HCM: In a study by Zhang et al., WES was performed on 529 Chinese patients with HCM and 307 healthy controls. They identified 14 TTN truncating variants (six non-sense, two frameshift and six splice site variants) in 13 HCM patients (13 of 529 [2.5%]) and eight controls (8 of 307 [2.6%]). Thus, no significant difference was observed between the HCM patients and the controls carrying the TTN variants (Zhang et al., 2017).
In a study by Taylor et al., 38 families with ACM were sequenced and eight TTN missense variants were detected in seven families. One of the variants (p.Thr2896Ile) is located at the 10th Ig domain and was shown to reduce the structural stability of this domain (Taylor et al., 2011).
Due to the high abundance of TTN missense variants in normal cohorts (Xiao et al., 2021), their role in disease is harder to evaluate. Nevertheless, individual TTN missense variants have been identified as cardiomyopathy-associated (Supplementary Table S3); the following description of individual variants will be limited to the Z-disk portion of titin:
In a study by Micheu et al., NGS was used in 45 patients with HCM, and seven novel TTN missense variants were identified as possibly pathogenic. The two TTN variants (p.Ala840Ser and p. Val17Leu) were predicted to destabilize and affect the function of titin (Micheu et al., 2021). In another study by Satoh et al., 82 patients with HCM were tested, and a novel TTN variant (p.Arg740Leu) was mapped. This variant was shown to increase the binding affinity between titin and alpha-actinin (Satoh et al., 1999).
In a further study, two TTN variants (p.Ala743Val and p. Val54Met) were found in a cohort of 120 DCM patients. These variants were found to decrease the binding affinity between titin and alpha-actinin and the binding between titin and telthonin/T-cap (Itoh-Satoh et al., 2002).
In a study by Hastings et al., a three-generation family affected by DCM and LVNC was screened. They identified a TTN missense variant (p.Ala178Asp) as likely pathogenic. In vitro experiments documented protein degradation and destabilization, partial protein unfolding, and altered binding properties to the ligand telethonin (Hastings et al., 2016). A follow-on study by Jiang et al. generated a mouse model for the same TTN missense variant (p.Ala178Asp). Heterozygous mice had no detectable phenotype, however, the homozygous mouse model developed DCM at 3-month age and the binding partner telethonin was found to be absent from the Z-disks of these mice (Jiang et al., 2021).
In summary, truncating variants in TTN are now recognized as the leading genetic cause for DCM. In addition, individual TTN missense variants have been associated with cardiomyopathies, but due to the giant size of titin and the high abundance of missense variants in normal cohorts, it is challenging to evaluate variants identified in an individual by genetic testing. One solution proposed is a web application called TITINdb, which integrates information on titin structure, sequence, variant, and isoforms. This information, along with pre-computed predictions of the nucleotide variant’s impact on the protein, can facilitate a classification of titin variants (Laddach et al., 2017). Moreover, the emerging quantitative understanding of which exons are expressed in adult cardiac transcripts (often expressed as percentage spliced (Aufiero et al., 2018)), can help to disregard variants in exons which are not expressed in adult cardiac transcripts.
2.4 Muscle LIM protein (MLP)
2.4.1 Structure and function of MLP
Muscle LIM protein (MLP) belongs to the cysteine-rich protein (CRP) family and is also known as cysteine- and glycine-rich protein 3 (encoded by CSRP3) (Geier et al., 2008). The CSPR3 gene is located on chromosome 11p15.1 and it consists of five exons (Fung et al., 1995). MLP is 194 amino acids long and has a molecular weight of 23 kDa, consisting of two LIM domains (LIM1 and LIM2). LIM1 is followed by a nuclear-localization sequence (NLS) (Harper et al., 2000); together both form the amino-terminus of MLP (Figure 5) (Harper et al., 2000). The LIM1 domain is responsible for binding to alpha-actinin at the Z-disk (Arber et al., 1997; Harper et al., 2000). It also interacts with telethonin and calcineurin, which are involved in the detection and transduction of hypertrophic signaling pathways in response to mechanical stress (Knöll et al., 2002). MLP also has roles in calcium handling, myofibrillogenesis, and actin polymerization (Buyandelger et al., 2011). It is further important for the maintenance, assembly, and organization of the actin cytoskeleton (Arber et al., 1997; Buyandelger et al., 2011). Other roles involve mediating protein interactions and attachment of the contractile apparatus to the plasma membrane (Schallus et al., 2009).
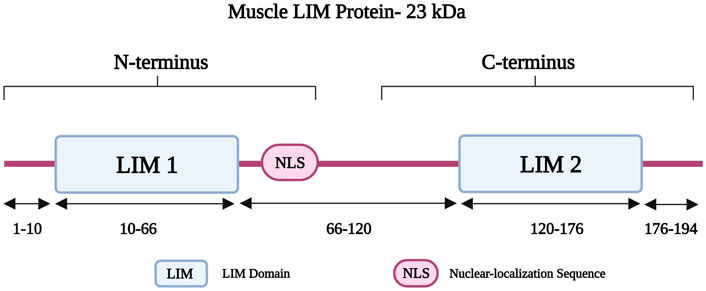
FIGURE 5. Schematic representation of the muscle LIM protein regions with different residues in the two LIM domains and the Nuclear-localization Sequence (NLS). Created with BioRender.com. Adapted from (Chauhan and Sowdhamini, 2022).
Inactivation of CSRP3 in mice showed significant ventricular dilation and systolic dysfunction along with substantial myocardial hypertrophy resulting in a phenotype resembling DCM (Arber et al., 1997). Since then, the CSRP3 knockout mouse model has been used as a genetic form of heart failure in many studies (Gardiwal et al., 2007; Heineke et al., 2010; Lange et al., 2016).
2.4.2 Muscle LIM protein and cardiomyopathies
Genetic variants in CSPR3 have been linked to the pathogenesis of HCM and DCM (Supplementary Table S4). In a study by Bos et al., 389 patients with HCM were analyzed, and 12 different variants were detected in 16 HCM patients including the following CSRP3 variants (p.Leu44Pro, p. Arg64Cys, and p. Tyr66Cys). This study also identified a frameshift variant (p. Lys42fs/165) (Bos et al., 2006). Another CSRP3 variant (p.Arg70Trp), which is located in the T-cap binding region, was reported in an HCM patient with left ventricular hypertrophy, who had a septal wall thickness of 46 mm (Bos et al., 2006).
A study by Geier et al. screened 1100 individuals (200 HCM patients, 400 DCM patients, and 500 controls) for CSRP3 pathogenic variants by Sanger sequencing. Three HCM-associated variants were identified (p.Leu44Pro, p. Cys58GLy, and p. Ser54Arg/p.Glu55GLy) (Geier et al., 2003). Using a yeast 2-hybrid assay, the CSRP3 variant (p. Cys58GLy) showed impaired binding affinity to alpha-actinin (Geier et al., 2003). In another study involving 6456 HCM patients, the CSRP3 variant (p.Cys150Tyr) was identified in 11 unrelated patients (Salazar-Mendiguchía et al., 2020). In a more recent study by Huang et al. (2022), WES was performed in a Chinese family with HCM, and the truncating CSRP3 variant (p.Arg122Ter) was identified and considered pathogenic (Huang et al., 2022). Lipari et al. performed NGS in 29 Polish patients with HCM. The same CSRP3 variant (p.Arg122Ter) was found in one HCM case in this cohort (Lipari et al., 2020).
To gain insights into the functional consequences of CSRP3 variants, Ehsan et al. generated a knock-in mouse model (KI), carrying an HCM-causing variant (p.Cys58GLy). The heterozygous KI mice showed no cardiac phenotype, while the homozygous KI mice had enlarged left ventricular dimensions, decreased systolic function, and increased left ventricular mass. They further showed an 80% decrease in MLP abundance at protein level in homozygous KI mice compared to 50% in the heterozygous KI mice. In conclusion, the (p.Cys58GLy) variant could cause cardiomyopathy through protein depletion, lack of functional protein and overload of the protein degrading activity of the ubiquitin-proteasome system (Ehsan et al., 2018).
Another study showed that a CSRP3 variant (p.Leu44Pro) can affect the structure of the MLP LIM1 domain due to the loss of hydrophobic interaction between Leucine and Phenylananine (Chauhan and Sowdhamini, 2022).
Another study by Mohapatra et al. involved 291 patients with DCM and a CSRP3 variant (p.Lys69Arg) was identified in one patient. In this variant, the interaction between MLP and alpha-actinin two was abolished and localization of MLP was also found to be altered (Mohapatra et al., 2003). A genetic study by Hershberger involved 313 patients with DCM (divided between familial and idiopathic), and different variants were found including one CSRP3 missense variant (p.Gly72Arg) (Hershberger et al., 2008).
For a discussion of CSRP3 p. Trp4Arg, which was initially described as a pathogenic variant, but is now considered a disease modifier, see section 4. Conclusion.
While the association between different CSRP3 pathogenic missense variants and HCM is very well documented (Geier et al., 2003; Ehsan et al., 2018; Lipari et al., 2020), further studies are required to better understand the association between CSRP3 variants and DCM.
2.5 Telethonin
2.5.1 Telethonin structure and function
Telethonin, also known as titin cap or T-cap, is a 19 kDa protein of 167 amino acids (Valle et al., 1997). It is encoded by TCAP on chromosome 17q12 (Anand, 2019). Telethonin is expressed in both cardiac and skeletal muscle and localizes to the Z-disk (Valle et al., 1997). It contributes to the assembly, stability, and structural integrity of the sarcomere (Anand, 2019). Telethonin is also part of a mechanosensing protein complex, together with titin, and MLP (Faulkner et al., 2001; Knöll et al., 2002). It anchors titin within the Z-disk (Gregorio et al., 1998) and it mediates the assembly of two adjacent titin molecules through the interaction of the first 140 residues of T-cap with the titin amino-terminal Ig domains Z1 and Z2 (Figure 6) (Mues et al., 1998; Lopes and Elliott, 2014). Telethonin is further implicated in T-tubule biogenesis (Al-Qusairi and Laporte, 2011); it regulates the T-tubular structure and integrity by binding to proteins in the T-tubule membrane (Ibrahim et al., 2013).
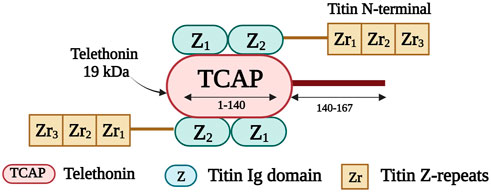
FIGURE 6. Schematic representation of the interaction taking place between the first 140 residues of telethonin and the first two titin Ig domains (Z1 and Z2). Created with BioRender.com.
Genetic inactivation of telethonin in mice does not induce a baseline phenotype, however, heart failure response to biomechanical stress is aggravated in the mouse heart in the absence of telethonin (Knöll et al., 2011b).
2.5.2 Telethonin in cardiomyopathies
Pathogenic variants in TCAP are rare, nevertheless they have been associated with HCM and DCM (Supplmentary Table S5). In a study by Toste et al., a novel TCAP variant (p.Cys57Trp) was identified in a small family with HCM presenting with left ventricular hypertrophy, atrial fibrillation, and heart failure with preserved ejection fraction (HFpEF). The variant is localized in the binding region to MLP and titin (Toste et al., 2020). In a study by Bos et al., 389 HCM patients were screened and three TCAP variants (p. Glu13del, p. Arg70Trp, and p. Pro90Leu) were identified in four patients (Bos et al., 2006). In another study by Hayashi et al., three TCAP variants were found after analyzing 346 patients with HCM (p.Thr137Ile) and 136 patients with DCM (p.Glu132GLn and p. Arg87GLn), respectively (Hayashi et al., 2004). Further qualitative tests showed that HCM-associated TCAP variants enhanced the interaction between telethonin and titin, while DCM-associated variants impaired the interaction between telethonin, MLP, and titin (Hayashi et al., 2004).
In summary, TCAP variants have been associated with both DCM and HCM, but further insights at cellular level are needed to understand the mechanism by which TCAP variants cause cardiomyopathies (Faulkner et al., 2001).
2.6 Myopalladin
2.6.1 Function and structure of myopalladin
Myopalladin is encoded by MYPN on chromosome 10q21.1; the protein has a molecular weight of 145 kDa (Bang et al., 2001b). It belongs to a small family of proteins, that includes myopalladin, palladin and myotilin, which are characterized by the presence of actin-associated Ig domains (Otey et al., 2005). Myopalladin contains five Ig domains separated by six interdomain insertions (IS) where the IS3 includes a proline-rich motif (Figure 7) (Bang et al., 2001b). It is present in both the nucleus and sarcomere, with dual localization at the Z-disk and I-band (Purevjav et al., 2012).
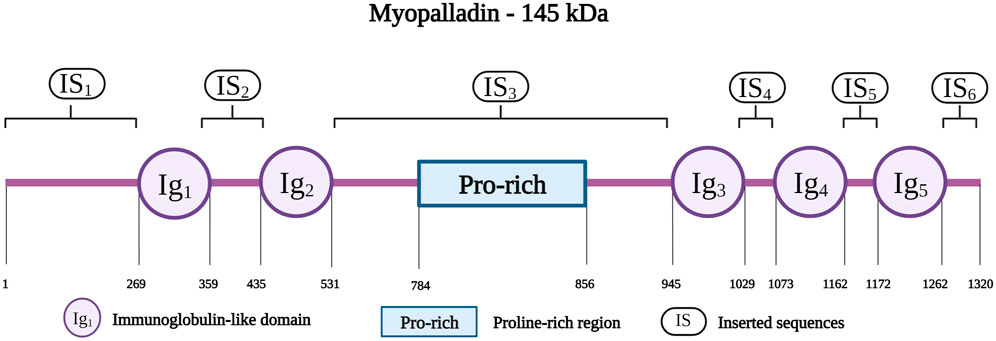
FIGURE 7. Schematic representation of the myopalladin with different residues spanning the five Ig domains (IG1 to IG5), Proline-rich region and the six inserted sequences (IS1 to IS6). Created with BioRender.com.
Myopalladin interacts with several Z-disk proteins. A study showed that the myopalladin carboxy-terminal region binds to fourth and fifth Ig domains (Z4 and Z5) of titin (Filomena et al., 2021). The C-terminus also has binding sites for alpha-actinin, nebulin in skeletal muscle, and nebulette in cardiac muscle (Taylor et al., 2006). The amino-terminal domain of myopalladin binds to cardiac ankyrin repeat protein (CARP), a nuclear protein involved in hypertrophic signaling in muscles (Zou et al., 1997). Myopalladin has also been shown to bind F-actin to prevent its depolymerization (Filomena et al., 2020). In addition, myopalladin plays an important role in regulating signal transduction and gene expression during muscle stress (Purevjav et al., 2012).
In a study by Filimena et al., a myopalladin knockout mouse model showed systolic dysfunction associated with fibrosis and decreased isometric tension of the myofibrils (Filomena et al., 2021). Moreover, delays in Ca2+ release and uptake were observed, suggesting that altered Ca2+ handling is a major contributor to DCM observed in mice lacking myopalladin (Filomena et al., 2021).
2.6.2 Myopalladin and cardiomyopathies
Pathogenic variants in MYPN have been identified and associated with DCM (Supplementary Table S6). In a study by Zhao et al., 21 patients with DCM were analyzed using NGS, and a pathogenic variant in MYPN (p.Glu630Lys) was identified (Zhao et al., 2015). In another study, 114 patients with DCM (65 familial and 49 sporadic) and 400 controls were included. Two heterozygous variants (p. Arg1088His and p. Ile83fsX105) were found in the familial DCM group (Duboscq-Bidot et al., 2008). In a study by Meyer et al., MYPN coding regions were analyzed in a cohort of 255 patients with DCM. In one DCM patients, a heterozygous missense MYPN variant (p.Pro961Leu) was identified and localized in the actin-binding region of myopalladin. An endomyocardial biopsy of the patient showed marked disruption of sarcomere assembly, indicating myofibrillogenesis (Meyer et al., 2013). In a genetic study by Purevjav et al., MYPN was examined in 484 patients with HCM and in 348 patients with DCM (Purevjav et al., 2012). Several non-sense and missense variants were found in patients with HCM (p.Arg855Ter, p. Lys153Arg, p. Ala217GLu, p. Pro841Thr, and p. Ala265Pro) and DCM (p.Ile213Val, p. Ala611Thr, and p. Ala882Thr) (Purevjav et al., 2012).
A variant initially described as pathogenic for both DCM and HCM (e.g., p. Tyr20Cys) is too common to be disease causing (MAF of 9.34 × 10−4), despite clear functional effects of the variant: neonatal rat cardiomyocytes expressing this variant showed disrupted intercalated discs, impaired nuclear translocation and abnormal assembly of the terminal Z-disk within the intercalated discs (Purevjav et al., 2012). However, the high MAF of this variant suggests that it is a disease modifier instead, if at all.
In conclusion, both truncating and missense variants in MYPN have been associated with DCM, but the role of MYPN variants for HCM is not fully established.
2.7 Nebulette
2.7.1 Structure and function of nebulette
Nebulette and nebulin are two thin filament-associated isoforms found in the cardiac and skeletal muscles, respectively. They belong to the nebulin family, which consists of different actin-binding proteins (Moncman and Wang, 1999). Nebulette and nebulin are encoded by the same gene, NEBL, located on chromosome 10p12 (Li et al., 2004). Nebulette, the cardiac-specific isoform, has a small molecular weight (109 kDa) compared to nebulin (800 kDa) (Millevoi et al., 1998). The carboxyl-terminus of nebulette is located at the Z-disk, whereas its amino-terminus protrudes outside the Z-disk along the I-band at approximately 150 nm (Bang and Chen, 2015). The nebulette structure consists of a glutamate-rich region followed by 23-residue nebulin repeats of 35 amino acid residues each (Figure 8) (Moncman and Wang, 1995; Millevoi et al., 1998). A serine-rich linker region connects the nebulin repeats to a Src homology (SH3) domain located at the carboxy-terminus of nebulette (Arimura et al., 2000; Bang and Chen, 2015).
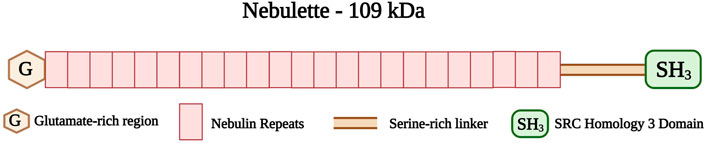
FIGURE 8. Schematic representation of the nebulette with glutamate-rich region (G) at the amino-terminus followed by 23 Nebulin repeats (35 amino acid residues each) and serine-rich linker connecting the SRC Homology 3 (SH3) domain at the carboxy-terminus. Created with BioRender.com.
Nebulette has a dual function, it acts as a Z-disk structural protein and further regulates signaling pathways between the nucleus and Z-disk during cardiac mechanical stretching (Ram and Blaxall, 2010). Nebulette contributes to the assembly of Z-disks (Ram and Blaxall, 2010), where it stabilizes and aligns actin filaments and cytoskeletal structures (Littlefield and Fowler, 2008). It is also involved in actin cytoskeleton organization (Ader et al., 2019). In addition, the SH3 domain of nebulette interacts with some proteins including filamin C (Holmes and Moncman, 2008), myopalladin (Bang et al., 2001b), alpha-actinin 2 (Esham et al., 2007), tropomyosin, and troponin T (Ogut et al., 2003). Through these interactions with various binding Z-disk-associated proteins, nebulette may participate in the regulation of signaling pathways (Ader et al., 2019).
A study by Mastrototaro et al. involved a knockout mouse model for nebulette. This model displayed normal cardiac function under basal conditions and in response to transaortic constriction (TAC). Molecular analysis showed no cardiac abnormalities. Nevertheless, transmission electron microscopy showed widening of the Z-disk, suggesting an essential role of nebulette in Z-disk integrity. Moreover, an upregulation in cardiac stress-responsive genes was observed, suggesting the presence of chronic cardiac stress (Mastrototaro et al., 2015).
2.7.2 Nebulette and cardiomyopathies
Different pathogenic variants in the NEBL gene are associated with DCM and HCM (Supplementary Table S7). In a study by Perrot et al., a cohort of 217 HCM patients, 148 DCM patients, and 320 controls was investigated. Sequencing of 28 exons of NEBL identified three rare heterozygous missense variants in six different patients. The (p.His171Arg) variant was found in two patients with HCM. Two other variants (p.Gln581Arg and p. Ser747Leu) were detected in three patients with DCM (Perrot et al., 2016).
In a study by Purevjav et al., a pathogenic variant (p.Gln128Arg) was identified and mapped to nebulette ABD. A transgenic mouse model with the (p.Gln128Arg) variant was embryonic lethal. Embryos harvested up to E12.5 showed an irregular localization pattern of nebulette with possible dissociation from the Z-disk. In addition, there was an impairment in the expression of the desmin protein (Purevjav et al., 2010).
A genetic study screened several genes in six cohorts of patients diagnosed with LVNC. Four NEBL missense variants (p.Ala726Thr, p. Ser863Cys, p. Ser296GLy, and p. Asn330Ser) were found in four cases out of a total of 278 patients (Mazzarotto et al., 2021).
In conclusion, NEBL variants have been described as rare causes of HCM, DCM and LVNC. However, many NEBL variants initially described do not meet stringent MAF cut-off criteria (Supplementary Table S7), and cannot be considered pathogenic, despite showing functional abnormalities in transgenic mouse studies (Purevjav et al., 2010; Maiellaro-Rafferty et al., 2013).
2.8 Nexilin
2.8.1 Nexilin structure and function
Nexilin is an F-actin-binding protein, encoded by the gene NEXN on chromosome 1p31.1. Nexilin has a molecular weight of 78 kDa and consists of 656 amino acids (Ohtsuka et al., 1998). Its amino-terminal consists of an ABD, followed by a coiled-coil (CC) domain. This is followed by another ABD, and a carboxy-terminal immunoglobulin superfamily class (IGcam) domain (Figure 9) (Ohtsuka et al., 1998; Hassel et al., 2009). Nexilin is located at the sarcomeric Z-disk and interacts with highly specialized sarcoplasmic reticulum (SR) proteins, termed junctional membrane complexes (Liu et al., 2019). It is considered an essential component of this complex where it participates in the development and maintenance of cardiac T-tubules (Liu et al., 2020; Spinozzi et al., 2020). In addition, this protein is essential for cardiac function and development (Johansson et al., 2022), and is involved in cell adhesion and migration in various tissues including cardiac and skeletal muscle tissues (Wang et al., 2005). Other roles include maintaining the integrity of the Z-disks against the tension generated within the sarcomere (Lopes and Elliott, 2014).
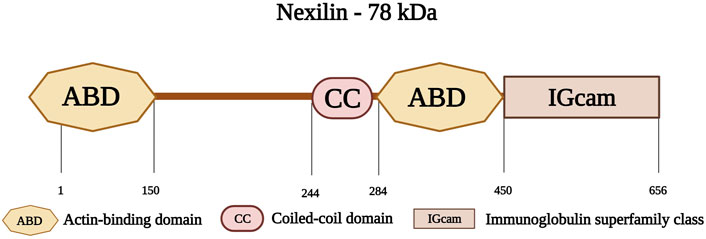
FIGURE 9. Schematic representation of nexilin with its first actin-binding domain (ABD) at the amino-terminus followed by a coiled-coil domain (CC) at the middle region and a second ABD. The carboxy-terminus consists of an Immunoglobulin superfamily class domain (IGcam). Created with BioRender.com.
In a study by Spinozzi et al., a nexilin knockout mouse model was generated. The authors studied isolated cardiomyocytes from the mouse model. Nexilin loss was associated with reduced cardiac function and progressive DCM, characterized by increased left ventricular diameter, ventricular wall thinning, and reduced ejection fraction. Other results revealed disorganization in the T-tubule network, with a significant decrease in the transversal component, indicating the importance of nexilin in the maintenance and organization of the transverse-axial tubule system in adult cardiomyocytes (Spinozzi et al., 2020).
Another study by Aherrahrou et al., involved a nexilin KO mouse model. The heart weight of the knockout mice was 2.3-fold higher than the WT hearts and knockout mice developed rapid progressive cardiomyopathy on day 6, had decreased systolic cardiac function, left ventricular dilation, and wall thinning. In addition, elastin and collagen deposits were observed within the cavity of the left ventricle, resembling features of endomyocardial fibroelastosis (Aherrahrou et al., 2016).
2.8.2 Nexilin and cardiomyopathy
Several studies have shown that pathogenic variants NEXN can lead to progressive DCM (Spinozzi et al., 2020; Bruyndonckx et al., 2021). A study by Johansson et al. studied a case of a female with three consecutive pregnancies and intrauterine fetal deaths caused by a lethal form of DCM. WES of the fetus revealed homozygosity for the NEXN variant (p.Ile435SerfsTer3). Histology revealed cardiomegaly and endocardial fibroelastosis, with immunohistochemical staining using a polyclonal nexilin antibody showing loss of striation in the heart (Johansson et al., 2022).
In a study by Hass et al., NGS was used in 639 patients with DCM. Five missense NEXN variants (p.Glu110GLn, p. Gly157Val, p. Thr363Arg, p. Glu485Lys, and p. Thr666Ala) were identified in the DCM cohort. One non-sense variant (p.Arg392Ter) and one deletion (p.Glu468del) were also identified (Haas et al., 2015).
Another study by Wang et al. involved 121 patients with HCM and a missense variant (p.Gln131GLu) was identified in two patients. This variant was identified in exons five of the NEXN gene and was absent in 384 controls. Cellular transfection studies showed that the variant affecting conserved amino acid residues, led to local accumulation of nexilin. Moreover, the expressed fragment of the nexilin actin-binding domain with the (p.Gln131GLu) variant could not bind F-actin or alpha-actinin (Wang et al., 2010).
Another study examined a 11-years-old asymptomatic boy with DCM. Genetic testing revealed a heterozygous NEXN variant (p.Gly650del) inherited from his father, who was clinically characterized by mild DCM (Bruyndonckx et al., 2021). This study involved another patient who presented with left ventricular systolic dysfunction, and she required mechanical ventilation and continuous inotropic support. A few weeks later, the patient died once her therapy was discontinued. A microscopic examination of the heart revealed endomyocardial fibroelastosis. WES revealed a homozygous (p.Arg392Ter) variant. This variant was mapped to the actin-binding domain of nexilin, resulting in a premature stop codon, and non-functional nexilin protein (Bruyndonckx et al., 2021).
In a further study by Hassel et al., 1,000 patients with DCM were screened and three NEXN variants (p.Gly650del, p. Tyr652Cys, and p. Pro611Thr) were identified in nine individuals. These variants were mapped to the nexilin carboxy-terminal Ig domain. In patients with these NEXN variants, a disruption of sarcomeric units and detached Z-disks were observed. A zebrafish model was used to demonstrate the effects of these variants on atrial and ventricular chambers. Electron microscopy was performed on the zebrafish embryonic hearts having the NEXN variants (p.Tyr652Cys and p. Pro611Thr) and the results showed a disruption in the Z-disk integrity and architecture. Further, the zebrafish model was also subjected to a three bp deletion in nexilin gene (1948-1950del), leading to a loss of glycine at position 650 (p.Gly650del). Embryonic hearts of zebrafish carrying the (p.Gly650del) variant were severely dilated, with a marked reduction in systolic function (Hassel et al., 2009).
In a study by Liu et al., CRISPR/CAS9 technology was used to generate a mouse model for the same in frame deletion (p.Gly650del). Homozygous mice with the (p.Gly650del) variant exhibited a progressive DCM phenotype characterized by disorganization of the transverse-axial tubular system and a reduction in T-tubule formation, underpinning the importance of the functional NEXN gene in cardiac function and tubular system organization (Liu et al., 2020).
Therefore, the NEXN variants described (Supplementary Table S8) contribute to the further understanding of the importance of nexilin protein in cardiac contractility and function. These studies add to the current knowledge of the role of nexilin in the Z-disk and its pertubations in DCM.
3 Z-disk associated proteins
3.1 Desmin
3.1.1 Desmin structure and function
Desmin, encoded by DES on chromosome 2q35, belongs to the intermediate filament family and is found mainly in the cardiac and skeletal muscles (Hnia et al., 2015). In this review, we will be discussing desmin as a protein associated with the Z-disk. Desmin extends from the Z-disks of the superficial myofibrils to the Z-region of the costameres in the sarcolemma (Capetanaki et al., 2015). It links the contractile apparatus to the costameres of the plasma membrane (Hayman et al., 2000; Clemen et al., 2013; Capetanaki et al., 2015).
Desmin is a 53-kDa protein; it assembles as oligomers to form filaments (Vicart et al., 1996; Clemen et al., 2013). Desmin monomers, consisting of 470 amino acids, have a tripartite structure with an amino-terminal head, an amphipathic central alpha-helical rod domain consisting of a pre-coiled coil domain (PCD) followed by four segments (Coil 1A, 1B, 2A, and 2B), three short non-helical rod segments (L1, L12, and L2), and a tail domain (Figure 10) (Goldfarb and Dalakas, 2009; Capetanaki et al., 2015). The amino-terminal head is important for the proper assembly of mature desmin monomers (Brodehl et al., 2018). This assembly occurs when two desmin monomers form a dimer and two desmin dimers form a tetramer that aligns in an antiparallel fashion with two coils overlapping (Coil 1A and 1B) (Clemen et al., 2013). Eight tetramers polymerize and assemble longitudinally to form a desmin intermediate filament (Clemen et al., 2013; Capetanaki et al., 2015).
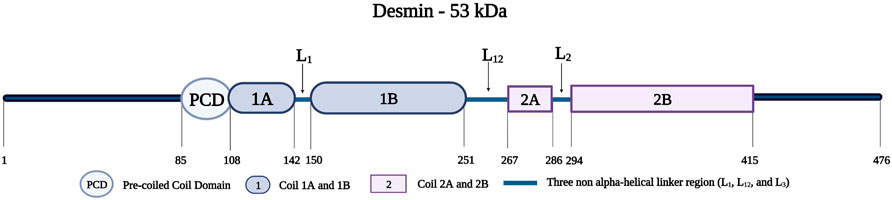
FIGURE 10. Schematic representation of the desmin protein consisting of a pre-coiled coil domain, followed by four coiled domains (Coil 1A, 1B, 2A, and 2B) that are separated by three linker regions (L1, L12, and L2). Created with BioRender.com. Adapted from (Bär et al., 2005).
Furthermore, desmin is an important cytoarchitectural protein that plays several crucial roles in maintaining the structure of myocytes and their organelles. For instance, desmin links the contractile apparatus to the cytoskeleton of the sarcolemma and various cytoplasmic organelles, including the nucleus, mitochondria, and lysosomes (Milner et al., 1999; Capetanaki et al., 2015; Dayal et al., 2020). In addition, desmin facilitates transport and mechanochemical signaling between different cell organelles and the extracellular matrix (Capetanaki et al., 2015). Desmin also plays a vital role in the maintenance of muscle contraction (Singh et al., 2020) and transduction of longitudinal stretch signals (Frank et al., 2007). Other roles include maintaining the structural and functional integrity of the cardiomyocyte structure and organizing the cytoskeleton (Goldfarb and Dalakas, 2009; Dayal et al., 2020). Furthermore, desmin interacts with mitochondria to ensure their proximity to the A- and I-bands. This interaction ensures that ATP produced in mitochondria can meet the energy requirements of the contractile apparatus through close proximity (McLendon and Robbins, 2011). A study further studied the interaction between desmin and the mitochondrial voltage-dependent anion channel (VDAC). This channel has several functions including the modulation of ATP transportation to the outer mitochondrial membrane, and controlling the transport of metabolites between the mitochondria and cytoplasm. The study used a desminopathy rat model and showed an increased accumulation of VDAC1 in areas of muscle fibers that highly stained for desmin (Li et al., 2016).
In a study by Elsnicova et al., a desmin knock-out mice model was used to assess for metabolic myocardial phenotyping. The analysis revealed a decrease in mitochondrial number, mitochondrial defects, and impaired fatty acid transport, activation and catabolism (Elsnicova et al., 2022). Another study used a desmin knock-out mouse model and demonstrated cardiomyocytes death leading to calcification and fibrosis (Milner et al., 1999).
3.1.2 Desmin and cardiomyopathies
Genetic variants in DES can lead to dysregulation of mechanochemical signaling processes in the cardiac contractile apparatus, resulting in different cardiomyopathies (Supplementary Table S9). A study by Harada et al. identified a novel homozygous DES variant (p.Thr219Pro) in a 20-year-old patient with HCM. The patient’s parents were heterozygous carriers of this variant without a cardiac phenotype, suggesting recessive inheritance. This variant was mapped to the 1B α-helix domain of desmin and was determined to be disease-causing for HCM (Harada et al., 2018). Another study reported a case of an 8-year-old girl with HCM and congenital atrioventricular heart block. A de novo heterozygous DES missense variant (p.Arg406Trp) was suggested to be disease-causing (Oka et al., 2021).
In a study by Miyamoto et al., 265 Japanese patients with DCM were examined, and a DES missense variant (p.Ile451Met) in exon eight was identified in three patients (Miyamoto et al., 2001). The same variant was studied by Li et al. in 44 patients with DCM and 460 controls. This DES variant was identified in four DCM patients and was absent in the control group. It localizes to the carboxy-terminus (Li et al., 1999). A study by Mavroidis et al. generated a mouse model expressing the same DES variant (p.Ile451Met) and showed that mutant desmin had architecture defect and an altered desmin Z-disk localization (Mavroidis et al., 2008).
In a study by Fischer et al., a large German family showing ECG abnormalities was tested using both Sanger sequencing and NGS. Two novel DES variants (p.Ile402Thr and p. Glu410Lys) were identified. Both localized to the second coil region (Coil 2B) of the rod domain. Individuals carrying these variants have DCM or ACM. Cardiomyocytes transfected with the two variants showed defects in filament assembly, resulting in desmin aggregates. The two variants were classified as disease-causing (Fischer et al., 2021).
In another study, RCM was investigated by screening for DES variants in four families (with a total of 19 individuals). Three DES variants (p.Arg16Cys, p. Thr453Ile, and p. Arg406Trp) were found in nine patients (Arbustini et al., 2006).
Another study by Herrmann et al. reported a case of an adolescent patient with RCM. A heterozygous DES variant (p.Arg406Trp) was identified by NGS. A knock-in mouse model for this variant showed aggregates of desmin and the absence of desmin filaments at the level of the intercalated discs on microscopic analysis. Other findings were structural changes within the intercalated discs, as demonstrated by the abnormal organization of desmoplakin, plectin, and connexin. In addition, cell transfection studies suggested that desmin formed unusually thick filaments organized into complex filament aggregates and fibrillar sheets. Hence, the study concludes that the DES variant (p.Arg406Trp) can cause cardiomyopathy with severe disarrangement of intercalated disks (Herrmann et al., 2020).
A study by Klauke et al. screened 22 patients with ACM and several DES variants were detected (see Supplementary Table S9). The DES variant (p.Asn116Ser) was found in one ACM patient with heart failure requiring transplantation. This variant was located to segment 1A of desmin rod domain. Immunohistochemical studies of the myocardial sections showed desmin and myotilin accumulation of immunoreactive aggresomes in both the right and left ventricle (Klauke et al., 2010). Another study by Brodehl et al. screened two families with different cardiac phenotypes including ACM, DCM, and atrioventricular block. Two novel DES variants were identified (p.Ala120Asp and p. His326Arg) and followed by in vitro experiments. The cellular model with the (p.Ala120Asp) variant showed severe intrinsic defect in filament formation causing cytoplasmic aggregates of desmin. Ex vivo analysis also showed severe cytoplasmic aggregate formation and a loss of desmin staining within the intercalated disk (Brodehl et al., 2013).
Furthermore, some studies have addressed desmin-related cardiomyopathy (DRM), defined as a rare genetic cardiac and skeletal muscle disease caused by DES variants. This disease is characterized by the combination of skeletal muscle weakness and DCM. A case report of a Japanese female with a dilated left ventricle and reduced left ventricular wall motion was studied. A disease-causing de novo missense DES variant (p.Arg454Trp) was identified (Tamiya et al., 2020). Another study by Olivé et al. included ten patients with DRM and 123 healthy controls. The DES pathogenic variant (p.Arg406Trp) was identified in two patients (Olivé et al., 2004). Another study targeted DRM by using a transgenic mouse model with a 7-amino-acid deletion (p.Arg173_Glu179del). Mice heterozygous for the variant exhibited myofibrillar disarray and left ventricular hypertrophy. Other findings included electron-dense granular and filamentous desmin aggregates located in the perinuclear region of cardiomyocytes and the intermyofibrillar space (Wang et al., 2001).
In conclusion, DES pathogenic variants are highly associated with different types of cardiomyopathies. The association of DES with DCM is the best documented (Li et al., 1999; Miyamoto et al., 2001), in addition to variants affecteing both the heart and skeletal muscle in DRM.
3.2 Obscurin
3.2.1 Structure and function of obscurin
Obscurin is encoded by OBSCN in the chromosomal region 1q42.13 (Russell et al., 2002). The protein ranges in size from 40 to 870 kDa (Young et al., 2001) and has multiple isoforms: Obscurin-A (720 kDa), Obscurin-B (870 kDa), intermediate Obscurin (260–600 kDa), and small Obscurin (40–260 kDa) (Manring et al., 2017). Obscurin A and B are abundantly expressed in both skeletal and cardiac muscle, while the smaller obscurins are located in the nucleus (Bowman et al., 2007). Obscurin consists of 88-amino acid Ig-like repeats (Nagase et al., 2000) with an amino-terminus consisting of 49 Ig domains and two fibronectin type III-like (FN3) domains (Young et al., 2001). An IQ motif and four additional Ig domains follow this region. The carboxy-terminus of obscurin contains an SH3 domain, followed by a tandem dbl homology (DH), a pleckstrin homology (PH) domain, and two further Ig repeats (Figure 11) (Young et al., 2001; Russell et al., 2002; Kontrogianni-Konstantopoulos et al., 2006). Furthermore, obscurin A and B share the same modular architecture but differ in their carboxy-termini with obscurin-A having an ankyrin binding site and obscurin-B having two active Ser/Thr kinase domains belonging to the myosin light chain kinase family (Russell et al., 2002)
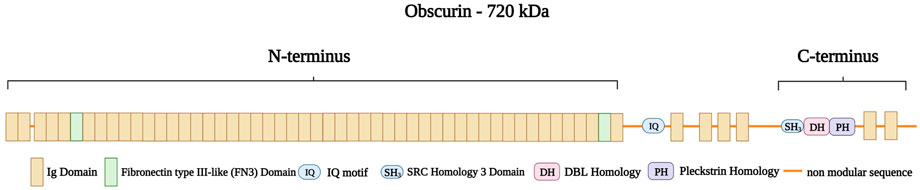
FIGURE 11. Schematic representation of obscurin A with the amino-terminus consisting of 49 Ig domains (brown) with two Fibronectin type III-like (FN3 domain, green) spanning the boundaries of the amino-terminus. This structure is followed by a non-modular sequence intersected by an IQ motif and four Ig domains. The caboxy-terminus consists of a SRC Homology three Domain (SH3), a DBL Homology domain (DH), and a Pleckstrin Homology domain (PH) followed by two Ig domains. Created with BioRender.com.
Obscurin localizes to both the Z-disk as well as to M-bands (Young et al., 2001). The obscurin Ig domains (48–49) localize to the Z-disk where they interact directly with the peripheral Z-disk Ig domains (Z9 and Z10) of titin during early development and myofibrillogenesis (Young et al., 2001). During the progression of myofibrillogenesis, obscurin is detectable at the M-band (Bang et al., 2001a; Young et al., 2001) and is implicated in the regulation of M-band assembly and structure (Benian et al., 1996). In another study, obscurin domains were found to target to the Z-disk region and were shown to interact with the titin Novex-3 isoform (Bang et al., 2001a). Obscurin also interacts with calmodulin in a calcium-dependent manner through its Ig52 domains (Young et al., 2001). It further binds to small ankyrin 1, an integral component of the sarcoplasmic reticulum (SR) membrane (Kontrogianni-Konstantopoulos et al., 2006).
Obscurin has multiple functions in facilitating myofibrillogenesis and contributing to sarcomeric organization and stabilization (Randazzo et al., 2017). Obscurin maintains sarcomere integrity and aligns the SR with the contractile apparatus (Subramaniam et al., 2022). Other roles include the maintenance and assembly of A- and M-bands (Kontrogianni-Konstantopoulos and Bloch, 2005) to transmit contractile forces across the sarcolemma (Subramaniam et al., 2022).
A study by Blondelle et al. showed that the global knockout of obscurin is embryonically lethal (Blondelle et al., 2019). Other studies have investigated the consequence of lack of obscurin on the sarcroplasmic reticulum (SR) (Lange et al., 2009; Randazzo et al., 2013). The lack of obscurin in skeletal muscles has been linked to changes in longitudinal SR architecture and sarcolemmal integrity (Lange et al., 2009; Randazzo et al., 2013). Another study by Grogan et al. used a mice model with a deletion in Ig58/59 of obsucrin. These 58 and 59 Ig domains are essential to obscurin as they mediate binding to titin. The mice model developed left ventricular hypertrophy, contractile impairment, atrial enlargement, and arrhythmia (Grogan et al., 2020).
3.2.2 Obscurin and cardiomyopathy
Several variants in OBSCN have been associated with different cardiomyopathies (Supplementary Table S10). A study by Arimura et al. included 144 Japanese patients with HCM and 288 healthy controls. Two novel OBSCN variants (p.Arg4344GLn and p. Ala4484Thr) were identified. Functional studies revealed that (p.Arg4344GLn) impairs the ability of obscurin to bind to the Z9-Z10 titin domains, thereby impairing Z-disk localization of obscurin (Arimura et al., 2007). In another study by Hu et al., a knock-in mouse model with the same OBSCN variant (p.Arg4344GLn) was investigated, but did not reveal any cardiac pathology (Hu and Kontrogianni-Konstantopoulos, 2020). In contrast, a study by Fukuzawa et al. used a different knock-in mouse model for the same variant (p.Arg4344GLn) and described a cardiomyopathy-like phenotype with abnormal Ca2+handling. However, no changes in the thermostability or binding of obscurin to Z9-Z10 titin were detected (Fukuzawa et al., 2021). A further knock-in mouse model for this variant (p.Arg4344GLn) showed that the expression patterns of the sarcomeric and Ca2+ cycling proteins were unchanged. However, isolated cardiomyocytes from a 1-year-old knock-in hearts exhibit an increase in Ca2+ transients in the SR, as well as faster contraction kinetics (Hu et al., 2017).
In a study by Marston et al., WES was performed on 30 patients with DCM, and five potentially disease-causing OBSCN variants (p.Glu963Lys, p. Val2161Asp, p. Phe2809Val, p. Asp5966Asn, and p. Arg4856His) were identified in four patients (Marston et al., 2015). Rowland et al. studied a cohort of 325 DCM and 10 LVNC patients. Two OBSCN variants (p.Thr7266ArgfsTer53 and p. Ser7947ProfsTer82) were identified in two LVNC patients and one OBSCN variant (p.Ala7950ProfsTer79) was identified in one DCM patient (Rowland et al., 2016). In a study by Xu et al., a cohort of 74 patients with HCM was studied. WES was performed and two OBSCN missense variants (p.Arg5215His, and p. Gly7500Arg) and four frameshift variants (p. Ala996fs, p. Ala1088fs, p. Ala1272fs, and p. Ala1640fs) were identified (Xu et al., 2015).
A further study by Chen et al. generated iPSC-CMs from a 46-year-old female diagnosed with ACM, carrying a frameshift variant in OBSCN (p.Leu5218fs). These cells showed impaired localization and decreased expression of the obscurin anchoring protein (Ank1.5), supporting a pathogenic role of this variant (Chen et al., 2020).
In conclusion, most of the documented OBSCN variants are associated with HCM and DCM. However, the effect of these variants on Z-disk assembly and cardiac contractility have not been well elucidated.
4 Conclusion
As discussed in the review, Z-disk proteins and Z-disk-associated proteins play a critical role in maintaining the structure and function of the cardiac contractile apparatus. The interaction of these different proteins within the Z-disk allows the structure to perform its role as a signaling hub where it transmits mechanical and biochemical signals (Frank and Frey, 2011; Filomena et al., 2021). A large number of pathogenic variants of the Z-disk proteins have been shown to associate with several types of cardiomyopathies. These genetic variants lead to disruption or inactivation of the corresponding proteins thus advancing research on rare cardiomyopathies by providing a rich source of information on disease phenotypes (Karczewski et al., 2020).
Over the past decade, the advancement and wide application of high-throughput sequencing technologies have made genetic analyses more accessible and accurate (Karczewski et al., 2020). In addition, sequencing of normal cohorts has revealed insight into the surprisingly large genetic variation not associated with disease (GnomAD, 2014). Hence, minor allelic frequency (MAF) cut-offs have become more stringent for monogenetic disease, with a MAF threshold of less than 1 × 10−4 for autosomal dominant cardiomyopathies (Walsh et al., 2017). This will facilitate the detection of disease-causing variants and will allow earlier disease diagnosis and timely treatment.
Our increasing knowledge about genetic variant in diseased and normal cohorts as well as biological functions of proteins also means that not all genetic variants historically reported would still be considered pathogenic today. This is also reflected in a more nuanced classification of the likelihood of variants to be pathogenic (Richards et al., 2015).
Furthermore, an important focus in genetic studies is the distinction between monogenic and polygenic risk variants. Monogenic risk variants are defined as a single variant that disrupt a physiological pathway with a major impact on the disease phenotype (Fahed et al., 2020). In contrast, polygenic risk includes multiple variants with small effect in different pathways (Fahed et al., 2020). Recent studies have shown that combinations of common variants with small effect sizes can cause HCM and DCM that is phenotypically similar to monogenic disease in the affected individual (Harper et al., 2021; Tadros et al., 2021). It is important to analyze both monogenic risk and polygenic risk variants (Walsh et al., 2017), however, very few studies have examined the interplay between them.
Another important focus is to differentiate between disease-causing and disease-modifying variants. A disease modifier is defined as a newly-identified variant of unknown significance that can modify the disease phenotypic outcome (Nollet et al., 2020). Such disease modifying variants may show clear functional changes to protein characteristics (e.g., stability or ligand binding), but have high prevalence in normal cohorts which contradicts them as the sole genetic cause for disease, as it would imply an implausible low penetrance (Ye et al., 2019).
A prime example is a variant in NEBL (p.Gly202Arg) which was initially described as disease-causing based on the phenotype of a mouse model (Purevjav et al., 2010), however it has a high MAF value (2.3 × 10−3), which is too high to be considered disease-causing.
Another example is CSRP3 variant (p.Trp4Arg). It was identified by Knöll et al. in a study involving 1400 individuals divided into control and DCM patients. The CSRP3 missense variant (p.Trp4Arg) impair binding to telethonin, leading to Z-disk misalignment, and eventually DCM (Knöll et al., 2002).
A study by Riaz et al. generated cellular models (iPSC-CMs and engineered heart tissues (EHTs) with the same (p.Trp4Arg) variant. The derived cardiomyocytes showed a higher decay rate in the muscle LIM protein expression, and the derived EHTs showed impaired relaxation and prolonged force generation (Riaz et al., 2022). Despite this variant showing clear phenotypic changes in clinical studies, iPSC-CM cultures and engineered heart tissues; it has an implausible high MAF value (2.28 × 10−3) and now considered a disease modifier (Knöll et al., 2010).
Moreover, genetic variants may predispose different responses to environmental factors. It is emerging that genetic variants in TTN are enriched in cohorts of cardiac disease caused by pregnancy, alcohol abuse or cancer therapy (Ware et al., 2016; Ware et al., 2018; Garcia-Pavia et al., 2019), suggesting that an aggravated response to an environmental trigger could be caused by the presence of the TTN variant.
Finally, the field of genetic analysis field is very broad, and new bioinformatics and experimental approaches are needed to facilitate analysis of the pathogenicity of different variants in the future. The continued development and improvement of iPSC-CMs is an exciting trend for disease modelling [reviewed in (Reilly et al., 2022)], also of Z-disk associated cardiomyopathies, and will complement whole organ investigations in animal models [reviewed in (Sheikh and Chen, 2007)] to allow better insights into disease mechanisms.
Author contributions
Both authors have made a substantial and direct contribution to the work. Both authors have read and agreed to the published version of the manuscript.
Funding
This work is supported by the Advanced Inter-Disciplinary Models (AIM) Doctoral Training Partnership (DTP) funded by the Medical Research Council (MR/W007002/1) to KG and MN KG is supported by the Medical Research Council (MR/V009540/1). The Institute of Cardiovascular Sciences, University of Birmingham, has received an Accelerator Award by the British Heart Foundation (AA/18/2/34218).
Conflict of interest
The authors declare that the research was conducted in the absence of any commercial or financial relationships that could be construed as a potential conflict of interest.
Publisher’s note
All claims expressed in this article are solely those of the authors and do not necessarily represent those of their affiliated organizations, or those of the publisher, the editors and the reviewers. Any product that may be evaluated in this article, or claim that may be made by its manufacturer, is not guaranteed or endorsed by the publisher.
Supplementary material
The Supplementary Material for this article can be found online at: https://www.frontiersin.org/articles/10.3389/fphys.2023.1143858/full#supplementary-material
References
Ader, F., De Groote, P., Réant, P., Rooryck-Thambo, C., Dupin-Deguine, D., Rambaud, C., et al. (2019). FLNC pathogenic variants in patients with cardiomyopathies: Prevalence and genotype-phenotype correlations. Clin. Genet. 96 (4), 317–329. doi:10.1111/cge.13594
Agarwal, R., Paulo, J. A., Toepfer, C. N., Ewoldt, J. K., Sundaram, S., Chopra, A., et al. (2021). Filamin C cardiomyopathy variants cause protein and lysosome accumulation. Circ. Res. 129 (7), 751–766. doi:10.1161/CIRCRESAHA.120.317076
Aherrahrou, Z., Schlossarek, S., Stoelting, S., Klinger, M., Geertz, B., Weinberger, F., et al. (2016). Knock-out of nexilin in mice leads to dilated cardiomyopathy and endomyocardial fibroelastosis. Basic Res. Cardiol. 111 (1), 6. doi:10.1007/s00395-015-0522-5
Al-Qusairi, L., and Laporte, J. (2011). T-tubule biogenesis and triad formation in skeletal muscle and implication in human diseases. Skelet. Muscle 1 (1), 26. doi:10.1186/2044-5040-1-26
Anand, P. P. (2019). Computational modelling of human Sarcomeric Telethonin protein and predicting the functional effect of missense single nucleotide polymorphsim. Curr. Sci. 117 (4), 638–648. doi:10.18520/cs/v117/i4/638-648
Arber, S., Hunter, J. J., Ross, J., Hongo, M., Sansig, G., Borg, J., et al. (1997). MLP-deficient mice exhibit a disruption of cardiac cytoarchitectural organization, dilated cardiomyopathy, and heart failure. Cell. 88 (3), 393–403. doi:10.1016/s0092-8674(00)81878-4
Arbustini, E., Pasotti, M., Pilotto, A., Pellegrini, C., Grasso, M., Previtali, S., et al. (2006). Desmin accumulation restrictive cardiomyopathy and atrioventricular block associated with desmin gene defects. Eur. J. Heart Fail 8 (5), 477–483. doi:10.1016/j.ejheart.2005.11.003
Arimura, T., Matsumoto, Y., Okazaki, O., Hayashi, T., Takahashi, M., Inagaki, N., et al. (2007). Structural analysis of obscurin gene in hypertrophic cardiomyopathy. Biochem. Biophys. Res. Commun. 362 (2), 281–287. doi:10.1016/j.bbrc.2007.07.183
Arimura, T., Nakamura, T., Hiroi, S., Satoh, M., Takahashi, M., Ohbuchi, N., et al. (2000). Characterization of the human nebulette gene: A polymorphism in an actin-binding motif is associated with nonfamilial idiopathic dilated cardiomyopathy. Hum. Genet. 107 (5), 440–451. doi:10.1007/s004390000389
Atkinson, R. A., Joseph, C., Dal Piaz, F., Birolo, L., Stier, G., Pucci, P., et al. (2000). Binding of alpha-actinin to titin: Implications for Z-disk assembly. Biochemistry 39 (18), 5255–5264. doi:10.1021/bi991891u
Au, Y. (2004). The muscle ultrastructure: A structural perspective of the sarcomere. Cell. Mol. Life Sci. 61 (24), 3016–3033. doi:10.1007/s00018-004-4282-x
Aufiero, S., van den Hoogenhof, M. M. G., Reckman, Y. J., Beqqali, A., van der Made, I., Kluin, J., et al. (2018). Cardiac circRNAs arise mainly from constitutive exons rather than alternatively spliced exons. Rna 24 (6), 815–827. doi:10.1261/rna.064394.117
Augusto, J. B., Eiros, R., Nakou, E., Moura-Ferreira, S., Treibel, T. A., Captur, G., et al. (2020). Dilated cardiomyopathy and arrhythmogenic left ventricular cardiomyopathy: A comprehensive genotype-imaging phenotype study. Eur. Heart J. Cardiovasc Imaging 21 (3), 326–336. doi:10.1093/ehjci/jez188
Avnika, R., Raquel, V., and Robert, B.-R. (2012). “Myofibrillar myopathies and the Z-disk associated proteins,” in Skeletal muscle. Editor C. Julianna (Rijeka: IntechOpen). Ch. 14.
Bagnall, R. D., Molloy, L. K., Kalman, J. M., and Semsarian, C. (2014). Exome sequencing identifies a mutation in the ACTN2 gene in a family with idiopathic ventricular fibrillation, left ventricular noncompaction, and sudden death. BMC Med. Genet. 15, 99. doi:10.1186/s12881-014-0099-0
Bang, M. L., Centner, T., Fornoff, F., Geach, A. J., Gotthardt, M., McNabb, M., et al. (2001a). The complete gene sequence of titin, expression of an unusual approximately 700-kDa titin isoform, and its interaction with obscurin identify a novel Z-line to I-band linking system. Circ. Res. 89 (11), 1065–1072. doi:10.1161/hh2301.100981
Bang, M. L., and Chen, J. (2015). Roles of nebulin family members in the heart. Circ. J. 79 (10), 2081–2087. doi:10.1253/circj.CJ-15-0854
Bang, M. L., Mudry, R. E., McElhinny, A. S., Trombitás, K., Geach, A. J., Yamasaki, R., et al. (2001b). Myopalladin, a novel 145-kilodalton sarcomeric protein with multiple roles in Z-disc and I-band protein assemblies. J. Cell. Biol. 153 (2), 413–427. doi:10.1083/jcb.153.2.413
Bañuelos, S., Saraste, M., and Djinović Carugo, K. (1998). Structural comparisons of calponin homology domains: Implications for actin binding. Structure 6 (11), 1419–1431. doi:10.1016/s0969-2126(98)00141-5
Bär, H., Mücke, N., Kostareva, A., Sjöberg, G., Aebi, U., and Herrmann, H. (2005). Severe muscle disease-causing desmin mutations interfere with in vitro filament assembly at distinct stages. Proc. Natl. Acad. Sci. U. S. A. 102 (42), 15099–15104. doi:10.1073/pnas.0504568102
Begay, R. L., Graw, S. L., Sinagra, G., Asimaki, A., Rowland, T. J., Slavov, D. B., et al. (2018). Filamin C truncation mutations are associated with arrhythmogenic dilated cardiomyopathy and changes in the cell-cell adhesion structures. JACC Clin. Electrophysiol. 4 (4), 504–514. doi:10.1016/j.jacep.2017.12.003
Begay, R. L., Tharp, C. A., Martin, A., Graw, S. L., Sinagra, G., Miani, D., et al. (2016). FLNC gene splice mutations cause dilated cardiomyopathy. JACC Basic Transl. Sci. 1 (5), 344–359. doi:10.1016/j.jacbts.2016.05.004
Benian, G. M., Tinley, T. L., Tang, X., and Borodovsky, M. (1996). The Caenorhabditis elegans gene unc-89, required fpr muscle M-line assembly, encodes a giant modular protein composed of Ig and signal transduction domains. J. Cell. Biol. 132 (5), 835–848. doi:10.1083/jcb.132.5.835
Bertz, M., Wilmanns, M., and Rief, M. (2009). The titin-telethonin complex is a directed, superstable molecular bond in the muscle Z-disk. Proc. Natl. Acad. Sci. U. S. A. 106 (32), 13307–133310. doi:10.1073/pnas.0902312106
Blondelle, J., Marrocco, V., Clark, M., Desmond, P., Myers, S., Nguyen, J., et al. (2019). Murine obscurin and Obsl1 have functionally redundant roles in sarcolemmal integrity, sarcoplasmic reticulum organization, and muscle metabolism. Commun. Biol. 2, 178. doi:10.1038/s42003-019-0405-7
Bos, J. M., Poley, R. N., Ny, M., Tester, D. J., Xu, X., Vatta, M., et al. (2006). Genotype-phenotype relationships involving hypertrophic cardiomyopathy-associated mutations in titin, muscle LIM protein, and telethonin. Mol. Genet. Metab. 88 (1), 78–85. doi:10.1016/j.ymgme.2005.10.008
Bowman, A. L., Kontrogianni-Konstantopoulos, A., Hirsch, S. S., Geisler, S. B., Gonzalez-Serratos, H., Russell, M. W., et al. (2007). Different obscurin isoforms localize to distinct sites at sarcomeres. FEBS Lett. 581 (8), 1549–1554. doi:10.1016/j.febslet.2007.03.011
Brodehl, A., Dieding, M., Klauke, B., Dec, E., Madaan, S., Huang, T., et al. (2013). The novel desmin mutant p.A120D impairs filament formation, prevents intercalated disk localization, and causes sudden cardiac death. Circ. Cardiovasc Genet. 6 (6), 615–623. doi:10.1161/CIRCGENETICS.113.000103
Brodehl, A., Ferrier, R. A., Hamilton, S. J., Greenway, S. C., Brundler, M. A., Yu, W., et al. (2016). Mutations in FLNC are associated with familial restrictive cardiomyopathy. Hum. Mutat. 37 (3), 269–279. doi:10.1002/humu.22942
Brodehl, A., Gaertner-Rommel, A., and Milting, H. (2017). FLNC (filamin-C): A new(er) player in the field of genetic cardiomyopathies. Circ. Cardiovasc Genet. 10 (6), e001959. doi:10.1161/circgenetics.117.001959(
Brodehl, A., Gaertner-Rommel, A., and Milting, H. (2018). Molecular insights into cardiomyopathies associated with desmin (DES) mutations. Biophys. Rev. 10 (4), 983–1006. doi:10.1007/s12551-018-0429-0
Brun, F., Gigli, M., Graw, S. L., Judge, D. P., Merlo, M., Murray, B., et al. (2020). FLNC truncations cause arrhythmogenic right ventricular cardiomyopathy. J. Med. Genet. 57 (4), 254–257. doi:10.1136/jmedgenet-2019-106394
Bruyndonckx, L., Vogelzang, J. L., Bugiani, M., Straver, B., Kuipers, I. M., Onland, W., et al. (2021). Childhood onset nexilin dilated cardiomyopathy: A heterozygous and a homozygous case. Am. J. Med. Genet. A 185 (8), 2464–2470. doi:10.1002/ajmg.a.62231
Buyandelger, B., Ng, K. E., Miocic, S., Piotrowska, I., Gunkel, S., Ku, C. H., et al. (2011). MLP (muscle LIM protein) as a stress sensor in the heart. Pflugers Arch. 462 (1), 135–142. doi:10.1007/s00424-011-0961-2
Capetanaki, Y., Papathanasiou, S., Diokmetzidou, A., Vatsellas, G., and Tsikitis, M. (2015). Desmin related disease: A matter of cell survival failure. Curr. Opin. Cell. Biol. 32, 113–120. doi:10.1016/j.ceb.2015.01.004
Celeghin, R., Cipriani, A., Bariani, R., Bueno Marinas, M., Cason, M., Bevilacqua, M., et al. (2022). Filamin-C variant-associated cardiomyopathy: A pooled analysis of individual patient data to evaluate the clinical profile and risk of sudden cardiac death. Heart Rhythm. 19 (2), 235–243. doi:10.1016/j.hrthm.2021.09.029
Chanavat, V., Janin, A., and Millat, G. (2016). A fast and cost-effective molecular diagnostic tool for genetic diseases involved in sudden cardiac death. Clin. Chim. Acta 453, 80–85. doi:10.1016/j.cca.2015.12.011
Chauhan, P. K., and Sowdhamini, R. (2022). LIM domain-wide comprehensive virtual mutagenesis provides structural rationale for cardiomyopathy mutations in CSRP3. Sci. Rep. 12 (1), 3562. doi:10.1038/s41598-022-07553-1
Chauveau, C., Rowell, J., and Ferreiro, A. (2014). A rising titan: TTN review and mutation update. Hum. Mutat. 35 (9), 1046–1059. doi:10.1002/humu.22611
Chen, P., Xiao, Y., Wang, Y., Zheng, Z., Chen, L., Yang, X., et al. (2020). Intracellular calcium current disorder and disease phenotype in OBSCN mutant iPSC-based cardiomyocytes in arrhythmogenic right ventricular cardiomyopathy. Theranostics 10 (24), 11215–11229. doi:10.7150/thno.45172
Chen, S. N., Lam, C. K., Wan, Y. W., Gao, S., Malak, O. A., Zhao, S. R., et al. (2022). Activation of PDGFRA signaling contributes to filamin C-related arrhythmogenic cardiomyopathy. Sci. Adv. 8, eabk0052. doi:10.1126/sciadv.abk0052
Chiu, C., Bagnall, R. D., Ingles, J., Yeates, L., Kennerson, M., Donald, J. A., et al. (2010). Mutations in alpha-actinin-2 cause hypertrophic cardiomyopathy: A genome-wide analysis. J. Am. Coll. Cardiol. 55 (11), 1127–1135. doi:10.1016/j.jacc.2009.11.016
Clark, K. A., McElhinny, A. S., Beckerle, M. C., and Gregorio, C. C. (2002). Striated muscle cytoarchitecture: An intricate web of form and function. Annu. Rev. Cell. Dev. Biol. 18, 637–706. doi:10.1146/annurev.cellbio.18.012502.105840
Clemen, C. S., Herrmann, H., Strelkov, S. V., and Schröder, R. (2013). Desminopathies: Pathology and mechanisms. Acta Neuropathol. 125 (1), 47–75. doi:10.1007/s00401-012-1057-6
Corrado, D., and Zorzi, A. (2018). Filamin C: A new arrhythmogenic cardiomyopathy-causing gene? JACC Clin. Electrophysiol. 4 (4), 515–517. doi:10.1016/j.jacep.2018.01.004
Critchley, D. R. (2000). Focal adhesions - the cytoskeletal connection. Curr. Opin. Cell. Biol. 12 (1), 133–139. doi:10.1016/s0955-0674(99)00067-8
Cukovic, D., Lu, G. W., Wible, B., Steele, D. F., and Fedida, D. (2001). A discrete amino terminal domain of Kv1.5 and Kv1.4 potassium channels interacts with the spectrin repeats of alpha-actinin-2. FEBS Lett. 498 (1), 87–92. doi:10.1016/s0014-5793(01)02505-4
Dayal, A. A., Medvedeva, N. V., Nekrasova, T. M., Duhalin, S. D., Surin, A. K., and Minin, A. A. (2020). Desmin interacts directly with mitochondria. Int. J. Mol. Sci. 21, 8122. doi:10.3390/ijms21218122
Dixson, J. D., Forstner, M. J., and Garcia, D. M. (2003). The alpha-actinin gene family: A revised classification. J. Mol. Evol. 56 (1), 1–10. doi:10.1007/s00239-002-2374-5
Duboscq-Bidot, L., Xu, P., Charron, P., Neyroud, N., Dilanian, G., Millaire, A., et al. (2008). Mutations in the Z-band protein myopalladin gene and idiopathic dilated cardiomyopathy. Cardiovasc Res. 77 (1), 118–125. doi:10.1093/cvr/cvm015
Ehsan, M., Jiang, H., Thomson, K. L., and Gehmlich, K. (2017). When signalling goes wrong: Pathogenic variants in structural and signalling proteins causing cardiomyopathies. J. Muscle Res. Cell. Motil. 38 (3-4), 303–316. doi:10.1007/s10974-017-9487-3
Ehsan, M., Kelly, M., Hooper, C., Yavari, A., Beglov, J., Bellahcene, M., et al. (2018). Mutant Muscle LIM Protein C58G causes cardiomyopathy through protein depletion. J. Mol. Cell. Cardiol. 121, 287–296. doi:10.1016/j.yjmcc.2018.07.248
Elsnicova, B., Hornikova, D., Tibenska, V., Kolar, D., Tlapakova, T., Schmid, B., et al. (2022). Desmin knock-out cardiomyopathy: A heart on the verge of metabolic crisis. Int. J. Mol. Sci. 23, 12020. doi:10.3390/ijms231912020
Esham, M., Bryan, K., Milnes, J., Holmes, W. B., and Moncman, C. L. (2007). Expression of nebulette during early cardiac development. Cell. Motil. Cytoskelet. 64 (4), 258–273. doi:10.1002/cm.20180
Fahed, A. C., Wang, M., Homburger, J. R., Patel, A. P., Bick, A. G., Neben, C. L., et al. (2020). Polygenic background modifies penetrance of monogenic variants for tier 1 genomic conditions. Nat. Commun. 11 (1), 3635. doi:10.1038/s41467-020-17374-3
Fan, L. L., Huang, H., Jin, J. Y., Li, J. J., Chen, Y. Q., and Xiang, R. (2019). Whole-exome sequencing identifies a novel mutation (p.L320R) of alpha-actinin 2 in a Chinese family with dilated cardiomyopathy and ventricular tachycardia. Cytogenet Genome Res. 157 (3), 148–152. doi:10.1159/000496077
Faulkner, G., Lanfranchi, G., and Valle, G. (2001). Telethonin and other new proteins of the Z-disc of skeletal muscle. IUBMB Life 51 (5), 275–282. doi:10.1080/152165401317190761
Feng, Y., and Walsh, C. A. (2004). The many faces of filamin: A versatile molecular scaffold for cell motility and signalling. Nat. Cell. Biol. 6 (11), 1034–1038. doi:10.1038/ncb1104-1034
Filomena, M. C., Yamamoto, D. L., Caremani, M., Kadarla, V. K., Mastrototaro, G., Serio, S., et al. (2020). Myopalladin promotes muscle growth through modulation of the serum response factor pathway. J. Cachexia Sarcopenia Muscle 11 (1), 169–194. doi:10.1002/jcsm.12486
Filomena, M. C., Yamamoto, D. L., Carullo, P., Medvedev, R., Ghisleni, A., Piroddi, N., et al. (2021). Myopalladin knockout mice develop cardiac dilation and show a maladaptive response to mechanical pressure overload. Elife 10, e58313. doi:10.7554/eLife.58313
Fischer, B., Dittmann, S., Brodehl, A., Unger, A., Stallmeyer, B., Paul, M., et al. (2021). Functional characterization of novel alpha-helical rod domain desmin (DES) pathogenic variants associated with dilated cardiomyopathy, atrioventricular block and a risk for sudden cardiac death. Int. J. Cardiol. 329, 167–174. doi:10.1016/j.ijcard.2020.12.050
Foley, K. S., and Young, P. W. (2014). The non-muscle functions of actinins: An update. Biochem. J. 459 (1), 1–13. doi:10.1042/BJ20131511
Frank, D., and Frey, N. (2011). Cardiac Z-disc signaling network. J. Biol. Chem. 286 (12), 9897–9904. doi:10.1074/jbc.R110.174268
Frank, D., Kuhn, C., Katus, H. A., and Frey, N. (2007). Role of the sarcomeric Z-disc in the pathogenesis of cardiomyopathy. Future Cardiol. 3 (6), 611–622. doi:10.2217/14796678.3.6.611
Fukuzawa, A., Koch, D., Grover, S., Rees, M., and Gautel, M. (2021). When is an obscurin variant pathogenic? The impact of Arg4344Gln and Arg4444Trp variants on protein-protein interactions and protein stability. Hum. Mol. Genet. 30 (12), 1131–1141. doi:10.1093/hmg/ddab010
Fung, Y. W., Wang, R. X., Heng, H. H., and Liew, C. C. (1995). Mapping of a human LIM protein (CLP) to human chromosome 11p15.1 by fluorescence in situ hybridization. Genomics 28 (3), 602–603. doi:10.1006/geno.1995.1200
Fürst, D. O., Goldfarb, L. G., Kley, R. A., Vorgerd, M., Olivé, M., and van der Ven, P. F. (2013). Filamin C-related myopathies: Pathology and mechanisms. Acta Neuropathol. 125 (1), 33–46. doi:10.1007/s00401-012-1054-9
Fürst, D. O., Osborn, M., Nave, R., and Weber, K. (1988). The organization of titin filaments in the half-sarcomere revealed by monoclonal antibodies in immunoelectron microscopy: A map of ten nonrepetitive epitopes starting at the Z line extends close to the M line. J. Cell. Biol. 106 (5), 1563–1572. doi:10.1083/jcb.106.5.1563
Garcia-Pavia, P., Kim, Y., Restrepo-Cordoba, M. A., Lunde, I. G., Wakimoto, H., Smith, A. M., et al. (2019). Genetic variants associated with cancer therapy-induced cardiomyopathy. Circulation 140 (1), 31–41. doi:10.1161/CIRCULATIONAHA.118.037934
Gardiwal, A., Klein, G., Kraemer, K., Durgac, T., Koenig, T., Niehaus, M., et al. (2007). Reduced delayed rectifier K+ current, altered electrophysiology, and increased ventricular vulnerability in MLP-deficient mice. J. Card. Fail 13 (8), 687–693. doi:10.1016/j.cardfail.2007.04.015
Gariboldi, M., Maestrini, E., Canzian, F., Manenti, G., De Gregorio, L., Rivella, S., et al. (1994). Comparative mapping of the actin-binding protein 280 genes in human and mouse. Genomics 21 (2), 428–430. doi:10.1006/geno.1994.1288
Gautel, M., Goulding, D., Bullard, B., Weber, K., and Fürst, D. O. (1996). The central Z-disk region of titin is assembled from a novel repeat in variable copy numbers. J. Cell. Sci. 109, 2747–2754. doi:10.1242/jcs.109.11.2747
Geier, C., Gehmlich, K., Ehler, E., Hassfeld, S., Perrot, A., Hayess, K., et al. (2008). Beyond the sarcomere: CSRP3 mutations cause hypertrophic cardiomyopathy. Hum. Mol. Genet. 17 (18), 2753–2765. doi:10.1093/hmg/ddn160
Geier, C., Perrot, A., Ozcelik, C., Binner, P., Counsell, D., Hoffmann, K., et al. (2003). Mutations in the human muscle LIM protein gene in families with hypertrophic cardiomyopathy. Circulation 107 (10), 1390–1395. doi:10.1161/01.cir.0000056522.82563.5f
Gigli, M., Begay, R. L., Morea, G., Graw, S. L., Sinagra, G., Taylor, M. R., et al. (2016). A review of the giant protein titin in clinical molecular diagnostics of cardiomyopathies. Front. Cardiovasc Med. 3, 21. doi:10.3389/fcvm.2016.00021
Girolami, F., Iascone, M., Tomberli, B., Bardi, S., Benelli, M., Marseglia, G., et al. (2014). Novel α-actinin 2 variant associated with familial hypertrophic cardiomyopathy and juvenile atrial arrhythmias: A massively parallel sequencing study. Circ. Cardiovasc Genet. 7 (6), 741–750. doi:10.1161/CIRCGENETICS.113.000486
GnomAD (2014). Genome aggregation database. Available at: https://gnomad.broadinstitute.org/.
Goldfarb, L. G., and Dalakas, M. C. (2009). Tragedy in a heartbeat: Malfunctioning desmin causes skeletal and cardiac muscle disease. J. Clin. Invest. 119 (7), 1806–1813. doi:10.1172/JCI38027
Gómez, J., Lorca, R., Reguero, J. R., Morís, C., Martín, M., Tranche, S., et al. (2017). Screening of the filamin C gene in a large cohort of hypertrophic cardiomyopathy patients. Circ. Cardiovasc Genet. 10, e001584. doi:10.1161/CIRCGENETICS.116.001584
Good, J. M., Fellmann, F., Bhuiyan, Z. A., Rotman, S., Pruvot, E., and Schläpfer, J. (2020). ACTN2 variant associated with a cardiac phenotype suggestive of left-dominant arrhythmogenic cardiomyopathy. Hear. Case Rep. 6 (1), 15–19. doi:10.1016/j.hrcr.2019.10.001
Granzier, H., and Labeit, S. (2002). Cardiac titin: An adjustable multi-functional spring. J. Physiol. 541, 335–342. doi:10.1113/jphysiol.2001.014381
Granzier, H. L., and Labeit, S. (2004). The giant protein titin: A major player in myocardial mechanics, signaling, and disease. Circ. Res. 94 (3), 284–295. doi:10.1161/01.RES.0000117769.88862.F8
Granzier, H. L., Radke, M. H., Peng, J., Westermann, D., Nelson, O. L., Rost, K., et al. (2009). Truncation of titin's elastic PEVK region leads to cardiomyopathy with diastolic dysfunction. Circ. Res. 105 (6), 557–564. doi:10.1161/CIRCRESAHA.109.200964
Gregorio, C. C., Trombitás, K., Centner, T., Kolmerer, B., Stier, G., Kunke, K., et al. (1998). The NH2 terminus of titin spans the Z-disc: Its interaction with a novel 19-kD ligand (T-cap) is required for sarcomeric integrity. J. Cell. Biol. 143 (4), 1013–1027. doi:10.1083/jcb.143.4.1013
Groeneweg, J. A., van der Heijden, J. F., Dooijes, D., van Veen, T. A., van Tintelen, J. P., and Hauer, R. N. (2014). Arrhythmogenic cardiomyopathy: Diagnosis, genetic background, and risk management. Neth Heart J. 22 (7-8), 316–325. doi:10.1007/s12471-014-0563-7
Grogan, A., Coleman, A., Joca, H., Granzier, H., Russel, M. W., Ward, C. W., et al. (2020). Deletion of obscurin immunoglobulin domains Ig58/59 leads to age-dependent cardiac remodeling and arrhythmia. Basic Res. Cardiol. 115 (6), 60. doi:10.1007/s00395-020-00818-8
Guo, Y., Cao, Y., Jardin, B. D., Sethi, I., Ma, Q., Moghadaszadeh, B., et al. (2021). Sarcomeres regulate murine cardiomyocyte maturation through MRTF-SRF signaling. Proc. Natl. Acad. Sci. U. S. A. 118, e2008861118. doi:10.1073/pnas.2008861118
Haas, J., Frese, K. S., Peil, B., Kloos, W., Keller, A., Nietsch, R., et al. (2015). Atlas of the clinical genetics of human dilated cardiomyopathy. Eur. Heart J. 36 (18), 1123–135a. doi:10.1093/eurheartj/ehu301
Hall, C. L., Akhtar, M. M., Sabater-Molina, M., Futema, M., Asimaki, A., Protonotarios, A., et al. (2020). Filamin C variants are associated with a distinctive clinical and immunohistochemical arrhythmogenic cardiomyopathy phenotype. Int. J. Cardiol. 307, 101–108. doi:10.1016/j.ijcard.2019.09.048
Harada, H., Hayashi, T., Nishi, H., Kusaba, K., Koga, Y., Koga, Y., et al. (2018). Phenotypic expression of a novel desmin gene mutation: Hypertrophic cardiomyopathy followed by systemic myopathy. J. Hum. Genet. 63 (2), 249–254. doi:10.1038/s10038-017-0383-x
Harper, A. R., Goel, A., Grace, C., Thomson, K. L., Petersen, S. E., Xu, X., et al. (2021). Common genetic variants and modifiable risk factors underpin hypertrophic cardiomyopathy susceptibility and expressivity. Nat. Genet. 53 (2), 135–142. doi:10.1038/s41588-020-00764-0
Harper, B. D., Beckerle, M. C., and Pomiès, P. (2000). Fine mapping of the alpha-actinin binding site within cysteine-rich protein. Biochem. J. 1, 269–274. doi:10.1042/bj3500269
Hassel, D., Dahme, T., Erdmann, J., Meder, B., Huge, A., Stoll, M., et al. (2009). Nexilin mutations destabilize cardiac Z-disks and lead to dilated cardiomyopathy. Nat. Med. 15 (11), 1281–1288. doi:10.1038/nm.2037
Hastings, R., de Villiers, C. P., Hooper, C., Ormondroyd, L., Pagnamenta, A., Lise, S., et al. (2016). Combination of whole genome sequencing, linkage, and functional studies implicates a missense mutation in titin as a cause of autosomal dominant cardiomyopathy with features of left ventricular noncompaction. Circ. Cardiovasc Genet. 9 (5), 426–435. doi:10.1161/CIRCGENETICS.116.001431
Hayashi, T., Arimura, T., Itoh-Satoh, M., Ueda, K., Hohda, S., Inagaki, N., et al. (2004). Tcap gene mutations in hypertrophic cardiomyopathy and dilated cardiomyopathy. J. Am. Coll. Cardiol. 44 (11), 2192–2201. doi:10.1016/j.jacc.2004.08.058
Hayman, R., Une, Y., and Nomura, Y. (2000). Desmin as a possible immunohistochemical marker for feline hypertrophic cardiomyopathy. J. Vet. Med. Sci. 62 (3), 343–346. doi:10.1292/jvms.62.343
Haywood, N. J., Wolny, M., Rogers, B., Trinh, C. H., Shuping, Y., Edwards, T. A., et al. (2016). Hypertrophic cardiomyopathy mutations in the calponin-homology domain of ACTN2 affect actin binding and cardiomyocyte Z-disc incorporation. Biochem. J. 473 (16), 2485–2493. doi:10.1042/BCJ20160421
Hein, S., Block, T., Zimmermann, R., Kostin, S., Scheffold, T., Kubin, T., et al. (2009). Deposition of nonsarcomeric alpha-actinin in cardiomyocytes from patients with dilated cardiomyopathy or chronic pressure overload. Exp. Clin. Cardiol. 14 (3), e68–e75.
Heineke, J., Wollert, K. C., Osinska, H., Sargent, M. A., York, A. J., Robbins, J., et al. (2010). Calcineurin protects the heart in a murine model of dilated cardiomyopathy. J. Mol. Cell. Cardiol. 48 (6), 1080–1087. doi:10.1016/j.yjmcc.2009.10.012
Herman, D. S., Lam, L., Taylor, M. R., Wang, L., Teekakirikul, P., Christodoulou, D., et al. (2012). Truncations of titin causing dilated cardiomyopathy. N. Engl. J. Med. 366 (7), 619–628. doi:10.1056/NEJMoa1110186
Herrmann, H., Cabet, E., Chevalier, N. R., Moosmann, J., Schultheis, D., Haas, J., et al. (2020). Dual functional States of R406W-desmin assembly complexes cause cardiomyopathy with severe intercalated disc derangement in humans and in knock-in mice. Mice. Circ. 142 (22), 2155–2171. doi:10.1161/CIRCULATIONAHA.120.050218
Hershberger, R. E., Hedges, D. J., and Morales, A. (2013). Dilated cardiomyopathy: The complexity of a diverse genetic architecture. Nat. Rev. Cardiol. 10 (9), 531–547. doi:10.1038/nrcardio.2013.105
Hershberger, R. E., Parks, S. B., Kushner, J. D., Li, D., Ludwigsen, S., Jakobs, P., et al. (2008). Coding sequence mutations identified in MYH7, TNNT2, SCN5A, CSRP3, LBD3, and TCAP from 313 patients with familial or idiopathic dilated cardiomyopathy. Clin. Transl. Sci. 1 (1), 21–26. doi:10.1111/j.1752-8062.2008.00017.x
Himmel, M., Van Der Ven, P. F., Stöcklein, W., and Fürst, D. O. (2003). The limits of promiscuity: Isoform-specific dimerization of filamins. Biochemistry 42 (2), 430–439. doi:10.1021/bi026501++
Hnia, K., Ramspacher, C., Vermot, J., and Laporte, J. (2015). Desmin in muscle and associated diseases: Beyond the structural function. Cell. Tissue Res. 360 (3), 591–608. doi:10.1007/s00441-014-2016-4
Holmes, W. B., and Moncman, C. L. (2008). Nebulette interacts with filamin C. Cell. Motil. Cytoskelet. 65 (2), 130–142. doi:10.1002/cm.20249
Hu, L. R., Ackermann, M. A., Hecker, P. A., Prosser, B. L., King, B., O'Connell, K. A., et al. (2017). Deregulated Ca(2+) cycling underlies the development of arrhythmia and heart disease due to mutant obscurin. Sci. Adv. 3 (6), e1603081. doi:10.1126/sciadv.1603081
Hu, L. R., and Kontrogianni-Konstantopoulos, A. (2020). Proteomic analysis of myocardia containing the obscurin R4344Q mutation linked to hypertrophic cardiomyopathy. Front. Physiol. 11, 478. doi:10.3389/fphys.2020.00478
Huang, H., Chen, Y., Jin, J., Du, R., Tang, K., Fan, L., et al. (2022). CSRP3, p.Arg122*, is responsible for hypertrophic cardiomyopathy in a Chinese family. J. Gene Med. 24 (1), e3390. doi:10.1002/jgm.3390
Huang, S. M., Huang, C. J., Wang, W. M., Kang, J. C., and Hsu, W. C. (2004). The enhancement of nuclear receptor transcriptional activation by a mouse actin-binding protein, alpha actinin 2. J. Mol. Endocrinol. 32 (2), 481–496. doi:10.1677/jme.0.0320481
Ibrahim, M., Siedlecka, U., Buyandelger, B., Harada, M., Rao, C., Moshkov, A., et al. (2013). A critical role for Telethonin in regulating t-tubule structure and function in the mammalian heart. Hum. Mol. Genet. 22 (2), 372–383. doi:10.1093/hmg/dds434
Ingles, J., Goldstein, J., Thaxton, C., Caleshu, C., Corty, E. W., Crowley, S. B., et al. (2019). Evaluating the clinical validity of hypertrophic cardiomyopathy genes. Circ. Genom Precis. Med. 12, e002460. doi:10.1161/CIRCGEN.119.002460
Itoh-Satoh, M., Hayashi, T., Nishi, H., Koga, Y., Arimura, T., Koyanagi, T., et al. (2002). Titin mutations as the molecular basis for dilated cardiomyopathy. Biochem. Biophys. Res. Commun. 291 (2), 385–393. doi:10.1006/bbrc.2002.6448
Jiang, H., Hooper, C., Kelly, M., Steeples, V., Simon, J. N., Beglov, J., et al. (2021). Functional analysis of a gene-edited mouse model to gain insights into the disease mechanisms of a titin missense variant. Basic Res. Cardiol. 116 (1), 14. doi:10.1007/s00395-021-00853-z
Johansson, J., Frykholm, C., Ericson, K., Kazamia, K., Lindberg, A., Mulaiese, N., et al. (2022). Loss of Nexilin function leads to a recessive lethal fetal cardiomyopathy characterized by cardiomegaly and endocardial fibroelastosis. Am. J. Med. Genet. A 188 (6), 1676–1687. doi:10.1002/ajmg.a.62685
Jordan, E., Peterson, L., Ai, T., Asatryan, B., Bronicki, L., Brown, E., et al. (2021). Evidence-based assessment of genes in dilated cardiomyopathy. Circulation 144 (1), 7–19. doi:10.1161/CIRCULATIONAHA.120.053033
Joseph, C., Stier, G., O'Brien, R., Politou, A. S., Atkinson, R. A., Bianco, A., et al. (2001). A structural characterization of the interactions between titin Z-repeats and the alpha-actinin C-terminal domain. Biochemistry 40 (16), 4957–4965. doi:10.1021/bi002739r
Karczewski, K. J., Francioli, L. C., Tiao, G., Cummings, B. B., Alföldi, J., Wang, Q., et al. (2020). The mutational constraint spectrum quantified from variation in 141,456 humans. Nature 581 (7809), 434–443. doi:10.1038/s41586-020-2308-7
Kiselev, A., Vaz, R., Knyazeva, A., Khudiakov, A., Tarnovskaya, S., Liu, J., et al. (2018). De novo mutations in FLNC leading to early-onset restrictive cardiomyopathy and congenital myopathy. Hum. Mutat. 39 (9), 1161–1172. doi:10.1002/humu.23559
Klauke, B., Kossmann, S., Gaertner, A., Brand, K., Stork, I., Brodehl, A., et al. (2010). De novo desmin-mutation N116S is associated with arrhythmogenic right ventricular cardiomyopathy. Hum. Mol. Genet. 19 (23), 4595–4607. doi:10.1093/hmg/ddq387
Kley, R. A., Serdaroglu-Oflazer, P., Leber, Y., Odgerel, Z., van der Ven, P. F., Olivé, M., et al. (2012). Pathophysiology of protein aggregation and extended phenotyping in filaminopathy. Brain 135, 2642–2660. doi:10.1093/brain/aws200
Knöll, R., Buyandelger, B., and Lab, M. (2011a). The sarcomeric Z-disc and Z-discopathies. J. Biomed. Biotechnol. 2011, 569628. doi:10.1155/2011/569628
Knöll, R., Hoshijima, M., Hoffman, H. M., Person, V., Lorenzen-Schmidt, I., Bang, M. L., et al. (2002). The cardiac mechanical stretch sensor machinery involves a Z disc complex that is defective in a subset of human dilated cardiomyopathy. Cell. 111 (7), 943–955. doi:10.1016/s0092-8674(02)01226-6
Knöll, R., Kostin, S., Klede, S., Savvatis, K., Klinge, L., Stehle, I., et al. (2010). A common MLP (muscle LIM protein) variant is associated with cardiomyopathy. Circ. Res. 106 (4), 695–704. doi:10.1161/CIRCRESAHA.109.206243
Knöll, R., Linke, W. A., Zou, P., Miocic, S., Kostin, S., Buyandelger, B., et al. (2011b). Telethonin deficiency is associated with maladaptation to biomechanical stress in the mammalian heart. Circ. Res. 109 (7), 758–769. doi:10.1161/CIRCRESAHA.111.245787
Kollár, V., Szatmári, D., Grama, L., and Kellermayer, M. S. (2010). Dynamic strength of titin's Z-disk end. J. Biomed. Biotechnol. 2010, 838530. doi:10.1155/2010/838530
Kontrogianni-Konstantopoulos, A., and Bloch, R. J. (2005). Obscurin: A multitasking muscle giant. J. Muscle Res. Cell. Motil. 26 (6-8), 419–426. doi:10.1007/s10974-005-9024-7
Kontrogianni-Konstantopoulos, A., Catino, D. H., Strong, J. C., Sutter, S., Borisov, A. B., Pumplin, D. W., et al. (2006). Obscurin modulates the assembly and organization of sarcomeres and the sarcoplasmic reticulum. Faseb J. 20 (12), 2102–2111. doi:10.1096/fj.06-5761com
Lindholm, M. E., Jimenez-Morales, D., Zhu, H., Seo, K., Amar, D., Zhao, C., et al. (2021). Mono- and biallelic protein-truncating variants in alpha-actinin 2 cause cardiomyopathy through distinct mechanisms. Circ. Genom Precis. Med. 14, e003419. doi:10.1161/CIRCGEN.121.003419
Labeit, S., and Kolmerer, B. (1995). Titins: Giant proteins in charge of muscle ultrastructure and elasticity. Science 270 (5234), 293–296. doi:10.1126/science.270.5234.293
Laddach, A., Gautel, M., and Fraternali, F. (2017). TITINdb-a computational tool to assess titin's role as a disease gene. Bioinformatics 33 (21), 3482–3485. doi:10.1093/bioinformatics/btx424
Ladha, F. A., Thakar, K., Pettinato, A. M., Legere, N., Cohn, R., Romano, R., et al. (2020). Identifying cardiac actinin interactomes reveals sarcomere crosstalk with RNA-binding proteins. bioRxiv. 2020.2003.2018.994004. doi:10.1101/2020.03.18.994004
Lange, S., Gehmlich, K., Lun, A. S., Blondelle, J., Hooper, C., Dalton, N. D., et al. (2016). MLP and CARP are linked to chronic PKCα signalling in dilated cardiomyopathy. Nat. Commun. 7, 12120. doi:10.1038/ncomms12120
Lange, S., Ouyang, K., Meyer, G., Cui, L., Cheng, H., Lieber, R. L., et al. (2009). Obscurin determines the architecture of the longitudinal sarcoplasmic reticulum. J. Cell. Sci. 122, 2640–2650. doi:10.1242/jcs.046193
Leber, Y., Ruparelia, A. A., Kirfel, G., van der Ven, P. F., Hoffmann, B., Merkel, R., et al. (2016). Filamin C is a highly dynamic protein associated with fast repair of myofibrillar microdamage. Hum. Mol. Genet. 25 (13), 2776–2788. doi:10.1093/hmg/ddw135
Li, B., Zhuang, L., and Trueb, B. (2004). Zyxin interacts with the SH3 domains of the cytoskeletal proteins LIM-nebulette and Lasp-1. J. Biol. Chem. 279 (19), 20401–20410. doi:10.1074/jbc.M310304200
Li, D., Tapscoft, T., Gonzalez, O., Burch, P. E., Quiñones, M. A., Zoghbi, W. A., et al. (1999). Desmin mutation responsible for idiopathic dilated cardiomyopathy. Circulation 100 (5), 461–464. doi:10.1161/01.cir.100.5.461
Li, H., Zheng, L., Mo, Y., Gong, Q., Jiang, A., and Zhao, J. (2016). Voltage-dependent anion channel 1(VDAC1) participates the apoptosis of the mitochondrial dysfunction in desminopathy. PLoS One 11, e0167908. doi:10.1371/journal.pone.0167908
Lipari, M., Wypasek, E., Karpiński, M., Tomkiewicz-Pajak, L., Laino, L., Binni, F., et al. (2020). Identification of a variant hotspot in MYBPC3 and of a novel CSRP3 autosomal recessive alteration in a cohort of Polish patients with hypertrophic cardiomyopathy. Pol. Arch. Intern Med. 130 (2), 89–99. doi:10.20452/pamw.15130
Littlefield, R. S., and Fowler, V. M. (2008). Thin filament length regulation in striated muscle sarcomeres: Pointed-end dynamics go beyond a nebulin ruler. Semin. Cell. Dev. Biol. 19 (6), 511–519. doi:10.1016/j.semcdb.2008.08.009
Liu, C., Spinozzi, S., Chen, J. Y., Fang, X., Feng, W., Perkins, G., et al. (2019). Nexilin is a new component of junctional membrane complexes required for cardiac T-tubule formation. Circulation 140 (1), 55–66. doi:10.1161/CIRCULATIONAHA.119.039751
Liu, C., Spinozzi, S., Feng, W., Chen, Z., Zhang, L., Zhu, S., et al. (2020). Homozygous G650del nexilin variant causes cardiomyopathy in mice. JCI Insight 5, e138780. doi:10.1172/jci.insight.138780
Loo, D. T., Kanner, S. B., and Aruffo, A. (1998). Filamin binds to the cytoplasmic domain of the beta1-integrin. Identification of amino acids responsible for this interaction. J. Biol. Chem. 273 (36), 23304–23312. doi:10.1074/jbc.273.36.23304
Lopes, L. R., and Elliott, P. M. (2014). A straightforward guide to the sarcomeric basis of cardiomyopathies. Heart 100 (24), 1916–1923. doi:10.1136/heartjnl-2014-305645
Lu, L., Timofeyev, V., Li, N., Rafizadeh, S., Singapuri, A., Harris, T. R., et al. (2009). Alpha-actinin2 cytoskeletal protein is required for the functional membrane localization of a Ca2+-activated K+ channel (SK2 channel). Proc. Natl. Acad. Sci. U. S. A. 106 (43), 18402–18407. doi:10.1073/pnas.0908207106
Luther, P. K., and Squire, J. M. (2002). Muscle Z-band ultrastructure: Titin Z-repeats and Z-band periodicities do not match. J. Mol. Biol. 319 (5), 1157–1164. doi:10.1016/S0022-2836(02)00372-8
Maiellaro-Rafferty, K., Wansapura, J. P., Mendsaikhan, U., Osinska, H., James, J. F., Taylor, M. D., et al. (2013). Altered regional cardiac wall mechanics are associated with differential cardiomyocyte calcium handling due to nebulette mutations in preclinical inherited dilated cardiomyopathy. J. Mol. Cell. Cardiol. 60, 151–160. doi:10.1016/j.yjmcc.2013.04.021
Manring, H. R., Carter, O. A., and Ackermann, M. A. (2017). Obscure functions: The location-function relationship of obscurins. Biophys. Rev. 9 (3), 245–258. doi:10.1007/s12551-017-0254-x
Mao, Z., and Nakamura, F. (2020). Structure and function of filamin C in the muscle Z-disc. Int. J. Mol. Sci. 21, 2696. doi:10.3390/ijms21082696
Marcello, M., Cetrangolo, V., Savarese, M., and Udd, B. (2022). Use of animal models to understand titin physiology and pathology. J. Cell. Mol. Med. 26 (20), 5103–5112. doi:10.1111/jcmm.17533
Marian, A. J., and Braunwald, E. (2017). Hypertrophic cardiomyopathy: Genetics, pathogenesis, clinical manifestations, diagnosis, and therapy. Circ. Res. 121 (7), 749–770. doi:10.1161/CIRCRESAHA.117.311059
Marston, S., Montgiraud, C., Munster, A. B., Copeland, O., Choi, O., Dos Remedios, C., et al. (2015). OBSCN mutations associated with dilated cardiomyopathy and haploinsufficiency. PLoS One 10, e0138568. doi:10.1371/journal.pone.0138568
Maruyama, K. (1997). Connectin/titin, giant elastic protein of muscle. Faseb J. 11 (5), 341–345. doi:10.1096/fasebj.11.5.9141500
Mastrototaro, G., Liang, X., Li, X., Carullo, P., Piroddi, N., Tesi, C., et al. (2015). Nebulette knockout mice have normal cardiac function, but show Z-line widening and up-regulation of cardiac stress markers. Cardiovasc Res. 107 (2), 216–225. doi:10.1093/cvr/cvv156
Mavroidis, M., Panagopoulou, P., Kostavasili, I., Weisleder, N., and Capetanaki, Y. (2008). A missense mutation in desmin tail domain linked to human dilated cardiomyopathy promotes cleavage of the head domain and abolishes its Z-disc localization. Faseb J. 22 (9), 3318–3327. doi:10.1096/fj.07-088724
Mazzarotto, F., Hawley, M. H., Beltrami, M., Beekman, L., de Marvao, A., McGurk, K. A., et al. (2021). Systematic large-scale assessment of the genetic architecture of left ventricular noncompaction reveals diverse etiologies. Genet. Med. 23 (5), 856–864. doi:10.1038/s41436-020-01049-x
McLendon, P. M., and Robbins, J. (2011). Desmin-related cardiomyopathy: An unfolding story. Am. J. Physiol. Heart Circ. Physiol. 301 (4), H1220–H1228. doi:10.1152/ajpheart.00601.2011
Mendes de Almeida, R., Tavares, J., Martins, S., Carvalho, T., Enguita, F. J., Brito, D., et al. (2017). Whole gene sequencing identifies deep-intronic variants with potential functional impact in patients with hypertrophic cardiomyopathy. PLoS One 12, e0182946. doi:10.1371/journal.pone.0182946
Meyer, T., Ruppert, V., Ackermann, S., Richter, A., Perrot, A., Sperling, S. R., et al. (2013). Novel mutations in the sarcomeric protein myopalladin in patients with dilated cardiomyopathy. Eur. J. Hum. Genet. 21 (3), 294–300. doi:10.1038/ejhg.2012.173
Micheu, M. M., Oprescu, N., and Popa-Fotea, N.-M. (2021). In silico analysis of novel titin non-synonymous missense variants detected by targeted next-generation sequencing in a cohort of Romanian index patients with hypertrophic cardiomyopathy. Romanian J. Cardiol. 31 (3), 565–571. doi:10.47803/rjc.2021.31.3.565
Millevoi, S., Trombitas, K., Kolmerer, B., Kostin, S., Schaper, J., Pelin, K., et al. (1998). Characterization of nebulette and nebulin and emerging concepts of their roles for vertebrate Z-discs. J. Mol. Biol. 282 (1), 111–123. doi:10.1006/jmbi.1998.1999
Milner, D. J., Taffet, G. E., Wang, X., Pham, T., Tamura, T., Hartley, C., et al. (1999). The absence of desmin leads to cardiomyocyte hypertrophy and cardiac dilation with compromised systolic function. J. Mol. Cell. Cardiol. 31 (11), 2063–2076. doi:10.1006/jmcc.1999.1037
Miyamoto, Y., Akita, H., Shiga, N., Takai, E., Iwai, C., Mizutani, K., et al. (2001). Frequency and clinical characteristics of dilated cardiomyopathy caused by desmin gene mutation in a Japanese population. Eur. Heart J. 22 (24), 2284–2289. doi:10.1053/euhj.2001.2836
Mohapatra, B., Jimenez, S., Lin, J. H., Bowles, K. R., Coveler, K. J., Marx, J. G., et al. (2003). Mutations in the muscle LIM protein and alpha-actinin-2 genes in dilated cardiomyopathy and endocardial fibroelastosis. Mol. Genet. Metab. 80 (1-2), 207–215. doi:10.1016/s1096-7192(03)00142-2
Molt, S., Bührdel, J. B., Yakovlev, S., Schein, P., Orfanos, Z., Kirfel, G., et al. (2014). Aciculin interacts with filamin C and Xin and is essential for myofibril assembly, remodeling and maintenance. J. Cell. Sci. 127, 3578–3592. doi:10.1242/jcs.152157
Moncman, C. L., and Wang, K. (1999). Functional dissection of nebulette demonstrates actin binding of nebulin-like repeats and Z-line targeting of SH3 and linker domains. Cell. Motil. Cytoskelet. 44 (1), 1–22. doi:10.1002/(SICI)1097-0169(199909)44:1<1:AID-CM1>3.0.CO;2-8:1<1:Aid-cm1>3.0.Co;1–22
Moncman, C. L., and Wang, K. (1995). Nebulette: A 107 kD nebulin-like protein in cardiac muscle. Cell. Motil. Cytoskelet. 32 (3), 205–225. doi:10.1002/cm.970320305
Muchtar, E., Blauwet, L. A., and Gertz, M. A. (2017). Restrictive cardiomyopathy: Genetics, pathogenesis, clinical manifestations, diagnosis, and therapy. Circ. Res. 121 (7), 819–837. doi:10.1161/CIRCRESAHA.117.310982
Mues, A., van der Ven, P. F., Young, P., Fürst, D. O., and Gautel, M. (1998). Two immunoglobulin-like domains of the Z-disc portion of titin interact in a conformation-dependent way with telethonin. FEBS Lett. 428 (1-2), 111–114. doi:10.1016/s0014-5793(98)00501-8
Mukund, K., and Subramaniam, S. (2020). Skeletal muscle: A review of molecular structure and function, in health and disease. Wiley Interdiscip. Rev. Syst. Biol. Med. 12 (1), e1462. doi:10.1002/wsbm.1462
Nagase, T., Kikuno, R., Nakayama, M., Hirosawa, M., and Ohara, O. (2000). Prediction of the coding sequences of unidentified human genes. XVIII. The complete sequences of 100 new cDNA clones from brain which code for large proteins in vitro. DNA Res. 7 (4), 273–281. doi:10.1093/dnares/7.4.271
Nagueh, S. F., Shah, G., Wu, Y., Torre-Amione, G., King, N. M., Lahmers, S., et al. (2004). Altered titin expression, myocardial stiffness, and left ventricular function in patients with dilated cardiomyopathy. Circulation 110 (2), 155–162. doi:10.1161/01.CIR.0000135591.37759.AF
Nakamura, F., Stossel, T. P., and Hartwig, J. H. (2011). The filamins: Organizers of cell structure and function. Cell. Adh Migr. 5 (2), 160–169. doi:10.4161/cam.5.2.14401
Nollet, E. E., Westenbrink, B. D., de Boer, R. A., Kuster, D. W. D., and van der Velden, J. (2020). Unraveling the genotype-phenotype relationship in hypertrophic cardiomyopathy: Obesity-related cardiac defects as a major disease modifier. J. Am. Heart Assoc. 9, e018641. doi:10.1161/JAHA.120.018641
Ogut, O., Hossain, M. M., and Jin, J. P. (2003). Interactions between nebulin-like motifs and thin filament regulatory proteins. J. Biol. Chem. 278 (5), 3089–3097. doi:10.1074/jbc.M205853200
Ohtsuka, H., Yajima, H., Maruyama, K., and Kimura, S. (1997). The N-terminal Z repeat 5 of connectin/titin binds to the C-terminal region of alpha-actinin. Biochem. Biophys. Res. Commun. 235 (1), 1–3. doi:10.1006/bbrc.1997.6534
Ohtsuka, T., Nakanishi, H., Ikeda, W., Satoh, A., Momose, Y., Nishioka, H., et al. (1998). Nexilin: A novel actin filament-binding protein localized at cell-matrix adherens junction. J. Cell. Biol. 143 (5), 1227–1238. doi:10.1083/jcb.143.5.1227
Oka, H., Nakau, K., Imanishi, R., Furukawa, T., Tanabe, Y., Hirono, K., et al. (2021). A case report of a rare heterozygous variant in the desmin gene associated with hypertrophic cardiomyopathy and complete atrioventricular block. CJC Open 3 (9), 1195–1198. doi:10.1016/j.cjco.2021.05.003
Olivé, M., Goldfarb, L., Moreno, D., Laforet, E., Dagvadorj, A., Sambuughin, N., et al. (2004). Desmin-related myopathy: Clinical, electrophysiological, radiological, neuropathological and genetic studies. J. Neurol. Sci. 219 (1-2), 125–137. doi:10.1016/j.jns.2004.01.007
Ortiz-Genga, M. F., Cuenca, S., Dal Ferro, M., Zorio, E., Salgado-Aranda, R., Climent, V., et al. (2016). Truncating FLNC mutations are associated with high-risk dilated and arrhythmogenic cardiomyopathies. J. Am. Coll. Cardiol. 68 (22), 2440–2451. doi:10.1016/j.jacc.2016.09.927
Otey, C. A., and Carpen, O. (2004). Alpha-actinin revisited: A fresh look at an old player. Cell. Motil. Cytoskelet. 58 (2), 104–111. doi:10.1002/cm.20007
Otey, C. A., Rachlin, A., Moza, M., Arneman, D., and Carpen, O. (2005). The palladin/myotilin/myopalladin family of actin-associated scaffolds. Int. Rev. Cytol. 246, 31–58. doi:10.1016/S0074-7696(05)46002-7
Perrot, A., Tomasov, P., Villard, E., Faludi, R., Melacini, P., Lossie, J., et al. (2016). Mutations in NEBL encoding the cardiac Z-disk protein nebulette are associated with various cardiomyopathies. Arch. Med. Sci. 12 (2), 263–278. doi:10.5114/aoms.2016.59250
Popowicz, G. M., Schleicher, M., Noegel, A. A., and Holak, T. A. (2006). Filamins: Promiscuous organizers of the cytoskeleton. Trends Biochem. Sci. 31 (7), 411–419. doi:10.1016/j.tibs.2006.05.006
Powers, J. D., Kirkland, N. J., Liu, C., Razu, S. S., Fang, X., Engler, A. J., et al. (2022). Subcellular remodeling in filamin C deficient mouse hearts impairs myocyte tension development during progression of dilated cardiomyopathy. Int. J. Mol. Sci. 23, 871. doi:10.3390/ijms23020871
Prondzynski, M., Lemoine, M. D., Zech, A. T., Horváth, A., Di Mauro, V., Koivumäki, J. T., et al. (2019). Disease modeling of a mutation in α-actinin 2 guides clinical therapy in hypertrophic cardiomyopathy. EMBO Mol. Med. 11 (12), e11115. doi:10.15252/emmm.201911115
Pudas, R., Kiema, T. R., Butler, P. J., Stewart, M., and Ylänne, J. (2005). Structural basis for vertebrate filamin dimerization. Structure 13 (1), 111–119. doi:10.1016/j.str.2004.10.014
Purevjav, E., Arimura, T., Augustin, S., Huby, A. C., Takagi, K., Nunoda, S., et al. (2012). Molecular basis for clinical heterogeneity in inherited cardiomyopathies due to myopalladin mutations. Hum. Mol. Genet. 21 (9), 2039–2053. doi:10.1093/hmg/dds022
Purevjav, E., Varela, J., Morgado, M., Kearney, D. L., Li, H., Taylor, M. D., et al. (2010). Nebulette mutations are associated with dilated cardiomyopathy and endocardial fibroelastosis. J. Am. Coll. Cardiol. 56 (18), 1493–1502. doi:10.1016/j.jacc.2010.05.045
Pyle, W. G., and Solaro, R. J. (2004). At the crossroads of myocardial signaling: The role of Z-discs in intracellular signaling and cardiac function. Circ. Res. 94 (3), 296–305. doi:10.1161/01.RES.0000116143.74830.A9
Rakar, S., Sinagra, G., Di Lenarda, A., Poletti, A., Bussani, R., Silvestri, F., et al. (1997). Epidemiology of dilated cardiomyopathy. A prospective post-mortem study of 5252 necropsies. The Heart Muscle Disease Study Group. Eur. Heart J. 18 (1), 117–123. doi:10.1093/oxfordjournals.eurheartj.a015092
Ram, R., and Blaxall, B. C. (2010). Nebulette mutations in cardiac remodeling: Big effects from a small mechanosensor. J. Am. Coll. Cardiol. 56 (18), 1503–1505. doi:10.1016/j.jacc.2010.06.031
Randazzo, D., Giacomello, E., Lorenzini, S., Rossi, D., Pierantozzi, E., Blaauw, B., et al. (2013). Obscurin is required for ankyrinB-dependent dystrophin localization and sarcolemma integrity. J. Cell. Biol. 200 (4), 523–536. doi:10.1083/jcb.201205118
Randazzo, D., Pierantozzi, E., Rossi, D., and Sorrentino, V. (2017). The potential of obscurin as a therapeutic target in muscle disorders. Expert Opin. Ther. Targets 21 (9), 897–910. doi:10.1080/14728222.2017.1361931
Razinia, Z., Mäkelä, T., Ylänne, J., and Calderwood, D. A. (2012). Filamins in mechanosensing and signaling. Annu. Rev. Biophys. 41, 227–246. doi:10.1146/annurev-biophys-050511-102252
Reilly, L., Munawar, S., Zhang, J., Crone, W. C., and Eckhardt, L. L. (2022). Challenges and innovation: Disease modeling using human-induced pluripotent stem cell-derived cardiomyocytes. Front. Cardiovasc Med. 9, 966094. doi:10.3389/fcvm.2022.966094
Riaz, M., Park, J., Sewanan, L. R., Ren, Y., Schwan, J., Das, S. K., et al. (2022). Muscle LIM protein force-sensing mediates sarcomeric biomechanical signaling in human familial hypertrophic cardiomyopathy. Circulation 145 (16), 1238–1253. doi:10.1161/CIRCULATIONAHA.121.056265
Ribeiro Ede, A., Pinotsis, N., Ghisleni, A., Salmazo, A., Konarev, P. V., Kostan, J., et al. (2014). The structure and regulation of human muscle α-actinin. Cell. 159 (6), 1447–1460. doi:10.1016/j.cell.2014.10.056
Richards, S., Aziz, N., Bale, S., Bick, D., Das, S., Gastier-Foster, J., et al. (2015). Standards and guidelines for the interpretation of sequence variants: A joint consensus recommendation of the American College of medical genetics and Genomics and the association for molecular pathology. Genet. Med. 17 (5), 405–424. doi:10.1038/gim.2015.30
Roberts, A. M., Ware, J. S., Herman, D. S., Schafer, S., Baksi, J., Bick, A. G., et al. (2015). Integrated allelic, transcriptional, and phenomic dissection of the cardiac effects of titin truncations in health and disease. Sci. Transl. Med. 7 (270), 270ra6. doi:10.1126/scitranslmed.3010134
Rowland, T. J., Graw, S. L., Sweet, M. E., Gigli, M., Taylor, M. R., and Mestroni, L. (2016). Obscurin variants in patients with left ventricular noncompaction. J. Am. Coll. Cardiol. 68 (20), 2237–2238. doi:10.1016/j.jacc.2016.08.052
Russell, M. W., Raeker, M. O., Korytkowski, K. A., and Sonneman, K. J. (2002). Identification, tissue expression and chromosomal localization of human Obscurin-MLCK, a member of the titin and Dbl families of myosin light chain kinases. Gene 282 (1-2), 237–246. doi:10.1016/s0378-1119(01)00795-8
Subramaniam, J., Yamankurt, G., and Cunha, S. R. (2022). Obscurin regulates ankyrin macromolecular complex formation. J. Mol. Cell. Cardiol. 168, 44–57. doi:10.1016/j.yjmcc.2022.04.008
Sabater-Molina, M., Pérez-Sánchez, I., Hernández Del Rincón, J. P., and Gimeno, J. R. (2018). Genetics of hypertrophic cardiomyopathy: A review of current state. Clin. Genet. 93 (1), 3–14. doi:10.1111/cge.13027
Salazar-Mendiguchía, J., Barriales-Villa, R., Lopes, L. R., Ochoa, J. P., Rodríguez-Vilela, A., Palomino-Doza, J., et al. (2020). The p. (Cys150Tyr) variant in CSRP3 is associated with late-onset hypertrophic cardiomyopathy in heterozygous individuals. Eur. J. Med. Genet. 63 (12), 104079. doi:10.1016/j.ejmg.2020.104079
Satoh, M., Takahashi, M., Sakamoto, T., Hiroe, M., Marumo, F., and Kimura, A. (1999). Structural analysis of the titin gene in hypertrophic cardiomyopathy: Identification of a novel disease gene. Biochem. Biophys. Res. Commun. 262 (2), 411–417. doi:10.1006/bbrc.1999.1221
Schafer, S., de Marvao, A., Adami, E., Fiedler, L. R., Ng, B., Khin, E., et al. (2017). Titin-truncating variants affect heart function in disease cohorts and the general population. Nat. Genet. 49 (1), 46–53. doi:10.1038/ng.3719
Schallus, T., Fehér, K., Ulrich, A. S., Stier, G., and Muhle-Goll, C. (2009). Structure and dynamics of the human muscle LIM protein. FEBS Lett. 583 (6), 1017–1022. doi:10.1016/j.febslet.2009.02.021
Schubert, J., Tariq, M., Geddes, G., Kindel, S., Miller, E. M., and Ware, S. M. (2018). Novel pathogenic variants in filamin C identified in pediatric restrictive cardiomyopathy. Hum. Mutat. 39 (12), 2083–2096. doi:10.1002/humu.23661
Selcen, D., and Carpén, O. (2008). The Z-disk diseases. Adv. Exp. Med. Biol. 642, 116–130. doi:10.1007/978-0-387-84847-1_10
Seppälä, J., Tossavainen, H., Rodic, N., Permi, P., Pentikäinen, U., and Ylänne, J. (2015). Flexible structure of peptide-bound filamin A mechanosensor domain pair 20-21. PLoS One 10, e0136969. doi:10.1371/journal.pone.0136969
Sheikh, F., and Chen, J. (2007). Mouse models for cardiomyopathy research. Prog. Pediatr. Cardiol. 24 (1), 27–34. doi:10.1016/j.ppedcard.2007.08.006
Singh, S. R., Kadioglu, H., Patel, K., Carrier, L., and Agnetti, G. (2020). Is desmin propensity to aggregate part of its protective function? Cells 9, 491. doi:10.3390/cells9020491
Sjöblom, B., Salmazo, A., and Djinović-Carugo, K. (2008). Alpha-actinin structure and regulation. Cell. Mol. Life Sci. 65 (17), 2688–2701. doi:10.1007/s00018-008-8080-8
Sorimachi, H., Freiburg, A., Kolmerer, B., Ishiura, S., Stier, G., Gregorio, C. C., et al. (1997). Tissue-specific expression and alpha-actinin binding properties of the Z-disc titin: Implications for the nature of vertebrate Z-discs. J. Mol. Biol. 270 (5), 688–695. doi:10.1006/jmbi.1997.1145
Spinozzi, S., Liu, C., Chen, Z., Feng, W., Zhang, L., Ouyang, K., et al. (2020). Nexilin is necessary for maintaining the transverse-axial tubular system in adult cardiomyocytes. Circ. Heart Fail 13, e006935. doi:10.1161/CIRCHEARTFAILURE.120.006935
Srivastava, S., Yavari, M., Al-Abcha, A., Banga, S., and Abela, G. (2022). Ventricular non-compaction review. Heart Fail Rev. 27 (4), 1063–1076. doi:10.1007/s10741-021-10128-3
Stossel, T. P., Condeelis, J., Cooley, L., Hartwig, J. H., Noegel, A., Schleicher, M., et al. (2001). Filamins as integrators of cell mechanics and signalling. Nat. Rev. Mol. Cell. Biol. 2 (2), 138–145. doi:10.1038/35052082
Tadros, R., Francis, C., Xu, X., Vermeer, A. M. C., Harper, A. R., Huurman, R., et al. (2021). Shared genetic pathways contribute to risk of hypertrophic and dilated cardiomyopathies with opposite directions of effect. Nat. Genet. 53 (2), 128–134. doi:10.1038/s41588-020-00762-2
Tamiya, R., Saito, Y., Fukamachi, D., Nagashima, K., Aizawa, Y., Ohkubo, K., et al. (2020). Desmin-related myopathy characterized by non-compaction cardiomyopathy, cardiac conduction defect, and coronary artery dissection. Esc. Heart Fail 7 (3), 1338–1343. doi:10.1002/ehf2.12667
Taylor, M., Graw, S., Sinagra, G., Barnes, C., Slavov, D., Brun, F., et al. (2011). Genetic variation in titin in arrhythmogenic right ventricular cardiomyopathy-overlap syndromes. Circulation 124 (8), 876–885. doi:10.1161/CIRCULATIONAHA.110.005405
Taylor, M. R., Carniel, E., and Mestroni, L. (2006). Cardiomyopathy, familial dilated. Orphanet J. Rare Dis. 1, 27. doi:10.1186/1750-1172-1-27
Theis, J. L., Bos, J. M., Bartleson, V. B., Will, M. L., Binder, J., Vatta, M., et al. (2006). Echocardiographic-determined septal morphology in Z-disc hypertrophic cardiomyopathy. Biochem. Biophys. Res. Commun. 351 (4), 896–902. doi:10.1016/j.bbrc.2006.10.119
Thompson, T. G., Chan, Y. M., Hack, A. A., Brosius, M., Rajala, M., Lidov, H. G., et al. (2000). Filamin 2 (FLN2): A muscle-specific sarcoglycan interacting protein. J. Cell. Biol. 148 (1), 115–126. doi:10.1083/jcb.148.1.115
Tonino, P., Kiss, B., Gohlke, J., Smith, J. E., and Granzier, H. (2019). Fine mapping titin's C-zone: Matching cardiac myosin-binding protein C stripes with titin's super-repeats. J. Mol. Cell. Cardiol. 133, 47–56. doi:10.1016/j.yjmcc.2019.05.026
Toste, A., Perrot, A., Özcelik, C., and Cardim, N. (2020). Identification of a novel titin-cap/telethonin mutation in a Portuguese family with hypertrophic cardiomyopathy. Rev. Port. Cardiol. Engl. Ed. 39 (6), 317–327. doi:10.1016/j.repc.2019.12.007
Tucker, N. R., McLellan, M. A., Hu, D., Ye, J., Parsons, V. A., Mills, R. W., et al. (2017). Novel mutation in FLNC (filamin C) causes familial restrictive cardiomyopathy. Circ. Cardiovasc Genet. 10, e001780. doi:10.1161/CIRCGENETICS.117.001780
Valdés-Mas, R., Gutiérrez-Fernández, A., Gómez, J., Coto, E., Astudillo, A., Puente, D. A., et al. (2014). Mutations in filamin C cause a new form of familial hypertrophic cardiomyopathy. Nat. Commun. 5, 5326. doi:10.1038/ncomms6326
Valle, G., Faulkner, G., De Antoni, A., Pacchioni, B., Pallavicini, A., Pandolfo, D., et al. (1997). Telethonin, a novel sarcomeric protein of heart and skeletal muscle. FEBS Lett. 415 (2), 163–168. doi:10.1016/s0014-5793(97)01108-3
van der Flier, A., and Sonnenberg, A. (2001). Structural and functional aspects of filamins. Biochim. Biophys. Acta 1538 (2-3), 99–117. doi:10.1016/s0167-4889(01)00072-6
van der Ven, P. F., Ehler, E., Vakeel, P., Eulitz, S., Schenk, J. A., Milting, H., et al. (2006). Unusual splicing events result in distinct Xin isoforms that associate differentially with filamin c and Mena/VASP. Exp. Cell. Res. 312 (11), 2154–2167. doi:10.1016/j.yexcr.2006.03.015
van der Ven, P. F., Obermann, W. M., Lemke, B., Gautel, M., Weber, K., and Fürst, D. O. (2000). Characterization of muscle filamin isoforms suggests a possible role of gamma-filamin/ABP-L in sarcomeric Z-disc formation. Cell. Motil. Cytoskelet. 45 (2), 149–162. doi:10.1002/(SICI)1097-0169(200002)45:2<149:AID-CM6>3.0.CO;2-G:2<149:Aid-cm6>3.0.Co;2-g
Vicart, P., Dupret, J. M., Hazan, J., Li, Z., Gyapay, G., Krishnamoorthy, R., et al. (1996). Human desmin gene: cDNA sequence, regional localization and exclusion of the locus in a familial desmin-related myopathy. Hum. Genet. 98 (4), 422–429. doi:10.1007/s004390050233
Wadmore, K., Azad, A. J., and Gehmlich, K. (2021). The role of Z-disc proteins in myopathy and cardiomyopathy. Int. J. Mol. Sci. 22, 3058. doi:10.3390/ijms22063058
Walsh, R., Thomson, K. L., Ware, J. S., Funke, B. H., Woodley, J., McGuire, K. J., et al. (2017). Reassessment of Mendelian gene pathogenicity using 7,855 cardiomyopathy cases and 60,706 reference samples. Genet. Med. 19 (2), 192–203. doi:10.1038/gim.2016.90
Wang, H., Li, Z., Wang, J., Sun, K., Cui, Q., Song, L., et al. (2010). Mutations in NEXN, a Z-disc gene, are associated with hypertrophic cardiomyopathy. Am. J. Hum. Genet. 87 (5), 687–693. doi:10.1016/j.ajhg.2010.10.002
Wang, W., Zhang, W., Han, Y., Chen, J., Wang, Y., Zhang, Z., et al. (2005). NELIN, a new F-actin associated protein, stimulates HeLa cell migration and adhesion. Biochem. Biophys. Res. Commun. 330 (4), 1127–1131. doi:10.1016/j.bbrc.2005.03.082
Wang, X., Osinska, H., Dorn, G. W., Nieman, M., Lorenz, J. N., Gerdes, A. M., et al. (2001). Mouse model of desmin-related cardiomyopathy. Circulation 103 (19), 2402–2407. doi:10.1161/01.cir.103.19.2402
Ware, J. S., Amor-Salamanca, A., Tayal, U., Govind, R., Serrano, I., Salazar-Mendiguchía, J., et al. (2018). Genetic etiology for alcohol-induced cardiac toxicity. J. Am. Coll. Cardiol. 71 (20), 2293–2302. doi:10.1016/j.jacc.2018.03.462
Ware, J. S., Li, J., Mazaika, E., Yasso, C. M., DeSouza, T., Cappola, T. P., et al. (2016). Shared genetic predisposition in peripartum and dilated cardiomyopathies. N. Engl. J. Med. 374 (3), 233–241. doi:10.1056/NEJMoa1505517
Whiting, A., Wardale, J., and Trinick, J. (1989). Does titin regulate the length of muscle thick filaments? J. Mol. Biol. 205 (1), 263–268. doi:10.1016/0022-2836(89)90381-1
Xiao, L., Li, C., Sun, Y., Chen, Y., Wei, H., Hu, D., et al. (2021). Clinical significance of variants in the TTN gene in a large cohort of patients with sporadic dilated cardiomyopathy. Front. Cardiovasc Med. 8, 657689. doi:10.3389/fcvm.2021.657689
Xu, J., Li, Z., Ren, X., Dong, M., Li, J., Shi, X., et al. (2015). Investigation of pathogenic genes in Chinese sporadic hypertrophic cardiomyopathy patients by whole exome sequencing. Sci. Rep. 5, 16609. doi:10.1038/srep16609
Ye, J. Z., Delmar, M., Lundby, A., and Olesen, M. S. (2019). Reevaluation of genetic variants previously associated with arrhythmogenic right ventricular cardiomyopathy integrating population-based cohorts and proteomics data. Clin. Genet. 96 (6), 506–514. doi:10.1111/cge.13621
Ylänne, J., Scheffzek, K., Young, P., and Saraste, M. (2001). Crystal structure of the alpha-actinin rod reveals an extensive torsional twist. Structure 9 (7), 597–604. doi:10.1016/s0969-2126(01)00619-0
Young, P., Ehler, E., and Gautel, M. (2001). Obscurin, a giant sarcomeric Rho guanine nucleotide exchange factor protein involved in sarcomere assembly. J. Cell. Biol. 154 (1), 123–136. doi:10.1083/jcb.200102110
Young, P., Ferguson, C., Bañuelos, S., and Gautel, M. (1998). Molecular structure of the sarcomeric Z-disk: Two types of titin interactions lead to an asymmetrical sorting of alpha-actinin. Embo J. 17 (6), 1614–1624. doi:10.1093/emboj/17.6.1614
Zech, A. T. L., Prondzynski, M., Singh, S. R., Pietsch, N., Orthey, E., Alizoti, E., et al. (2022). ACTN2 mutant causes proteopathy in human iPSC-derived cardiomyocytes. Cells 11, 2745. doi:10.3390/cells11172745
Zhang, C., Zhang, H., Wu, G., Luo, X., Zhang, C., Zou, Y., et al. (2017). Titin-truncating variants increase the risk of cardiovascular death in patients with hypertrophic cardiomyopathy. Can. J. Cardiol. 33 (10), 1292–1297. doi:10.1016/j.cjca.2017.05.020
Zhang, M., Liu, J., Cheng, A., Deyoung, S. M., and Saltiel, A. R. (2007). Identification of CAP as a costameric protein that interacts with filamin C. Mol. Biol. Cell. 18 (12), 4731–4740. doi:10.1091/mbc.e07-06-0628
Zhao, Y., Feng, Y., Zhang, Y. M., Ding, X. X., Song, Y. Z., Zhang, A. M., et al. (2015). Targeted next-generation sequencing of candidate genes reveals novel mutations in patients with dilated cardiomyopathy. Int. J. Mol. Med. 36 (6), 1479–1486. doi:10.3892/ijmm.2015.2361
Zhou, X., Borén, J., and Akyürek, L. M. (2007). Filamins in cardiovascular development. Trends Cardiovasc Med. 17 (7), 222–229. doi:10.1016/j.tcm.2007.08.001
Zhou, Y., Chen, Z., Zhang, L., Zhu, M., Tan, C., Zhou, X., et al. (2020). Loss of filamin C is catastrophic for heart function. Circulation 141 (10), 869–871. doi:10.1161/CIRCULATIONAHA.119.044061
Zou, P., Pinotsis, N., Lange, S., Song, Y. H., Popov, A., Mavridis, I., et al. (2006). Palindromic assembly of the giant muscle protein titin in the sarcomeric Z-disk. Nature 439 (7073), 229–233. doi:10.1038/nature04343
Keywords: pathogenic variant, Z-disk protein, cardiomyopathy, alpha-actinin, filamin C (FLNC), titin (TTN), myopalladin (MYPN), desmin (DES)
Citation: Noureddine M and Gehmlich K (2023) Structural and signaling proteins in the Z-disk and their role in cardiomyopathies. Front. Physiol. 14:1143858. doi: 10.3389/fphys.2023.1143858
Received: 13 January 2023; Accepted: 21 February 2023;
Published: 02 March 2023.
Edited by:
Emma Louise Robinson, University of Colorado, United StatesReviewed by:
Alex Christensen, University of Copenhagen, DenmarkMary Tsikitis, Biomedical Research Foundation of the Academy of Athens (BRFAA), Greece
Copyright © 2023 Noureddine and Gehmlich. This is an open-access article distributed under the terms of the Creative Commons Attribution License (CC BY). The use, distribution or reproduction in other forums is permitted, provided the original author(s) and the copyright owner(s) are credited and that the original publication in this journal is cited, in accordance with accepted academic practice. No use, distribution or reproduction is permitted which does not comply with these terms.
*Correspondence: Maya Noureddine, bW1uMjA3QHN0dWRlbnQuYmhhbS5hYy51aw==; Katja Gehmlich, ay5nZWhtbGljaEBiaGFtLmFjLnVr